- 1Aix Marseille Université, CEA, CNRS, Biosciences and Biotechnologies Institute of Aix-Marseille (BIAM), Equipe de Luminy de Génétique et Biophysique des Plantes, Marseille, F-13009, France
- 2EA2106 Biomolécules et Biotechnologies Végétales, Université de Tours, Tours, F-13009, France
As in other eukaryotes, the plant genome is functionally organized in two mutually exclusive chromatin fractions, a gene-rich and transcriptionally active euchromatin, and a gene-poor, repeat-rich, and transcriptionally silent heterochromatin. In Drosophila and humans, the molecular mechanisms by which euchromatin is preserved from heterochromatin spreading have been extensively studied, leading to the identification of insulator DNA elements and associated chromatin factors (insulator proteins), which form boundaries between chromatin domains with antagonistic features. In contrast, the identity of factors assuring such a barrier function remains largely elusive in plants. Nevertheless, several genomic elements and associated protein factors have recently been shown to regulate the spreading of chromatin marks across their natural boundaries in plants. In this minireview, we focus on recent findings that describe the spreading of chromatin and propose avenues to improve the understanding of how plant chromatin architecture and transitions between different chromatin domains are defined.
Introduction
It has been almost a century since the crucial role of chromatin functional compartmentation in gene expression was revealed by the study of the white mutation in Drosophila that causes white rather than red eye pigmentation. By using X-ray mutagenesis, Muller identified partial suppressors of white in which some facets of the eye remained white while others developed the red pigmentation, suggesting that the white allele itself was not mutated (Muller, 1930). It was much later shown that X-rays induced chromosomal rearrangements that resulted in a change of position of white from its original euchromatic position to the centromeric heterochromatin (Zhimulev et al., 1986, 1988; Umbetova et al., 1991). Heterochromatin is characterized by its high compactness and repressive epigenetic marks, which include DNA methylation and specific post-translation modifications of histones (or histone marks). DNA sequences in heterochromatin include many transposable elements (TEs) whose replication and reactivation are therefore prevented. Since Muller’s pioneering work, a series of studies have revealed that, besides the particular context of X-ray-induced chromosomal rearrangements, heterochromatin can spread stochastically into the adjacent euchromatin and effectively repress the expression of underlying genes. Some DNA sequences and associated protein modules that normally prevent heterochromatin spreading and maintain transitions between distinct chromatin domains have been identified and named “barrier insulators.” Insulator functions, however, encompass a wider range of mechanisms than the simple delimitation of antagonistic chromatin domains. Indeed, the term “insulator” is likewise attributed to factors that regulate the accessibility of enhancers and silencers to promoters or to factors participating in the structuration of DNA loops such as Topologically-Associated Domains (TAD; Ali et al., 2016). In this minireview, we focus on barrier insulators. We briefly introduce the establishment and maintenance of heterochromatin in plants (for a more complete review, see Law and Jacobsen, 2010), and subsequently present several loci within which a barrier activity is carried out in plants. Finally, we discuss several strategies aimed at increasing our knowledge of this understudied function in plants.
What can we learn from animal and yeast models about chromatin barrier formation in plants?
In animals and yeasts, the barrier function preventing the spreading of chromatin features beyond their natural boundaries relies on the presence of DNA sequences and their related DNA binding proteins such as the Transcription Factors for polymerase III B and C (TFIIIB, TFIIIC), which limit the spreading of the Silent Information Regulatory proteins (Sir) promoting the establishment of silenced chromatin in Saccharomyces cerevisiae (Sun et al., 2011). Similarly, the Upstream Stimulatory Factors 1 and 2 (USF1, USF2) bind the 5′HS4 insulator element and are necessary to avoid the spreading of neighboring heterochromatin in chicken erythrocytes (Ghirlando et al., 2012). Another example is the Heterochromatin Protein 1 (HP1), which binds H3K9me, a typical heterochromatin histone post-translational modification, and is required to prevent the spreading of H3K9me and H3K27me3 across natural boundaries in fungi (Stunnenberg et al., 2015; Jamieson et al., 2016). However, the unique homolog of HP1 in Arabidopsis, LHP1, regulates the deposition of H3K27me3 but does not prevent its spreading (Veluchamy et al., 2016). In general, no homologs of barrier elements described in animals and yeast have been reported to have similar functions in plants. Despite the absence of such barrier insulators in the literature, plant genomes have well-delineated euchromatin/heterochromatin compartmentation (Schoborg and Labrador, 2010). This raises the questions of the existence and identity of plant-specific barrier insulators.
Heterochromatin establishment and maintenance in plants
In plants, the histone modification H3K9me2 is a hallmark of heterochromatin. It is established and maintained by SUPPRESSORS OF VARIEGATION HOMOLOG (SUVH) histone methyltransferases (Ebbs et al., 2005). CHH and CHG (where H is any base except G) DNA methylation and H3K9me2 establishment were shown to be maintained through a positive feedback loop: SUVH proteins bind preferentially methylated non-CG cytosines and establish H3K9me2. Reciprocally, plant-specific CHROMOMETHYLASES 2 and 3 (CMT2/3) bind H3K9me2 and establish DNA methylation on CHH (CMT2) and CHG (CMT3) (Papa, 2001; Jackson et al., 2002, 2004; Johnson et al., 2007, 2014; Du et al., 2012; Stroud et al., 2014; Li et al., 2018b). Maintenance of CG methylation is ensured by the METHYLTRANSFERASE 1 (MET1; Cokus et al., 2008). De novo methylation of DNA (CG, CHG, and CHH) involves the RNA-directed DNA methylation pathway (RdDM) as well as the DOMAINS REARRANGED METHYLASE 1 and 2 (DRM1/2) proteins (Cao and Jacobsen, 2002a,b).
The initiation of the silencing of an active TE is mediated by non-canonical RdDM mechanisms based on the activity of RNA polymerase II (Nuthikattu et al., 2013): the RNA DEPENDENT RNA POLYMERASE 6 (RDR6) and the DICER LIKE 3 (DCL3) pathways. In Arabidopsis thaliana, the RDR6 pathway starts with the processing of TE mRNAs by RDR6 in order to produce a double-stranded RNA, which is cleaved by the endonucleases DCL2 and DCL4 (McCue et al., 2012). A pool of 21–22 nt siRNAs is thus generated and bound by the ARGONAUTE 1 (AGO1) protein (McCue et al., 2012), creating a complex able to bind the long non-coding RNAs (lncRNAs) produced in situ by Pol V. This complex recruits the DOMAINS REARRANGED METHYLTRANSFERASE 2 (DRM2), which catalyzes de novo cytosine methylation (Cao and Jacobsen, 2002b; Zhong et al., 2014). In the DCL3 pathway, the production of 24-nt siRNAs is independent of RDR2 and RDR6 and generated directly by DCL3, probably thanks to internal hairpin structures (Panda et al., 2016). Maintenance of TE silencing first depends on the addressing of Pol IV at H3K9me2 and unmethylated H3K4 sites. Following recruitment, Pol IV, with the help of RDR2, produces a pool of double-stranded precursor RNAs, which are then cleaved by DCL3 to generate 24 nt siRNAs (Law et al., 2011, 2013; Zhang et al., 2013; Li et al., 2015b; Singh et al., 2019). In the second phase, Pol V produces a second pool of lncRNAs which can associate with AGO4 or AGO6 loaded with the RNAs produced by Pol IV, thus allowing the recruitment of DRM2 (Wierzbicki et al., 2009; Matzke and Mosher, 2014; Duan et al., 2015). This model was recently refined after the demonstration that Pol IV transcripts can also guide the DNA methylation process in a DICER-independent manner at several loci (Yang et al., 2016; Ye et al., 2016; Kuo et al., 2017).
Heterochromatin spreading is counteracted by active DNA demethylation in Arabidopsis thaliana
Several studies have shown that plants are prone to heterochromatin spreading (Saze et al., 2008; Miura et al., 2009; Eichten et al., 2012; Lang et al., 2015; Noshay et al., 2019). However, there is still little evidence for the mechanisms involved in spreading heterochromatin into neighboring regions or, conversely, in preventing heterochromatin spreading by maintaining boundaries between different chromatin domains with antagonistic features. In Arabidopsis, mutations in RdDM factors suppressed the epidermal patterning defects observed in a mutant of the DNA glycosylase/lyase ROS1, a phenotype caused by the hypermethylation of the EPIDERMAL PATTERNING FACTOR 2 gene (Yamamuro et al., 2014). Another DNA glycosylase, DEMETER (DME), is required for the expression of imprinted genes localized near TEs, in the endosperm of Arabidopsis (Gehring et al., 2009). Mutations in DEMETER-Like (DML) 2, 3 and ROS1 are associated with increased DNA methylation of promoter and terminator regions (Lister et al., 2008). In addition, mutations in CMT3 and SUVHs suppressed the phenotypes resulting from the mutation of the gene encoding the H3K9 demethylase INCREASE IN BONZAI METHYLATION 1 (IBM1; Saze et al., 2008). RdDM and SUVH/CMT activities therefore appear to be counteracted by several DNA or histone demethylases as ROS1, DME, DMLs, and IBM1, but how these different antagonistic actions are orchestrated genome-wide is poorly understood. Nevertheless, a recent genetic screen revealed that mutants of Methyl-CpG-Binding Domain 7 (MBD7) and Increased DNA Methylation 3 (IDM3) fail to express a transgene composed of the sucrose transporter SUC2 coding sequence under the control of the 35S promoter. Subsequently, bisulfite sequencing analysis revealed that in a WT plant, 35S promoter accumulates DNA methylations in the region flanking the coding sequence of SUC2, whereas in mbd7 and idm3 mutants, the DNA methylations spread toward the 5′ region of the 35S promoter (Lang et al., 2015). In the model established by Lang and colleagues, MBD7 binds to mCG dense regions and interacts with IDM2 and IDM3. The complex composed of MBD7-IDM2-IDM3 would then recruit the histone acetyltransferase IDM1 to highly methylated loci, leading to in situ deposition of H3K23ac and H3K18ac marks, thus creating a permissive chromatin environment for ROS1 to locally remove the methylated cytosines in the regions bound by MBD7 (Lang et al., 2015; Figure 1). Subsequently, the base excision repair pathway, composed of the Apurinic/Apyrimidinic Endonucleases (APE1L and APE2) and the Zinc Finger DNA 3’ Phosphoesterase (ZDP), processes the excision product of the 5 mC and generates a 3’OH gap which is filled by an unknown DNA polymerase (Li et al., 2015c, 2018a; Figure 1). Thus, the combined action of these different actors is thought to prevent the diffusion of DNA methylations beyond their natural boundaries in plants. Very recently, the same genetic screen that allowed the identification of MBD7 has led to the discovery of another barrier factor, AGDP3. AGDP3 associates with H3K9me2 in a pH-dependent manner and protects several loci against ectopic DNA methylation. Since the targeting of ROS1 and subsequent demethylation of targeted loci appear to be dependent on AGDP3, it reinforces the idea that active demethylation acts downstream of the barrier activity (Zhou et al., 2021).
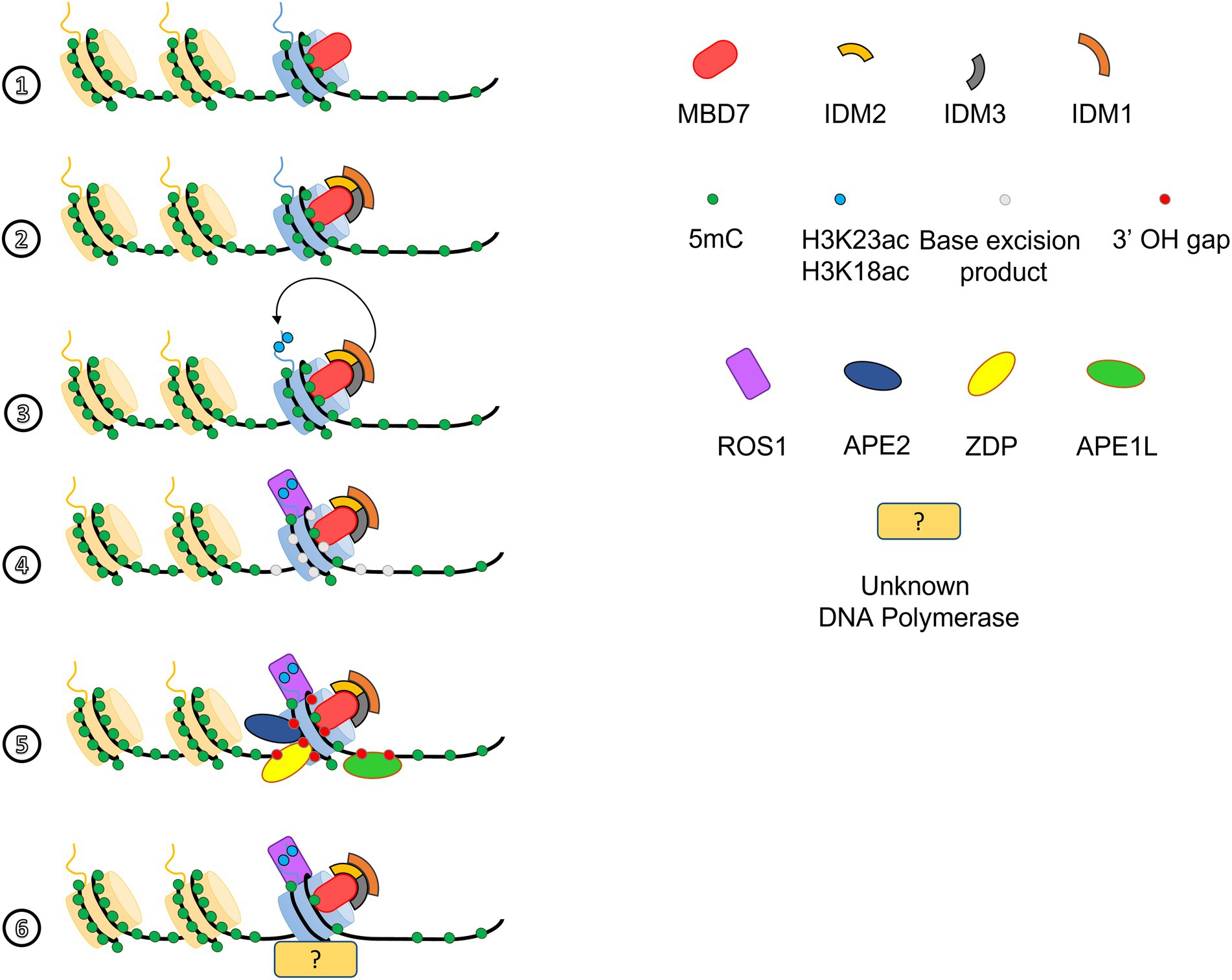
Figure 1. Methyl-CpG-Binding Domain 7 (MBD7), ROS1, and the base excision repair pathway act together to locally demethylate DNA. MBD7 binds highly methylated loci (1) and, through binding of IDM2 and IDM3, targets the histone methyl transferase IDM1 to mCG-dense regions (2). IDM1 ensures in situ deposition of H3K18ac and H3K23ac marks (3), which allows ROS1 to be addressed to hypermethylated regions (4; Lang et al., 2015). After removal of 5mC by ROS1 (4), the excision product can be processed by APE1L, APE2, and ZDP to create a 5’P-3’OH gap (5), which is then filled by a yet unknown DNA polymerase and sealed by DNA ligase I (6; Li et al., 2015c,d, 2018a).
Euchromatin islands
Euchromatin islands (EIs) were initially defined in humans as small euchromatin regions within large heterochromatin regions, which contain expressed genes, are depleted for H3K9me2, and are enriched for CTCF binding sites (Wen et al., 2012). Despite the absence of CTCF homologs, similar EI structures were described in Arabidopsis (Méteignier et al., 2022), rice (Wu et al., 2011), and barley (Baker et al., 2015) and represent ideal context to study heterochromatin spreading and identify barrier insulator elements. No consensus DNA sequences at the boundaries between EIs and the flanking heterochromatin regions, which could serve as barrier insulator sequences, were reported. However, chromatin state 8, an AT-rich heterochromatic state, less inaccessible and dense than the classic GC-rich heterochromatic state 9 (Sequeira-Mendes et al., 2014), was always present in the proximal borders of Arabidopsis EIs (Méteignier et al., 2022). In Arabidopsis, mutants of the topoisomerase VI (Topo VI) complex failed to express EI genes at their physiological levels. Strikingly, EIs are invaded by H3K9me2 in a hypomorphic Topo VI mutant, which suggests that Topo VI participates in a chromatin barrier function in Arabidopsis (Méteignier et al., 2022). Interestingly, the BRASSINOSTEROID INSENSITIVE 4 (BIN4) subunit of Topo VI directly interacts with METHIONINE ADENOSYL TRANSFERASE 3 (MAT3), the major methyl donor of methylation reactions (Meng et al., 2018; Méteignier et al., 2022). In addition, MAT3 was required for H3K9me2 deposition at TEs, and Topo VI was required to exclude MAT3 from EIs, thereby providing a possible mechanistic explanation to the participation of Topo VI in a barrier function (Figure 2). The spreading of H3K9me2 over EIs was, however, not accompanied by an increase in cytosine methylation levels in CHG or CHH contexts, suggesting that Topo VI is involved in controlling the spreading of some chromatin marks rather than heterochromatin itself (Méteignier et al., 2022). This uncoupling between H3K9me2 and DNA methylation has been observed in several other specific contexts. For instance, in triploid Arabidopsis seeds, small euchromatic AT-rich TEs overaccumulate H3K9me2 but lose CHH methylation (no change in CHG nor CG), which correlates with altered expression of neighboring genes (Jiang et al., 2017). This result suggests that H3K9me2 may repress RdDM, as it was observed in another independent study (Zemach et al., 2013). Another study in Eutrema salsugineum, which lacks CMT3 and gene body cytosine methylation, has highlighted a similar disruption of the reinforcement loops between H3K9me2 and non-CG methylation. In this organism, the ectopic expression of AtCMT3 increased CHG methylation over repetitive sequences containing pre-existing H3K9me2. Additionally, ectopic gene body CHG methylations at loci devoid of pre-existing H3K9me2 were also reported. In the latter case, the CHG methylations were stably inherited through generations, but did not lead to subsequent induction of H3K9me2 deposition (Wendte et al., 2019). Hence, in the context of Topo VI-dependent barrier, H3K9me2 might also be established and maintained in a CHG-independent manner by unknown histone methyl transferases.
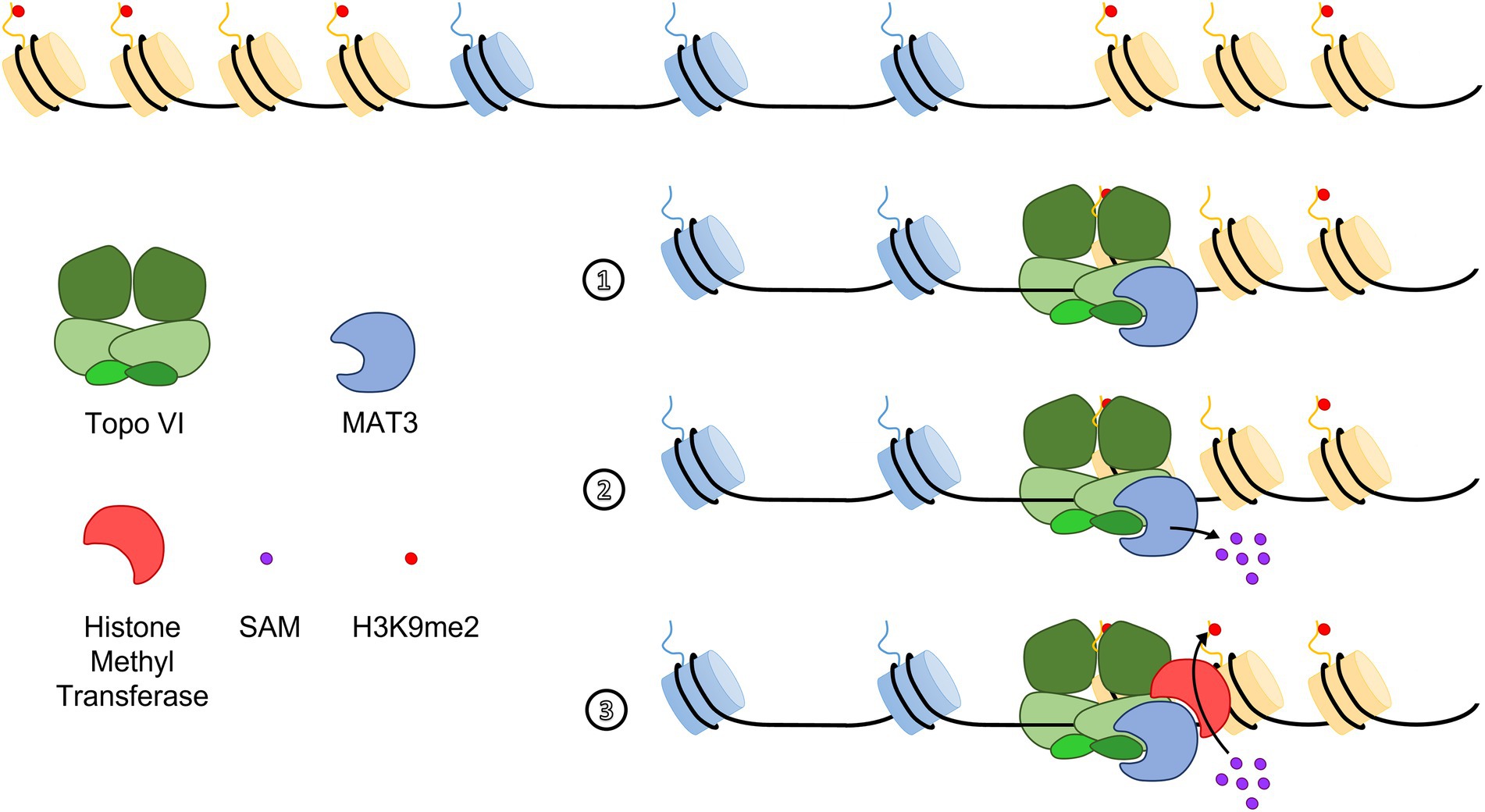
Figure 2. A hypothetical model for topoisomerase VI (Topo VI)-dependent insulation at Euchromatin Islands. The BIN4 subunit of Topo VI interacts with METHIONINE ADENOSYL TRANSFERASE 3 (MAT3) and is required to exclude MAT proteins from EIs (1). MAT3 localization at heterochromatin edges would allow local production of S-Adenosyl Methionine [SAM, (2)], the main methyl donor in methylation reactions, which is processed by a yet unknown histone methyltransferase to ensure in situ deposition of the repressive mark H3K9me2 in heterochromatin regions (3; Méteignier et al., 2022).
mCHH islands
In the heterochromatin-rich maize genome, gene-flanking regions are heavily methylated in CHH context and were therefore called mCHH islands (Gent et al., 2013). Mutation in the RDR2 ortholog MOP1 decreased the production of 24-nt siRNAs by 100-fold and altered the formation of mCHH islands (Nobuta et al., 2008; Gent et al., 2014), revealing the important role of RdDM for CHH methylation in maize, which lacks known orthologs of CMT2 (Bewick et al., 2017). Epigenome mapping of maize revealed that mCHH islands are specific to transition zones between CG/CHG/H3K9me2-rich intergenic regions and H3K4me3-rich gene extremities. In addition, the same study suggested increased chromatin accessibility around promoters and terminators of genes adjacent to mCHH islands (Li et al., 2015a). These studies thus identified mCHH islands as good candidates for barrier insulator elements. Interestingly, methylome analyses of mop1 and mop3 mutants revealed that loss of mCHH islands was not associated with altered expression of neighboring genes, but rather with decreased CG/CHG methylation and elevated expression of adjacent TEs. This suggests that mCHH islands are essential for preserving silent TEs from activation by euchromatin spreading (Li et al., 2015a). Reinforcing this idea, a recent study reports that disruption of mop1 leads to loss of DNA methylation at TEs which are then susceptible to reactivation upon heat stress (Guo et al., 2021). Since then, mCHH islands have been identified in the genomes of many angiosperms (Zemach et al., 2010; Niederhuth et al., 2016; Corem et al., 2018; Martin et al., 2021). In Poaceae, mCHH islands are enriched at the extremities of 39% of genes, particularly at their 5′ extremity in smaller genomes. Among gene expression, gene length, distance from a TE, and gene body methylation, TE proximity and absence of gene body methylation were the strongest predictors for the presence of 5′ CHH islands (Martin et al., 2021). In Arabidopsis, the edges of long heterochromatic TEs are hypermethylated in CHH context and siRNAs overaccumulate at these positions (Zemach et al., 2013). Thus, mCHH islands could exert a similar function in very different genomes by establishing nearby TEs. Methylation in CHH context increases locally in the proximity of genes and overlaps almost exclusively with neighboring TEs in response to phosphate starvation in rice. Moreover, the large majority of genes containing differentially methylated regions after a long-term phosphate starvation are upregulated (Secco et al., 2015). A similar result has recently been observed in tomato (Tian et al., 2021). Such a dynamic appearance of mCHH islands in the vicinity of highly expressed genes neighboring TEs is consistent with the hypothesis that mCHH islands prevent euchromatin spreading to adjacent TEs. Thus, mCHH islands might constitute a conserved epigenetic response aimed at protecting plant genomes against opportunistic reactivation of TEs during stress exposure. However, to assert that mCHH islands constitute a genuine and widespread barrier against euchromatin spreading, further studies are required, such as the determination of H3K4me3 distribution in the neighborhood of mCHH islands in mop mutants. In addition to their likely barrier function, mCHH islands also promote the expression of genes in the vicinity to TEs through the sequential recruitment of SUVH and DNAJ domain-containing proteins (Harris et al., 2018), raising the question of the causality relationship between mCHH islands and gene expression.
Are there common features in DNA loop formation and chromatin barriers in plants?
The interplay between the different insulator functions, such as enhancer-blocking, TAD structuration, and chromatin barrier, is rarely discussed in plants. In animals, repetitive sequences, such as tRNA genes, MIR, and Gyspy TEs, are found close to TADs and provide insulator sequences (She et al., 2010; Raab et al., 2012; Van Bortle and Corces, 2012; Wang et al., 2015). In contrast, plant TAD borders are enriched for specific transcription factor-binding sites. For instance in rice and Marchantia polymorpha, borders of a type of TADs are enriched for a TCP (Teosinte Branched1/Cycloidea/Proliferating cell factor) binding motif (Liu et al., 2017; Karaaslan et al., 2020). However, TCP is not required for TAD formation in Marchantia (Karaaslan et al., 2020), suggesting that TAD formation is complex and redundantly controlled in plants. Indeed, in maize, single-cell chromatin accessibility mapping has revealed that TCP-binding sites are enriched in co-accessible cis-regulatory regions, which corresponded to the borders of chromatin loops. Other transcription factor-binding sites were found at a similar location, such as APETALA2/ETHYLENE-RESPONSIVE ELEMENT BINDING PROTEINS and LATERAL ORGAN BOUNDARIES DOMAIN, all representing GC-rich motifs (Marand et al., 2021). Recently, the OSH1 transcription factor, which binds the RS2-9 DNA element in rice, was proposed to act as an insulator module (Liu et al., 2022). Although this module was able to block an enhancer-promoter interaction, histone marks and TAD changes were not investigated in an osh1 mutant. In euchromatin and at a more local scale, the BORDER plant-specific proteins are enriched at gene transcription start and end sites and are required to prevent transcriptional interference between neighboring genes (Yu et al., 2019). Taken together, these recent findings suggest potential candidates for a plant insulator function, which remains to be extensively characterized by histone modification analysis and chromatin contact mapping in mutant plants.
Discussion
The mechanisms initiating the diffusion of heterochromatin in plants are currently unclear. Heterochromatin spreading observed in ros1 is highly dependent on RdDM (He et al., 2009; Yamamuro et al., 2014). However, in the context of EIs, SUVH proteins can also play a minor role in the diffusion of chromatin marks (Méteignier et al., 2022). In maize, where the vicinity of several TEs is subjected to the diffusion of heterochromatin marks (Noshay et al., 2019), neither the mutation of MOP1, a component of RdDM, nor the mutation of MET2, the chromomethylase involved in mCHG maintenance, seem to alter methylation levels in the neighborhood of these TEs (Eichten et al., 2012). Thus, there is currently no consensus on the molecular bases underlying heterochromatin spreading in plants.
The apparent absence of homologs of well-known animal insulators in plants highlighted those plants seem to use specific protein-DNA modules as well as lineage-specific proteins to ensure insulator function. Studying the barrier function in different plant models with a wide range of genome size and structure could allow a better understanding of insulation and insulator dynamics. For instance, the Topo VI-dependent barrier function at the edges of euchromatin islands has only been highlighted in Arabidopsis (Méteignier et al., 2022) and would benefit from further study in other organisms. Since Topo VI is encoded in all known plant genomes, designing a Topo VI hypomorphic or conditional mutant in maize, for instance, and studying the genome-wide distribution of H3K9me2 and other chromatin marks could provide a better insight into how genes insulate away from the adjacent TE-rich heterochromatin. Additionally, the proposed model of barrier mechanism mediated by Topo VI is incomplete. First, the physical position of Topo VI in the context of EIs and whether Topo VI binds specific DNA sequences remain elusive. However, it should be noted that in animals, topoisomerases are prone to recognize and bind particular DNA structures rather than defined DNA sequences (René et al., 2007). Moreover, the human Top IIα is addressed to the centromeres by interacting with the phosphorylated form of histone H2A at Thr 120 during mitosis (Zhang et al., 2020). These observations raise the possibility that Topo VI barrier function might also be independent of a specific nucleotide sequence. Furthermore, the putative histone methyl transferase involved in the Topo VI-controlled H3K9me2 deposition is unknown. The accumulation of RNA-seq and ChIP-seq data could make it possible to consider a data-mining approach to identify the missing factors whose mutation would also result, like Topo VI, in the repression of EI genes and changes in chromatin mark levels in EIs.
Like the barrier model involving Topo VI, the active demethylation pathway is still incomplete. Indeed, the DNA polymerase that fills the 5’P-3’OH gap resulting from the activity of the base excision repair mechanism with an unmethylated cytosine remains unknown. The discovery of the yet unidentified factors participating to the active demethylation pathway, or the Topo VI-mediated chromatin barrier, could be achieved by proximity labeling strategies allowing the identification of very transient interactions (Arora et al., 2020). Moreover, the power of the CRISPR-CAS9-based gene editing could allow inserting reporter genes within EIs, then use them in genetic screens aimed at identifying mutant lines defective in EI gene expression and thus in putative new players involved in heterochromatin containment.
Author contributions
All authors listed have made a substantial, direct, and intellectual contribution to the work and approved it for publication.
Funding
Work by CL was supported by the French National Research Agency (ANR-14-CE02-0010). FV is a recipient of a PhD fellowship from the French Ministry of Higher Education, Research and Innovation. Work by LVM was supported by the French National Research Agency (ANR-20-CE43-0010).
Acknowledgments
We thank Ben Field for critical reading of the manuscript. We apologize for not citing all the relevant primary research articles and reviews due to space limitations.
Conflict of interest
The authors declare that the research was conducted in the absence of any commercial or financial relationships that could be construed as a potential conflict of interest.
Publisher’s note
All claims expressed in this article are solely those of the authors and do not necessarily represent those of their affiliated organizations, or those of the publisher, the editors and the reviewers. Any product that may be evaluated in this article, or claim that may be made by its manufacturer, is not guaranteed or endorsed by the publisher.
References
Ali, T., Renkawitz, R., and Bartkuhn, M. (2016). Insulators and domains of gene expression. Curr. Opin. Genet. Dev. 37, 17–26. doi: 10.1016/j.gde.2015.11.009
Arora, D., Abel, N. B., Liu, C., van Damme, P., Yperman, K., Eeckhout, D., et al. (2020). Establishment of proximity-dependent biotinylation approaches in different plant model systems. Plant Cell 32, 3388–3407. doi: 10.1105/TPC.20.00235
Baker, K., Dhillon, T., Colas, I., Cook, N., Milne, I., Milne, L., et al. (2015). Chromatin state analysis of the barley epigenome reveals a higher-order structure defined by H3K27me1 and H3K27me3 abundance. Plant J. 84, 111–124. doi: 10.1111/tpj.12963
Bewick, A. J., Niederhuth, C. E., Ji, L., Rohr, N. A., Griffin, P. T., Leebens-Mack, J., et al. (2017). The evolution of CHROMOMETHYLASES and gene body DNA methylation in plants. Genome Biol. 18, 65–13. doi: 10.1186/s13059-017-1195-1
Cao, X., and Jacobsen, S. E. (2002a). Locus-specific control of asymmetric and CpNpG methylation by the DRM and CMT3 methyltransferase genes. Proc. Natl. Acad. Sci. 99, 16491–16498. doi: 10.1073/pnas.162371599
Cao, X., and Jacobsen, S. E. (2002b). Role of the Arabidopsis DRM Methyltransferases in De novo DNA methylation and gene silencing. Curr. Biol. 12, 1138–1144. doi: 10.1016/S0960-9822(02)00925-9
Cokus, S. J., Feng, S., Zhang, X., Chen, Z., Merriman, B., Haudenschild, C. D., et al. (2008). Shotgun bisulphite sequencing of the Arabidopsis genome reveals DNA methylation patterning. Nature 452, 215–219. doi: 10.1038/nature06745
Corem, S., Doron-Faigenboim, A., Jouffroy, O., Maumus, F., Arazi, T., and Bouché, N. (2018). Redistribution of CHH methylation and small interfering RNAs across the genome of tomato ddm1 mutants. Plant Cell 30, 1628–1644. doi: 10.1105/tpc.18.00167
Du, J., Zhong, X., Bernatavichute, Y. V., Stroud, H., Feng, S., Caro, E., et al. (2012). Dual binding of Chromomethylase domains to H3K9me2-containing nucleosomes directs DNA methylation in plants. Cell 151, 167–180. doi: 10.1016/j.cell.2012.07.034
Duan, C., Zhang, H., Tang, K., Zhu, X., Qian, W., Hou, Y., et al. (2015). Specific but interdependent functions for A rabidopsis AGO 4 and AGO 6 in RNA-directed DNA methylation. EMBO J. 34, 581–592. doi: 10.15252/embj.201489453
Ebbs, M. L., Bartee, L., and Bender, J. (2005). H3 lysine 9 methylation is maintained on a transcribed inverted repeat by combined action of SUVH6 and SUVH4 Methyltransferases. Mol. Cell. Biol. 25, 10507–10515. doi: 10.1128/mcb.25.23.10507-10515.2005
Eichten, S. R., Ellis, N. A., Makarevitch, I., Yeh, C. T., Gent, J. I., Guo, L., et al. (2012). Spreading of heterochromatin is limited to specific families of maize Retrotransposons. PLoS Genet. 8, e1003127. doi: 10.1371/journal.pgen.1003127
Gehring, M., Bubb, K. L., and Henikoff, S. (2009). Extensive demethylation of repetitive elements during seed development underlies gene imprinting. Science 324, 1447–1451. doi: 10.1126/science.1171609
Gent, J. I., Ellis, N. A., Guo, L., Harkess, A. E., Yao, Y., Zhang, X., et al. (2013). CHH islands: De novo DNA methylation in near-gene chromatin regulation in maize. Genome Res. 23, 628–637. doi: 10.1101/gr.146985.112
Gent, J. I., Madzima, T. F., Bader, R., Kent, M. R., Zhang, X., Stam, M., et al. (2014). Accessible DNA and relative depletion of H3K9me2 at maize loci undergoing RNA-directed DNA methylation. Plant Cell 26, 4903–4917. doi: 10.1105/tpc.114.130427
Ghirlando, R., Giles, K., Gowher, H., Xiao, T., Xu, Z., Yao, H., et al. (2012). Chromatin domains, insulators, and the regulation of gene expression. Biochim. Biophys. Acta Gene Regul. Mech. 1819, 644–651. doi: 10.1016/j.bbagrm.2012.01.016
Guo, W., Wang, D., and Lisch, D. (2021). RNA-directed DNA methylation prevents rapid and heritable reversal of transposon silencing under heat stress in Zea mays. PLoS Genet. 17, e1009326–e1009328. doi: 10.1371/journal.pgen.1009326
Harris, C. J., Scheibe, M., Wongpalee, S. P., Liu, W., Cornett, E. M., Vaughan, R. M., et al. (2018). A DNA methylation reader complex that enhances gene transcription. Science 362, 1182–1186. doi: 10.1126/science.aar7854
He, X. J., Hsu, Y. F., Pontes, O., Zhu, J., Lu, J., Bressan, R. A., et al. (2009). NRPD4, a protein related to the RPB4 subunit of RNA polymerase II, is a component of RNA polymerases IV and v and is required for RNA-directed DNA methylation. Genes Dev. 23, 318–330. doi: 10.1101/gad.1765209
Jackson, J. P., Johnson, L., Jasencakova, Z., Zhang, X., PerezBurgos, L., Singh, P. B., et al. (2004). Dimethylation of histone H3 lysine 9 is a critical mark for DNA methylation and gene silencing in Arabidopsis thaliana. Chromosoma 112, 308–315. doi: 10.1007/s00412-004-0275-7
Jackson, J. P., Lindroth, A. M., Cao, X., and Jacobsen, S. E. (2002). Control of CpNpG DNA methylation by the KRYPTONITE histone H3 methyltransferase. Nature 416, 556–560. doi: 10.1038/nature731
Jamieson, K., Wiles, E. T., McNaught, K. J., Sidoli, S., Leggett, N., Shao, Y., et al. (2016). Loss of HP1 causes depletion of H3K27me3 from facultative heterochromatin and gain of H3K27me2 at constitutive heterochromatin. Genome Res. 26, 97–107. doi: 10.1101/gr.194555.115
Jiang, H., Moreno-Romero, J., Santos-González, J., De Jaeger, G., Gevaert, K., Van De Slijke, E., et al. (2017). Ectopic application of the repressive histone modification H3K9me2 establishes post-zygotic reproductive isolation in Arabidopsis thaliana. Genes Dev. 31, 1272–1287. doi: 10.1101/gad.299347.117
Johnson, L. M., Bostick, M., Zhang, X., Kraft, E., Henderson, I., Callis, J., et al. (2007). The SRA methyl-cytosine-binding domain links DNA and histone methylation. Curr. Biol. 17, 379–384. doi: 10.1016/j.cub.2007.01.009
Johnson, L. M., Du, J., Hale, C. J., Bischof, S., Feng, S., Chodavarapu, R. K., et al. (2014). SRA-and SET-domain-containing proteins link RNA polymerase V occupancy to DNA methylation. Nature 507, 124–128. doi: 10.1038/nature12931
Karaaslan, E. S., Wang, N., Faiß, N., Liang, Y., Montgomery, S. A., Laubinger, S., et al. (2020). Marchantia TCP transcription factor activity correlates with three-dimensional chromatin structure. Nat. Plants 6, 1250–1261. doi: 10.1038/s41477-020-00766-0
Kuo, H. Y., Jacobsen, E. L., Long, Y., Chen, X., and Zhai, J. (2017). Characteristics and processing of pol IV-dependent transcripts in Arabidopsis. J. Genet. Genomics 44, 3–6. doi: 10.1016/j.jgg.2016.10.009
Lang, Z., Lei, M., Wang, X., Tang, K., Miki, D., Zhang, H., et al. (2015). The methyl-CpG-binding protein MBD7 facilitates active DNA Demethylation to limit DNA hyper-methylation and transcriptional gene silencing. Mol. Cell 57, 971–983. doi: 10.1016/j.molcel.2015.01.009
Law, J. A., Du, J., Hale, C. J., Feng, S., Krajewski, K., Palanca, A. M. S., et al. (2013). Polymerase IV occupancy at RNA-directed DNA methylation sites requires SHH1. Nature 498, 385–389. doi: 10.1038/nature12178
Law, J. A., and Jacobsen, S. E. (2010). Establishing, maintaining and modifying DNA methylation patterns in plants and animals. Nat. Rev. Genet. 11, 204–220. doi: 10.1038/nrg2719
Law, J. A., Vashisht, A. A., Wohlschlegel, J. A., and Jacobsen, S. E. (2011). SHH1, a Homeodomain protein required for DNA methylation, as well as RDR2, RDM4, and chromatin remodeling factors, associate with RNA polymerase IV. PLoS Genet. 7, e1002195. doi: 10.1371/journal.pgen.1002195
Li, Y., Córdoba-Cañero, D., Qian, W., Zhu, X., Tang, K., Zhang, H., et al. (2015c). An AP endonuclease functions in active DNA Dimethylation and gene imprinting in Arabidopsis. PLoS Genet. 11, e1004905. doi: 10.1371/journal.pgen.1004905
Li, Y., Duan, C. G., Zhu, X., Qian, W., and Zhu, J. K. (2015d). A DNA ligase required for active DNA demethylation and genomic imprinting in Arabidopsis. Cell Res. 25, 757–760. doi: 10.1038/cr.2015.45
Li, Q., Gent, J. I., Zynda, G., Song, J., Makarevitch, I., Hirsch, C. D., et al. (2015a). RNA-directed DNA methylation enforces boundaries between heterochromatin and euchromatin in the maize genome. Proc. Natl. Acad. Sci. U. S. A. 112, 14728–14733. doi: 10.1073/pnas.1514680112
Li, X., Jake Harris, C., Zhong, Z., Chen, W., Liu, R., Jia, B., et al. (2018b). Mechanistic insights into plant SUVH family H3K9 methyltransferases and their binding to context-biased non-CG DNA methylation. Proc. Natl. Acad. Sci. U. S. A. 115, E8793–E8802. doi: 10.1073/pnas.1809841115
Li, J., Liang, W., Li, Y., and Qian, W. (2018a). APURINIC/APYRIMIDINIC ENDONUCLEASE2 AND ZINC FINGER DNA 3′-PHOSPHOESTERASE play overlapping roles in the maintenance of epigenome and genome stability. Plant Cell 30, 1954–1970. doi: 10.1105/tpc.18.00287
Li, S., Vandivier, L. E., Tu, B., Gao, L., Won, S. Y., Li, S., et al. (2015b). Detection of pol IV/RDR2-dependent transcripts at the genomic scale in arabidopsis reveals features and regulation of sirna biogenesis. Genome Res. 25, 235–245. doi: 10.1101/gr.182238.114
Lister, R., O’Malley, R. C., Tonti-Filippini, J., Gregory, B. D., Berry, C. C., Millar, A. H., et al. (2008). Highly integrated single-base resolution maps of the Epigenome in Arabidopsis. Cell 133, 523–536. doi: 10.1016/j.cell.2008.03.029
Liu, C., Cheng, Y.-J., Wang, J.-W., and Weigel, D. (2017). Prominent topologically associated domains differentiate global chromatin packing in rice from Arabidopsis. Nat. Plants 3, 742–748. doi: 10.1038/s41477-017-0005-9
Liu, H., Jiang, L., Wen, Z., Yang, Y., Singer, S. D., Bennett, D., et al. (2022). Rice RS2-9, which is bound by transcription factor OSH1, blocks enhancer–promoter interactions in plants. Plant J. 109, 541–554. doi: 10.1111/TPJ.15574
Marand, A. P., Chen, Z., Gallavotti, A., and Schmitz, R. J. (2021). A cis-regulatory atlas in maize at single-cell resolution. Cell 184, 3041–3055.e21. doi: 10.1016/j.cell.2021.04.014
Martin, G. T., Seymour, D. K., and Gaut, B. S. (2021). CHH Methylation Islands: a nonconserved feature of grass genomes that is positively associated with transposable elements but negatively associated with gene-body methylation. Genome Biol. Evol. 13, 1–16. doi: 10.1093/gbe/evab144
Matzke, M. A., and Mosher, R. A. (2014). RNA-directed DNA methylation: an epigenetic pathway of increasing complexity. Nat. Rev. Genet. 15, 394–408. doi: 10.1038/nrg3683
McCue, A. D., Nuthikattu, S., Reeder, S. H., and Slotkin, R. K. (2012). Gene expression and stress response mediated by the epigenetic regulation of a transposable element small RNA. PLoS Genet. 8, e1002474. doi: 10.1371/journal.pgen.1002474
Meng, J., Wang, L., Wang, J., Zhao, X., Cheng, J., Yu, W., et al. (2018). METHIONINE ADENOSYLTRANSFERASE4 mediates DNA and histone methylation. Plant Physiol. 177, 652–670. doi: 10.1104/pp.18.00183
Méteignier, L.-V., Lecampion, C., Velay, F., Vriet, C., Dimnet, L., Térèse, M., et al. (2022). Topoisomerase VI participates in an insulator-like function that prevents H3K9me2 spreading into euchromatic islands. Proc. Natl. Acad. Sci. U. S. A. 119:e2001290119. doi: 10.1073/pnas.2001290119
Miura, A., Nakamura, M., Inagaki, S., Kobayashi, A., Saze, H., and Kakutani, T. (2009). An Arabidopsis jmjC domain protein protects transcribed genes from DNA methylation at CHG sites. EMBO J. 28, 1078–1086. doi: 10.1038/emboj.2009.59
Muller, H. J. (1930). Types of visible variations induced by X-rays in drosophila. J. Genet. 22, 299–334. doi: 10.1007/BF02984195
Niederhuth, C. E., Bewick, A. J., Ji, L., Alabady, M. S., Do Kim, K., Li, Q., et al. (2016). Widespread natural variation of DNA methylation within angiosperms. Genome Biol. 17, 194–119. doi: 10.1186/s13059-016-1059-0
Nobuta, K., Lu, C., Shrivastava, R., Pillay, M., De Paoli, E., Accerbi, M., et al. (2008). Distinct size distribution of endogenous siRNAs in maize: evidence from deep sequencing in the mop1-1 mutant. Proc. Natl. Acad. Sci. U. S. A. 105, 14958–14963. doi: 10.1073/pnas.0808066105
Noshay, J. M., Anderson, S. N., Zhou, P., Ji, L., Ricci, W., Lu, Z., et al. (2019). Monitoring the interplay between transposable element families and DNA methylation in maize. PLoS Genet. 15, e1008291–e1008225. doi: 10.1371/journal.pgen.1008291
Nuthikattu, S., McCue, A. D., Panda, K., Fultz, D., DeFraia, C., Thomas, E. N., et al. (2013). The initiation of epigenetic silencing of active transposable elements is triggered by RDR6 and 21-22 nucleotide small interfering RNAs. Plant Physiol. 162, 116–131. doi: 10.1104/pp.113.216481
Panda, K., Ji, L., Neumann, D. A., Daron, J., Schmitz, R. J., and Slotkin, R. K. (2016). Full-length autonomous transposable elements are preferentially targeted by expression-dependent forms of RNA-directed DNA methylation. Genome Biol. 17, 170–119. doi: 10.1186/s13059-016-1032-y
Papa, C. M. (2001). Maize Chromomethylase Zea methyltransferase2 is required for CpNpG methylation. Plant Cell Online 13, 1919–1928. doi: 10.1105/tpc.13.8.1919
Raab, J. R., Chiu, J., Zhu, J., Katzman, S., Kurukuti, S., Wade, P. A., et al. (2012). Human tRNA genes function as chromatin insulators. EMBO J. 31, 330–350. doi: 10.1038/emboj.2011.406
René, B., Fermandjian, S., and Mauffret, O. (2007). Does topoisomerase II specifically recognize and cleave hairpins, cruciforms and crossovers of DNA? Biochimie 89, 508–515. doi: 10.1016/j.biochi.2007.02.011
Saze, H., Shiraishi, A., Miura, A., and Kakutani, T. (2008). Control of genic DNA methylation by a jmjC domain-containing protein in Arabidopsis thaliana. Science 319, 462–465. doi: 10.1126/science.1150987
Schoborg, T. A., and Labrador, M. (2010). The phylogenetic distribution of non-CTCF insulator proteins is limited to insects and reveals that beaf-32 is drosophila lineage specific. J. Mol. Evol. 70, 74–84. doi: 10.1007/s00239-009-9310-x
Secco, D., Wang, C., Shou, H., Schultz, M. D., Chiarenza, S., Nussaume, L., et al. (2015). Stress induced gene expression drives transient DNA methylation changes at adjacent repetitive elements. elife 4, 1–26. doi: 10.7554/elife.09343
Sequeira-Mendes, J., Aragüez, I., Peiró, R., Mendez-Giraldez, R., Zhang, X., Jacobsen, S. E., et al. (2014). The functional topography of the Arabidopsis genome is organized in a reduced number of linear motifs of chromatin states. Plant Cell 26, 2351–2366. doi: 10.1105/tpc.114.124578
She, W., Lin, W., Zhu, Y., Chen, Y., Jin, W., Yang, Y., et al. (2010). The gypsy insulator of Drosophila melanogaster, together With its binding protein suppressor of hairy-wing, facilitate high and precise expression of transgenes in Arabidopsis thaliana. Genetics 185, 1141–1150. doi: 10.1534/genetics.110.117960
Singh, J., Mishra, V., Wang, F., Huang, H. Y., and Pikaard, C. S. (2019). Reaction mechanisms of pol IV, RDR2, and DCL3 drive RNA channeling in the siRNA-directed DNA methylation pathway. Mol. Cell 75, 576–589.e5. doi: 10.1016/j.molcel.2019.07.008
Stroud, H., Do, T., Du, J., Zhong, X., Feng, S., Johnson, L., et al. (2014). Non-CG methylation patterns shape the epigenetic landscape in Arabidopsis. Nat. Struct. Mol. Biol. 21, 64–72. doi: 10.1038/nsmb.2735
Stunnenberg, R., Kulasegaran-Shylini, R., Keller, C., Kirschmann, M. A., Gelman, L., and Buhler, M. (2015). H3K9 methylation extends across natural boundaries of heterochromatin in the absence of an HP1 protein. EMBO J. 34, 2789–2803. doi: 10.15252/embj.201591320
Sun, J. Q., Hatanaka, A., and Oki, M. (2011). Boundaries of transcriptionally silent chromatin in Saccharomyces cerevisiae. Genes Genet. Syst. 86, 73–81. doi: 10.1266/ggs.86.73
Tian, P., Lin, Z., Lin, D., Dong, S., Huang, J., and Huang, T. (2021). The pattern of DNA methylation alteration, and its association with the changes of gene expression and alternative splicing during phosphate starvation in tomato. Plant J. 108, 841–858. doi: 10.1111/tpj.15486
Umbetova, G. H., Belyaeva, E. S., Baricheva, E. M., and Zhimulev, I. F. (1991). Cytogenetic and molecular aspects of position effect variegation in Drosophila melanogaster. Chromosoma 101, 55–61. doi: 10.1007/BF00360687
Van Bortle, K., and Corces, V. G. (2012). tDNA insulators and the emerging role of TFIIIC in genome organization. Transcription 3, 277–284. doi: 10.4161/trns.21579
Veluchamy, A., Jégu, T., Ariel, F., Latrasse, D., Mariappan, K. G., Kim, S.-K., et al. (2016). LHP1 regulates H3K27me3 spreading and shapes the three-dimensional conformation of the Arabidopsis genome. PLoS One 11:e0158936. doi: 10.1371/journal.pone.0158936
Wang, J., Vicente-García, C., Seruggia, D., Moltó, E., Fernandez-Miñán, A., Neto, A., et al. (2015). MIR retrotransposon sequences provide insulators to the human genome. Proc. Natl. Acad. Sci. 112, E4428–E4437. doi: 10.1073/pnas.1507253112
Wen, B., Wu, H., Loh, Y. H., Briem, E., Daley, G. Q., and Feinberg, A. P. (2012). Euchromatin islands in large heterochromatin domains are enriched for CTCF binding and differentially DNA-methylated regions. BMC Genomics 13:566. doi: 10.1186/1471-2164-13-566
Wendte, J. M., Zhang, Y., Ji, L., Shi, X., Hazarika, R. R., Shahryary, Y., et al. (2019). Epimutations are associated with CHROMOMETHYLASE 3-induced de novo DNA methylation. elife 8, 1–27. doi: 10.7554/eLife.47891
Wierzbicki, A. T., Ream, T. S., Haag, J. R., and Pikaard, C. S. (2009). RNA polymerase v transcription guides ARGONAUTE4 to chromatin. Nat. Genet. 41, 630–634. doi: 10.1038/ng.365
Wu, Y., Kikuchi, S., Yan, H., Zhang, W., Rosenbaum, H., Iniguez, A. L., et al. (2011). Euchromatic subdomains in rice centromeres are associated with genes and transcription. Plant Cell 23, 4054–4064. doi: 10.1105/tpc.111.090043
Yamamuro, C., Miki, D., Zheng, Z., Ma, J., Wang, J., Yang, Z., et al. (2014). Overproduction of stomatal lineage cells in Arabidopsis mutants defective in active DNA demethylation. Nat. Commun. 5, 4062. doi: 10.1038/ncomms5062
Yang, D. L., Zhang, G., Tang, K., Li, J., Yang, L., Huang, H., et al. (2016). Dicer-independent RNA-directed DNA methylation in Arabidopsis. Cell Res. 26, 66–82. doi: 10.1038/cr.2015.145
Ye, R., Chen, Z., Lian, B., Rowley, M. J., Xia, N., Chai, J., et al. (2016). A Dicer-independent route for biogenesis of siRNAs that direct DNA methylation in Arabidopsis. Mol. Cell 61, 222–235. doi: 10.1016/j.molcel.2015.11.015
Yu, X., Martin, P. G. P., and Michaels, S. D. (2019). BORDER proteins protect expression of neighboring genes by promoting 3′ pol II pausing in plants. Nat. Commun. 10, 4359–4315. doi: 10.1038/s41467-019-12328-w
Zemach, A., Kim, M. Y., Hsieh, P.-H., Coleman-Derr, D., Eshed-Williams, L., Thao, K., et al. (2013). The Arabidopsis nucleosome remodeler DDM1 allows DNA Methyltransferases to access H1-containing heterochromatin. Cell 153, 193–205. doi: 10.1016/j.cell.2013.02.033
Zemach, A., McDaniel, I. E., Silva, P., and Zilberman, D. (2010). Genome-wide evolutionary analysis of eukaryotic DNA methylation. Science 328, 916–919. doi: 10.1126/science.1186366
Zhang, M., Liang, C., Chen, Q., Yan, H., Xu, J., Zhao, H., et al. (2020). Histone H2A phosphorylation recruits topoisomerase II α to centromeres to safeguard genomic stability. EMBO J. 39, e101863–e101822. doi: 10.15252/embj.2019101863
Zhang, H., Ma, Z. Y., Zeng, L., Tanaka, K., Zhang, C. J., Ma, J., et al. (2013). DTF1 is a core component of RNA-directed DNA methylation and may assist in the recruitment of pol IV. Proc. Natl. Acad. Sci. U. S. A. 110, 8290–8295. doi: 10.1073/pnas.1300585110
Zhimulev, I. F., Belyaeva, E. S., Bgatov, A. V., Baricheva, E. M., and Vlassova, I. E. (1988). Cytogenetic and molecular aspects of position effect variegation in Drosophila melanogaster. Chromosoma 96, 255–261. doi: 10.1007/BF00302365
Zhimulev, I. F., Belyaeva, E. S., Fomina, O. V., Protopopov, M. O., and Bolshakov, V. N. (1986). Cytogenetic and molecular aspects of position effect variegation in Drosophila melanogaster. Chromosoma 94, 492–504. doi: 10.1007/BF00292759
Zhong, X., Du, J., Hale, C. J., Gallego-Bartolome, J., Feng, S., Vashisht, A. A., et al. (2014). Molecular mechanism of action of plant DRM De novo DNA Methyltransferases. Cell 157, 1050–1060. doi: 10.1016/j.cell.2014.03.056
Keywords: insulators, topoisomerase, barrier function, heterochromatin spreading, chromatin domains, chromatin islands
Citation: Velay F, Méteignier L-V and Laloi C (2022) You shall not pass! A Chromatin barrier story in plants. Front. Plant Sci. 13:888102. doi: 10.3389/fpls.2022.888102
Edited by:
Clémentine Vitte, UMR8120 Génétique Quantitative et Evolution Le Moulon, FranceReviewed by:
Pao-Yang Chen, Academia Sinica, TaiwanChristine Queitsch, University of Washington, United States
Nicholas A. Mueth, University of Washington, United States in collaboration with reviewer CQ
Copyright © 2022 Velay, Méteignier and Laloi. This is an open-access article distributed under the terms of the Creative Commons Attribution License (CC BY). The use, distribution or reproduction in other forums is permitted, provided the original author(s) and the copyright owner(s) are credited and that the original publication in this journal is cited, in accordance with accepted academic practice. No use, distribution or reproduction is permitted which does not comply with these terms.
*Correspondence: Christophe Laloi, Y2hyaXN0b3BoZS5sYWxvaUB1bml2LWFtdS5mcg==