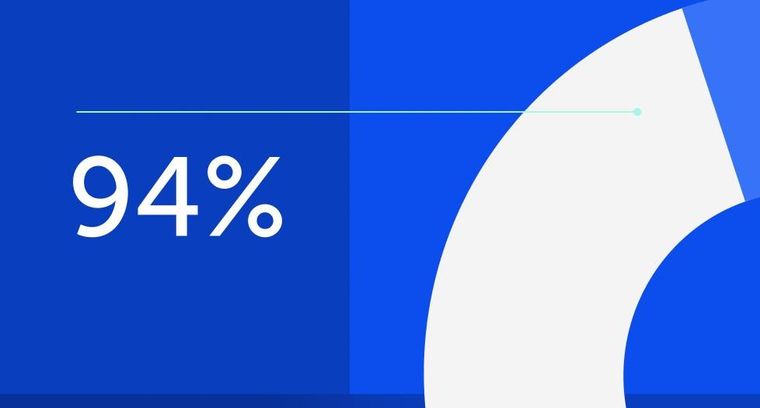
94% of researchers rate our articles as excellent or good
Learn more about the work of our research integrity team to safeguard the quality of each article we publish.
Find out more
REVIEW article
Front. Plant Sci., 09 June 2022
Sec. Plant Breeding
Volume 13 - 2022 | https://doi.org/10.3389/fpls.2022.882583
This article is part of the Research TopicMethods, Applications, and Protocols in Plant Science: Network Modeling-guided Understanding of Gene Regulation in PlantsView all 5 articles
Cotton is the most important source of natural fiber in the world as well as a key source of edible oil. The plant architecture and flowering time in cotton are crucial factors affecting cotton yield and the efficiency of mechanized harvest. In the model plant arabidopsis, the functions of genes related to plant height, inflorescence structure, and flowering time have been well studied. In the model crops, such as tomato and rice, the similar genetic explorations have greatly strengthened the economic benefits of these crops. Plants of the Gossypium genus have the characteristics of perennials with indeterminate growth and the cultivated allotetraploid cottons, G. hirsutum (Upland cotton), and G. barbadense (Sea-island cotton), have complex branching patterns. In this paper, we review the current progresses in the identification of genes affecting cotton architecture and flowering time in the cotton genome and the elucidation of their functional mechanisms associated with branching patterns, branching angle, fruit branch length, and plant height. This review focuses on the following aspects: (i) plant hormone signal transduction pathway; (ii) identification of cotton plant architecture QTLs and PEBP gene family members; (iii) functions of FT/SFT and SP genes; (iv) florigen and anti-florigen systems. We highlight areas that require further research, and should lay the groundwork for the targeted bioengineering of improved cotton cultivars with flowering times, plant architecture, growth habits and yields better suited for modern, mechanized cultivation.
The core aspects of the aerial architecture of plants are determined by the overall plant height, stem growth habits, and branching pattern, while the peripheral structural features reflect the size, shape, quantity, and relative arrangements of the leaves and flowers. The architecture of plant is species-specific, reflecting a strict genetic control. However, genes with an important role in determining plant structure can be influenced by many exogenous factors, such as light, temperature, humidity, and plant nutritional status (Reinhardt and Kuhlemeier, 2002; Wang et al., 2018). In flowering plants, the shoot apical meristem (SAM) and root apical meristem contains a large number of pluripotent stem cells that are maintained by continuous or periodic division and from which, the different cell lineages are formed through differentiation and continuously form new tissues and organs, such as leaves, stems, branches, and flower (Bowman and Eshed, 2000; Barton, 2010).
In agronomically important crops, plant architecture has a strong influence on the methods of crop cultivation and management as well as the design and efficiency of mechanized plant harvest. Changing the flowering time can enhance the ecological adaptability of plants, and changing the plant architecture can optimize the planting mode of crops. Therefore, flowering time and plant architecture traits are important goals of crop genetic improvement. Lang et al. (1977) first described the balance between positive flowering and negative flowering signals through grafting experiment in tomato and tobacco, i.e., the later identified florigen and antiflorigen (Lang et al., 1977; McGarry and Ayre, 2012a; Eshed and Lippman, 2019). Florigen and gibberellin systems control flowering time and plant architecture. The occurrences of natural mutations in these systems and their exploitation or bioengineering efforts have led to improved crop traits. For example, in the green revolution of the 1960s wheat varieties with shorter and sturdy stalks were selected for in breeding programs, so that crops could better resist lodging and weather hazards while maintaining a higher yield (Peng et al., 1999). By studying the tomato SELF-PRUNING mutant, scientists discovered the first antiflorigen molecule SP (Pnueli et al., 1998). In the model plant species, Arabidopsis thaliana, research into the gene functions related to plant height, flowering time and the formation of branch and inflorescence structures have been studied extensively. Research on crops of agronomical importance, such as tomato and rice, and the subsequent identification of gene targets for genetic breeding programs has greatly enhanced the economic benefits of these crops (Wang et al., 2018).
Cotton originated in tropical and subtropical regions, where it showed indeterminate growth and perennial growth habit, and cultivated cotton gradually lose its sensitivity to photoperiod, mainly annual. Cotton plant architecture and flowering time are the key factors affecting cotton yield and the efficiency of mechanization cultivation. Because, compared with the potential of increasing yield per plant, increasing the planting density has greater potential to increase the yield. In Xinjiang Uygur Autonomous Region, China, the main cultivation feature of cotton is dwarf, dense and early (Figure 1A), which has made great achievements in cotton production in Xinjiang. The planting density in this area is generally 180,000–220,000 plants per hectare, making it the largest and highest yield cotton planting area in China (Si et al., 2018; Zhan et al., 2021; Figure 1A). Cotton plant architecture is determined by many factors such as branching pattern, inflorescence structure and plant height, and its branching architecture is different from Solanaceae plants such as tomato (Park et al., 2014a; Moraes et al., 2019). The main stem of cotton displays monopodial branching, which maintains the characteristics of indeterminate growth and produces vegetative growth indefinitely. There are two kinds of branches in cotton. The basal vegetative branches are monopodial and show an indeterminate growth habit. The distal fruit branches show a sympodial inflorescence and each sympodial unit is composed of internodes, a terminal boll with flowers, an opposite leaf and an axillary bud that produces the next sympodial unit, thus forming a pyramidal plant structure (Figures 1B,C; Gore, 1935; McGarry et al., 2016; Si et al., 2018). In this paper, we will review recent progress in the identification and characterization of genes and quantitative trait loci (QTL) regulating cotton branching pattern, branching angle, fruit branch length, plant height and flowering time (Table 1), as well as their application and/or potential in breeding programs to obtain beneficial agronomical trait in cotton. A greater understanding of the genetic regulatory network of these progresses will lay the foundation for future targeted bioengineering of improved cotton cultivars for higher yields and optimized cultivation and harvesting.
Figure 1. The cultivation feature and plant architecture of cotton. (A) The planting pattern of short-dense-early cotton in Xinjiang Uygur Autonomous Region, China. Phenotype of normal fruit branch upland cotton (B) and its schematic diagram (C). Phenotype of short fruit branch upland cotton (D) and its schematic diagram (E). Blue triangles indicate monopodial shoot apical meristem; green triangles represent sympodial shoot meristem; red balls represent determinate floral buds; green peach-like shapes indicate leaves.
Plant hormones play an important role in regulating meristem activity, which has a profound influence on the establishment of plant architecture (Wang et al., 2018). The introduction of dwarfing traits into wheat and rice during the “Green Revolution” is now understood to have been achieved through the genetic alteration in gibberellin metabolism and action (Peng et al., 1999; Sasaki et al., 2002). In Arabidopsis thaliana, the role of hormones and their signaling pathways in plant development has been studied intensively (Ongaro and Leyser, 2008). However, there has been comparatively little systematic research into the role of plant hormones and their underlying molecular mechanisms in the regulation of cotton architecture and flowering. Nevertheless, some progress has been made through the identification and functional analysis of cotton orthologous genes important for hormonal signaling and their targets in mode plant systems.
Signals derived from several factors, including hormones, nutrients, and environmental factors, cooperatively regulate the growth of buds and their related signal pathways converge on BRANCHED1 (BRC1), which ultimately determines whether axillary buds are dormant or growing (González-Grandío et al., 2013; Wang et al., 2018). BRC1 gene encodes a TCP transcription factor, which is specifically expressed in axillary buds and participates in the inhibition of bud growth and branching (Hubbard et al., 2002). Maize TB1 is an ortholog of BRC1 in A. thaliana, which has been studied intensely for its regulatory role in branching patterns (Hubbard et al., 2002; Wang et al., 2018).
GhBRC1 is highly expressed in the axillary bud tissue of cotton with nulliplex-branch (no fruit branching) phenotypes, and is induced by various hormones (Sun et al., 2021). The overexpression of GhBRC1 in A. thaliana resulted in a significant reduction in the rosette-leaf branch number. In addition, transformation of A. thaliana mutant, brc1, rescued its high branch number phenotype (Sun et al., 2021). Silencing this gene in cotton using virus-induced gene silencing (VIGS) caused early flowering and formed a multi-branched phenotype, suggesting that similar to AtBRC1, GhBRC1 is a key gene exerting negative control over the branch growth and development (Sun et al., 2021).
TIE1 (TCP interactor containing EAR motif protein 1), a transcriptional repressor, interacts with and represses BRC1 activity, thus acting as a positive regulator of branching (Tao et al., 2013). The ectopic overexpression of GhTIE1 in A. thaliana led to a more highly branched phenotype, whereas silencing of GhTIE1 using VIGS technology decreased branching number in cotton, suggesting that GhTIE1 positively regulates shoot branching (Diao et al., 2019). The results showed that TIE1 exerts a conserved regulatory function over branching in arabidopsis and cotton, through modulations of the activities of TIE1 and TCP proteins, including BRC1 (Diao et al., 2019).
As one of the largest families of transcription factors in plants, NACs (NAM, ATAF1/F2 and CUC2) plays important roles in the formation and maintenance of different meristems and the development of bud branches. GhCUC2 interacts with GhBRC1 to negatively regulate cotton branching, while miR164 has the opposite effect and inhibits the function of GhCUC2 by cleavage of its mRNA. GhBRC1 can directly bind to the promoter region of NCED1 (Nine-Cis Epoxycarotenoid Dioxygenase) and activate the transcription of this gene, resulting in local ABA accumulation with the suppression of axillary bud and lateral branch formation. The miR164-GhCUC2-GhBRC1-GhNCED1 module is therefore an important regulatory axis in lateral branch development in arabidopsis and cotton (Zhan et al., 2021). The expression levels of the cotton NCED homologous genes in dwarf accessions were higher than that in taller ones. Silencing of GhNCED1 using VIGS increased plant height and internode numbers (Pei et al., 2021), suggesting that GhNECD1 negatively regulate plant height.
Strigolactone (SL) is considered an important plant hormone in the regulation of branch development. SL promotes the expression of TB1/BRC1 and thereby participates in the inhibition of axillary bud development and plant branch formation (Gomez-Roldan et al., 2008). DWARF4 (D14) is a α/β hydrolase in the SL signaling and a non-canonical receptor of SL, forms a covalent interaction with the SL hydrolysis product (Yao et al., 2016). The ortholog of D14 in sea-island cotton, GbD14, was found to be highly expressed in axillary buds, and the ectopic expression of GbD14 in A. thaliana could complement the multi-branched phenotype of the Atd14 mutant (Chevalier et al., 2014), confirming that GbD14 was involved in the regulation of cotton branching (Wang et al., 2019). GhPAGODA1 (PAG1) encodes a cytochrome P450 that catabolizes active brassinosteroid (BRs) via C-26 hydroxylation (Yang et al., 2014). GhPAGODA1 SUPPRESSOR 1 encodes a bHLH transcription factor of cotton, and GhPAS1 overexpression in arabidopsis could partially rescue the dwarf phenotype of Atpag1 mutant; while GhPAS1 silencing using VIGS inhibited plant growth and altered plant architecture in cotton (Wu et al., 2021). This result suggested that GhPAS1 regulates cotton development and architecture via mediating BR signaling.
In summary, from recent research into the hormonal regulation of branching in A. thaliana and rice, several important genes with key roles in this process have been characterized and their functional orthologs in cotton have been identified. However, the current research in cotton is still quite superficial and the number of key genes identified is still very limited. AiSheng, a natural dwarf mutant of cotton, has shorter fruit branches and presents at a more acute angle to the stems. Gene cloning revealed that the gene located on chromosome D12 and contained a tandem duplication of about 13.5 kb, which led to the doubling of the copy number of Dehydration Responsive Element Binding factor (GhDREB1B), with a significant increase in gene expression, resulting in phenotypic changes (Ji et al., 2021). RNA-seq analysis showed that the relative up-regulation of GhDREB1B down-regulated many genes of the auxin signaling pathway, but up-regulated many genes of the ethylene and BR signaling pathways, which might comprehensively lead to a more compact cotton plant architecture (lower plant height, shorter branches, and smaller included angle to the stem). This study showed that GhDREB1B was an important gene controlling cotton plant architecture, through its effects on hormones signal pathways (Ji et al., 2021). The present findings enrich the regulatory network of cotton architecture and provide candidate genes for precise control of cotton form.
Genetic maps have been used in cotton, and some new technologies developed with the sequencing of cotton genome have been used to fine locate QTLs related to cotton structural traits (Song and Zhang, 2009; Huang et al., 2017; Su et al., 2018; Wen et al., 2019; Grover et al., 2020; Wang et al., 2022).
Song and Zhang (2009) identified 26 single QTLs with seven plant architectural traits using the interspecific hybrid BC1 population of G. hirsutum and G. barbadense. Huang et al. (2017) identified 160 candidate QTLs associated with 16 agronomic traits, including QTLs for plant height, fruit branch length using CottonSNP63K array and 503 Chinese upland cotton materials.
Using 355 upland cotton accessions, a genome-wide association study (GWAS) identified one gene, Gh_ D03g0922, affecting plant height (Table 1; Su et al., 2018). This gene encodes a protein containing MADS box domain, which was up-regulated in the apical buds and young leaves of short and compact cotton varieties (Su et al., 2018). Also, GWAS have identified five QTLs controlling plant height and six QTLs for fruit spur branch number using 121 cotton varieties, including 100 brown-fiber cotton and 21 white-fiber accessions (Wen et al., 2019). A restricted two-stage multilocus GWAS (RTM-GWAS) have identified 68 gene loci related to plant height, 34 to fruit branch angle and 55 to fruit branch length in 315 natural populations of upland cotton (Wang et al., 2022).
Ten plant architecture traits were evaluated, which include plant height, fruiting branch length, branch angle, and stem pubescence using an intraspecific cross population within G. hirsutum (Grover et al., 2020). 120 QTLs related to phenotypic changes under domestication have been identified. Many QTLs are both clustered and environmentally labile (Grover et al., 2020).
Although QTL regions contain many genes, the identified QTLs related to cotton plant architecture provide targets for further mining candidate genes and key insights for in-depth understanding of the genetic basis of cotton plant architecture.
Phosphatidylethanolamine binding proteins (PEBPs) are also referred to as CETS from [CENTRORADIALIS (CEN)/TERMINAL FLOWER1 (TFL1)/SELF-PRUNING (SP)] proteins (McGarry and Ayre, 2012a; Lifschitz et al., 2014). The PEBP of higher plants contain three subfamilies, including the MOTHER OF FT AND TFL1 (MFT)-like proteins, the FLOWERING LOCUS T (FT)-like proteins and the TFL1-like proteins (Karlgren et al., 2011). In the TFL1-like clade, there are multiple genes including TFL1, BROTHER OF FT, and SP genes. Of these, FT protein is the florigen of plant, whereas TFL1, SP, and CEN proteins are antiflorigen components. They constitute the florigen and antiflorigen system of plants, which is essential to maintain the determinate and indeterminate growth of meristem (Lifschitz et al., 2014; Eshed and Lippman, 2019). Therefore, these genes are the key regulatory factors of plant architecture, controlling the transition to flowering and the meristem fate.
In recent years, the successful sequencing and sequence update of cotton genome has led to the identification of PEBP gene members by several research groups (Table 1; Hu et al., 2019; Huang et al., 2020). McGarry et al. (2016) and our genome-wide identification results showed that there were eight PEBP genes in diploid cotton (G. raimondii and G. arborum), and eight pairs of PEBP genes in tetraploid cottons (G. hirsutum and G. barbadense), of which At and Dt contained eight loci, respectively (McGarry et al., 2016; Si et al., 2018). In the G. hirsutum genome, there are two homoeologous MFT gene pairs (GhMFT-L1A/L1D, GhMFT-L2A/L2D) and one homoeologous FT gene pair (GhFT-A and GhFT-D, or named [GhSFT-A and GhSFT-D by McGarry et al. (2016)], two homoeologous pairs of TFL1 genes (GhTFL1-1A/1D, GhTFL1-2A/2D), one homoeologous pairs of SP genes (GhSP-A/D) and two homoeologous pairs of BFT genes (GhBFT-1A/1D, GhBFT-2A/2D). Most PEBP genes have similar intron and exon structures, and all PEBP proteins contain very conservative “D-P-D-x-P” and “G-x-H-R” motifs and key amino acids to distinguish FT and TFL1 proteins (Ahn et al., 2006). These results indicate that more PEBP gene members have been produced during the evolution of the cotton genome, and also imply the complexity of PEBP gene functions in cotton.
Florigen, encoded by FT, mainly maintains determinate growth and promotes flowering transition, which is not only functionally conserved in evolution, but also displays pleiotropic trait (Moraes et al., 2019; Jin et al., 2021). Plants perceive changes in the photoperiod in leaves and promote the expression of FT in leaf companion cells to synthesize the FT protein. At low temperatures, FT can bind to negatively charged phosphatidylglycerols in the cell membrane of companion cells, thus reducing the level of soluble FT in sieve tubes which, limits the long-distance transport of FT protein to the apical meristem and delays the transition to flowering (Susila et al., 2021). This study reveals the interactions between FT and phosphatidylglycerol and its molecular mechanism of jointly regulating plant flowering process, which provides a new research idea for the mechanism of plants adjusting flowering process in response to seasonal temperature changes. At more favorable temperatures, FT can be more easily transported to the shoot apex, where it forms a complex with 14-3-3 proteins (Susila et al., 2021). After transport to the nucleus, the complex binds with the bZIP transcription factor, FD, to form a flowering activation complex (FAC), which induces flowering through the activation of downstream flower identity genes, such as SUPPRESSOR OF OVEREXPRESSION OF CONSTANS 1 (SOC1) and APETALA1 (AP1) (Abe et al., 2005; Wigge et al., 2005; Taoka et al., 2011).
Some studies have revealed that FT orthologs are functionally conserved in different plant species. The ectopic overexpression of AtFT in wild and cultivated cotton using the virus-mediated transformation system enhanced the determinate growth habit of cotton aerial parts by the decoupling of flowering from photoperiod regulation to produce early flowering and accelerated ripening, with a dense growth of fruit branches on the main stem (McGarry and Ayre, 2012b). However, it did not affect the structural development of the cotton flower organs formed (McGarry et al., 2013). Similarly, the upregulation of GhFT/SFT expression in cotton mediated by a disarmed Cotton leaf crumple virus caused cotton flowering to separate from photoperiod leading to premature flowering (McGarry et al., 2016; McGarry and Ayre, 2021). However, the indeterminate growth habit of the main branches did not change, although some fruit branches became shorter or grew directly on the main stem. Conversely, the silencing of GhFT/SFT mediated by the tobacco rattle virus led to delayed cotton maturation and the display of more characteristics of indeterminate growth, such as larger main stem leaves and longer petioles (McGarry et al., 2016). Furthermore, Prewitt et al. (2018) reported that GhSFT rescued Atft-10 mutant, having similar numbers of leaves and flowering times with wild type. These studies show that the FT gene affects the plant architecture of cotton and that the judicious manipulation of FT and related genes could weaken perennial characteristics to improve domesticated strains. Our study indicated that overexpression of GhFT in A. thaliana promoted flowering and partially complemented the late flowering phenotype of the Atft-10 mutant, suggesting that GhFT is similar to the FTs of other plant species in the regulation process of controlling flowering (Guo et al., 2015; Cai et al., 2017; Sang et al., 2019). Previous studies have shown that the FT protein, as a general florigen, regulates multifaceted growth and development processes of plants (Shalit et al., 2009). We also showed that ectopic overexpression of GhFT in tobacco not only causes early flowering, but also promotes the overgrowth of lateral branches, leaf development, flower abscission. Molecular analyses showed that ectopic expression of GhFT in tobacco might affect the distribution of endogenous FT, affecting the plant morphogenesis (Li et al., 2015).
The FAC model reveals the functional conservation of florigen complex, because the three proteins constituting FAC are very conserved in seed plants (Karlgren et al., 2011; Taoka et al., 2011). Through genome-wide identification and analysis, we found that there were five FDs and 31 14-3-3 homoeologous gene pairs in G. hirsutum (Liu et al., 2021; Sang et al., 2021). GhFT–Gh14-3-3–GhFD1/2 complexes can activate the expression of GhAP1,the homologous flower identity gene in cotton, thus promoting the flowering. In contrast, GhFT–Gh14-3-3–GhFD3/4/5 complexes in roots activate the expression of AUXIN RESPONSE FACTOR 19, thereby promoting lateral root development (Liu et al., 2021). The function of FAC in cotton is also influenced by its component 14-3-3 protein, which can either promote or inhibit flowering by regulating the expression of GhAP1 and GhSOC1 (Sang et al., 2021). These studies show that although there is only one FT/SFT protein in polyploid upland cotton, it possesses diverse FD and 14-3-3 proteins available allowing the formation of diversified FACs to regulate different development processes.
TFL1/SP/CEN proteins are also homologous to FT, but have an opposite function. TFL1/SP/CEN mainly promotes indeterminate activity in meristem to maintain vegetative growth and inhibit the transition to flowering. Loss-of-function mutations in AtTFL1 and AmCEN decrease plant height with the onset of precociously flowering (Bradley et al., 1996, 1997). The tomato sp mutant is particularly short, dense and compact with restricted growth in the stems and branches which, avoids the necessity for pruning, enabling the tomato to be planted on a large scale (Pnueli et al., 1998). The mutation of the DETERMINATE STEM gene, a soybean ortholog of the arabidopsis TFL1, leads to a shorter and more compact plant, with a concentrated fruit setting (Tian et al., 2010). Therefore, TFL1/SP proteins in plants constitute the antiflorigen system and play a decisive role in establishing plant architecture. Crop TFL1/SP mutations are widely used in agriculture due to their better adaptation to dense planting regimes and mechanized harvesting with improvements in yield and reduced planting costs (Eshed and Lippman, 2019).
Although most of the modern cottons cultivated today are daily neutral and annual crops, the perennial habit of indeterminate growth of their ancestral varieties still persists to some degree. Some cotton mutants have been characterized as deficient in the cotton orthologous of tomato SELF-PRUNING (GoSP) and these display axillary flowers on short or nulliplex fruit branches (Chen et al., 2019). This results in a more compact architecture, allowing high-density planting to increase cotton yield. There is also a cluster boll mutation in upland cotton, in which one or more cluster flowers with slender pedicels form on the main stem (Figures 1D,E; Pathak and Singh, 1975). Almost all the long-staple cotton varieties cultivated and planted in Xinjiang display short fruiting branches. This kind of short fruit branch character makes it possible to increase the planting density and greatly increase the cotton yield. Therefore, short branch character is an ideal plant type for cotton breeding. This phenotype was found simultaneously by us and two other independent research groups to be due to mutations in a cotton ortholog of TFL1/SP/CEN. Although all three research groups identified the same gene, it was given different names by each (Liu et al., 2018; Si et al., 2018; Chen et al., 2019). Because the amino acid sequence deduced from this gene has the highest similarity with the tomato SP protein, this paper describes it as the cotton SP gene (McGarry et al., 2016; Si et al., 2018). Chen et al. (2019) reported four types of Ghsp mutations in upland cotton and that GhSP was mainly expressed in roots and shoot apexes. Overexpression of the GhSP gene in cotton inhibited flower bud growth, indicating that GhSP plays a key role in regulating flower bud differentiation and determinate growth (Chen et al., 2019). In situ hybridization revealed that GhSP was preferentially expressed in the meristems of cotton main stems and axillary buds (Liu et al., 2018). Overexpression of GhSP inhibited the transition from vegetative to reproductive branch formation (Liu et al., 2018; Si et al., 2018). Conversely, the silencing of GhSP resulted in early flowering and determinate growth, with the production of terminal flowers on the main stem and shorter lateral branches (McGarry et al., 2016; Liu et al., 2018). RNA-seq analysis showed that this phenotypic alteration generally occurred with the upregulation of a MADS-box gene (Liu et al., 2018). It was found that all varieties of sea-island cotton in Xinjiang with sp mutation displayed early maturity, indicating that they are better adapted for cultivation at high latitudes with short frost-free periods, indicating that the sp mutation had been strongly selected for early maturity breeding program (Liu et al., 2018; Si et al., 2018).
Similarly, our research revealed that the mutation of Gbsp113Ser in sea-island cotton produces nulliplex-branches, while the mutation of Ghsp73Asn in upland cotton produces cluster-bolls (Si et al., 2018). However, in both mutations, the apical meristem maintains indeterminate growth. In addition, when Ghsp73Asn or Gbsp113Ser gene was overexpressed in arabidopsis tfl1-1 mutant, the dwarfed, early flowering and multi-branched phenotypes of the Attfl1-1 mutant were not changed (Si et al., 2018). GhSP silencing terminates the indeterminate growth of cotton, leading to the termination of the monopodial main stem, thus leading to early flowering and early maturity. Subcellular localization analysis showed that Ghsp73Asn and Gbsp113Ser mutant proteins did not change the subcellular localization of proteins. Protein interaction analysis showed that GhSP could interact with GhFD1 and Gh14-3-3 in plant cells, but mutant proteins of Ghsp73Asn and Gbsp113Ser could not interact with GhFD1 and therefore it is likely that these mutant SPs cannot form a functional FAC to effect antiflorigen activities, leading to the cluster-bolls and nulliplex-branch phenotypes in cotton (Si et al., 2018). McGarry et al. (2016) found that GhSP plays an important role in the regulation of lignin and secondary cell wall synthesis, emphasizing the influence of SP on plant stem morphology.
To date, SP is considered the most important gene that controls the development of fruit branches and thus, in the regulation of cotton plant architecture. A single amino acid change in Ghsp73Asn (D73N) is located in the highly conserved domains D-P-D-x-P, whereas the amino acid change in Gbsp113Ser (P113S) is located next to the conserved domains G-x-H-R of PEBP proteins (Si et al., 2018). Both domains are located on both sides of anion binding sites (Ahn et al., 2006). Tomato sp76Leu (P76L) is also located in the D-P-D-x-P domain, resulting in determinate growth of the terminal buds (Pnueli et al., 1998). It shows that the changes of key amino acids in this domain of plant SP protein may result in the loss of protein function and plant type change, which may be a research direction of targeted gene directional change. The production of further site-directed mutations in SP orthologs will undoubtedly enhance our understanding of their protein-protein interactions with FD and 14-3-3- members in the future.
During the process of evolution, plants have evolved to coordinate endogenous factors and exogenous environmental stimuli to ensure that flowering occurs at the correct time. After domestication and the application of selection criteria in crops breeding, the flowering time and plant architecture have changed dramatically. Many of the successes in crop breeding for improved yield and architectural traits, can ultimately be related to changes to florigen and antiflorigen, and their inter-play (Eshed and Lippman, 2019).
Genetic evidence from several plant species, including arabidopsis, tomato and rice shows that all flowering pathways eventually converge to the florigen and antiflorigen system, which can be divided into core regulatory components and peripheral regulatory components (Lifschitz et al., 2014; Eshed and Lippman, 2019). Core components play a common role in all flowering plants, such as florigen FT/SFT and antiflorigen TFL1/SP/CEN, and their interacting proteins such as FD, 14-3-3 protein, etc. FT and TFL1 compete with FD proteins to regulate the expression of common target genes of flowering such as LFY, AP1 and others so as to control the determinate or indeterminate growth of meristem (Abe et al., 2005; Wigge et al., 2005; Collani et al., 2019; Romera-Branchat et al., 2020; Zhu et al., 2020). Moreover, the content, proportion and position of expressed FT and TFL1/SP proteins in different tissues and different developmental times accurately regulate the flowering time and architecture of plants (Lifschitz et al., 2014; Moraes et al., 2019). The manipulation of SFT/SP balance in tomato can change the planting pattern and management, and enhance yield (Park et al., 2014b).
The present research showed that in cotton, GhFT/SFT belongs to the florigen component, whereas GhSP exerts the effects of antiflorigen in cotton with a dose effect (McGarry et al., 2016; Liu et al., 2018; Prewitt et al., 2018; Si et al., 2018; Chen et al., 2019). Overexpression of the two cotton TFL1 (GhTFL1-1 and GhTFL1-2) genes in A. thaliana caused a late flowering phenotype, and partially rescued the early flowering phenotype of the Attfl1-14 mutant. Yeast two-hybrid assay showed that both could interact with an FD homologous protein (Prewitt et al., 2018), suggesting that the functions of GhTFL1-1 and GhTFL1-2 are antagonistic to GhFT/SFT and can be considered components of cotton antiflorigens, but their detailed functions remain unclear. AtTFL1 can also be translocated from its site of synthesis, but in a different way from FT (Conti and Bradley, 2007). AtTFL1 mRNA expressed in chalazal endosperm is transported to syncytial peripheral endosperm. ChIP-seq analyses have revealed a variety of target genes of AtTFL1-FD (Collani et al., 2019; Goretti et al., 2020). However, one such target is the ABA pathway ABA INSENSITIVE 5 protein which, after AtTFL1-FD binding is involved in seed size regulation (Zhang et al., 2020).
Because no natural mutant related to cotton TFL1 has been identified and cotton is a recalcitrant plant species for regeneration, an in-depth study of this gene function is practically more difficult. In-depth functional study of cotton TFL1 gene can better analyze the molecular mechanism of TFL1-clade gene regulating cotton plant architecture and establishing the genetic network of branches and plant architecture, which is of great significance in cotton. SP mainly regulates axillary meristem and promotes the formation of short fruit branches. At present, the short fruit branches varieties of long-staple cotton and sea-island cotton in production mostly use sp mutation, which is very popular because it is suitable for dense planting, convenient management and convenient mechanized harvesting (Si et al., 2018; Chen et al., 2019; Zhan et al., 2021). Because there is no mutant with self-pruning at the top like tomato sp, at present, the indeterminate growth of cotton is mainly restrained by spraying DPC and artificial topping with high cost. Genetic analysis of the cotton florigen-antiflorigen system and genetic improvement through gene editing technologies are expected to resolve these cultivation issues in the near future.
Although breeding and selection practices of cultivated upland and sea-island cotton have led to their day neutrality, the habit of indeterminate growth still persists, whereas a fully determinate growth habit would be preferable for modern cotton cultivation and harvest. At present, the short fruit branch plant architecture represents the most ideal plant type, since it is suitable for dense planting, mechanized harvesting and produces enhanced yield. Scientists have noticed that key factors in hormone signaling pathway play an important role in cotton branch development, such as BRC1, CUC2, D14, and DREB1B genes. FT/SFT and SP constitute the components of florigen and antiflorigen in cotton, respectively. The natural allelic variation of SP forms the short fruit branch plant type. However, the persistence of some undesirable perennial characteristics in the sp mutants used in modern cotton cultivation need to be further studied. Further exploration into the functions of the antiflorigen components, TFL1/SP, and the interactions between FT/SFT, TFL1/SP, and BRC1, as well as analyzing the FAC components and molecular essence of cotton will be the focus of future research. The purpose is to establish the genetic network underlying cotton flowering transition and the regulation of plant architecture. Subsequently, the knowledge gained will be used to finely adjust the balance between florigen and antiflorigen using CRISPR/Cas technology, thus facilitating further genetic improvement in cotton.
XH, HL, and BM designed the initial manuscript and contributed reviewing and discussing the manuscript. XH wrote the manuscript. HL conceived the figure. BM checked all the references. All authors contributed to the article and approved the submitted version.
This work was financially supported by the National Natural Science Foundation of China (Nos. 31860393 and 31360366), the Talent Introduction Start-up Fund Project of Anhui Science and Technology University (No. NXYJ202001), and the University Discipline (Major) Top Talent Cultivation Funding Project (No. gxbjZD2021072).
The authors declare that the research was conducted in the absence of any commercial or financial relationships that could be construed as a potential conflict of interest.
All claims expressed in this article are solely those of the authors and do not necessarily represent those of their affiliated organizations, or those of the publisher, the editors and the reviewers. Any product that may be evaluated in this article, or claim that may be made by its manufacturer, is not guaranteed or endorsed by the publisher.
Abe, M., Kobayashi, Y., Yamamoto, S., Daimon, Y., Yamaguchi, A., Ikeda, Y., et al. (2005). FD, a bZIP protein mediating signals from the floral pathway integrator FT at the shoot apex. Science 309, 1052–1056. doi: 10.1126/science.1115983
Ahn, J. H., Miller, D., Winter, V. J., Banfield, M. J., Lee, J. H., Yoo, S. Y., et al. (2006). A divergent external loop confers antagonistic activity on floral regulators FT and TFL1. EMBO J. 25, 605–614. doi: 10.1038/sj.emboj.7600950
Barton, M. K. (2010). Twenty years on: the inner workings of the shoot apical meristem, a developmental dynamo. Dev. Biol. 341, 95–113. doi: 10.1016/j.ydbio.2009.11.029
Bowman, J. L., and Eshed, Y. (2000). Formation and maintenance of the shoot apical meristem. Trends Plant Sci. 5, 110–115.
Bradley, D., Carpenter, R., Copsey, L., Vincent, C., Rothstein, S., and Coen, E. (1996). Control of inflorescence architecture in Antirrhinum. Nature 379, 791–797.
Bradley, D., Ratcliffe, O., Vincent, C., Carpenter, R., and Coen, E. (1997). Inflorescence commitment and architecture in Arabidopsis. Science 275, 80–83. doi: 10.1126/science.275.5296.80
Cai, D., Liu, H., Sang, N., and Huang, X. (2017). Identification and characterization of CONSTANS-like (COL) gene family in upland cotton (Gossypium hirsutum L.). PLoS One 12:e0179038. doi: 10.1371/journal.pone.0179038
Chen, W., Yao, J., Li, Y., Zhao, L., Liu, J., Guo, Y., et al. (2019). Nulliplex-branch, a TERMINAL FLOWER1 ortholog, controls plant growth habit in cotton. Theor. Appl. Genet. 132, 97–112.
Chevalier, F., Nieminen, K., Sánchez-Ferrero, J. C., Rodríguez, M. L., Chagoyen, M., Hardtke, C. S., et al. (2014). Strigolactone promotes degradation of DWARF14, an α/β hydrolase essential for strigolactone signaling in Arabidopsis. Plant Cell 26, 1134–1150. doi: 10.1105/tpc.114.122903
Collani, S., Neumann, M., Yant, L., and Schmid, M. (2019). FT modulates genome-wide DNA-binding of the bZIP transcription factor FD. Plant Physiol. 180, 367–380. doi: 10.1104/pp.18.01505
Conti, L., and Bradley, D. (2007). TERMINAL FLOWER1 is a mobile signal controlling Arabidopsis architecture. Plant cell 19, 767–778. doi: 10.1105/tpc.106.049767
Diao, Y., Zhan, J., Zhao, Y., Liu, L., Liu, P., Wei, X., et al. (2019). GhTIE1 regulates branching through modulating the transcriptional activity of TCPs in cotton and Arabidopsis. Front. Plant Sci. 10:1348. doi: 10.3389/fpls.2019.01348
Eshed, Y., and Lippman, Z. B. (2019). Revolutions in agriculture chart a course for targeted breeding of old and new crops. Science 366:eaax0025. doi: 10.1126/science.aax0025
Gomez-Roldan, V., Fermas, S., Brewer, P. B., Puech-Pagès, V., Dun, E. A., Pillot, J. P., et al. (2008). Strigolactone inhibition of shoot branching. Nature 455, 189–194.
González-Grandío, E., Poza-Carrión, C., Sorzano, C. O. S., and Cubas, P. (2013). BRANCHED1 promotes axillary bud dormancy in response to shade in Arabidopsis. Plant Cell 25, 834–850. doi: 10.1105/tpc.112.108480
Goretti, D., Silvestre, M., Collani, S., Langenecker, T., Méndez, C., Madueño, F., et al. (2020). TERMINAL FLOWER1 functions as a mobile transcriptional cofactor in the shoot apical meristem. Plant Physiol. 182, 2081–2095. doi: 10.1104/pp.19.00867
Grover, C. E., Yoo, M. J., Lin, M., Murphy, M. D., Harker, D. B., Byers, R. L., et al. (2020). Genetic analysis of the transition from wild to domesticated cotton (Gossypium hirsutum L.). G3 10, 731–754. doi: 10.1534/g3.119.400909
Guo, D., Li, C., Dong, R., Li, X., Xiao, X., and Huang, X. (2015). Molecular cloning and functional analysis of the FLOWERING LOCUS T (FT) homolog GhFT1 from Gossypium hirsutum. J. Integr. Plant Biol. 57, 522–533. doi: 10.1111/jipb.12316
Hu, Y., Chen, J., Fang, L., Zhang, Z., Ma, W., Niu, Y., et al. (2019). Gossypium barbadense and Gossypium hirsutum genomes provide insights into the origin and evolution of allotetraploid cotton. Nat. Genet. 51, 739–748.
Huang, C., Nie, X., Shen, C., You, C., Li, W., Zhao, W., et al. (2017). Population structure and genetic basis of the agronomic traits of upland cotton in China revealed by a genome-wide association study using high-density SNPs. Plant Biotechnol. J. 15, 1374–1386. doi: 10.1111/pbi.12722
Huang, G., Wu, Z., Percy, R. G., Bai, M., Li, Y., Frelichowski, J. E., et al. (2020). Genome sequence of Gossypium herbaceum and genome updates of Gossypium arboreum and Gossypium hirsutum provide insights into cotton A-genome evolution. Nat. Genet. 52, 516–524. doi: 10.1038/s41588-020-0607-4
Hubbard, L., McSteen, P., Doebley, J., and Hake, S. (2002). Expression patterns and mutant phenotype of teosinte branched1 correlate with growth suppression in maize and teosinte. Genetics 162, 1927–1935. doi: 10.1093/genetics/162.4.1927
Ji, G., Liang, C., Cai, Y., Pan, Z., Meng, Z., Li, Y., et al. (2021). A copy number variant at the HPDA-D12 locus confers compact plant architecture in cotton. New Phytol. 229, 2091–2103. doi: 10.1111/nph.17059
Jin, S., Nasim, Z., Susila, H., and Ahn, J. H. (2021). Evolution and functional diversification of FLOWERING LOCUS T/TERMINAL FLOWER 1 family genes in plants. Semin. Cell Dev. Biol. 109, 20–30. doi: 10.1016/j.semcdb.2020.05.007
Karlgren, A., Gyllenstrand, N., Källman, T., Sundström, J. F., Moore, D., Lascoux, M., et al. (2011). Evolution of the PEBP gene family in plants: functional diversification in seed plant evolution. Plant Physiol. 156, 1967–1977. doi: 10.1104/pp.111.176206
Lang, A., Chailakhyan, M. K., and Frolova, I. A. (1977). Promotion and inhibition of flower formation in a dayneutral plant in grafts with a short-day plant and a long-day plant. Proc. Natl. Acad. Sci. U.S.A. 74, 2412–2416. doi: 10.1073/pnas.74.6.2412
Li, C., Zhang, Y., Zhang, K., Guo, D., Cui, B., Wang, X., et al. (2015). Promoting flowering, lateral shoot outgrowth, leaf development, and flower abscission in tobacco plants overexpressing cotton FLOWERING LOCUS T (FT)-like gene GhFT1. Front. Plant Sci. 6:454. doi: 10.3389/fpls.2015.00454
Lifschitz, E., Ayre, B. G., and Eshed, Y. (2014). Florigen and anti-florigen – a systemic mechanism for coordinating growth and termination in flowering plants. Front. Plant Sci. 5:465. doi: 10.3389/fpls.2014.00465
Liu, D., Teng, Z., Kong, J., Liu, X., Wang, W., Zhang, X., et al. (2018). Natural variation in a CENTRORADIALIS homolog contributed to cluster fruiting and early maturity in cotton. BMC Plant Biol. 18:286. doi: 10.1186/s12870-018-1518-8
Liu, H., Huang, X., Ma, B., Zhang, T., Sang, N., Zhuo, L., et al. (2021). Components and functional diversification of florigen activation complexes in cotton. Plant Cell Physiol. 62, 1542–1555. doi: 10.1093/pcp/pcab107
McGarry, R. C., and Ayre, B. G. (2012a). Manipulation plant architecture with members of the CETS gene family. Plant Sci. 188, 71–81. doi: 10.1016/j.plantsci.2012.03.002
McGarry, R. C., and Ayre, B. G. (2012b). Geminivirus-mediated delivery of florigen promotes determinate growth in aerial organs and uncouples flowering from photoperiod in cotton. PLoS One 7:e36746. doi: 10.1371/journal.pone.0036746
McGarry, R. C., and Ayre, B. G. (2021). Cotton architecture: examining the roles of SINGLE FLOWER TRUSS and SELF-PRUNING in regulating growth habits of a woody perennial crop. Curr. Opin. Plant Biol. 59:101968. doi: 10.1016/j.pbi.2020.10.001
McGarry, R. C., Prewitt, S., and Ayre, B. G. (2013). Overexpression of FT in cotton affects architecture but not floral organogenesis. Plant Signal. Behav. 8:e23602. doi: 10.4161/psb.23602
McGarry, R. C., Prewitt, S. F., Culpepper, S., Eshed, Y., Lifschitz, E., and Ayre, B. G. (2016). Monopodial and sympodial branching architecture in cotton is differentially regulated by the Gossypium hirsutum SINGLE FLOWER TRUSS and SELF-PRUNING orthologs. New Phytol. 212, 244–258. doi: 10.1111/nph.14037
Moraes, T. S., Dornelas, M. C., and Martinelli, A. P. (2019). FT/TFL1: calibrating plant architecture. Front. Plant Sci. 10:97. doi: 10.3389/fpls.2019.00097
Ongaro, V., and Leyser, O. (2008). Hormonal control of shoot branching. J. Exp. Bot. 59, 67–74. doi: 10.1093/jxb/erm134
Park, S. J., Eshed, Y., and Lippman, Z. B. (2014a). Meristem maturation and inflorescence architecture–lessons from the Solanaceae. Curr. Opin. Plant Biol. 17, 70–77. doi: 10.1016/j.pbi.2013.11.006
Park, S. J., Jiang, K., Tal, L., Yichie, Y., Gar, O., Zamir, D., et al. (2014b). Optimization of crop productivity in tomato using induced mutation in the florigen pathway. Nat. Genet. 46, 1337–1342. doi: 10.1038/ng.3131
Pathak, R. S., and Singh, R. B. (1975). Genetic analysis of the duplicate loci, cluster and short branch in Gossypium hirsutum L. Theor. Appl. Genet. 46, 281–287. doi: 10.1007/BF00281150
Pei, X., Wang, X., Fu, G., Chen, B., Nazir, M. F., Pan, Z., et al. (2021). Identification and functional analysis of 9-cis-epoxy carotenoid dioxygenase (NCED) homologs in G. hirsutum. Int. J. Biol. Macromol. 182, 298–310. doi: 10.1016/j.ijbiomac.2021.03.154
Peng, J., Richards, D. E., Hartley, N. M., Murphy, G. P., Devos, K. M., Flintham, J. E., et al. (1999). ‘Green revolution’ genes encode mutant gibberellin response modulators. Nature 400, 256–261. doi: 10.1038/22307
Pnueli, L., Carmel-Goren, L., Hareven, D., Gutfinger, T., Alvarez, J., Ganal, M., et al. (1998). The SELF-PRUNING gene of tomato regulates vegetative to reproductive switching of sympodial meristems and is the ortholog of CEN and TFL1. Development 125, 1979–1989. doi: 10.1242/dev.125.11.1979
Prewitt, S. F., Ayre, B. G., and McGarry, R. C. (2018). Cotton CENTRORADIALIS/TERMINAL FLOWER 1/SELF-PRUNING genes functionally diverged to differentially impact plant architecture. J. Exp. Bot. 69, 403–417. doi: 10.1093/jxb/ery324
Romera-Branchat, M., Severing, E., Pocard, C., Ohr, H., Vincent, C., Née, G., et al. (2020). Functional divergence of the Arabidopsis florigen-interacting bZIP transcription factors FD and FDP. Cell Rep. 31:107717.
Sang, N., Cai, D., Li, C., Sun, Y., and Huang, X. (2019). Characterization and activity analyses of the flowering locus T promoter in Gossypium Hirsutum. Int. J. Mol. Sci. 20:4769. doi: 10.3390/ijms20194769
Sang, N., Liu, H., Ma, B., Huang, X., Zhuo, L., and Sun, Y. (2021). Roles of the 14-3-3 gene family in cotton flowering. BMC Plant Biol. 21:162. doi: 10.1186/s12870-021-02923-9
Sasaki, A., Ashikari, M., Ueguchi-Tanaka, M., Itoh, H., Nishimura, A., Swapan, D., et al. (2002). Green revolution: a mutant gibberellin-synthesis gene in rice. Nature 416, 701–702.
Shalit, A., Rozman, A., Goldshmidt, A., Alvarez, J. P., Bowman, J. L., Eshed, Y., et al. (2009). The flowering hormone florigen functions as a general systemic regulator of growth and termination. Proc. Natl. Acad. Sci. U.S.A. 106, 8392–8397. doi: 10.1073/pnas.0810810106
Si, Z., Liu, H., Zhu, J., Chen, J., Wang, Q., Fang, L., et al. (2018). Mutation of SELF-PRUNING homologs in cotton promotes short-branching plant architecture. J. Exp. Bot. 69, 2543–2553. doi: 10.1093/jxb/ery093
Song, X. L., and Zhang, T. Z. (2009). Quantitative trait loci controlling plant architectural traits in cotton. Plant Sci. 177, 317–323.
Su, J., Li, L., Zhang, C., Wang, C., Gu, L., Wang, H., et al. (2018). Genome-wide association study identified genetic variations and candidate genes for plant architecture component traits in Chinese upland cotton. Theor. Appl. Genet. 131, 1299–1314. doi: 10.1007/s00122-018-3079-5
Sun, Q., Xie, Y., Li, H., Liu, J., Geng, R., Wang, P., et al. (2021). Cotton GhBRC1 regulates branching, flowering, and growth by integrating multiple hormone pathways. Crop J. 10, 75–87.
Susila, H., Jurić, S., Liu, L., Gawarecka, K., Chung, K. S., Jin, S., et al. (2021). Florigen sequestration in cellular membranes modulates temperature-responsive flowering. Science 373, 1137–1142. doi: 10.1126/science.abh4054
Tao, Q., Guo, D., Wei, B., Zhang, F., Pang, C., Jiang, H., et al. (2013). The TIE1 transcriptional repressor links TCP transcription factors with TOPLESS/TOPLESS-RELATED corepressors and modulates leaf development in Arabidopsis. Plant Cell 25, 421–437. doi: 10.1105/tpc.113.109223
Taoka, K. I., Ohki, I., Tsuji, H., Furuita, K., Hayashi, K., Yanase, T., et al. (2011). 14-3-3 proteins act as intracellular receptors for rice Hd3a florigen. Nature 476, 332–335. doi: 10.1038/nature10272
Tian, Z., Wang, X., Lee, R., Li, Y., Specht, J. E., Nelson, R. L., et al. (2010). Artificial selection for determinate growth habit in soybean. Proc. Natl. Acad. Sci. U.S.A. 107, 8563–8568. doi: 10.1073/pnas.1000088107
Wang, B., Smith, S. M., and Li, J. (2018). Genetic regulation of shoot architecture. Annu. Rev. Plant Biol. 69, 437–468.
Wang, C., Ma, Qi, Xie, X., Zhang, X., Yang, D., Su, J., et al. (2022). Identification of favorable haplotypes/alleles and candidate genes for three plant architecture-related traits via a restricted two-stage multilocus genome-wide association study in upland cotton. Crop. Prod. 177:114458.
Wang, P., Zhang, S., Qiao, J., Sun, Q., Shi, Q., Cai, C., et al. (2019). Functional analysis of the GbDWARF14 gene associated with branching development in cotton. Peer J. 7:e6901. doi: 10.7717/peerj.6901
Wen, T. W., Dai, B. S., Wang, T., Liu, X. X., You, C. Y., and Lin, Z. X. (2019). Genetic variations in plant architecture traits in cotton (Gossypium hirsutum) revealed by a genome wide association study. Crop J. 7, 85–92.
Wigge, P. A., Kim, M. C., Jaeger, K. E., Busch, W., Schmid, M., Lohmann, J. U., et al. (2005). Integration of spatial and temporal information during floral induction in Arabidopsis. Science 309, 1056–1059. doi: 10.1126/science.1114358
Wu, H., Ren, Z., Zheng, L., Guo, M., Yang, J., Hou, L., et al. (2021). The bHLH transcription factor GhPAS1 mediates BR signaling to regulate plant development and architecture in cotton. Crop J. 9, 1049–1059.
Yang, Z., Zhang, C., Yang, X., Liu, K., Wu, Z., Zhang, X., et al. (2014). PAG1, a cotton brassinosteroid catabolism gene, modulates fiber elongation. New Phytol. 203, 437–448. doi: 10.1111/nph.12824
Yao, R., Ming, Z., Yan, L., Li, S., Wang, F., Ma, S., et al. (2016). DWARF14 is a non-canonical hormone receptor for strigolactone. Nature 536, 469–473. doi: 10.1038/nature19073
Zhan, J., Chu, Y., Wang, Y., Diao, Y., Zhao, Y., Liu, L., et al. (2021). The miR164-GhCUC2-GhBRC1 module regulates plant architecture through abscisic acid in cotton. Plant Biotechnol. J. 19, 1839–1851. doi: 10.1111/pbi.13599
Zhang, B., Li, C., Li, Y., and Yu, H. (2020). Mobile TERMINAL FLOWER1 determines seed size in Arabidopsis. Nat. Plants 6, 1146–1157. doi: 10.1038/s41477-020-0749-5
Keywords: cotton, plant architecture, genetic improvement, florigen and antiflorigen, flowering transition
Citation: Huang X, Liu H and Ma B (2022) The Current Progresses in the Genes and Networks Regulating Cotton Plant Architecture. Front. Plant Sci. 13:882583. doi: 10.3389/fpls.2022.882583
Received: 24 February 2022; Accepted: 12 May 2022;
Published: 09 June 2022.
Edited by:
Roisin C. McGarry, University of North Texas, United StatesReviewed by:
Shoupu He, National Key Laboratory of Cotton Biology, Institute of Cotton Research (CAAS), ChinaCopyright © 2022 Huang, Liu and Ma. This is an open-access article distributed under the terms of the Creative Commons Attribution License (CC BY). The use, distribution or reproduction in other forums is permitted, provided the original author(s) and the copyright owner(s) are credited and that the original publication in this journal is cited, in accordance with accepted academic practice. No use, distribution or reproduction is permitted which does not comply with these terms.
*Correspondence: Xianzhong Huang, SHVhbmd4ekBhaHN0dS5lZHUuY24=
Disclaimer: All claims expressed in this article are solely those of the authors and do not necessarily represent those of their affiliated organizations, or those of the publisher, the editors and the reviewers. Any product that may be evaluated in this article or claim that may be made by its manufacturer is not guaranteed or endorsed by the publisher.
Research integrity at Frontiers
Learn more about the work of our research integrity team to safeguard the quality of each article we publish.