- 1Wheat Biotechnology Lab, Agricultural Biotechnology Division, National Institute for Biotechnology and Genetic Engineering, Constituent College Pakistan Institute of Engineering and Applied Sciences, Faisalabad, Pakistan
- 2Center for Desert Agriculture, King Abdullah University of Science and Technology, Thuwal, Saudi Arabia
The ensuing heat stress drastically affects wheat plant growth and development, consequently compromising its grain yield. There are many thermoregulatory processes/mechanisms mediated by ion channels, lipids, and lipid-modifying enzymes that occur in the plasma membrane and the chloroplast. With the onset of abiotic or biotic stresses, phosphoinositide-specific phospholipase C (PI-PLC), as a signaling enzyme, hydrolyzes phosphatidylinositol 4,5-bisphosphate (PIP2) to generate inositol 1,4,5-trisphosphate (IP3) and diacylglycerol (DAG) which is further phosphorylated into phosphatidic acid (PA) as a secondary messenger and is involved in multiple processes. In the current study, a phospholipase C (PLC) signaling pathway was investigated in spring wheat (Triticum aestivum L.) and evaluated its four AtPLC5 overexpressed (OE)/transgenic lines under heat and osmotic stresses through 32Pi radioactive labeling. Naturally, the wheat harbors only a small amount of PIP2. However, with the sudden increase in temperature (40°C), PIP2 levels start to rise within 7.5 min in a time-dependent manner in wild-type (Wt) wheat. While the Phosphatidic acid (PA) level also elevated up to 1.6-fold upon exposing wild-type wheat to heat stress (40°C). However, at the anthesis stage, a significant increase of ∼4.5-folds in PIP2 level was observed within 30 min at 40°C in AtPLC5 over-expressed wheat lines. Significant differences in PIP2 level were observed in Wt and AtPLC5-OE lines when treated with 1200 mM sorbitol solution. It is assumed that the phenomenon might be a result of the activation of PLC/DGK pathways. Together, these results indicate that heat stress and osmotic stress activate several lipid responses in wild-type and transgenic wheat and can explain heat and osmotic stress tolerance in the wheat plant.
Introduction
Sustainability in agriculture depends on growing suitable crops for a particular climate in the defined areas. Prolonged exposure to high temperatures drastically affects crop productivity. Elevated temperatures also result in osmotic stress from the water evaporation within the soil causing excessive salt accumulation. Heat, drought, and salt are the major abiotic stresses affecting crop yield. These stresses in combination are becoming quite common in heat and drought-hit areas. Among cereals, wheat is domesticated first and considered a major staple food crop globally (Tack et al., 2015; Abhinandan et al., 2018). Heat negatively affects the wheat grain yield. It is estimated that every 1°C rise in temperature results in 6% yield losses in wheat crops; however, it depends on the specific growth stage of the crop, time, duration, and intensity. An increase in temperature above the optimum value before and during anthesis results in embryo abortion in developing seeds, reducing the grain number/ear without affecting the grain weight, whereas, after anthesis, the onset of high temperature does not affect the number of grains per ear but reduce the grain size and weight by hampering grain filling ultimately affecting the crop yield (Foulkes et al., 2002; Weldearegay et al., 2012; Schmidt et al., 2020).
Plants are sessile eukaryotes and are very sensitive to even slight changes in their environment. There are some receptors present on the plant cell membrane that perceive stress (abiotic/biotic) signals and transduce this information downstream for the activation of certain stress-responsive genes. The ultimate product of coordinated action of these genes results in signal transcription/proteins synthesis, protein modification like ubiquitination, glycosylation, methylation, adaptors attachment, and subsequently scaffolding of the plants to adapt/survive under harsh environmental conditions (Trewavas and Malho, 1997; McCarty and Chory, 2000; Gilroy and Trewavas, 2001; Mahajan and Tuteja, 2005; Tuteja, 2007; Tuteja and Sopory, 2008).
Under extreme temperatures, plants tend to maintain their membrane integrity and fluidity, acting as a permeable barrier. According to a rough estimate, the membrane surface of a plant cell is recycled every 90–120 min (Munnik et al., 2021). These lipids have amphipathic properties and can be differentiated as sphingolipids, glycerolipids, and sterols based on their unique chemical structure and biophysical properties (Enrique Gomez et al., 2017). Among glycerolipids, phospholipids are predominantly present in the mitochondrial envelope and plasma membrane (PM), which play a vital role in the development of the plant, regulating their responses against particular environmental stimuli (Dubots et al., 2012; Niu and Xiang, 2018; Wang X. et al., 2020). Plant phospholipases are involved in the hydrolysis of phospholipids and can be divided into four categories, that is, PLA 1 (phospholipase A1), PLA 2 (phospholipase A2), PLC (phospholipase C), and PLD (phospholipase D). Within each category, there are subfamilies with different structures, substrates, and binding sites (Wang X. et al., 2020). Three types of PLCs are reported based on their cellular function and substrates specificity: (1) PI-PLC (Phosphatidylinositol-specific PLCs) hydrolyzes phosphoinositide (PPI); (2) PC-PLC/NPC (phosphatidylcholine-specific PLC/Non-specific phospholipase C) hydrolyzes the commonly present phospholipids like PC and PE; and (3) GPI-PLC (Glycosyl phosphatidylinositol PLC) hydrolyzes the proteins attached to the glycosylphosphatidylinositol (GPI) (Hong et al., 2016).
Extracellular signals activate the PLCs responsible for the production of inositol 1,4,5 trisphosphate (InsP3) and diacylglycerol (DAG). InsP3 travels to the cytoplasm to bind and activate the ligand-gated calcium channel also known as the InsP3 receptor to release Ca+2 from intracellular channels, whereas, DAG deals with the protein kinase C (PKC) family which has a C1 conserved domain. Massive intracellular processes due to increase or decrease in calcium and phosphorylation levels result in the activation and deactivation of various target proteins to respond against extracellular changes (Shiva et al., 2020; Hayes et al., 2021).
The signaling pathway of plant PI-PLC is somewhat different from mammals, for example, in plants, inositol 1, 4, and 5 trisphosphates (InsP3) could phosphorylate further to inositol hexakisphosphate (InsP6), which is responsible for the release of calcium ions from intracellular calcium reserves and similarly, phosphatidic acid (PA) which is a product of diacylglycerol (DAG) might act as a second messenger in this pathway (Munnik, 2014). PIP2 is presumably a substrate of PLC, hardly found in the plasma membrane of flowering plants (Simon et al., 2014; Zhang et al., 2018c). PLC hydrolyze PIP (Phosphatidylinositol 4 monophosphate), that is, also known as the precursor of PLC and can be found in abundance in the plasma membrane, but to date, the typical precursor of PLC in plants is unknown in in vivo analysis (Munnik, 2014). Likewise, DGPP (Diacylglycerol pyrophosphate) can function as an attenuator of PA signaling and as a generator of new signals, but it needs to be investigated further (van Schooten et al., 2006).
Plants tend to upregulate many PLC genes upon the onset of various biotic and abiotic stresses. In Arabidopsis thaliana, 9 PI-PLCs and 6 NPCs genes (Munnik, 2014), 4 PI-PLC and 5 NPCs in Oryza sativa (Rice) (Singh et al., 2013), 12 PI-PLCs and 9 NPCs genes in Gossypium spp. (Cotton) (Zhang et al., 2018a), 5 PI-PLC and 4 NPCs genes in Zea mays L. (Maize), while 12 PLC genes in Glycine max (Soybean) (Wang F. et al., 2015) are reported. An increase in PIP2 and PA had been observed in response to heat, salt, cold, drought, and ABA stresses (Alcázar-Román and Wente, 2008; Darwish et al., 2009; Mishkind et al., 2009; Arisz et al., 2013; Balogh et al., 2013; Simon et al., 2014; Zhang et al., 2018c). Earlier it is reported that PLC is involved in plant growth and development, for example, PLC1 is known to contribute to pollen tube growth in tobacco and petunia (Dowd et al., 2006; Helling et al., 2006), over-expression of the PLC2 gene can increase drought tolerance and regulate phytochrome level in Brassica napus (Das et al., 2005; Nokhrina et al., 2014), PLC3 and PLC9 contributing in generating thermotolerance in Arabidopsis thaliana (Zheng et al., 2012; Gao et al., 2014), upregulation of AtPLC5 in response to drought stress could lead to subsequent novel phenotype including stunted root hair growth, reduced lateral root development, stomatal closure, and inhibition/reduction of seed germination (Zhang et al., 2018b,c). These findings are inconsistent with previous studies as reported on maize, tomato, and potato (Apone et al., 2003; Apostolakos et al., 2008; Wang et al., 2008; Vossen et al., 2010).
PI-PLC was initially reported in wheat in root plasma membrane vesicles (Melin et al., 1992). Based on their subfamily, genomic homology, and chromosomal position, a total of 26 TaPLC genes including 7 NPC genes have been reported in Triticum aestivum (wheat) which are located unevenly on 14 chromosomes (Wang X. et al., 2020), but to date, TaPLC1 (Zhang et al., 2014; Wang X. et al., 2021) and TaPI-PLC1-2B have been cloned and investigated for salt, drought, heat, and cold stress (Khalil et al., 2011; Wang X. et al., 2020). However, TaPLC5 has yet to be reported in the already identified wheat PLCs. There is growing evidence that phosphoinositide signaling is a major element of stress responses. It proposes that changes in the lipid signal levels are one of the early consequences of abiotic stresses. Therefore, this study focuses on investigating signaling phospholipids levels in response to high temperature and osmotic stresses. We observed that AtPLC5 over-expression causes a dramatic increase in PIP2 and PA levels at tillering and anthesis stages. These are the crucial stages for wheat grain development at various duration in varying intensity levels of heat and osmotic stresses.
Materials and Methods
Plant Material
Seeds of local wheat cultivar Faisalabad-2008 was used as wild-type (Wt) and four transgenic wheat lines over-expressing (OE) AtPLC5 gene were used in the current study. The transgenic lines OE1 and OE2 were processed under CaMV35S promoter, while lines OE3 and OE4 contained UBQ10 promotor. These transgenic wheat lines were obtained through Agrobacterium-mediated plant transformation method using immature embryos as explant (Ishida et al., 2015), and putative transgenic wheat lines were screened out based on PCR, quantitative PCR, and antibiotic leaf dip assay. Nevertheless, morpho-physiologically best representative lines were selected and used in this study (unpublished data). Plants were grown in small pots containing peat moss in a greenhouse with a 16/8 h day length regime at 20°C. Leaf samples from transgenic wheat lines were collected from the greenhouse and processed for further experimentation.
RNA Extraction and Q-PCR
The expression level of AtPLC5 (At5g58690) transgene in wheat was measured using primer pairs: 5’GT CGCTTTCAACATGCAGGG3’ and 5’TGGGTAACTTCGCTTT CGGG3’. Trizol reagent (Invitrogen, United States) was used for the extraction of RNA followed by DNases treatment. RevertAid First-strand cDNA synthesis kit (ThermoFisher Scientific, EU, Luthiana) was used for cDNA synthesis. A comparative threshold cycle value was used to determine the relative expression of the gene. Actin gene (AB181991.1) with primer pair 5’AA CTGGGATGACATGGGGAA3’ and 5’TTTTCTCTCTGTTGG CCTTGGG3’ was used for normalization of transcript level.
32Pi Labeling and Heat and Osmotic Stress Treatment
Leaf discs of 0.5 cm in size were taken from the center of collected leaf samples with the help of a vertical leaf disc puncher (Supplementary Figure 1). Two leaf discs for every replicate were taken. Leaf discs were metabolically labeled using labeling buffer 200 μl (MES-KOH 2.5 mM, pH 5.8, KCl 1 mM) containing carrier-free PO4–3 (5–10 μCi) in 2 ml Eppendorf tubes for overnight incubation, as described by Munnik et al. (1998) and Darwish et al. (2009). For PLD activity assay, n-butanol (0.5% v/v) was used as transphosphatidylation substrate (Darwish et al., 2009).
Heat Treatment
After overnight incubation for 32Pi labeling, samples were subjected to heat stress at 40°C using a heat block for the mentioned period of time, that is, 0, 7.5, 15, 30, and 60 min.
Osmotic Stress Treatment
For osmotic stress, 3–4-week-old leaf samples were treated with/without sorbitol by adding 200 μl of sorbitol in MES labeling buffer for 30 min and at 0, 600, and 1200 mM concentrations.
Lipid Extraction and Analysis
Treatments were stopped by adding PCA (Perchloric acid) to the Eppendorf tubes and centrifuged at 13,000 rpm for 30 s. Leaving behind the leaf tissues in the tube, all the remaining material was discarded carefully, then 400 μl CMH [CHCL3/MeOH/HCl (50:100:1, by volume)] was added in the same tube and shook them for 5 min (until tissues turned colorless). By adding 400 μl of CHCL3 and 200 μl of NaCl (0.9% w/v), two-phase system was induced followed by 2 min centrifugation at 13,000 rpm. The rest of the lipid extraction and isolation was carried out by Munnik and Zarza (2013). Heat-activated K-oxalate (KOX–) impregnated TLC plates, using an alkaline solvent containing CHCL3, MeOH, 25% NH3 and H2O [90:70:4:16] constituents or an ethyl acetate system containing: EtAc/iso-octane/HCOOH/H2O (12:2:3:10, by vol.) were used to separate radioactive lipids (Munnik et al., 1998). Radioactively labeled phospholipids were visualized on an autoradiograph by overnight exposure of TLC plate to autoradiography film and quantified by using phosphoimaging (Typhon FLA 7000, GE Healthcare).
Performance of Transgenic Lines of Wheat Under Heat and Combination of Stresses
Wild-type and transgenic lines (AtPLC5OE) of wheat were grown in pots under optimum conditions. These plants were subjected to heat stress (40°C) and drought together with heat stress (500 ml H2O + 40°C) in combination at the anthesis stage for 3 h daily for 14 days. Wild-type and transgenic lines of wheat were also grown at optimum temperature (25°C) as a control. Stay green character was recorded based on visual observation and leaf greenness. Data were recorded and analyzed in percentages.
Results
Expression of AtPLC5 in Wheat Under Heat Stress
The expression level of wild-type and AtPLC5 OE lines of wheat were determined by Q-PCR, relative to the expression of actin gene. For this, wild-type and transgenic lines (AtPLC5 OE) of wheat were subjected to heat stress at 40°C for 3 h at the anthesis stage. Leaf samples were collected immediately and stored in liquid nitrogen for further processing. Little to no expression was observed in Wt. Relative expression of AtPLC5 shows a significant increase in all four over-expression transgenic lines of wheat (Figure 1A). The transgenic lines OE1 and OE2 showed 9.9X and 12.3X, while OE3 and OE4 lines showed 36.1X and 27.3X significant increase in the expression levels in comparison to the wild-type.
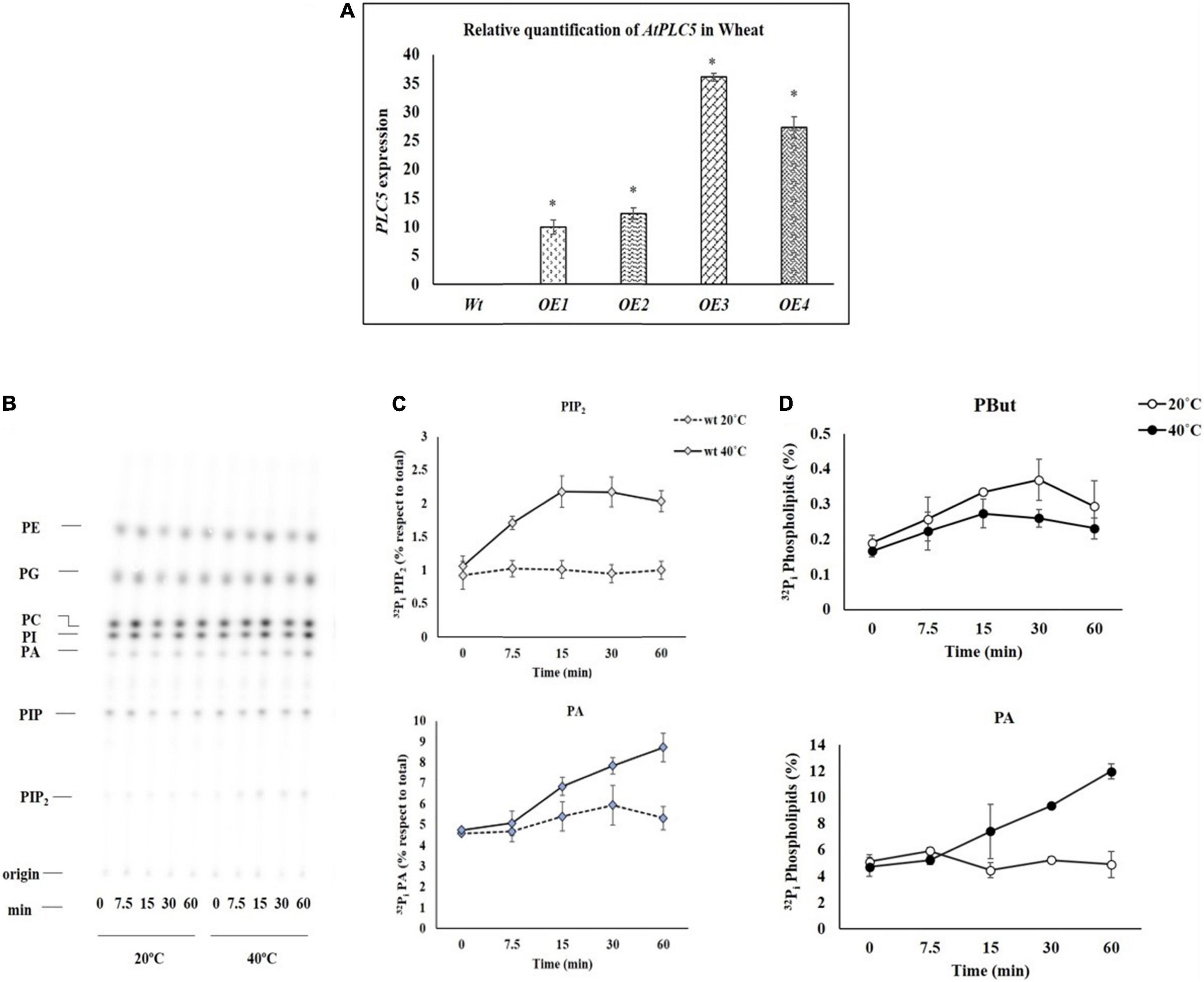
Figure 1. (A) The relative expression of AtPLC5 transgenes in wheat normalized with actin gene determined through Q-PCR with a significant level P < 0.05 (*). (B) Heat stress excitation of PIP2 and PA formation in a time-dependent manner. Wheat leaf discs labeled with 32Pi and tested at 20°C (control) and 40°C (heat stress) for 0, 7.5, 15, 30, and 60 min time periods. An autoradiograph of a time-course experiment with two different temperatures, each lane representing extract (1/5th) of two leaf discs of two different leaves. (C) Quantification of PIP2 and PA, respectively, after heat treatment for said time. The experiment was independently repeated two times and similar results were obtained. (D) PLD activity. Pre-labeled wheat leaf discs were subjected to heat stress for said time periods in the presence of n-butanol (0.5% v/v). Lipids were extracted and separated by EtAc TLC. Quantification of PBut and PA levels was done by phosphoimaging. Abbreviations: PIP2, Phosphatidylinositol 4,5-bisphosphate; PIP, Phosphatidylinositol phosphate; PA, Phosphatidic acid; PI, Phosphatidylinositol; PC, Phosphatidylcholine; PE, Phosphatidylethanolamine; PG, Phosphatidylglycerol; CL, Cardiolipin.
Heat Stress Rapidly Stimulates Phosphatidylinositol 4,5-bisphosphate and Phosphatidic Acid Accumulation
To study the effect of the heat stress in wheat, the leaf disc of Wt was labeled with 32P isotope by keeping the leaf discs for overnight incubation in MES buffer and exposed to 20°C and 40°C by using heat block for 0, 7.5, 15, 30, and 60 min. Then, Perchloric acid (2.4% final concentration) was added to stop the reaction and crude lipids were extracted. Alkaline TLC (thin layer chromatography) plates were used to separate the lipids that were further quantified by phosphoimaging.
To investigate how fast the PIP2 and PA start to produce when subjected to heat stress, leaf discs of 4-week-old seedlings of wheat were exposed to heat stress for different time durations. The results of the time course experiment are presented in Figure 1B. The PIP2 and PA responses increased with the increase in duration of exposure to heat stress in a time-dependent manner (Mishkind et al., 2009), expression of PIP2 increased up to 2.2-fold, and PA increased up to 1.6-fold (Figure 1C) depending on the time of exposure.
Assay for Phospholipase D Activity
An experiment was carried out to investigate the distinct route of heat-induced PA generation. Either it occurs through PLC which cleaves PIP2 into IP3 and DAG that are further phosphorylated by DGK enzyme to generate PA or PA generation directly through PLD. Therefore, transphosphatidylation activity of PLD was employed. For this, pre-labeled leaf discs were subjected to heat stress (20°C and 40°C) at said time intervals in the presence of n-butanol (0.5% v/v). Ethyl acetate TLC was used to separate lipids and to track PLD-catalyzed phosphatidyl butanol (PBut) formation by phosphoimaging. Under these conditions, a small increase was observed in the PBut level at some time points, while a decrease in PA level was observed. In contrast, a simultaneous decrease in the accumulation of PBut level was observed during subsequent incubation at 40°C with an increase in the level of PA (Figure 1D).
Mature Leaves Accumulate More Phosphatidylinositol 4,5-bisphosphate
Differential response of leaves of the same tiller of the same wheat plant was analyzed for accumulation of PIP2 upon exposure to heat stress. An experiment was designed to investigate which leaf (either younger or mature leaves) responds more efficiently to heat stress by producing a sufficient amount of PIP2, PIP, and PA, and four different leaves including the newly emerged leaf of the same tiller of Faisalabad-2008 wheat cultivar were taken and labeled radioactively by overnight incubation. 32Pi labeled leaf discs of four different leaves were subjected to heat stress at 21°C and 40°C for 15 min (Figure 2A). The results demonstrated a considerable gradual increase in PIP2 and PA levels among leaves with the increase in temperature, while the level of PIP declined (Figure 2B) upon receiving heat stress as described previously (Mishkind et al., 2009). The mature leaves showed a 3.3-fold increase in PIP2 and a 2.6-fold in PA but a 1-fold gradual decrease in PIP production was observed as compared to younger leaves (Figure 2C; Wang X. et al., 2021).
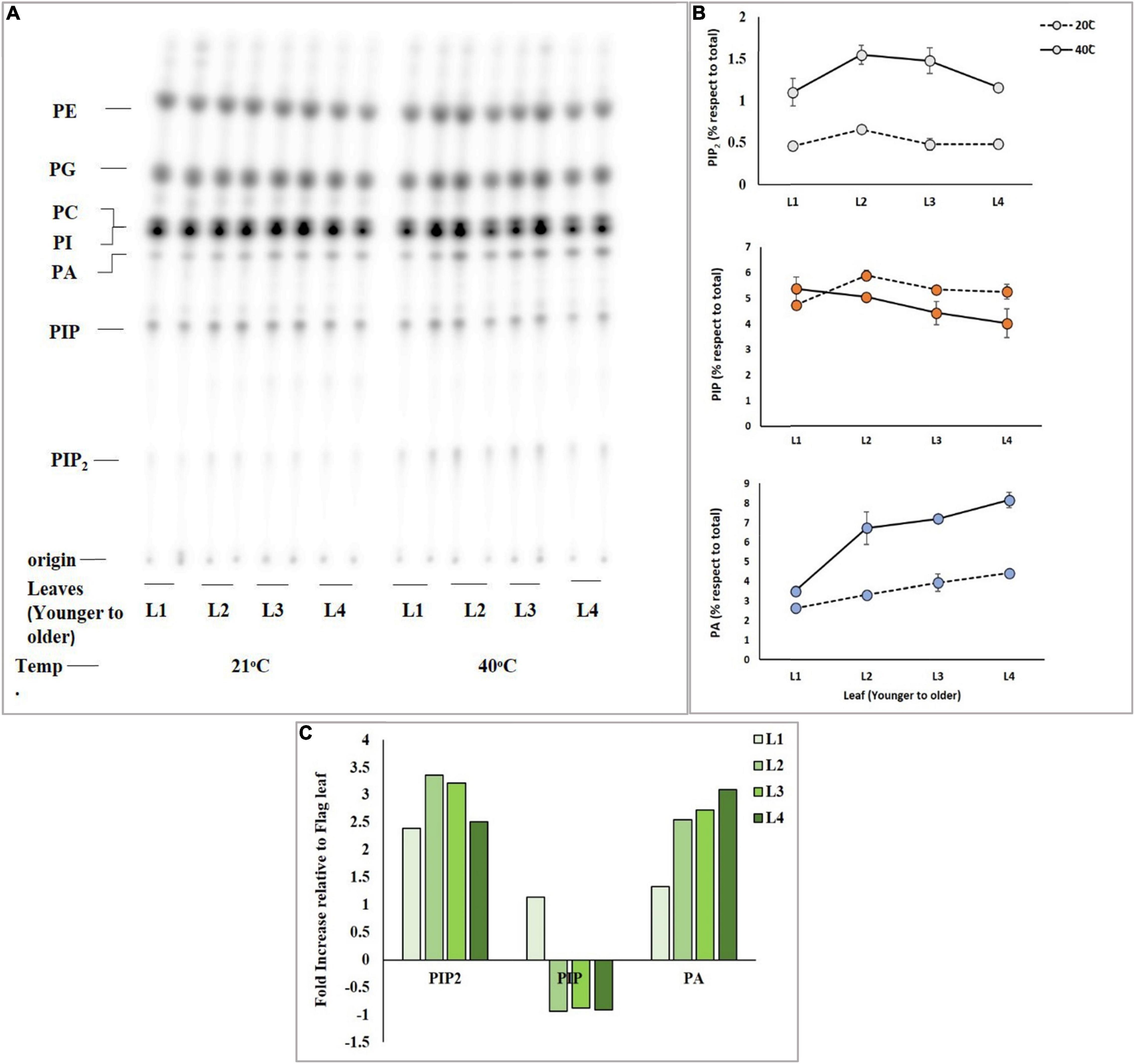
Figure 2. High temperature triggers the rapid accumulation of PIP2 and PA in mature leaves. Wheat leaf discs were pre-labeled overnight with 32PO43–, incubated for 15 min at 21°C and 40°C, and lipid extraction and separation were carried out by using alkaline TLC. Panel (A) shows autoradiograph of lipid TLC. (B) PIP2, PIP, and PA levels were quantified by densitometry of autoradiograph shown in panel (A). (C) Summary of fold increase in levels of PIP2, PIP, and PA relative to t = 0. The experiment was repeated three times independently and similar results were obtained. See Figure 1 for the definition of abbreviation.
Phosphatidylinositol 4,5-bisphosphate Level Increases at Anthesis Stage in Response to Heat Stress
The wheat anthesis stage is very sensitive to high temperatures. A rise in temperature beyond 25°C drastically affects pollen viability, decreases the chances of seed setting, and results in lesser crop yield. The lipid profile of transgenic wheat plants containing two different promoters and their response to heat stress at the anthesis stage was determined by subjecting their labeled leaf discs to 40°C for 30 min (Figure 3A). The lipid profile patterns showed a rise in PIP2 levels in response to heat stress in transgenic and wild-type wheat plants (Figure 3B). The PIP2 level revealed a significant increase in the transgenic lines under different promotors in comparison to the wild-type. While the wild-type showed little to no increase, the transgenic lines, OE1 and OE2, depicted a 2.0- to 2.5-fold increase, whereas, OE3 and OE4 transgenic plants showed ∼4.5-fold and 4-fold increase in PIP2 production, respectively (Figure 3C).
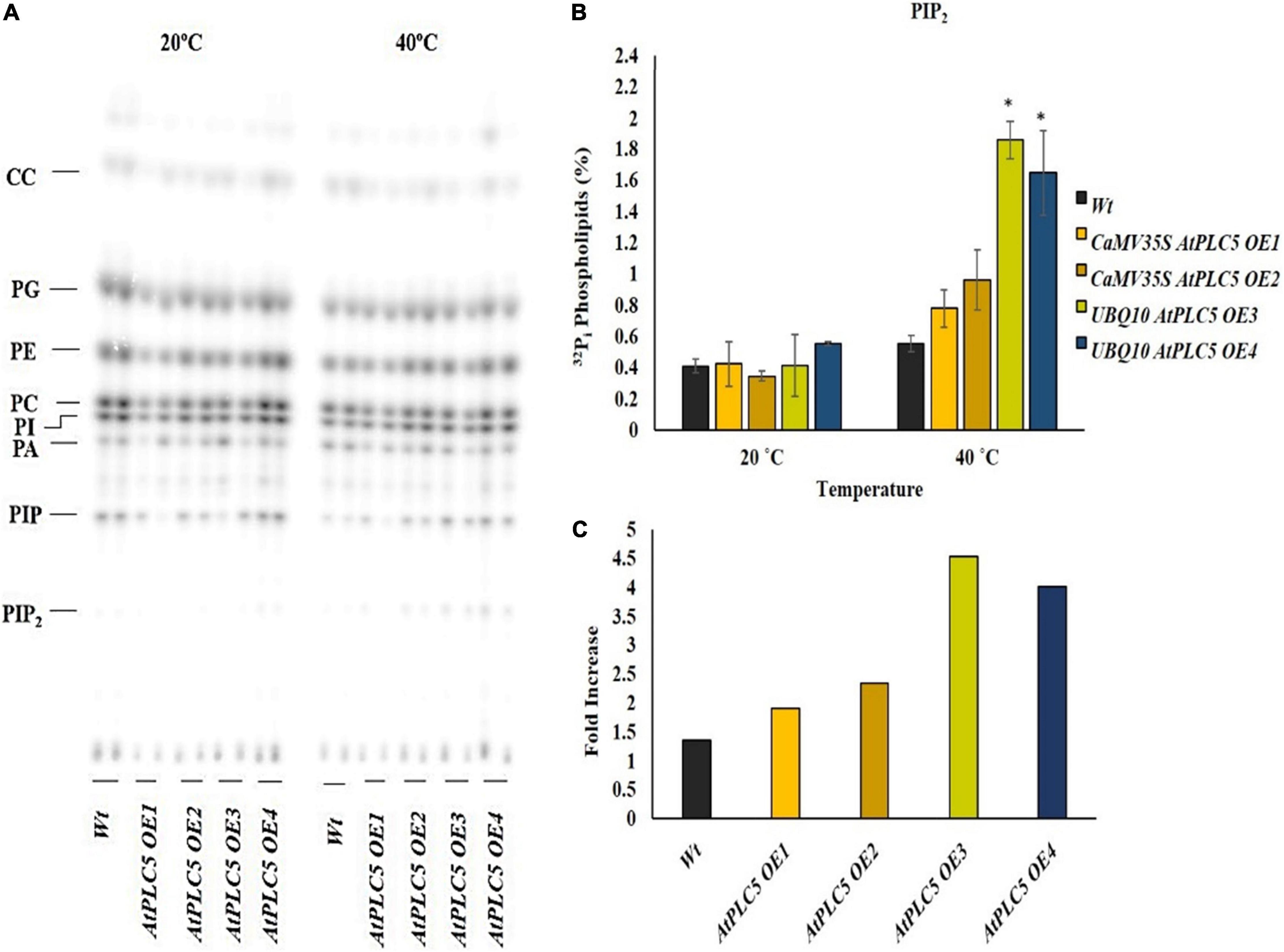
Figure 3. Heat stress increases the production of PIP2 at the anthesis stage. A small section (∼0.5 cm) of leaf disc of transgenic wheat lines and wild-type were taken at the anthesis stage, radioactively labeled O/N with 32Pi, and incubated for 30 min at 20°C and 40°C using a heat block. (A) An autoradiograph of alkaline TLC showing a complete lipid profile. (B) Quantified level of PIP2 after heat treatment at 20°C and 40°C. (C) Summary of fold increase transgenic lines show with respect to wild-type. Student t-test was used to determine significant differences between wild-type and transgenic lines of AtPLC5 at a significance level of P < 0.05 (*).
Osmotic Stress Triggers the Phosphatidylinositol 4,5-bisphosphate Production in AtPLC5 Over-Expressing Wheat Lines
The role of osmotic stress in the production of lipid was analyzed in Wt and AtPLC5 over-expressing wheat lines. 32Pi labeling of 4-week-old plant leaf discs was performed to test various concentrations of sorbitol to mimic water stress. Leaf discs were treated with 0, 600, and 1200 mM sorbitol pre-dissolved in MES labeling buffer for 30 min before extraction. Five percent perchloric acid (PCA) was used to stop preceding the reaction further and crude lipids were extracted. Potassium oxalate (KOX–)-treated TLC plates were used to separate the lipids and phosphoimaged for quantification purposes (Figure 4A).
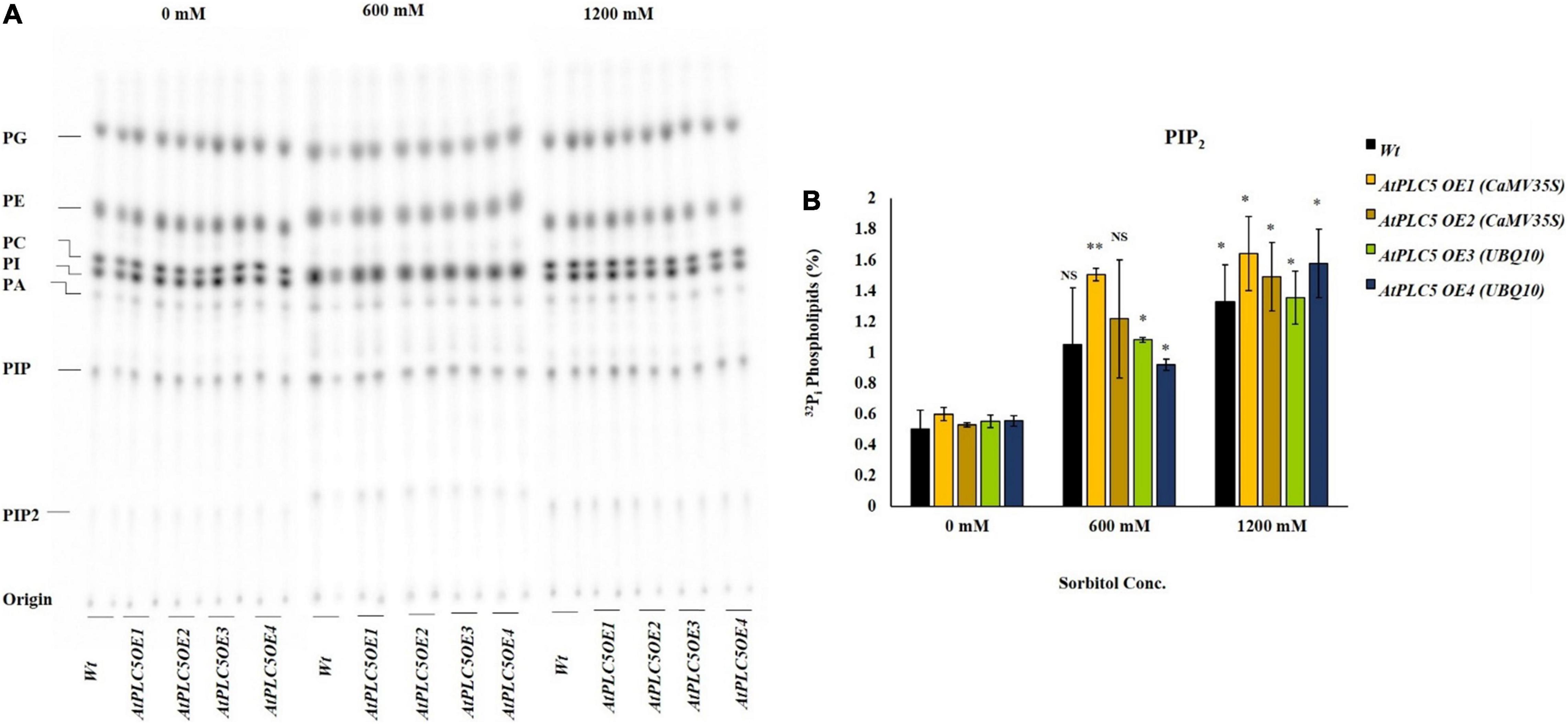
Figure 4. PIP2 accumulation in wheat AtPLC5 OE lines at different levels of osmotic stress. Overnight labeled leaf disc treated with different concentrations of sorbitol (0 mM, 600 mM, 1200 mM) dissolve in MES buffer for 30 min. Lipids were extracted, separated, and quantified by phosphoimaging. (A) A typical autoradiograph of structural lipids with each lane containing lipid extract of two leaf discs. (B) 32Pi level of PIP2 of the wild-type and AtPLC5 OE transgenic lines of wheat under control conditions and at said sorbitol concentrations. Data represented as means ± SD (n = 2). Two independent experiments were carried out with similar results obtained. Statistically significant differences at P < 0.05 (*) and at P < 0.01 (**) were observed in AtPLC5 OE of wheat at different sorbitol concentrations, based on the student’s t-test.
Under control conditions, the amount of PIP2 remained the same among AtPLC5 OE lines and wild-type (Figure 4B). A relative significant [P < 0.05 (*), P < 0.01 (**)] increase in PIP2 level was observed in AtPLC5 OE lines (OE1, OE2, OE3, and OE4) under different promoters at 600 mM sorbitol concentration, while a non-significant increase was observed in wild-type. Upon sorbitol treatment of 1200 mM, a significant increase in PIP2 level was observed in wild-type (∼2.7-fold) and AtPLC5 over-expression lines (∼3.3-fold) as compared to control condition, whereas non-significant differences were observed between the wild-type and AtPLC5-OE lines at 600 mM and 1200 mM sorbitol concentrations. However, the AtPLC5 OE4 line showed a significant (P < 0.05) increase (∼1.8 and ∼3.2-fold) in the PIP2 level at 600 mM and 1200 mM sorbitol treatment, respectively. The PA and PIP responses in wild-type and AtPLC5 OE lines appeared to be almost similar (a slight increase was observed in AtPLC5 over-expression lines) at said levels of sorbitol concentrations.
Combination of Heat and Osmotic Stress Elicit Phosphatidylinositol 4,5-bisphosphate Accumulation in AtPLC5 Over-Expression Line
Usually, owing to the duration of the wheat cultivation, the crop faces several stresses at the same time. The occurrence of more than one stress in combination severely affects plant growth and development. Moreover, any visible symptom of heat and osmotic stress cannot be detected at the early stages of plant growth. To determine the response of AtPLC5 in transgenic wheat under the combination of heat and osmotic stress conditions, 4-week-old plantlets were tested at 40°C and 600 mM sorbitol for 30 min simultaneously.
The amount of PIP2 under control/non-treated conditions was observed (Figure 5A) to be the same among the AtPLC5 OE4 line and wild-type (Figure 5B). A relative increase in PIP2 was observed at a significance level of P < 0.05 (*) in wild-type and AtPLC5 OE lines (containing UBQ10 promoter) at 600 mM sorbitol concentration at 40°C temperature when compared to the control condition. Under co-stress conditions, a significant increase of 2.8-folds in PIP2 was observed in wild-type and 3.5-folds in AtPLC5 over-expression line in a controlled environment.
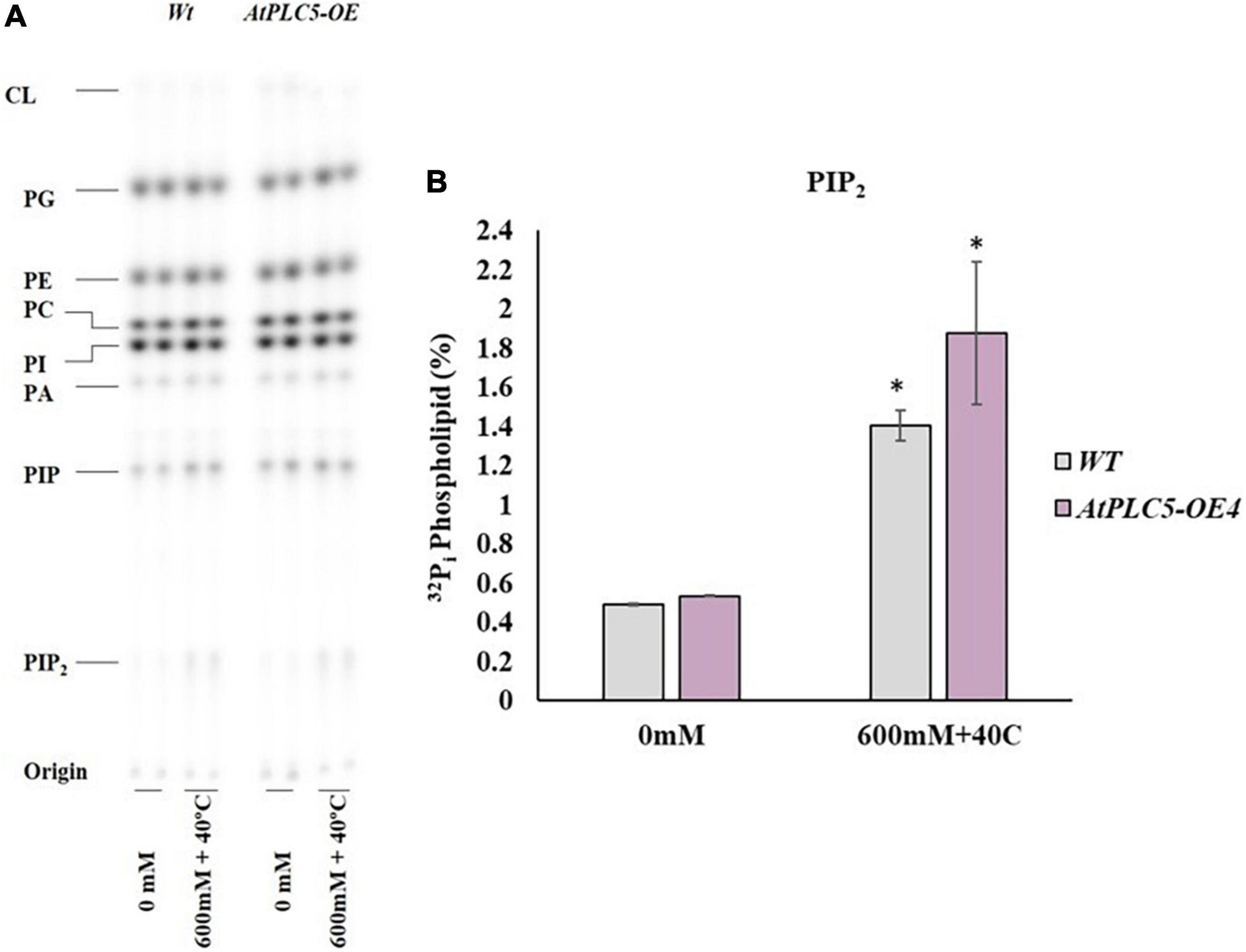
Figure 5. The occurrence of stresses in combination elicits PIP2 accumulation in AtPLC5 OE4 lines in wheat. Radioactively labeled leaf discs were treated with 600 mM sorbitol at 40°C for 30 min. Lipids were extracted, separated by Thin Layer Chromatography plates, and quantified by phosphoimaging. (A) A typical autoradiograph of lipids with each lane containing 1/5th lipid extract of two leaf discs. (B) 32Pi level of PIP2 of the wild-type and AtPLC5 OE4 line under control conditions and at co-stress of heat and osmotic stress situation. Data represented as means ± SD (n = 2). Two independent experiments were carried out with similar results. Statistically significant differences at P < 0.05 (*) were observed between the AtPLC5 OE4 line of wheat and wild-types under said stress conditions, based on the student’s t-test.
Performance of AtPLC5 Overexpression Line Under Abiotic Stress
To check the contribution of AtPLC5 overexpression in wheat physiology or its agronomic performance, two different experimental conditions were set up. First, we tested the physical response of AtPLC5 OE lines under heat stress at 40°C and second, when stress was applied in combination, such as heat with drought stress (40°C + 500 ml H2O). After the treatment of 2 weeks, we observed the stay-green character in Wt and AtPLC5 transgenics of wheat. We observed that at optimum conditions (32°C), Wt possesses ∼32%, while AtPLC5 transgenics possess ∼40% greenness (Figure 6). When stress was applied in combination with heat (40°C) and drought (500 ml water), we observed visible leaf necrosis in Wt (∼5% greenness) and AtPLC5 transgenic plants of wheat (∼25% greenness). Interestingly, we found that the transgenic plants of wheat that received treatment of heat stress (40°C) show the ∼70% stay-green character as compared to Wt.
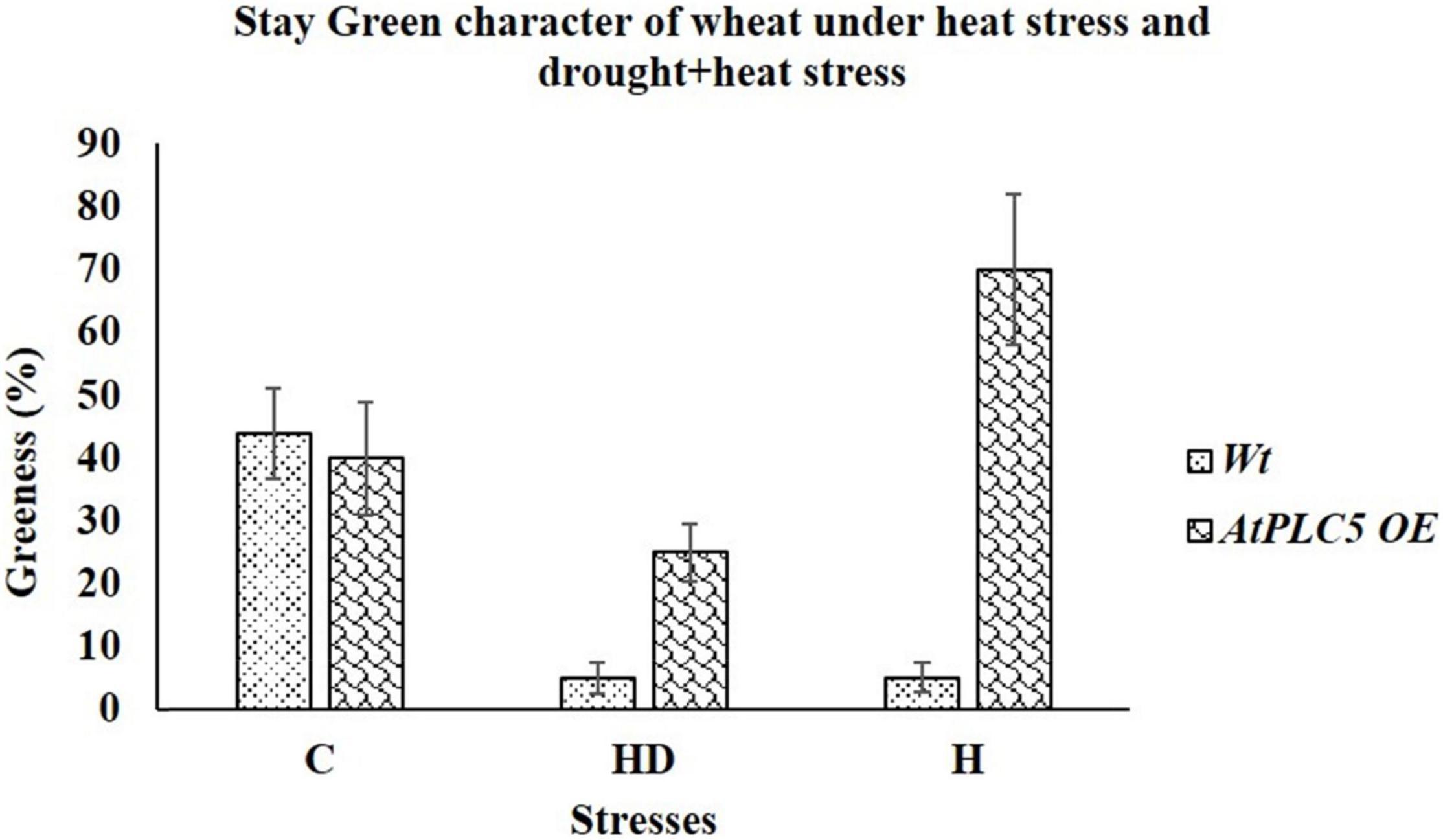
Figure 6. Morphological evaluation of Wt and AtPLC5 overexpression lines of wheat under heat stress and under the combined effect of heat and drought stress. Stay green character was recorded based on visual observation and leaf greenness. Data (n = 26, ±SD) were recorded and analyzed in percentage.
Discussion
Abiotic stresses can elicit a series of plant responses. Membrane plays an important role in vesicle transport and cell signaling not only through host-specific proteins but also provides a substrate for the production of lipid (as a second messenger). In addition to the role of lipids as components of membrane structure, they also work as a signal transducer, component of coordinated regulatory activator, and stimulate the expression of specialized proteins and trigger cellular responses to environmental cues (Hou et al., 2016; Kosová et al., 2018; Munnik et al., 2021). Phospholipases on the plasma membrane are the first receptors to receive environmental signals and respond accordingly. PLCs due to their regulatory roles in stress management have been extensively investigated in different plant species. It has been established that stress causes a synergistic increase in PIP2 levels and free calcium, which enhances IP3 synthesis and further releases cytosolic calcium through PI-PLC activity (Hunt et al., 2004; Gao et al., 2014; Zhang et al., 2014). Heat shock induces a rapid increase of Ca+2 in the cytoplasm, probably from intracellular reserves and extracellular sources. It is reported that Ca+2/calmodulin pathway is involved in thermotolerance. It is logical to claim that Ca+2 channels could be used as a thermosensor (Gao et al., 2012; Hayes et al., 2021). However, it is still a challenging task to identify the primary heat-activated Ca+2 channel.
Previously, PIP2 and PA abundance had been observed in Arabidopsis within 2 min of onset of heat (40°C) stress, and it was mediated by PLD and PIPK (Mishkind et al., 2009). In the current study, we investigated the stimulation of heat-induced PIP2 and PA accumulation in Triticum aestivum L. and observed that their induction proceeded in a time-dependent manner. The rapid rise in PIP2 level was evident with the onset of heat (40°C) that reached 2.2-folds in just 15 min and continue to increase with the increase in the duration of heat stress. However, after 60 min, the PIP2 level started to decline, which might indicate the stress-induced damage caused to the plasma membrane. In the current study, it was observed that the PA accumulation started just after 7.5 min of the onset of heat stress, and kept on increasing continuously with the increase in the duration of heat stress. The quick abundance of PIP2 and PA indicates the synthesis of these signaling lipids associated with thermosensing. Although it is still unclear how the elevated temperature activates these lipid-modifying enzymes, this increase in PIP2 and PA is either caused by PIP5K, PLC, or PLD activity, which is yet to be determined. It is reported previously that PA induction is closely associated with the activation of PLD under heat stress (Shiva et al., 2020; Hayes et al., 2021); however, it has been observed that in wheat at 40°C, PBut level seems to decrease while the total PBut content remains in lower limit. In contrast, PA level seems to increase in a time-dependent manner. It is still unknown which other factors are involved in the generation of PA through PLD or PLC. Similarly, it is yet to be explored what circumstances help in the activation/inhibition of PLD or PLC.
Plant leaves serve as a sensor for biotic and abiotic stresses. A slight change in the surrounding temperature is usually sensed by the plant through their leaves. The present study investigated PIP2 and PA responses in younger to older leaves against heat stress. We also observed PIP (Phosphatidylinositol monophosphate) response. Upon onset of heat stress (40°C), the young leaves depicted minor elevation in PIP2, PIP, and PA and contributed accordingly to stress responses as compared to mature leaves which showed a gradual increase up to 3.4-folds in PIP2 and PA accumulation, while illustrated 1-fold decrease in PIP level. Therefore, it could be suggested that although the younger leaves have actively dividing cells, they are quite sensitive to heat stress Zhang et al. (2014) reported a 16-fold increase in TaPLC1 expression level in older leaves upon salt and drought stress. This could be implied that an increase in expression in response to environmental changes might be considered an adaptive mechanism to manage abiotic stresses.
In the current study, PIP2 response was observed to be similar in wild-type (Faisalabad-2008) and AtPLC5 over-expressing lines of wheat under normal conditions (20°C). However, heat stress (40°C) at the anthesis stage caused a stronger and significant rise in PIP2 level in AtPLC5 over-expression lines (Figure 3B) as compared to wild-type that ultimately helped the plant to adapt/tolerate fluctuations in temperature and grain formation sustaining the crop yield. We also compared the strength of two constitutive promoters (CaMV35S and UBQ10). UBQ10 promoter indicated relatively higher expression of AtPLC5 in OE3 and OE4 lines with a consequent significant increase of ∼4.5-folds in PIP2 accumulation as compared to AtPLC5 expression driven under CaMV35S promoter in OE1 and OE2 transgenic wheat. Zhang et al. (2018c) reported a 12-fold increase in PIP2 level at the onset of osmotic stress in PLC5OE lines containing UBQ10 promoter in 6-day-old seedlings of Arabidopsis thaliana (Zhang et al., 2018c), which is in agreement with our findings and increase in PLC activity.
PI-PLC as a stress mediator had been reported along with their isoforms in many plants including maize (Apostolakos et al., 2008), rice (Darwish et al., 2009; Singh et al., 2013), tobacco (Helling et al., 2006), tomato (Vossen et al., 2010), cotton (Zhang et al., 2018a), soybean (Wang F. et al., 2015), brassica (Das et al., 2005), Arabidopsis (Gao et al., 2014), and wheat (Wang X. et al., 2021). Recent findings illustrated the over-expression of TaPLC1 aided in improved salt, drought, heat, and cold stress tolerance in wheat (Khalil et al., 2011; Wang Y. et al., 2020; Wang X. et al., 2021). PIP2, as a PLC substrate is hardly detected in plants’ plasma membrane under normal conditions, while its level significantly increased under osmotic stress, for example, cold, salinity, or heat stress (Darwish et al., 2009; Mishkind et al., 2009; Arisz et al., 2013; Munnik, 2014; Zhang et al., 2014). In the present study, it was observed that the lines that showed more PIP2 accumulation also revealed more transcript levels through real-time quantitative PCR. In addition, we also observed that these lines retained their stay green character relatively for a longer period of time when exposed continuously for 14 days to heat stress.
The structural lipids like PC (Phosphatidylcholine), PG (Phosphatidylglycerol), and PA (Phosphatidic acid) at the anthesis stage of wheat were reported to drop under high temperatures (Narayanan et al., 2016; Djanaguiraman et al., 2020). Likewise, we also observed a slight decrease in PA in our AtPLC5 over-expression lines of wheat during anthesis at 40°C. However, ∼2.2-fold increase in PA accumulation was observed in the wild when subjected to heat stress (40°C for 30 min). This increase might reflect the activity of PLD as previously reported by Hayes et al. (2021).
Upon rising environmental temperature, plants with sufficient water resources transpire more rapidly to keep their leaves cool, while on water scarcity in hot conditions, leaves close their stomata to prevent water loss through evaporation and to maintain their cells membrane integrity. Lee et al. (2007) reported PIP2 to be an important precursor for stomatal opening, as detected previously in the closed stomata phenotype of the PLC5OE line in Arabidopsis. In this study, sorbitol was used to mimic drought/osmotic stress in wheat and to observe its effect on the PIP2 level. Interestingly, a significant increase in PIP2 level was observed upon osmotic stress in AtPLC5 overexpression lines of wheat, this might result in the enhanced hydrolytic activity of PLC5 which might lead to an increase in PIP2 hydrolysis resulting in a subsequent increase in IP3 that might further be metabolized into IP6 which facilitate the stomatal closure by activating the release of Ca+2 from intracellular channels (Zhang et al., 2018b,c). In addition, in the current study, we observed that wheat transgenic lines containing CaMV35S promoter induced significantly higher PIP2 that matched with findings previously reported by Zhai et al. (2013). It is explained that ZmPI-PLC1 expressed under CaMV35S promoter induced drought tolerance in transgenic tobacco (Ruelland et al., 2015).
However, a detailed phosphoimager-based densitometry study demonstrated a meager decrease in PI and PE levels and a slight increase in PC and PG levels, when exposed to osmotic stresses. An increase in the level of cardiolipin (CL) was observed in OE lines of transgenic wheat. In plants, PG (phosphatidylglycerol) was found to be mainly present in the thylakoid membrane of chloroplast and supposed to be involved in the photosynthetic electron transport chain (Hagio et al., 2002; Kobayashi et al., 2017). Previous reports have suggested the prerequisite presence of PG for chloroplast biogenesis, as its deficiency yielded a pale-yellow green phenotype, indicating the failure of establishing thylakoid membrane networks inside leaf chloroplast (Haselier et al., 2010; Kobayashi et al., 2015). Interestingly, an increase in PG level of overexpressor lines of wheat was observed, which means they remained photosynthetically active when exposed to abiotic stress and could accumulate more synthates, more synthates mean more nutrients available to be assimilated during grain filling leading to enhanced crop productivity, which might ultimately yield higher grain and biomass.
Phosphatidylinositol 4,5-bisphosphate is claimed to be a PLC substrate in animals, its concentration is relatively hard to detect in the plasma membrane of plants where PLC activity mostly resides (Van Leeuwen et al., 2007; Munnik, 2014). In contrast to PIP2, PI4P is 20–30 times more abundant in plasma membrane under normal conditions. Under stress conditions such as abscisic acid (ABA), salinity, heat, or hyperosmotic stress, the level of PIP2 increased (Darwish et al., 2009; Mishkind et al., 2009; Zhang et al., 2018b), while the level of PI4P has been reported to drop in response to these stresses (Arisz et al., 2013). But does it go down due to conversion into PIP2 or PIP is an assumed substrate of PLC in the plant? Also, it remained debatable, whether this reflected the hydrolysis by phosphatase or a PLC or is a result of PIP5K activation. Further research is needed to decipher the exact role of PLC in wheat and the downstream process of PA, PPIs, and IPPs production and accumulation.
Data Availability Statement
The original contributions presented in the study are included in the article/Supplementary Material, further inquiries can be directed to the corresponding author.
Author Contributions
NA, MA, and NS conceived and designed the research. NA conducted the research experiments. NA and KI evaluated the data. MA, NS, and MT provided the research material. NA and MA wrote the manuscript. SM, MT, and NS critically reviewed and edited the manuscript. All authors contributed to the article and approved the submitted version.
Funding
This work was supported by the International Research Support Initiative Program (IRSIP) fellowship to Ph.D. scholar funded by Higher Education Commission (HEC), Pakistan IRSIP Fellowship No. (PIN) IRSIP 39, BMS 43, the National Research Program for Universities entitled “Improvement of heat tolerance in wheat under climate change scenario” Project No. 7202, and the Center for Desert Agriculture, King Abdullah University of Science and Technology (KAUST), Saudi Arabia, NIBGE-KAUST Grant No. ORS#2375.
Conflict of Interest
The authors declare that the research was conducted in the absence of any commercial or financial relationships that could be construed as a potential conflict of interest.
Publisher’s Note
All claims expressed in this article are solely those of the authors and do not necessarily represent those of their affiliated organizations, or those of the publisher, the editors and the reviewers. Any product that may be evaluated in this article, or claim that may be made by its manufacturer, is not guaranteed or endorsed by the publisher.
Acknowledgments
We would like to thank Teun Munnik, Research Cluster Green Life Sciences, Section Plant Cell Biology, Swammerdam Institute for Life Sciences, University of Amsterdam, Netherlands, for providing excellent learning and experimental environment, technical guidance, and support for this study.
Supplementary Material
The Supplementary Material for this article can be found online at: https://www.frontiersin.org/articles/10.3389/fpls.2022.881188/full#supplementary-material
Abbreviations
DAG, diacylglycerol; DGK, diacylglycerol kinase; IP3, inositol 1,4,5 trisphosphate; IPP, inositol polyphosphate; OE, overexpression; PA, phosphatidic acid; PIP, phosphatidylinositol monophosphate; PIP2, phosphatidylinositol 4,5-bisphosphate; PIPK, phosphatidylinositol phosphate kinase; PI-PLC, phosphoinositide specific phospholipase C; PLD, phospholipase D.
References
Abhinandan, K., Skori, L., Stanic, M., Hickerson, N. M. N., Jamshed, M., and Samuel, M. A. (2018). Abiotic stress signaling in wheat – an inclusive overview of hormonal interactions during abiotic stress responses in wheat. Front. Plant Sci. 9:734. doi: 10.3389/fpls.2018.00734
Alcázar-Román, A. R., and Wente, S. R. (2008). Inositol polyphosphates: a new frontier for regulating gene expression. Chromosoma 117, 1–13. doi: 10.1007/s00412-007-0126-4
Apone, F., Alyeshmerni, N., Wiens, K., Chalmers, D., Chrispeels, M. J., and Colucci, G. (2003). The G-protein-coupled receptor GCR1 regulates DNA synthesis through activation of phosphatidylinositol-specific phospholipase C. Plant Physiol. 133, 571–579. doi: 10.1104/pp.103.026005
Apostolakos, P., Panteris, E., and Galatis, B. (2008). The involvement of phospholipases C and D in the asymmetric division of subsidiary cell mother cells of Zea mays. Cell Motil. Cytoskeleton 65, 863–875. doi: 10.1002/cm.20308
Arisz, S. A., van Wijk, R., Roels, W., Zhu, J. K., Haring, M. A., and Munnik, T. (2013). Rapid phosphatidic acid accumulation in response to low temperature stress in Arabidopsis is generated through diacylglycerol kinase. Front. Plant Sci. 4:1. doi: 10.3389/fpls.2013.00001
Balogh, G., Péter, M., Glatz, A., Gombos, I., Török, Z., Horváth, I., et al. (2013). Key role of lipids in heat stress management. FEBS Lett. 587, 1970–1980. doi: 10.1016/j.febslet.2013.05.016
Darwish, E., Testerink, C., Khalil, M., El-Shihy, O., and Munnik, T. (2009). Phospholipid signaling responses in salt-stressed rice leaves. Plant Cell Physiol. 50, 986–997. doi: 10.1093/pcp/pcp051
Das, S., Hussain, A., Bock, C., Keller, W. A., and Georges, F. (2005). Cloning of Brassica napus phospholipase C2 (BnPLC2), phosphatidylinositol 3-kinase (BnVPS34) and phosphatidylinositol synthase1 (BnPtdIns S1)–comparative analysis of the effect of abiotic stresses on the expression of phosphatidylinositol signal transduction-related genes in B. napus. Planta 220, 777–784. doi: 10.1007/s00425-004-1389-0
Djanaguiraman, M., Narayanan, S., Erdayani, E., and Prasad, P. V. V. (2020). Effects of high temperature stress during anthesis and grain filling periods on photosynthesis, lipids and grain yield in wheat. BMC Plant Biol. 20:268. doi: 10.1186/s12870-020-02479-0
Dowd, P. E., Coursol, S., Skirpan, A. L., Kao, T.-h., and Gilroy, S. (2006). Petunia phospholipase c1 is involved in pollen tube growth. Plant cell 18, 1438–1453. doi: 10.1105/tpc.106.041582
Dubots, E., Botté, C., Boudière, L., Yamaryo-Botté, Y., Jouhet, J., Maréchal, E., et al. (2012). Role of phosphatidic acid in plant galactolipid synthesis. Biochimie 94, 86–93. doi: 10.1016/j.biochi.2011.03.012
Enrique Gomez, R., Joubès, J., Valentin, N., Batoko, H., Satiat-Jeunemaître, B., and Bernard, A. (2017). Lipids in membrane dynamics during autophagy in plants. J. Exp. Bot. 69, 1287–1299. doi: 10.1093/jxb/erx392
Foulkes, M. J., Scott, R. K., and Sylvester-Bradley, R. (2002). The ability of wheat cultivars to withstand drought in UK conditions: formation of grain yield. J. Agric. Sci. 138, 153–169. doi: 10.1017/s0021859601001836
Gao, F., Han, X., Wu, J., Zheng, S., Shang, Z., Sun, D., et al. (2012). A heat-activated calcium-permeable channel–Arabidopsis cyclic nucleotide-gated ion channel 6–is involved in heat shock responses. Plant J. 70, 1056–1069. doi: 10.1111/j.1365-313X.2012.04969.x
Gao, K., Liu, Y. L., Li, B., Zhou, R. G., Sun, D. Y., and Zheng, S. Z. (2014). Arabidopsis thaliana phosphoinositide-specific phospholipase C isoform 3 (AtPLC3) and AtPLC9 have an additive effect on thermotolerance. Plant Cell Physiol. 55, 1873–1883. doi: 10.1093/pcp/pcu116
Gilroy, S., and Trewavas, A. (2001). Signal processing and transduction in plant cells: the end of the beginning? Nat. Rev. Mol. Cell Biol. 2, 307–314. doi: 10.1038/35067109
Hagio, M., Sakurai, I., Sato, S., Kato, T., Tabata, S., and Wada, H. (2002). Phosphatidylglycerol is essential for the development of thylakoid membranes in Arabidopsis thaliana. Plant Cell Physiol. 43, 1456–1464. doi: 10.1093/pcp/pcf185
Haselier, A., Akbari, H., Weth, A., Baumgartner, W., and Frentzen, M. (2010). Two closely related genes of Arabidopsis encode plastidial cytidinediphosphate diacylglycerol synthases essential for photoautotrophic growth. Plant Physiol. 153, 1372–1384. doi: 10.1104/pp.110.156422
Hayes, S., Schachtschabel, J., Mishkind, M., Munnik, T., and Arisz, S. A. (2021). Hot topic: thermosensing in plants. Plant Cell Environ. 44, 2018–2033. doi: 10.1111/pce.13979
Helling, D., Possart, A., Cottier, S. p., Klahre, U., and Kost, B. (2006). Pollen tube tip growth depends on plasma membrane polarization mediated by tobacco PLC3 activity and endocytic membrane recycling. Plant Cell 18, 3519–3534. doi: 10.1105/tpc.106.047373
Hong, Y., Zhao, J., Guo, L., Kim, S. C., Deng, X., Wang, G., et al. (2016). Plant phospholipases D and C and their diverse functions in stress responses. Prog. Lipid Res. 62, 55–74. doi: 10.1016/j.plipres.2016.01.002
Hou, Q., Ufer, G., and Bartels, D. (2016). Lipid signalling in plant responses to abiotic stress. Plant Cell Environ. 39, 1029–1048. doi: 10.1111/pce.12666
Hunt, L., Otterhag, L., Lee, J. C., Lasheen, T., Hunt, J., Seki, M., et al. (2004). Gene-specific expression and calcium activation of Arabidopsis thaliana phospholipase C isoforms. New Phytol. 162, 643–654. doi: 10.1111/j.1469-8137.2004.01069.x
Ishida, Y., Tsunashima, M., Hiei, Y., and Komari, T. (2015). “Wheat (Triticum aestivum L.) transformation using immature embryos,” in Agrobacterium Protocols, ed. K. Wang (New York, NY: Springer), 189–198. doi: 10.1007/978-1-4939-1695-5_15
Khalil, H. B., Wang, Z., Wright, J. A., Ralevski, A., Donayo, A. O., and Gulick, P. J. (2011). Heterotrimeric Galpha subunit from wheat (Triticum aestivum), GA3, interacts with the calcium-binding protein, Clo3, and the phosphoinositide-specific phospholipase C, PI-PLC1. Plant Mol. Biol. 77, 145–158. doi: 10.1007/s11103-011-9801-1
Kobayashi, K., Endo, K., and Wada, H. (2017). Specific distribution of phosphatidylglycerol to photosystem complexes in the thylakoid membrane. Front. Plant Sci. 8:1991. doi: 10.3389/fpls.2017.01991
Kobayashi, K., Fujii, S., Sato, M., Toyooka, K., and Wada, H. (2015). Specific role of phosphatidylglycerol and functional overlaps with other thylakoid lipids in Arabidopsis chloroplast biogenesis. Plant Cell Rep. 34, 631–642. doi: 10.1007/s00299-014-1719-z
Kosová, K., Vítámvás, P., Urban, M. O., Prášil, I. T., and Renaut, J. (2018). Plant abiotic stress proteomics: the major factors determining alterations in cellular proteome. Front. Plant Sci. 9:122. doi: 10.3389/fpls.2018.00122
Lee, Y., Kim, Y. W., Jeon, B. W., Park, K. Y., Suh, S. J., Seo, J., et al. (2007). Phosphatidylinositol 4,5-bisphosphate is important for stomatal opening. Plant J. 52, 803–816. doi: 10.1111/j.1365-313X.2007.03277.x
Mahajan, S., and Tuteja, N. (2005). Cold, salinity and drought stresses: an overview. Arch. Biochem. Biophys. 444, 139–158. doi: 10.1016/j.abb.2005.10.018
McCarty, D. R., and Chory, J. (2000). Conservation and innovation in plant signaling pathways. Cell 103, 201–209. doi: 10.1016/S0092-8674(00)00113-6
Melin, P. M., Pical, C., Jergil, B., and Sommarin, M. (1992). Polyphosphoinositide phospholipase C in wheat root plasma membranes. Partial purification and characterization. Biochim. Biophys. Acta 24, 163–169. doi: 10.1016/0005-2760(92)90107-7
Mishkind, M., Vermeer, J. E., Darwish, E., and Munnik, T. (2009). Heat stress activates phospholipase D and triggers PIP accumulation at the plasma membrane and nucleus. Plant J. 60, 10–21. doi: 10.1111/j.1365-313X.2009.03933.x
Munnik, T. (2014). “PI-PLC: phosphoinositide-phospholipase C in plant signaling,” in Phospholipases in Plant Signaling, Vol. 20, ed. X. Wang (Berlin: Springer), 27–54.
Munnik, T., and Zarza, X. (2013). Analyzing plant signaling phospholipids through 32Pi-labeling and TLC. Methods Mol. Biol. 1009, 3–15. doi: 10.1007/978-1-62703-401-2_1
Munnik, T., Irvine, R., and Musgrave, A. (1998). Phospholipid signalling in plants. Biochim. Biophys. Acta (BBA) Lipids Lipid Metab. 1389, 222–272.
Munnik, T., Mongrand, S., Zársky, V., and Blatt, M. (2021). Dynamic membranes—the indispensable platform for plant growth, signaling, and development. Plant Physiol. 185, 547–549. doi: 10.1093/plphys/kiaa107
Narayanan, S., Tamura, P. J., Roth, M. R., Prasad, P. V., and Welti, R. (2016). Wheat leaf lipids during heat stress: I. High day and night temperatures result in major lipid alterations. Plant Cell Environ. 39, 787–803. doi: 10.1111/pce.12649
Niu, Y., and Xiang, Y. (2018). An overview of biomembrane functions in plant responses to high-temperature stress. Front. Plant Sci. 9:915. doi: 10.3389/fpls.2018.00915
Nokhrina, K., Ray, H., Bock, C., and Georges, F. (2014). Metabolomic shifts in Brassica napus lines with enhanced BnPLC2 expression impact their response to low temperature stress and plant pathogens. GM Crops Food 5, 120–131. doi: 10.4161/gmcr.28942
Ruelland, E., Kravets, V., Derevyanchuk, M., Martinec, J., Zachowski, A., and Pokotylo, I. (2015). Role of phospholipid signalling in plant environmental responses. Environ. Exp. Bot. 114, 129–143.
Schmidt, J., Claussen, J., Wörlein, N., Eggert, A., Fleury, D., Garnett, T., et al. (2020). Drought and heat stress tolerance screening in wheat using computed tomography. Plant Methods 16:15. doi: 10.1186/s13007-020-00565-w
Shiva, S., Samarakoon, T., Lowe, K. A., Roach, C., Vu, H. S., Colter, M., et al. (2020). Leaf lipid alterations in response to heat stress of Arabidopsis thaliana. Plants 9:845. doi: 10.3390/plants9070845
Simon, M. L., Platre, M. P., Assil, S., van Wijk, R., Chen, W. Y., Chory, J., et al. (2014). A multi-colour/multi-affinity marker set to visualize phosphoinositide dynamics in Arabidopsis. Plant J. 77, 322–337. doi: 10.1111/tpj.12358
Singh, A., Kanwar, P., Pandey, A., Tyagi, A. K., Sopory, S. K., Kapoor, S., et al. (2013). Comprehensive genomic analysis and expression profiling of phospholipase C gene family during abiotic stresses and development in rice. PLoS One 8:e62494. doi: 10.1371/journal.pone.0062494
Tack, J., Barkley, A., and Nalley, L. L. (2015). Effect of warming temperatures on US wheat yields. Proc. Natl. Acad. Sci. U.S.A. 112, 6931–6936. doi: 10.1073/pnas.1415181112
Trewavas, A. J., and Malho, R. (1997). Signal perception and transduction: the origin of the phenotype. Plant Cell 9, 1181–1195. doi: 10.1105/tpc.9.7.1181
Tuteja, N. (2007). Mechanisms of high salinity tolerance in plants. Methods Enzymol. 428, 419–438. doi: 10.1016/S0076-6879(07)28024-3
Tuteja, N., and Sopory, S. K. (2008). Chemical signaling under abiotic stress environment in plants. Plant Signal. Behav. 3, 525–536. doi: 10.4161/psb.3.8.6186
Van Leeuwen, W., Vermeer, J. E., Gadella, T. W. Jr., and Munnik, T. (2007). Visualization of phosphatidylinositol 4, 5-bisphosphate in the plasma membrane of suspension-cultured tobacco BY-2 cells and whole Arabidopsis seedlings. Plant J. 52, 1014–1026. doi: 10.1111/j.1365-313X.2007.03292.x
van Schooten, B., Testerink, C., and Munnik, T. (2006). Signalling diacylglycerol pyrophosphate, a new phosphatidic acid metabolite. Biochim. Biophys. Acta 2, 151–159. doi: 10.1016/j.bbalip.2005.12.010
Vossen, J. H., Abd-El-Haliem, A., Fradin, E. F., van den Berg, G. C., Ekengren, S. K., Meijer, H. J., et al. (2010). Identification of tomato phosphatidylinositol-specific phospholipase-C (PI-PLC) family members and the role of PLC4 and PLC6 in HR and disease resistance. Plant J. 62, 224–239. doi: 10.1111/j.1365-313X.2010.04136.x
Wang, C. R., Yang, A. F., Yue, G. D., Gao, Q., Yin, H. Y., and Zhang, J. R. (2008). Enhanced expression of phospholipase C 1 (ZmPLC1) improves drought tolerance in transgenic maize. Planta 227, 1127–1140. doi: 10.1007/s00425-007-0686-9
Wang, F., Deng, Y., Zhou, Y., Dong, J., Chen, H., Dong, Y., et al. (2015). Genome-wide analysis and expression profiling of the phospholipase C gene family in soybean (Glycine max). PLoS One 10:e0138467. doi: 10.1371/journal.pone.0138467
Wang, X., Liu, Y., Li, Z., Gao, X., Dong, J., Zhang, J., et al. (2020). Genome-wide identification and expression profile analysis of the phospholipase C gene family in wheat (Triticum aestivum L.). Plants 9:885. doi: 10.3390/plants9070885
Wang, X., Yao, X., Zhao, A., Yang, M., Zhao, W., LeTourneau, M. K., et al. (2021). Phosphoinositide-specific phospholipase C gene involved in heat and drought tolerance in wheat (Triticum aestivum L.). Genes Genomics 43, 1167–1177. doi: 10.1007/s13258-021-01123-x
Wang, Y., Zhang, X., Huang, G., Feng, F., Liu, X., Guo, R., et al. (2020). Dynamic changes in membrane lipid composition of leaves of winter wheat seedlings in response to PEG-induced water stress. BMC Plant Biol. 20:84. doi: 10.1186/s12870-020-2257-1
Weldearegay, D. F., Yan, F., Jiang, D., and Liu, F. (2012). Independent and combined effects of soil warming and drought stress during anthesis on seed set and grain yield in two spring wheat varieties. J. Agron. Crop Sci. 198, 245–253. doi: 10.1111/j.1439-037X.2012.00507.x
Zhai, S., Gao, Q., Liu, X., Sui, Z., and Zhang, J. (2013). Overexpression of a Zea mays phospholipase C1 gene enhances drought tolerance in tobacco in part by maintaining stability in the membrane lipid composition. Plant Cell Tissue Organ Cult. (PCTOC) 115, 253–262.
Zhang, Q., van Wijk, R., Zarza, X., Shahbaz, M., van Hooren, M., Guardia, A., et al. (2018c). Knock-Down of Arabidopsis PLC5 reduces primary root growth and secondary root formation while overexpression improves drought tolerance and causes stunted root hair growth. Plant Cell Physiol. 59, 2004–2019. doi: 10.1093/pcp/pcy120
Zhang, B., Wang, Y., and Liu, J. Y. (2018a). Genome-wide identification and characterization of phospholipase C gene family in cotton (Gossypium spp.). Sci. China Life Sci. 61, 88–99. doi: 10.1007/s11427-017-9053-y
Zhang, K., Jin, C., Wu, L., Hou, M., Dou, S., and Pan, Y. (2014). Expression analysis of a stress-related phosphoinositide-specific phospholipase C gene in wheat (Triticum aestivum L.). PLoS One 9:e105061. doi: 10.1371/journal.pone.0105061
Zhang, Q., van Wijk, R., Shahbaz, M., Roels, W., Schooten, B. V., Vermeer, J. E. M., et al. (2018b). Arabidopsis phospholipase C3 is involved in lateral root initiation and ABA responses in seed germination and stomatal closure. Plant Cell Physiol. 59, 469–486. doi: 10.1093/pcp/pcx194
Keywords: heat stress, osmotic stress, PA, PIP2, 32Pi, wheat
Citation: Annum N, Ahmed M, Imtiaz K, Mansoor S, Tester M and Saeed NA (2022) 32Pi Labeled Transgenic Wheat Shows the Accumulation of Phosphatidylinositol 4,5-bisphosphate and Phosphatidic Acid Under Heat and Osmotic Stress. Front. Plant Sci. 13:881188. doi: 10.3389/fpls.2022.881188
Received: 22 February 2022; Accepted: 16 May 2022;
Published: 14 June 2022.
Edited by:
Nabin Bhusal, Agriculture and Forestry University, NepalReviewed by:
Girish Mishra, University of Delhi, IndiaJasdeep Chatrath Padaria, Indian Council of Agricultural Research, India
Copyright © 2022 Annum, Ahmed, Imtiaz, Mansoor, Tester and Saeed. This is an open-access article distributed under the terms of the Creative Commons Attribution License (CC BY). The use, distribution or reproduction in other forums is permitted, provided the original author(s) and the copyright owner(s) are credited and that the original publication in this journal is cited, in accordance with accepted academic practice. No use, distribution or reproduction is permitted which does not comply with these terms.
*Correspondence: Moddassir Ahmed, Y21tYW5pYmdlQHlhaG9vLmNvLnVr