- 1Department of Chemistry, Seoul National University, Seoul, South Korea
- 2Research Institute of Basic Sciences, Seoul National University, Seoul, South Korea
- 3Plant Genomics and Breeding Institute, Seoul National University, Seoul, South Korea
Wounding not only induces the expression of damage-responsive genes, but also initiates physiological changes, such as tissue repair, vascular reconnection, and de novo organogenesis in locally damaged tissues. Wound-induced signals also propagate from the site of wounding to distal organs to elicit a systemic response. Electrical signaling, which is the most conserved type of systemic signaling in eukaryotes, is triggered by wound-induced membrane potential changes. Changes in membrane potential spread toward systemic tissues in synergy with chemical and hydraulic signals. Here, we review current knowledge on wound-induced local and systemic responses in plants. We focus particularly on how wound-activated plasma membrane-localized ion channels and pumps propagate systemic information about wounding to induce downstream molecular responses in distal tissues. Finally, we propose future studies that could lead to a better understanding of plant electrical signals and their role in physiological responses to wounding.
Introduction
Plants are sessile organisms that are constantly exposed to a variety of external stimuli. Wounding is one external stimulus that induces physical damages threating plant survival and also makes them more susceptible to pathogen invasion (Savatin et al., 2014). Wounding is caused by insect feeding, pathogen infection, animal grazing, wind, and heavy rain (Reymond et al., 2000; Sozen et al., 2020). To survive, plants have evolved sophisticated defense mechanisms, such as the activation of defense gene expression, tissue repair, and de novo tissue regeneration in regions of the tissue where wounding occurs (Reymond et al., 2000; Liu et al., 2014; Savatin et al., 2014; Matsuoka et al., 2021). Signals in response to wounding propagate from the site of wound perception to distal tissues. These signals typically travel long distances, and this process is referred to as a systemic response (Huber and Bauerle, 2016). Wounding triggers distal systemic responses in plants, such as accumulation of defensive proteinase inhibitors (PIs) and jasmonates (JAs) in systemic tissues (Koo et al., 2009; Kiep et al., 2015; Gilroy et al., 2016; Fichman and Mittler, 2020).
Long-distance signals transduce environmental information throughout the whole plant body, and three major types of long-distance signals have been proposed: (i) chemical; (ii) hydraulic; and (iii) electrical signals (Huber and Bauerle, 2016; Canales et al., 2017). In particular, plant electrical signaling is the most conserved long-distance signaling system, which travels to plant distal tissues through vascular tissues to systemically induce responses in a whole plant (Hedrich et al., 2016). It is capable of transmitting signals more quickly over long distances, compared with hormone signals (Choi et al., 2017; Moe-Lange et al., 2021). Electrical signals in plants can be divided into several sub-types: local electrical potential (LEP), action potential (AP), systemic potential (SP), and slow wave potential (SWP; also known as variation potential; Szechynska-Hebda et al., 2017; Mudrilov et al., 2021). LEP is a sub-threshold response induced by change in environmental conditions, such as humidity light and temperatures. While LEP is only locally generated and is not transferred to other parts of a plant, AP, SP, and SWP can transmit from the stimulated site to other parts of the plant. AP can be defined as a rapid and transient changes in cell membrane potential (depolarization and subsequent repolarization) in response to non-damaging stimuli. AP induces an all-or-nothing response, which occurs only above a threshold of stimuli. In contrast, SWP defined as a transient membrane depolarization with an irregular shape and a longer and delayed repolarization phase is a hydraulically propagating electrical signal exclusively found in plant cells. SWP is usually induced by severe tissue damage. SP is a most recently proposed type that has variable intensities according to stimuli. SP displays a transient hyperpolarization that is usually induced by herbivore feeding or adding cations to a damaged tissues (Zimmermann et al., 2009, 2016; Szechynska-Hebda et al., 2017). To date, SWP has been extensively investigated in plants. SWP is characterized by a decrease in magnitude as it spreads away from the stimulated site, and magnitude and shape of SWP vary with the intensity of the environmental stimulus (Huber and Bauerle, 2016). Notably, wound-induced changes in membrane potential possibly generate a unique profile of electrical signals (Mousavi et al., 2014; Farmer et al., 2020), which is possibly shaped by wound-activated ion channels and pumps (Gadsby, 2009; Kumari et al., 2019; Farmer et al., 2020).
Here, we summarize current knowledge about local and systemic wound responses in plants. We also discuss how wound-activated ion channels and pumps generate and propagate electrical signals to induce downstream wound responses. Finally, we propose future studies that could help to identify the novel roles of electrical signaling upon wounding.
Responses to Wounding
To mitigate the plant damages from wounding, plants not only trigger tissue healing and regeneration, but also activate multiple defense responses (Leon et al., 2001; Cheong et al., 2002). Signals that are generated by wounding are transmitted through long distances. The activation of defense genes in tissues located away from the site of wounding is a well-known systemic response (Huber and Bauerle, 2016). In this section, we summarize the cellular and molecular processes underlying local and systemic responses to wounding.
Local Responses to Wounding
The healing of injured tissue is a primary local response to wounding (Hoermayer and Friml, 2019; Marhava et al., 2019; Matsuoka et al., 2021). Upon wounding, plants rely on targeted cell division and expansion to restore their damaged tissues. For example, elimination of specific root cells by laser ablation promotes periclinal cell division of cells adjacent to the inner side of the injury site through the reactivation of stem cell transcriptional programs, ensuring the correct replacement of injured cells (Marhava et al., 2019). Moreover, excision of the root apical meristem triggers root tip regeneration via embryonic developmental process as well as hormone redistributions (Efroni et al., 2016). The root tip regeneration in Arabidopsis is known to rely on genetic program consisting of PLETHORA 2 (PLT2) and ETHYLENE RESPONSE FACTOR 115 (ERF115; Durgaprasad et al., 2019; Canher et al., 2020). The tissue repair is also pervasive in other tissues. Damage to vascular tissues leads to the formation of cambial cells from differentiated cells, which then re-differentiate into xylem and phloem vessels in an auxin-dependent manner (Sussex et al., 1972; Mazur et al., 2016; Matsuoka et al., 2021; Figure 1).
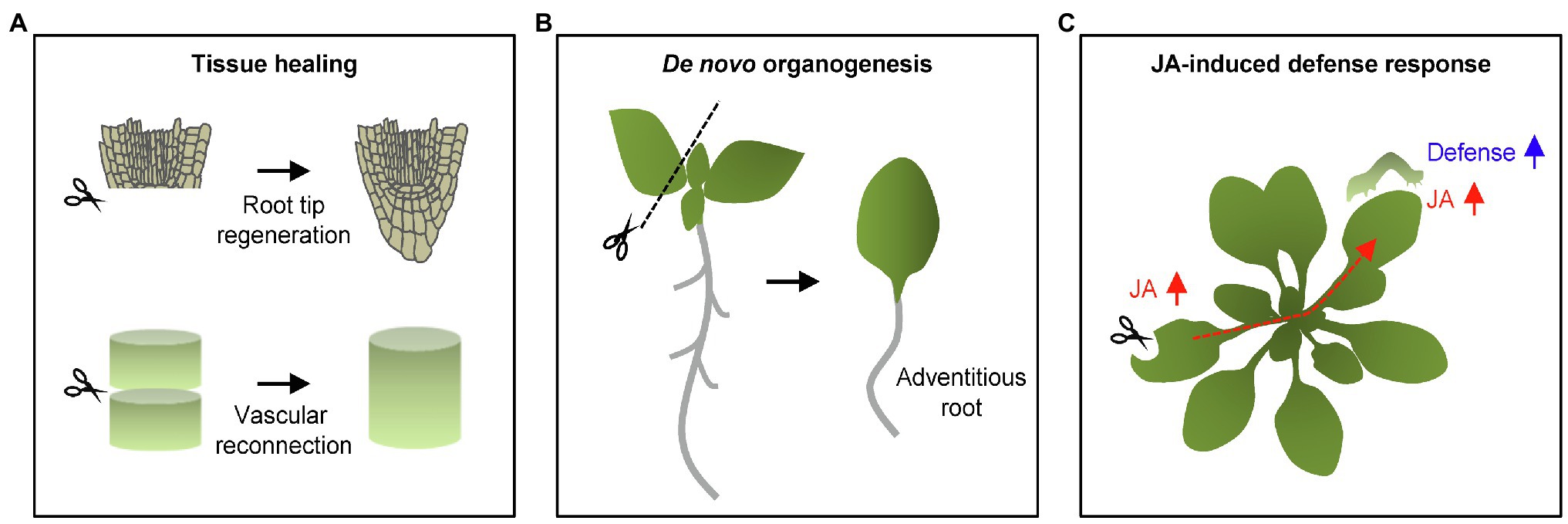
Figure 1. Wound-induced local and systemic responses in plants. (A) Wound healing and tissue regeneration. Upon wounding, plants stimulate wound healing and tissue regeneration. Excision of the root apical meristem and vascular tissues leads to the tissue restoration in local damaged regions. (B) Wound-induced adventitious root formation. Wounding occasionally results in cell fate changes as shown by the adventitious root formation from detached leaf explants. (C) Wound-induced defense responses. Wounding also increases defense capacity in both local and systemic tissues. Jasmonic acid (JA) is a pivotal phytohormone stimulating plant defense responses not only in damaged local tissues, but also distal plant organs. Long-distance signals propagate through vasculatures in order to confer systemic defense resistance in a whole plant.
Wounding triggers neighboring cells to re-enter the cell cycle, frequently inducing callus from differentiated somatic cells (Ikeuchi et al., 2017; Iwase et al., 2021). The AP2/ERF transcription factor gene, WOUND-INDUCED DEDIFFERENTIATION1 (WIND1), and its close homologs WIND2, WIND3, and WIND4 are rapidly induced by wounding, and they promote callus formation by activating cytokinin responses (Iwase et al., 2011). Callus has the capacity for cell fate transition, which is essential for regenerating new organs (Iwase et al., 2021). In parallel, wounding frequently activates de novo organogenesis, sometimes independently of callus formation. For instance, cutting leaf explants triggers de novo root organogenesis (Liu et al., 2014). Up-regulation of JA biosynthesis and signaling subsequently stimulates auxin biosynthesis that precedes cell fate transition into root founder cell (Zhang et al., 2019; Figure 1).
Consistent with the fact that JA is a major wound-responsive phytohormone (Koo et al., 2009), JA-induced defense responses are mainly activated in local damaged tissues. JA biosynthesis is rapidly initiated within 30 s by 13-lipoxygenases (13-LOXs) in local damaged leaves, which catalyze the incorporation of an oxygen molecule to chloroplastic tri-unsaturated fatty acids (Glauser et al., 2009; Ahmad et al., 2016; Li et al., 2016; Figure 1). Following wound-induced increases in JA levels, the F-box protein, CORONATINE INSENSITIVE 1 (COI1), physically interacts with JASMONATE-ZIM DOMAIN (JAZ) proteins, which are repressors of JA signaling (Gfeller et al., 2010). JA-induced COI1–JAZ interaction triggers JAZ degradation through the ubiquitin-dependent pathway, de-repressing MYC2 transcription factor activity (Guo et al., 2018). MYC2 activates defense genes and therefore increases the capacity of plants to defend themselves against wounding as well as herbivory (Chauvin et al., 2013; Major et al., 2017).
Systemic Responses to Wounding
Upon injury, wound-responsive genes are also induced in undamaged systemic tissues to protect plants against potential future damages. Accumulation of JA is essential to elicit defensive traits in systemic tissues. JA accumulation in systemic tissues significantly increases 120 s after wounding (Glauser et al., 2009). Among the four 13-LOXs, LOX6 contributes the most to JA biosynthesis in Arabidopsis systemic leaves (Chauvin et al., 2013). JA-inducible PIs protect plant systemic tissues from herbivores by reducing the activity of digestive enzymes in insect guts (Howe, 2004). In tomato, expression of PI genes is induced in systemic tissues within 2 h after wounding, and their induction is impaired in JA signaling mutants (Li et al., 2002). Application of methyl JA to one leaf induces PI gene expression in both local and systemic leaves even in the absence of wounding (Farmer and Ryan, 1990; Devoto and Turner, 2003).
Extracellular signals such as cell wall-derived oligogalacturonides (OGs) and peptides have been characterized as typical systemic signals of wounding, which are known as Damage-Associated Molecular Patterns (DAMPs). For instance, systemin, a 18-amino acid peptide, was identified in tomato after wounding or insect attack as a cleavage product released into the apoplast from the 200-amino acid precursor prosystemin protein (Sun et al., 2011). Systemin triggers systemic responses by acting upstream of JA (Sun et al., 2011). Ectopic expression of prosystemin triggers the constitutive expression of PI genes in the absence of wounding, and the increased PI levels are reduced in JA-deficient mutants (Sun et al., 2011). In addition to PIs, expression of PATHOGENESIS-RELATED (PR) genes, encoding PR-1, PR-10, chitinase, thaumatin-like protein, and plant defensin, is induced in systemic tissues to enhance plant survival and resistance against further herbivore attack (Kang et al., 2021).
Long-Distance Signals in Response to Wounding
As noted, wound-induced defense responses propagate from injured local tissues to systemic tissues through long-distance signals. Long-distance signals are divided into three types: chemical, hydraulic, and electrical (Huber and Bauerle, 2016; Moe-Lange et al., 2021). Chemical signaling usually employs reactive oxygen species (ROS) and calcium ion (Ca2+; Moe-Lange et al., 2021). Wounding rapidly triggers the production of ROS in local and systemic tissues that is dependent on the NADPH oxidase, RESPIRATORY BURST OXIDASE HOMOLOG D (RBOHD; Miller et al., 2009; Prasad et al., 2019). Transgenic plants expressing luciferase driven by a ROS-responsive ZAT12 gene promoter show that ROS signaling propagates into systemic tissues at a rate of ~8 cm/min, and this rate is substantially delayed in pZAT12::Luc/rbohD plants (Miller et al., 2009). An increase in cytosolic Ca2+ is another hallmark of long-distance signaling (Shao et al., 2020). Wounding elevates cytosolic Ca2+ within seconds at the injured site, which generates a Ca2+ wave that travels into systemic tissues through vasculature tissues at rates of ~6 cm/min (Tian et al., 2020). The Glu-activated GLUTAMATE RECEPTOR-LIKE (GLR) ion channel family expressed in phloem and xylem is responsible for the increases in intracellular Ca2+ concentration in local and systemic tissues (Gilroy et al., 2016; Toyota et al., 2018). Notably, accumulation of Ca2+ is required for systemic JA induction, and consistently, blocking Ca2+ entry inhibits JA-induced defense responses (Tian et al., 2020). Moreover, hydraulic signaling is a class of long-distance signaling that is closely associated with initial Ca2+ entry into cells (Gilroy et al., 2016). Wounding causes rapid axial changes of hydrostatic pressure in the xylem and then, converts it to slower, radially dispersed changes of pressure associated with activation of a clade 3 GLR-dependent signaling pathway that prepares distal leaves for imminent attack (Farmer et al., 2014).
Electrical signaling is another crucial type of long-distance signaling generated by a rapid collapse of membrane potential in response to wounding (Farmer et al., 2020). Among several sub-types of electrical signals, SWP is particularly triggered by local wounding and transmitted to undamaged tissues at a speed of ~7 cm/min through vascular connections in plants (Mousavi et al., 2013; Szechynska-Hebda et al., 2017; Nguyen et al., 2018; Kurenda et al., 2019). Although waves of SWP have variable dynamics, it is generally characterized by a rapid (< 2 s) and massive (>50 mV) depolarization phase, and a slow (> 5 min) repolarization phase after wounding in Arabidopsis (Nguyen et al., 2018; Kumari et al., 2019). Notably, SWP has a diverse range of intensities, propagation speed and amplitude depending on the severity of the injury and distance from the injured site (Huber and Bauerle, 2016). The variable SWP dynamics are likely decoded into appropriate defense responses in plants.
Although the long distance signals are closely interconnected (Farmer et al., 2020), SWPs act as key early responses that initiate wound-induced systemic responses, which precede increases in cytosolic Ca2+ accumulation and JA biosynthesis in distal tissues (Vega-Munoz et al., 2020; Moe-Lange et al., 2021). Several plasma membrane-localized ion channels and pumps are characterized as core regulators in generating and propagating SWPs in response to wounding (Mousavi et al., 2013; Kumari et al., 2019; Moe-Lange et al., 2021).
Glutamate Receptor-Like Channels
The GLR genes encode putative glutamate receptors and Ca2+ permeable channels (Nguyen et al., 2018). The Arabidopsis genome contains 20 GLR genes that are divided into three clades (Ni et al., 2016). Among others, Clade 3 GLR members have key roles in the generation and propagation of SWP (Mousavi et al., 2013; Vega-Munoz et al., 2020). Upon wounding, the concentration of apoplastic Glu increases in local damaged tissues. The Glu excretion subsequently activates GLRs to induce Ca2+ influx, which is potentially linked to membrane depolarization (Mousavi et al., 2013; Toyota et al., 2018). In support, mutating clade 3 GLR genes, such as GLR3.1, GLR3.2, GLR3.3, or GLR3.6, reduces Ca2+ influx and also the duration of SWPs in local wounded leaves (Mousavi et al., 2013). Propagation of SWP is particularly dependent on two GLR members, GLR3.3 and GLR3.6, which are expressed in the phloem and xylem contact cells, respectively, as SWP propagation in distal leaves is abolished in glr3.3 glr3.6 double mutants (Mousavi et al., 2013; Salvador-Recatala, 2016; Toyota et al., 2018; Li et al., 2021; Moe-Lange et al., 2021). Similarly, OsGLR3.4, which is a clade 3 GLR member in rice, also functions in wound-induced SWP propagation (Yu et al., 2022). Induction of SWPs in systemic leaves by mechanical stress in roots is significantly reduced in osglr3.4 mutants, indicating the functional conservation of GLRs across diverse plant species (Yu et al., 2022). Given that the GLR ion channels stimulate an increase in intracellular Ca2+ concentration that propagates to distant organs, the intimate connection between electrical and Ca2+ signals synergistically leads to efficient activation of systemic responses (Figure 2).
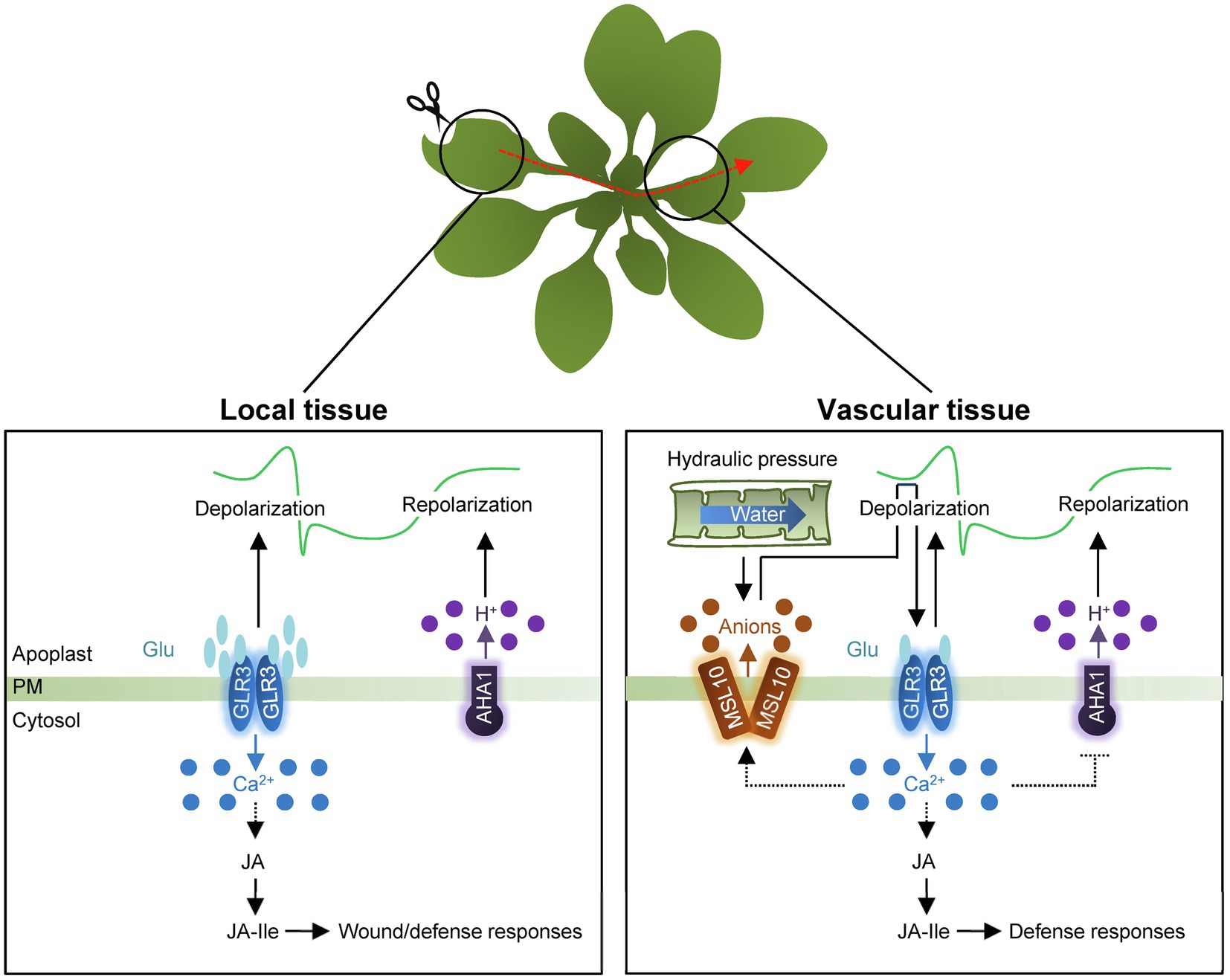
Figure 2. Schematic representation of electrical signaling in local and systemic tissues. In local tissue, wounding triggers an increase in apoplastic Glu concentration and then, induces GLR-dependent Ca2+ influx as well as depolarization of membrane potential. The GLR proteins likely act as tetramers. Following depolarization, H+-ATPase AHA1 is then required for membrane repolarization. The electrical signals systemically propagate through vasculature tissues in a connection with Ca2+ wave. Changes in turgor pressure affect membrane tension and activate the heptameric anion channel, MSL10, causing membrane depolarization through anion efflux. The membrane depolarization fully activates GLRs in response to wounding. GLR-dependent Ca2+ influx potentially causes activation of MSL10, simultaneously with the deactivation of AHA1. Synergistic propagation of Ca2+ waves and SWPs promotes JA biosynthesis to induce defense responses in systemic tissues. PM, plasma membrane.
Notably, different GLR members further fine-tune distribution of systemic electrical signals. GLR3.5 prevents the propagation of wound-induced electrical potentials to distal non-neighbor leaves. The glr3.5 mutant propagates systemic electrical signal in non-neighbor leaves where wound-induced electrical signal is not transmitted in wild type (Salvador-Recatala, 2016). Collectively, electrical signaling is propagated throughout the plant, and distribution of the systemic information is elaborately regulated by GLR proteins.
Phosphorylation (P)-Type Proton ATPase 1
P-type ATPases facilitate movement of protons, ions, and molecules across membranes, and determine membrane potential in animal kingdom (Kumari et al., 2019). Notably, plasma membrane-localized H+-ATPases within the P-type superfamily act as ion pumps and are involved in SWP propagation in plants (Falhof et al., 2016), and treatment with fusicoccin (FC), which activates plasma membrane H+ -ATPases, attenuates SWP duration (Kumari et al., 2019).
The Arabidopsis genome contains 11 members encoding H+-ATPases (AHA; Robertson et al., 2004; Haruta et al., 2010; Lin et al., 2021). AHA1 primarily functions in regulating SWPs in response to wounding (Kumari et al., 2019; Shao et al., 2020). In contrast to GLRs, AHA1 negatively controls SWP duration (Kumari et al., 2019; Figure 2). aha1 mutants extend the duration of the repolarization phase of SWPs in both local and systemic tissues, while the AHA1 gain-of-function mutant, ost2-2D, reduces SWP repolarization duration in systemic leaves (Kumari et al., 2019). Consistent with changes in the duration of SWPs, prolonged Ca2+ transients and JA biosynthesis increase in systemic tissues of aha1 mutants (Kumari et al., 2019).
It should be noted that, GLRs and AHA1 may function in common pathways for SWP generation, as revealed by double mutant studies (Kumari et al., 2019). The duration of the repolarization phase of aha1 mutants was lower than that of wild type in both local and systemic tissues when crossed with glr3.3 mutants (Kumari et al., 2019). In addition, co-treatment with Glu and the H+-ATPase activating compound FC abolishes the amplitude and duration of SWP depolarization, demonstrating that constitutive activation of H+-ATPase blocks Glu-induced depolarization (Shao et al., 2020).
Mechanosensitive Ion Channel-Like 10 (MSL10)
Mechanosensitive ion channels (MscS) respond to physical forces or plasma membrane deformation and generate mechanosensitive ion flux (Basu and Haswell, 2017; Canales Coutino and Mayor, 2021). MscS-Like (MSL) channels are the best-characterized MscS in diverse organisms (Basu and Haswell, 2017). Among 10 MSL proteins in Arabidopsis (Haswell et al., 2008), MSL10 is known to be directly gated by lateral membrane tension (Basu and Haswell, 2020) and has preference for anions with the conductance of about 100 pS (Basu and Haswell, 2020). Notably, the vasculature-expressed MSL10 protein functions in systemic SWP propagation upon wounding in Arabidopsis (Moe-Lange et al., 2021). The mutation of MSL10 significantly reduces the duration of SWP in systemic leaves, which also leads to reduced Ca2+ waves and JASMONATE-ZIM-DOMAIN PROTEIN 10 expression in distal tissues of msl10 mutants (Moe-Lange et al., 2021), indicating that the mechanosensitive ion channel MSL10 is required for wound-elicited electrical signals and systemic Ca2+ wave in distal tissues (Figure 2).
The msl10 mutants display ~fourfold shortened systemic SWPs and reduced Ca2+ wave in distal tissues, similar to the effects observed in the glr3.1, glr3.3, and glr3.6 single mutants (Moe-Lange et al., 2021). Considering that not only the spatial expression of GLR3.1, GLR3.3, and GLR3.6 overlaps with that of MSL10 in vasculature tissues, but also reduced SWP magnitudes of msl10;glr3.3 and msl10;glr3.6 double mutants are indistinguishable from those of their single mutants, MSL10 and GLRs function in overlapping genetic pathways (Moe-Lange et al., 2021). Taken together, wounding triggers the release of glutamate into the apoplasm, in turn activating Ca2+-permeable GLR3s that also influence electrical propagation. In parallel, changes in turgor pressure affect membrane tension and activate MSL10, causing anion efflux and depolarization of membrane potential. Membrane depolarization promotes full activity of the GLRs in distal tissues in response to wounding. Synergistic propagation of Ca2+ waves and SWPs efficiently induces systemic wound responses for plant survival (Fromm and Lautner, 2007; Feng et al., 2021).
Conclusion and Prospects
Plants have evolved long-distance communication system that systemically coordinates plant growth and development, especially enabling them to cope with adverse environments. Electrochemical signals are likely to mediate intercellular and intracellular communication in association with environmental changes. In this context, this study focused particularly on wound-responsive electrical signaling. Despite advances in understanding on the role of electrical signaling in systemic wound responses, several key questions still remain to be resolved. First, it is currently elusive how different environmental stimuli induce appropriate electrical signals with unique phase signatures. Functional and mechanical studies on ion channels and pumps that shape dynamics of SWPs would be required. Second, given that Glu-mediated activation of GLR ion channels plays a central role in the generation and systemic propagation of SWPs after wounding, it should be addressed whether subcellular localization of GLRs is related to the systemic signal propagation. Several GLR proteins are predicted to be targeted to the endoplasmic reticulum and play a potential function in secretory pathway (Davenport, 2002; Bassham et al., 2008; Nguyen et al., 2018), as shown by the rice GLR3.1 protein (Li et al., 2006). Localization-dependent GLR functions should be investigated to understand a possible linkage of intracellular and intercellular communications. Third, although wounding increase apoplastic Glu in local injured tissue, it should be answered how it is conveyed to the distal parts of the plant (Qiu et al., 2019). Additionally, a range of cellular Glu concentrations that trigger systemic calcium and electrical waves via GLR proteins should also be elucidated.
The SWP contains information about the severity of wounding and the distance from injured sites. Based on the information, plants induce appropriate wound responses by regulating intracellular Ca2+ concentration and endogenous JA levels in local and systemic tissues. Since current studies on wound-responsive electrical signals focus mainly on systemic aspects of defense responses and there is limited understanding on potential impact of SWPs in tissue healing and regeneration and pathogen resistance in local tissues (Kral et al., 2016; Hernandez-Coronado et al., 2022), it would be necessary to unravel the relevance of electrical signals in wound responses in local tissues in the near future.
The electrical signal intricately interacts with other systemic signals. Hydraulic signals contribute to propagation of SWP (Stahlberg and Cosgrove, 1996; Farmer et al., 2020). Altered hydrostatic pressure by wounding activates mechanosensitive elements that in turn affect membrane depolarization in systemic tissues (Moe-Lange et al., 2021). Similarly, ROS-dependent long-distance signaling also participates in SWP propagation (Farmer et al., 2020). Treatment with the NAD(P)H oxidase inhibitor, diphenyleneiodonium, reduces SWP duration in systemic tissues (Mousavi et al., 2013), indicating that ROS signaling potentially regulates SWP. Moreover, Ca2+ and SWP waves are also interdependent on each other. Despite the extensive connections among systemic signals, the molecular mechanisms underlying these interactions have been limitedly unveiled. It will be needed to identify molecular factors that are responsible for the crosstalk of systemic signals and ultimately comprehensive mechanisms that coordinate systemic signals to further understand efficiency and robustness of long-distance wound responses.
Author Contributions
All authors listed have made a substantial, direct, and intellectual contribution to the work, and approved it for publication.
Funding
This work was supported by the Basic Science Research (NRF-2021R1I1A1A01054378 to KL; NRF-2022R1A2B5B02001266 to PJS) and Basic Research Laboratory (NRF-2020R1A4A2002901) programs funded by the National Research Foundation of Korea and by the Creative-Pioneering Researchers Program through Seoul National University (0409–20210288).
Conflict of Interest
The authors declare that the research was conducted in the absence of any commercial or financial relationships that could be construed as a potential conflict of interest.
Publisher’s Note
All claims expressed in this article are solely those of the authors and do not necessarily represent those of their affiliated organizations, or those of the publisher, the editors and the reviewers. Any product that may be evaluated in this article, or claim that may be made by its manufacturer, is not guaranteed or endorsed by the publisher.
References
Ahmad, P., Rasool, S., Gul, A., Sheikh, S. A., Akram, N. A., Ashraf, M., et al. (2016). Jasmonates: multifunctional roles in stress tolerance. Front. Plant Sci. 7:813. doi: 10.3389/fpls.2016.00813
Bassham, D. C., Brandizzi, F., Otegui, M. S., and Sanderfoot, A. A. (2008). The secretory system of Arabidopsis. Arabidopsis Book 6:e0116. doi: 10.1199/tab.0116
Basu, D., and Haswell, E. S. (2017). Plant mechanosensitive ion channels: an ocean of possibilities. Curr. Opin. Plant Biol. 40, 43–48. doi: 10.1016/j.pbi.2017.07.002
Basu, D., and Haswell, E. S. (2020). The mechanosensitive ion channel MSL10 potentiates responses to cell swelling in Arabidopsis seedlings. Curr. Biol. 30:e2716. doi: 10.1016/j.cub.2020.05.015
Canales Coutino, B., and Mayor, R. (2021). Mechanosensitive ion channels in cell migration. Cells Dev. 166:203683. doi: 10.1016/j.cdev.2021.203683
Canales, J., Henriquez-Valencia, C., and Brauchi, S. (2017). The integration of electrical signals originating in the root of vascular plants. Front. Plant Sci. 8:2173. doi: 10.3389/fpls.2017.02173
Canher, B., Heyman, J., Savina, M., Devendran, A., Eekhout, T., Vercauteren, I., et al. (2020). Rocks in the auxin stream: wound-induced auxin accumulation and ERF115 expression synergistically drive stem cell regeneration. Proc. Natl. Acad. Sci. U. S. A. 117, 16667–16677. doi: 10.1073/pnas.2006620117
Chauvin, A., Caldelari, D., Wolfender, J. L., and Farmer, E. E. (2013). Four 13-lipoxygenases contribute to rapid jasmonate synthesis in wounded Arabidopsis thaliana leaves: a role for lipoxygenase 6 in responses to long-distance wound signals. New Phytol. 197, 566–575. doi: 10.1111/nph.12029
Cheong, Y. H., Chang, H. S., Gupta, R., Wang, X., Zhu, T., and Luan, S. (2002). Transcriptional profiling reveals novel interactions between wounding, pathogen, abiotic stress, and hormonal responses in Arabidopsis. Plant Physiol. 129, 661–677. doi: 10.1104/pp.002857
Choi, W. G., Miller, G., Wallace, I., Harper, J., Mittler, R., and Gilroy, S. (2017). Orchestrating rapid long-distance signaling in plants with Ca2+, ROS and electrical signals. Plant J. 90, 698–707. doi: 10.1111/tpj.13492
Devoto, A., and Turner, J. G. (2003). Regulation of jasmonate-mediated plant responses in Arabidopsis. Ann. Bot. 92, 329–337. doi: 10.1093/aob/mcg151
Durgaprasad, K., Roy, M. V., Venugopal, M. A., Kareem, A., Raj, K., Willemsen, V., et al. (2019). Gradient expression of transcription factor imposes a boundary on organ regeneration potential in plants. Cell Rep. 29:e453. doi: 10.1016/j.celrep.2019.08.099
Efroni, I., Mello, A., Nawy, T., Ip, P. L., Rahni, R., Delrose, N., et al. (2016). Root regeneration triggers an embryo-like sequence guided by hormonal interactions. Cell 165, 1721–1733.
Falhof, J., Pedersen, J. T., Fuglsang, A. T., and Palmgren, M. (2016). Plasma membrane H(+)-ATPase regulation in the center of plant physiology. Mol. Plant 9, 323–337. doi: 10.1016/j.molp.2015.11.002
Farmer, E. E., Gao, Y. Q., Lenzoni, G., Wolfender, J. L., and Wu, Q. (2020). Wound- and mechanostimulated electrical signals control hormone responses. New Phytol. 227, 1037–1050. doi: 10.1111/nph.16646
Farmer, E. E., Gasperini, D., and Acosta, I. F. (2014). The squeeze cell hypothesis for the activation of jasmonate synthesis in response to wounding. New Phytol. 204, 282–288. doi: 10.1111/nph.12897
Farmer, E. E., and Ryan, C. A. (1990). Interplant communication: airborne methyl jasmonate induces synthesis of proteinase inhibitors in plant leaves. Proc. Natl. Acad. Sci. U. S. A. 87, 7713–7716. doi: 10.1073/pnas.87.19.7713
Feng, S., Pan, C., Ding, S., Ma, Q., Hu, C., Wang, P., et al. (2021). The glutamate receptor plays a role in defense against Botrytis cinerea through electrical signaling in tomato. Appl. Sci. 11:11217. doi: 10.3390/app112311217
Fichman, Y., and Mittler, R. (2020). Rapid systemic signaling during abiotic and biotic stresses: is the ROS wave master of all trades? Plant J. 102, 887–896. doi: 10.1111/tpj.14685
Fromm, J., and Lautner, S. (2007). Electrical signals and their physiological significance in plants. Plant Cell Environ. 30, 249–257. doi: 10.1111/j.1365-3040.2006.01614.x
Gadsby, D. C. (2009). Ion channels versus ion pumps: the principal difference, in principle. Nat. Rev. Mol. Cell Biol. 10, 344–352. doi: 10.1038/nrm2668
Gfeller, A., Liechti, R., and Farmer, E. E. (2010). Arabidopsis jasmonate signaling pathway. Sci. Signal. 3:cm4. doi: 10.1126/scisignal.3109cm4
Gilroy, S., Bialasek, M., Suzuki, N., Gorecka, M., Devireddy, A. R., Karpinski, S., et al. (2016). ROS, calcium, and electric signals: key mediators of rapid systemic signaling in plants. Plant Physiol. 171, 1606–1615. doi: 10.1104/pp.16.00434
Glauser, G., Dubugnon, L., Mousavi, S. A., Rudaz, S., Wolfender, J. L., and Farmer, E. E. (2009). Velocity estimates for signal propagation leading to systemic jasmonic acid accumulation in wounded Arabidopsis. J. Biol. Chem. 284, 34506–34513. doi: 10.1074/jbc.M109.061432
Guo, Q., Yoshida, Y., Major, I. T., Wang, K., Sugimoto, K., Kapali, G., et al. (2018). JAZ repressors of metabolic defense promote growth and reproductive fitness in Arabidopsis. Proc. Natl. Acad. Sci. U. S. A. 115, E10768–E10777. doi: 10.1073/pnas.1811828115
Haruta, M., Burch, H. L., Nelson, R. B., Barrett-Wilt, G., Kline, K. G., Mohsin, S. B., et al. (2010). Molecular characterization of mutant Arabidopsis plants with reduced plasma membrane proton pump activity. J. Biol. Chem. 285, 17918–17929. doi: 10.1074/jbc.M110.101733
Haswell, E. S., Peyronnet, R., Barbier-Brygoo, H., Meyerowitz, E. M., and Frachisse, J. M. (2008). Two MscS homologs provide mechanosensitive channel activities in the Arabidopsis root. Curr. Biol. 18, 730–734. doi: 10.1016/j.cub.2008.04.039
Hedrich, R., Salvador-Recatala, V., and Dreyer, I. (2016). Electrical wiring and long-distance plant communication. Trends Plant Sci. 21, 376–387. doi: 10.1016/j.tplants.2016.01.016
Hernandez-Coronado, M., Dias Araujo, P. C., Ip, P. L., Nunes, C. O., Rahni, R., Wudick, M. M., et al. (2022). Plant glutamate receptors mediate a bet-hedging strategy between regeneration and defense. Dev. Cell 57:e456. doi: 10.1016/j.devcel.2022.01.013
Hoermayer, L., and Friml, J. (2019). Targeted cell ablation-based insights into wound healing and restorative patterning. Curr. Opin. Plant Biol. 52, 124–130. doi: 10.1016/j.pbi.2019.08.006
Howe, G. A. (2004). Jasmonates as signals in the wound response. J. Plant Growth Regul. 23, 223–237. doi: 10.1007/s00344-004-0030-6
Huber, A. E., and Bauerle, T. L. (2016). Long-distance plant signaling pathways in response to multiple stressors: the gap in knowledge. J. Exp. Bot. 67, 2063–2079. doi: 10.1093/jxb/erw099
Ikeuchi, M., Iwase, A., Rymen, B., Lambolez, A., Kojima, M., Takebayashi, Y., et al. (2017). Wounding triggers callus formation via dynamic hormonal and transcriptional changes. Plant Physiol. 175, 1158–1174. doi: 10.1104/pp.17.01035
Iwase, A., Kondo, Y., Laohavisit, A., Takebayashi, A., Ikeuchi, M., Matsuoka, K., et al. (2021). WIND transcription factors orchestrate wound-induced callus formation, vascular reconnection and defense response in Arabidopsis. New Phytol. 232, 734–752. doi: 10.1111/nph.17594
Iwase, A., Mitsuda, N., Koyama, T., Hiratsu, K., Kojima, M., Arai, T., et al. (2011). The AP2/ERF transcription factor WIND1 controls cell dedifferentiation in Arabidopsis. Curr. Biol. 21, 508–514. doi: 10.1016/j.cub.2011.02.020
Kang, J. N., Lee, W. H., Won, S. Y., Chang, S., Hong, J. P., Oh, T. J., et al. (2021). Systemic expression of genes involved in the plant defense response induced by wounding in Senna tora. Int. J. Mol. Sci. 22:10073. doi: 10.3390/ijms221810073
Kiep, V., Vadassery, J., Lattke, J., Maass, J. P., Boland, W., Peiter, E., et al. (2015). Systemic cytosolic Ca2+ elevation is activated upon wounding and herbivory in Arabidopsis. New Phytol. 207, 996–1004. doi: 10.1111/nph.13493
Koo, A. J., Gao, X., Jones, A. D., and Howe, G. A. (2009). A rapid wound signal activates the systemic synthesis of bioactive jasmonates in Arabidopsis. Plant J. 59, 974–986. doi: 10.1111/j.1365-313X.2009.03924.x
Kral, N., Hanna Ougolnikova, A., and Sena, G. (2016). Externally imposed electric field enhances plant root tip regeneration. Regeneration (Oxf) 3, 156–167. doi: 10.1002/reg2.59
Kumari, A., Chetelat, A., Nguyen, C. T., and Farmer, E. E. (2019). Arabidopsis H(+)-ATPase AHA1 controls slow wave potential duration and wound-response jasmonate pathway activation. Proc. Natl. Acad. Sci. U. S. A. 116, 20226–20231. doi: 10.1073/pnas.1907379116
Kurenda, A., Nguyen, C. T., Chetelat, A., Stolz, S., and Farmer, E. E. (2019). Insect-damaged Arabidopsis moves like wounded Mimosa pudica. Proc. Natl. Acad. Sci. U. S. A. 116, 26066–26071. doi: 10.1073/pnas.1912386116
Leon, J., Rojo, E., and Sanchez-Serrano, J. J. (2001). Wound signalling in plants. J. Exp. Bot. 52, 1–9. doi: 10.1093/jexbot/52.354.1
Li, J. H., Fan, L. F., Zhao, D. J., Zhou, Q., Yao, J. P., Wang, Z. Y., et al. (2021). Plant electrical signals: A multidisciplinary challenge. J. Plant Physiol. 261:153418. doi: 10.1016/j.jplph.2021.153418
Li, Q., Lei, S., Du, K., Li, L., Pang, X., Wang, Z., et al. (2016). RNA-seq based transcriptomic analysis uncovers alpha-linolenic acid and jasmonic acid biosynthesis pathways respond to cold acclimation in Camellia japonica. Sci. Rep. 6:36463. doi: 10.1038/srep36463
Li, L., Li, C., Lee, G. I., and Howe, G. A. (2002). Distinct roles for jasmonate synthesis and action in the systemic wound response of tomato. Proc. Natl. Acad. Sci. U. S. A. 99, 6416–6421. doi: 10.1073/pnas.072072599
Li, J., Zhu, S., Song, X., Shen, Y., Chen, H., Yu, J., et al. (2006). A rice glutamate receptor-like gene is critical for the division and survival of individual cells in the root apical meristem. Plant Cell 18, 340–349. doi: 10.1105/tpc.105.037713
Lin, W., Zhou, X., Tang, W., Takahashi, K., Pan, X., Dai, J., et al. (2021). TMK-based cell-surface auxin signalling activates cell-wall acidification. Nature 599, 278–282. doi: 10.1038/s41586-021-03976-4
Liu, J., Sheng, L., Xu, Y., Li, J., Yang, Z., Huang, H., et al. (2014). WOX11 and 12 are involved in the first-step cell fate transition during de novo root organogenesis in Arabidopsis. Plant Cell 26, 1081–1093. doi: 10.1105/tpc.114.122887
Major, I. T., Yoshida, Y., Campos, M. L., Kapali, G., Xin, X. F., Sugimoto, K., et al. (2017). Regulation of growth-defense balance by the JASMONATE ZIM-DOMAIN (JAZ)-MYC transcriptional module. New Phytol. 215, 1533–1547. doi: 10.1111/nph.14638
Marhava, P., Hoermayer, L., Yoshida, S., Marhavy, P., Benkova, E., and Friml, J. (2019). Re-activation of stem cell pathways for pattern restoration in plant wound healing. Cell 177:e913, 957–969.e13. doi: 10.1016/j.cell.2019.04.015
Matsuoka, K., Sato, R., Matsukura, Y., Kawajiri, Y., Iino, H., Nozawa, N., et al. (2021). Wound-inducible ANAC071 and ANAC096 transcription factors promote cambial cell formation in incised Arabidopsis flowering stems. Commun. Biol. 4:369. doi: 10.1038/s42003-021-01895-8
Mazur, E., Benkova, E., and Friml, J. (2016). Vascular cambium regeneration and vessel formation in wounded inflorescence stems of Arabidopsis. Sci. Rep. 6:33754. doi: 10.1038/srep33754
Miller, G., Schlauch, K., Tam, R., Cortes, D., Torres, M. A., Shulaev, V., et al. (2009). The plant NADPH oxidase RBOHD mediates rapid systemic signaling in response to diverse stimuli. Sci. Signal. 2:ra45. doi: 10.1126/scisignal.2000448
Moe-Lange, J., Gappel, N. M., Machado, M., Wudick, M. M., Sies, C. S. A., Schott-Verdugo, S. N., et al. (2021). Interdependence of a mechanosensitive anion channel and glutamate receptors in distal wound signaling. Sci. Adv. 7:eabg4298. doi: 10.1126/sciadv.abg4298
Mousavi, S. A., Chauvin, A., Pascaud, F., Kellenberger, S., and Farmer, E. E. (2013). GLUTAMATE RECEPTOR-LIKE genes mediate leaf-to-leaf wound signalling. Nature 500, 422–426. doi: 10.1038/nature12478
Mousavi, S. A., Nguyen, C. T., Farmer, E. E., and Kellenberger, S. (2014). Measuring surface potential changes on leaves. Nat. Protoc. 9, 1997–2004. doi: 10.1038/nprot.2014.136
Mudrilov, M., Ladeynova, M., Grinberg, M., Balalaeva, I., and Vodeneev, V. (2021). Electrical signaling of plants under abiotic stressors: transmission of stimulus-specific information. Int. J. Mol. Sci. 22:10715. doi: 10.3390/ijms221910715
Nguyen, C. T., Kurenda, A., Stolz, S., Chetelat, A., and Farmer, E. E. (2018). Identification of cell populations necessary for leaf-to-leaf electrical signaling in a wounded plant. Proc. Natl. Acad. Sci. U. S. A. 115, 10178–10183. doi: 10.1073/pnas.1807049115
Ni, J., Yu, Z., Du, G., Zhang, Y., Taylor, J. L., Shen, C., et al. (2016). Heterologous expression and functional analysis of rice GLUTAMATE RECEPTOR-LIKE family indicates its role in glutamate triggered calcium flux in rice roots. Rice (N Y) 9:9. doi: 10.1186/s12284-016-0081-x
Prasad, A., Sedlarova, M., Balukova, A., Rac, M., and Pospisil, P. (2019). Reactive oxygen species as a response to wounding: in vivo imaging in Arabidopsis thaliana. Front. Plant Sci. 10:1660. doi: 10.3389/fpls.2019.01660
Qiu, X. M., Sun, Y. Y., Ye, X. Y., and Li, Z. G. (2019). Signaling role of glutamate in plants. Front. Plant Sci. 10:1743. doi: 10.3389/fpls.2019.01743
Reymond, P., Weber, H., Damond, M., and Farmer, E. E. (2000). Differential gene expression in response to mechanical wounding and insect feeding in Arabidopsis. Plant Cell 12, 707–719. doi: 10.1105/tpc.12.5.707
Robertson, W. R., Clark, K., Young, J. C., and Sussman, M. R. (2004). An Arabidopsis thaliana plasma membrane proton pump is essential for pollen development. Genetics 168, 1677–1687. doi: 10.1534/genetics.104.032326
Salvador-Recatala, V. (2016). New roles for the GLUTAMATE RECEPTOR-LIKE 3.3, 3.5, and 3.6 genes as on/off switches of wound-induced systemic electrical signals. Plant Signal. Behav. 11:e1161879. doi: 10.1080/15592324.2016.1161879
Savatin, D. V., Gramegna, G., Modesti, V., and Cervone, F. (2014). Wounding in the plant tissue: the defense of a dangerous passage. Front. Plant Sci. 5:470. doi: 10.3389/fpls.2014.00470
Shao, Q., Gao, Q., Lhamo, D., Zhang, H., and Luan, S. (2020). Two glutamate- and pH-regulated Ca2+ channels are required for systemic wound signaling in Arabidopsis. Sci. Signal. 13:eaba1453. doi: 10.1126/scisignal.aba1453
Sozen, C., Schenk, S. T., Boudsocq, M., Chardin, C., Almeida-Trapp, M., Krapp, A., et al. (2020). Wounding and insect feeding trigger two independent MAPK pathways with distinct regulation and kinetics. Plant Cell 32, 1988–2003. doi: 10.1105/tpc.19.00917
Stahlberg, R., and Cosgrove, D. J. (1996). Induction and ionic basis of slow wave potentials in seedlings of Pisum sativum L. Planta 200, 416–425. doi: 10.1093/aob/mcg151
Sun, J. Q., Jiang, H. L., and Li, C. Y. (2011). Systemin/Jasmonate-mediated systemic defense signaling in tomato. Mol. Plant 4, 607–615. doi: 10.1093/mp/ssr008
Sussex, I. M., Goldsmith, M. H., and Clutter, M. E. (1972). Wound recovery by pith cell redifferentiation: structural changes. Am. J. Bot. 59, 797–804. doi: 10.1002/j.1537-2197.1972.tb10154.x
Szechynska-Hebda, M., Lewandowska, M., and Karpinski, S. (2017). Electrical signaling, photosynthesis and systemic acquired acclimation. Front. Physiol. 8:684. doi: 10.3389/fphys.2017.00684
Tian, W., Wang, C., Gao, Q., Li, L., and Luan, S. (2020). Calcium spikes, waves and oscillations in plant development and biotic interactions. Nat. Plants 6, 750–759. doi: 10.1038/s41477-020-0667-6
Toyota, M., Spencer, D., Sawai-Toyota, S., Jiaqi, W., Zhang, T., Koo, A. J., et al. (2018). Glutamate triggers long-distance, calcium-based plant defense signaling. Science 361, 1112–1115. doi: 10.1126/science.aat7744
Vega-Munoz, I., Duran-Flores, D., Fernandez-Fernandez, A. D., Heyman, J., Ritter, A., and Stael, S. (2020). Breaking bad news: dynamic molecular mechanisms of wound response in plants. Front. Plant Sci. 11:610445. doi: 10.3389/fpls.2020.610445
Yu, B., Wu, Q., Li, X., Zeng, R., Min, Q., and Huang, J. (2022). GLUTAMATE RECEPTOR-like gene OsGLR3.4 is required for plant growth and systemic wound signaling in rice (Oryza sativa). New Phytol. 233, 1238–1256. doi: 10.1111/nph.17859
Zhang, G., Zhao, F., Chen, L., Pan, Y., Sun, L., Bao, N., et al. (2019). Jasmonate-mediated wound signalling promotes plant regeneration. Nat. Plants 5, 491–497. doi: 10.1038/s41477-019-0408-x
Zimmermann, M. R., Maischak, H., Mithofer, A., Boland, W., and Felle, H. H. (2009). System potentials, a novel electrical long-distance apoplastic signal in plants, induced by wounding. Plant Physiol. 149, 1593–1600. doi: 10.1104/pp.108.133884
Keywords: electrical signal, ion channel, ion pump, jasmonic acid, membrane potential, systemic signaling, wounding
Citation: Lee K and Seo PJ (2022) Wound-Induced Systemic Responses and Their Coordination by Electrical Signals. Front. Plant Sci. 13:880680. doi: 10.3389/fpls.2022.880680
Edited by:
Simon Gilroy, University of Wisconsin-Madison, United StatesReviewed by:
Michael M. Wudick, Heinrich Heine University of Düsseldorf, GermanyMasatsugu Toyota, Saitama University, Japan
Copyright © 2022 Lee and Seo. This is an open-access article distributed under the terms of the Creative Commons Attribution License (CC BY). The use, distribution or reproduction in other forums is permitted, provided the original author(s) and the copyright owner(s) are credited and that the original publication in this journal is cited, in accordance with accepted academic practice. No use, distribution or reproduction is permitted which does not comply with these terms.
*Correspondence: Pil Joon Seo, cGpzZW8xQHNudS5hYy5rcg==