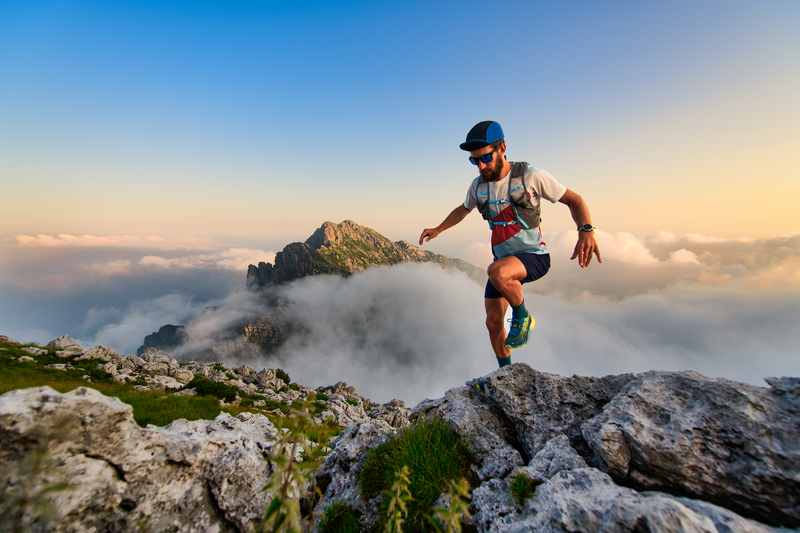
95% of researchers rate our articles as excellent or good
Learn more about the work of our research integrity team to safeguard the quality of each article we publish.
Find out more
REVIEW article
Front. Plant Sci. , 05 April 2022
Sec. Plant Breeding
Volume 13 - 2022 | https://doi.org/10.3389/fpls.2022.869713
This article is part of the Research Topic Legumes for Global Food Security, volume II View all 23 articles
Lentil (Lens culinaris Medik.) is a nutritionally dense crop with significant quantities of protein, low-digestible carbohydrates, minerals, and vitamins. The amino acid composition of lentil protein can impact human health by maintaining amino acid balance for physiological functions and preventing protein-energy malnutrition and non-communicable diseases (NCDs). Thus, enhancing lentil protein quality through genetic biofortification, i.e., conventional plant breeding and molecular technologies, is vital for the nutritional improvement of lentil crops across the globe. This review highlights variation in protein concentration and quality across Lens species, genetic mechanisms controlling amino acid synthesis in plants, functions of amino acids, and the effect of antinutrients on the absorption of amino acids into the human body. Successful breeding strategies in lentils and other pulses are reviewed to demonstrate robust breeding approaches for protein biofortification. Future lentil breeding approaches will include rapid germplasm selection, phenotypic evaluation, genome-wide association studies, genetic engineering, and genome editing to select sequences that improve protein concentration and quality.
Nutritional imbalances and deficiencies cause several malnutritional and non-communicable diseases (NCDs) in humans. A poor diet that lacks macro- and micronutrients, such as proteins, low-digestible carbohydrates (LDCs), fats, vitamins, and minerals, results in protein and micronutrient malnutrition. Low-digestible carbohydrates (LDs) are, also known as prebiotic carbohydrates, defined as ‘a substrate that is selectively utilized by host microorganisms conferring a health benefit’ (Gibson et al., 2017). These dietary prebiotic carbohydrates pass undigested through the upper digestive tract and are fermented by microorganisms in the colon for increased gut health. The most common human health impacts of malnutrition are stunting, intestinal health issues impairing digestion, obesity, overweight, and an increased risk of diet-related NCDs (Branca et al., 2019). Major NCDs related to poor dietary intake that threatens human life include cardiovascular diseases, cancer, chronic respiratory diseases, and diabetes (World Health Organization, 2019). Notably, a protein-deficient diet leading to protein malnutrition has alarming consequences that affect infants, young children, and females across the globe (Semba, 2016). However, a protein-rich legume-based diet is a viable, sustainable, and healthy option to prevent malnutrition in developing countries. Though animal proteins are extensively utilized in human diets, plant-based proteins have grown in popularity. Their demand has increased globally due to nutritional value, low carbon input, and environmental concerns (Asif et al., 2013).
Staple foods rich in macro- and micronutrients can alleviate the risk of malnutrition. Plant-based diets comprised mainly of cereals and legume staples are popular worldwide. Legume crops, including lentils (Lens culinaris Medikk.), have a protein concentration (20–30%) higher than cereals (10–12%) and thus have the potential to combat protein malnutrition and serve as gluten- and allergen-free protein sources. Lentil is highly nutritious, affordable and has a shorter cooking time than other pulse crops, and features high protein concentrations, low-digestible carbohydrates, minerals, vitamins, and low concentrations of phytic acid (Thavarajah et al., 2009; Kumar et al., 2015). Lentil is not a source of cholesterol, and its low-fat content makes it easier to digest than other pulse crops. Lentil proteins include both essential and non-essential amino acids but are notably low in the sulfur-containing amino acids methionine (Met) and cysteine (Cys; Khazaei et al., 2019). Biofortification is a possible approach to improve the unbalanced composition of amino acids in lentils through appropriate conventional breeding strategies and genomic selection. With increasing global protein demand, protein biofortification would justify lentils as a ‘nutritional booster’ to increase global nutritional security and combat malnutrition and NCDs.
Lentil proteins are stored in the cotyledonary cells in membranous protein bodies called ‘storage proteins’ (Duranti and Gius, 1997). These seed proteins supply carbon (C), nitrogen (N), and sulfur (S) and compose 80% of the total protein available for germination, subsequent plant growth, and disease resistance (Khazaei et al., 2019). Storage proteins also play a defensive role against bruchids, insects of the family Bruchidae, in cowpea (Vigna unguiculata; Sales et al., 2000). These proteins are classified into four types: globulins (salt soluble), albumins (water-soluble), prolamins (ethanol soluble), and glutelins (acid-soluble; Osborne, 1924). Like other pulse crops, lentils are rich in globulins and albumins, whereas prolamins and glutelins are more prominent in cereals (Osborne, 1924). Globulins were the first type of storage protein reported in lentils (Osborne and Campbell, 1898) and are the principal proteins in lentils, making up ~44–70% of all storage proteins. Two subclasses of globulins, i.e., 11 s type (legumin) and 7 s type (vicilin/convicilin), were also defined (Danielson, 1950). Albumins comprise 26–61% of lentil proteins, and prolamins and glutelins only make up a small fraction (Saint-Clair, 1972; Sulieman et al., 2008).
Storage protein quantities demonstrate high variability due to the quantitative nature of the genes regulating protein synthesis in the seeds (Kumar et al., 2020). Higher genotype × environmental interactions, indicated by the moderate broad sense heritability (31.31%), is another reason for the high variation in the storage protein concentration in lentil seeds (Gautam et al., 2018). Lentil seed proteins, excluding storage proteins, also have metabolic functions. These metabolic proteins regulate numerous physiological processes in the plant, including enzymatic activity and structural and physiological functions (Scippa et al., 2010). Ultimately, lentil seed protein composition contributes to human health by providing essential amino acids necessary for metabolic processes and nutritional balance in the human body. Optimizing the plant breeding process and location sourcing may help develop better protein-enriched lentil cultivars for global plant-based protein demand. The objectives of this paper are to review the protein concentration and quality variations within the genus Lens, pathways and genes regulating the synthesis of amino acids, functions of amino acids for human health, and breeding strategies related to lentil protein biofortification.
Lentil is an annual diploid (2n = 2x = 14) cool-season food legume that originated in the Middle East (Cubero, 1981). The genus Lens comprises L. culinaris, L. ervoides, L. nigricans, and L. lamoletti. L. culinaris is further divided into four taxa: L. culinaris ssp. culinaris, L. culinaris ssp. orientalis, L. culinaris ssp. tomentosus, and L. culinaris ssp. odemensis (Ferguson et al., 2000). Lens genus has been classified as primary, secondary, tertiary, and quaternary genetic pools according to the phylogeny using the Genotyping-by-sequencing (GBS). The primary gene pool contains L. culinaris, L. orientalis, and L. tomentosus, whereas L. odemensis and L. lamoletti are in the secondary gene pool. However, each tertiary and quaternary gene pools contain single species, L. ervoides and L. nigricans, respectively (Wong et al., 2015). Of these, only L. culinaris ssp. culinaris is domesticated and cultivated worldwide, representing crops over a 5.01 M ha area with an annual production of 6.54 M tonnes. Canada is a leading producer, contributing about 44% of the world’s lentils; other major lentil-producing countries are India, the United States of America (United States), Turkey, Australia, Nepal, and Bangladesh (FAOSTAT, 2021).
Lentils are a staple food that is easily digested compared to other legumes. The biofortification of lentils could significantly fight hidden hunger and nutritional disorders. Hidden hunger is also known as micronutrient deficiency despite sufficient calorie intake (Lowe, 2021). Several breeding programs have been established worldwide that seek to biofortify lentils with protein, prebiotic carbohydrates, micronutrients, vitamins, etc. (Kumar et al., 2016a). Many lentil accessions have been screened for amino acid concentration (Iqbal et al., 2006), protein (Bhatty and Slinkard, 1979), starch (Zia-Ul-Haq et al., 2011), fatty acids (Grusak, 2009), macro- and micronutrients (Kumar et al., 2016a; Podder et al., 2020; Rasheed et al., 2020), folates (Sen Gupta et al., 2013), and antinutritional factors (Thavarajah et al., 2009, 2011). Marker-assisted breeding has also demonstrated the potential for identifying genes/quantitative trait loci (QTL) for iron (Fe) uptake (Kumar et al., 2015; Aldemir et al., 2017), Fe and Zinc (Zn) concentration (Kumar et al., 2014), and selenium (Se) concentration (Ates et al., 2016). Furthermore, the HarvestPlus Challenge program, established in 2004, was a landmark effort that increased lentil biofortification efforts worldwide. They released several lentil cultivars to economically underprivileged global regions in Asia and Africa (Kumar et al., 2016a). Notably, numerous high Fe and Zn cultivars have been released, including Barimasur-4, -5, -6, -7, -8 and -9 in Bangladesh; Khajuraho-1, -2 and -3, Sital, Shekhar, Sisir, and Simal in Nepal; L 4704, IPL 220, Pusa Agaiti and Pusa Vaibhav in India; Idlib-2 and -3 in Syria/Lebanon; and Alemeya in Ethiopia. Smallholder farmers regularly use these biofortified lentils in Africa and Southeast Asia (Harvest Plus, 2014).
Various researchers have reported protein concentrations in current lentil cultivars in the range of 20–30% (Table 1). In a study (Bhatty, 1986), similar protein concentrations in wild and cultivated lentils, indicating homogeneity for protein concentration in the genus Lens, were identified. However, a recent study (Kumar et al., 2016b) efficiently distinguished wild species from cultivated lentils for protein concentration. In this study, L. orientalis, an immediate progenitor of cultivated lentils, expressed the highest average protein (24.15%) among all the wild species, followed by L. ervoides (22.99%). Other wild species, L. odemensis, and L. nigricans showed slightly higher average protein content (19.7 and 19.53%, respectively) than L. culinaris. A similar protein level was seen in L. tomentosus (18.75%) and cultivated lentils (18.7%). Extensive variation was observed for protein content within L. orientalis and L. ervoides, ranging from 18.3 to 27.75% and 18.9 to 32.7%, respectively. ILWL-47, an L. ervoides accession, had an exceptionally high protein content of about 32.7% and is, therefore, a potential candidate for protein quality improvement in lentil breeding programs (Kumar et al., 2016b). Protein subunit fraction profiling has indicated variable levels of the albumin protein fraction (APF) and globulin protein fraction (GPF) among Lens species, with the wild species having higher APF and GPF concentrations than the cultivated species (Bhatty, 1982). Among the evaluated wild species, L. orientalis and L. ervoides contained higher APF and GPF levels than L. nigricans (Bhatty, 1982).
The proportion of amino acids in lentil proteins varies across genotypes in the cultivated gene pool (Table 2). Met and tryptophan (Trp) represent a minor fraction among all amino acids and are thus termed limiting amino acids. Comparing lentil protein with cereal proteins indicates the good nutritional complementation between Met and lysine (Lys), but to some extent, for Trp and threonine (Thr) because cereals are rich in both Met and Trp (Bhatty, 1986). Generally, all essential amino acids except Lys are deficient in lentils, but a moderate to the high proportion of non-essential amino acids are present (Khazaei et al., 2019). Lentil proteins are also lacking in other S-containing amino acids such as Cys. The albumin fraction of lentils contains more essential amino acids than the globulin fraction (Bhatty, 1982). Recent studies also indicate that amino acids vary among distinct species of the genus Lens, with a spectrum of variation seen for amino acid content among L. culinaris, L. orientalis, L. ervoides, L. nigricans, and L. odemensis. Phenylalanine (Phe), Met, valine (Val), leucine (Leu), and isoleucine (Ile) concentrations are significantly higher in wild species than cultivated lentils (Table 3; Rozan et al., 2001). Similarly, the non-essential amino acid content is also higher in wild species than in L. culinaris. Such evidence signifies wild species are a potential source of candidate genes that can be harnessed to improve protein quality in cultivated lentils.
Table 2. Amino acid profile of cultivated lentil genotypes (Sayeed and Njaa, 1985; Shekib et al., 1986; Kahraman, 2018).
Table 3. Amino acid concentrations among different Lens species (Rozan et al., 2001).
The genetic mechanisms controlling seed protein concentration have similar regulation and pathways in different plants, including pulse crops. In pulse crops, genetic control of seed protein content has not been widely studied except in chickpea (Cicer arietinum), soybean (Glycine max), and pea (Pisum sativum). However, genetic control of seed protein content has been studied extensively in cereals (Mann et al., 2009; Olsen and Phillips, 2009; Chen et al., 2018; Borisjuk et al., 2019) and the model plant Arabidopsis thaliana (Jasinski et al., 2016). In chickpea, seven candidate genes that regulate seed protein concentration were identified using a genome-wide association study of 336 desi and Kabuli accessions (Upadhyaya et al., 2016). In soybean, three QTL (qPro10a, qPro13a, and qPro17b) for protein were identified in a recombinant inbred line (RIL) population (Zhonghuang 24 × Huaxia 3) on chromosomes 10, 13, and 17, respectively (Liu et al., 2017).
Several genes regulating the seed protein concentration in soybean were found on chromosomes 15 and 20 (Patil et al., 2017). Another gene, BIG SEEDS1 (BS1), controlling seed size, weight, and composition of amino acids in the protein, has been characterized in Medicago trunculata and soybean (Ge et al., 2016). Groups of highly coordinated genes (HCGs) controlling the aspartate family (Met, Ile, Lys, Thr, and Gly) and branched aromatic amino acid formation were also identified in A. thaliana (Less and Galili, 2009). These two HCGs have several genes controlling the formation of amino acids. The first group related to the aspartate family contained catabolic genes for THA1 (Thr to Gly metabolism), BCAT2 (Ile metabolism), MGL (Met catabolism), and LKR/SDH (Lys metabolism). However, the second group exclusively regulated Met metabolism and was termed the ‘Met metabolism group.’ It contained the genes AK/HSDH1 (encoding aspartate kinase enzyme for the formation of aspartate-4-semialdehyde, the first substrate for amino acid synthesis), CGS1 (Met synthesis), DAPD (Lys synthesis), SAMS3 (Met catabolism), BCAT3 (Ile metabolism), and BCAT4, MAM1, and MAML (Met catabolism). One of the two groups related to branched aromatic acids contained ten genes (ASA1, ASB, TSA2, TSB1/2, IGPS for Trp synthesis, CYP79B2 for Trp catabolism, PD for Phe synthesis, PAL1 and PAL2 for Phe catabolism, and TAT3 for tyrosine (Tyr) catabolism). In contrast, two genes (PAL3 and IGPS) were reported in the second group (Less and Galili, 2009).
The genes regulating the synthesis of enzymes that mediate the formation of amino acids and their precursors have been extensively studied in plants (Table 4; Figure 1). In A. thaliana, glutamate is formed from precursor 2-oxoglutarate by enzymatic aminotransferases, a process that is regulated by 44 putative genes (Liepman and Olsen, 2004). Glutamate synthase production, which converts glutamine (Gln) to glutamate, is controlled by either one or two genes in the chloroplast and mitochondria (Gaufichon et al., 2016). Similarly, six genes encode Gln synthase, which converts glutamate to Gln, in A. thaliana (Forde and Lea, 2007). Glutamate is a precursor that synthesizes arginine (Arg) and proline (Pro) using 20 enzymes encoded by about 30 genes in A. thaliana (Majumdar et al., 2016). Glutamine with aspartate also forms asparagine (Asn) in plants by the transamination action of the Asn synthetase (AS) enzyme encoded by the asnB gene in eukaryotes (Gaufichon et al., 2010) and the ASN gene family (ASN1, ASN2, and ASN3) in Arabidopsis (Table 4; Arabidopsis Genome Initiative, 2000). A histidine (His) synthesis pathway revealed eight genes (ATP-PRT, PRATP/CH, ProFAR-I, IGPS, IGPD, HPA, HPP, and HDH) forming eight enzymes in A. thaliana (Rees et al., 2009). Two branched-chain amino acids, Val and Leu, form with the acetohydroxyacid synthase (AHAS) enzyme acting on pyruvate producing acetolactate. This enzyme forms the third branched-chain amino acid, Ile, by serving on a substrate formed from Thr in the pathway for 2-ketobutyrate converting Thr to Ile. A single gene encodes the AHAS enzyme in Arabidopsis (Singh and Shaner, 1995).
Figure 1. Pathways synthesizing various essential (green boxes) and non-essential (purple boxes) amino acids. Amino acids: Ala, alanine; Arg, arginine; Asn, asparagine; Asp, aspartate/aspartic acid; Cys, cysteine; Gln, glutamine; Glu, glutamate/glutamic acid; Gly, glycine; His, histidine; Ile, isoleucine; Leu, leucine; Lys, lysine; Met, methionine; Phe, phenylalanine; Pro, proline; Ser, serine; Thr, threonine; Trp, tryptophan; Tyr, tyrosine; Val, valine). Substrates/precursors: acetyl-CoA, acetyl-coenzyme A; DAHP, 3-deoxy-D-arabinoheptulosonate-7-phosphate; ESPS, 5-enolpyruvylshikimate-3-phosphate; E4P, erythrose 4-phosphate; fructose-6-P, fructose-6-phosphate; GA3P, glyceraldehyde 3-phosphate; glucose-6-P, glucose-6-phosphate; histidinol-P, histidinol phosphate; IAP, imidazole acetol-phosphate; PEP, phosphoenol pyruvate; 3-PGA, 3-phosphoglyceric acid; PRFAR, (N´-[(5′-phosphoribulosyl)formimino]-5-aminoimidazole-4-carboxamide) ribonucleotide); PRPP, phosphoribosyl diphosphate; R5P, ribose 5-phosphate; Ru5P, ribulose 5-phosphate. Enzymes indicated in parentheses: ADH, arogenate dehydrogenase; ADT, arogenate dehydratase; AHAS, acetohydroxyacid synthase; AK, aspartate kinase; ALT, alanine transferase; AS, anthranilate synthase; AsnS, asparagine synthetase; AspAT, aspartate aminotransferase; BCAT, branched-chain amino acid aminotransferase; CGS, cystathionine gamma synthase; CM, chorismate mutase; DHDPS, dihydrodipicolinate synthase; GOGAT, glutamate synthase; GS, glutamine synthetase; HSD, homoserine dehydrogenase; IPMS, isopropylmalate synthase; MS, methionine synthase; OASTL, O-acetylserine(thiol)lyase; SAT, serine acetyltransferase; SHM, serine hydroxymethyltransferase; TD, threonine deaminase; TrpS, tryptophan synthase; TS, threonine synthase.
The enzyme chorismate mutase (CM) is encoded by three genes (AtCM1, AtCM2, and AtCM3) and is a precursor for chorismate to form prephenate for Phe and Tyr biosynthesis in plants (Figure 1). The formation of Trp from chorismate is regulated by three genes (ASa1, ASa2, and ASb1) and seven putative genes (two Asa and five ASb genes) encoding anthranilate synthase (AS) enzyme-producing anthranilate (Table 4). This anthranilate generates Trp using five enzymes (PAT1, PAI, IGPS, TS a, and TS b) encoded by eight genes in plants (Tzin and Galili, 2010; Parthasarathy et al., 2018). Aspartate regulates the formation of four essential amino acids, Ile, Lys, Met, and Thr, also termed aspartate-derived amino acids. Five genes encode aspartate formation enzymes in A. thaliana (Han et al., 2021). In C3 plants, including lentils, two pathways are identified for serine (Ser) formation, namely photorespiratory and non-photorespiratory pathways in photosynthetic and non-photosynthetic tissues, respectively (Figure 1). The Ser produced in different pathways is converted into glycine (Gly) in non-photosynthetic tissues in the presence of the Ser hydroxymethyltransferase (SHM) enzyme. Ser also synthesizes Cys by following a two-step pathway in plants regulated by Ser acetyltransferase (SAT) and O-acetylserine (thiol)lyase (OASTL) enzymes encoded by five and nine genes, respectively (Howarth et al., 1997; Wirtz et al., 2004).
Amino acids are the foundational units of proteins. Structural conformations have unique chemical properties due to basic (amide) and acidic (carboxylic) chemical groups. Based on the human nutritional requirements, amino acids have been classified in several ways—essential or non-essential. Essential amino acids are indispensable because the human body cannot synthesize them; hence, appropriate concentrations in the diet are necessary (Table 5). Non-essential amino acids, synthesized in the human body, are also called dispensable amino acids (Reeds, 2000). However, some non-essential amino acids are considered conditionally non-essential because their abundance in the human body declines in times of stress or sickness. External sources are required to maintain necessary quantities (Fürst and Young, 2000).
The role of amino acids (individually or in combination) was first studied in rats to evaluate the necessity of Lys and Trp in food sources containing gliadin proteins. This initial study documented the adverse effects of amino acid deficiency on rats (Osborne and Mendel, 1914). Based on preliminary classical studies using model organisms (Ackroyd and Hopkins, 1916; Rose and Cox, 1924), an analogy of amino acid functions and dietary requirements in humans was first established by Rose and co-workers in 1947 (Rose et al., 1947). This study played a significant role in recognizing and classifying essential and non-essential amino acids based on their impacts on human health. Amino acids perform several crucial functions in the human body, either directly or indirectly. Amino acids have a specific role in gene expression (Oommen et al., 2005), signaling pathways for activation of immune systems (Kim et al., 2007), have nutraceutical effects for improving health status by regulating metabolic activities (Duranti, 2006), and can be used to treat genetic disorders (van Vliet et al., 2014).
Amino acids govern the epigenetic regulation of gene expression through DNA modifications. DNA modifications such as methylation and acetylation occur due to the binding of DNA to C groups (methyl, acetyl) donated by Met, His, Ser, and Gly (Oommen et al., 2005; Kouzarides, 2007). Acetylation leads to the detachment of histones from DNA to favor its exposure-promoting transcription process. However, methylation plays a role in the reverse direction by densely packing the DNA and encouraging gene silencing (Wu, 2010). Studies also demonstrate the role of Gln in the regulation of intestinal gene expression in rats, promoting intestinal health concerning cell growth and antioxidation activity (Wang et al., 2008). Arg supplementation in rats leads to the upregulation of gene expression, preventing oxidative stress and promoting fatty acid metabolism and glucose metabolism (McKnight et al., 2010). At the transcriptional level, amino acids regulate the activity of RNA polymerase by altering its specificity for promoters and enhancing the binding of some repressors near the non-coding sequences adjacent to the promoter region (Oommen et al., 2005). Such studies demonstrate the remarkable contribution of different amino acids in regulating gene expression.
The human immune system consists of both innate and acquired immune subsystems that regulate the response and protection of the human body upon pathogen attack (Calder, 1995). The innate immune system is a natural system that immediately activates when pathogens enter the body and can only prevent the entry and initial establishment of the pathogen. It comprises the physiological barriers, monocytes, macrophages, neutrophils, basophils, natural killer cells, mast cells, platelets, and various humoral factors (Buchanan et al., 2006). However, once the pathogen invades the innate immune system and colonizes, the acquired immune system is activated to decrease further pathogen progress. The acquired immune system consists of lymphocytes (T- and B-lymphocytes) that have immunological memory for invading pathogens (Calder, 2006). Human immune systems require a range of amino acids to produce immunoglobulins, cytokines, and other biomolecules to prevent diseases (Kim et al., 2007).
Several amino acids (branched-chain amino acids: BCAA (Leu, Ile, and Val), alanine (Ala), Gln, Ser, Pro, and Thr) regulate the proliferation of lymphocytes (Li et al., 2007). These amino acids either directly participate (Ala, Ser, and Thr) or produce signal molecules or hormones (BCAA, Gln, and Pro) to stimulate lymphocyte proliferation and create various immune responses (Li et al., 2007). Moreover, BCAAs participate in lipid metabolism (Nishimura et al., 2010) and blood glucose maintenance. In females, BCAAs also regulate blastocyst development and embryo implantation, fetal growth by hormonal secretions, stimulate mammary gland function and lactation, and increase aspartate, Gln, and glutamate synthesis (Zhang et al., 2018). Met, His, Gly, and Phe regulate the synthesis of signaling molecules controlling immune responses. Individually or in combination, these amino acids control the production of immune cell signaling molecules, leading to major immunity-boosting elements such as cytokines and antibodies (Li et al., 2007). Amino acid oxidases (AAOs) derived from L-isomers of Phe, Trp, Tyr, and Leu possess antimicrobial (Phua et al., 2012) and antitumoral functions (Lee et al., 2014).
Legumes have antinutritional compounds, including trypsin and chymotrypsin inhibitors, phytic acids, and tannins, which reduce nutrient bioavailability (Vidal-Valverde et al., 1994; Shi et al., 2017). Lentil is naturally low in phytic acid (Thavarajah et al., 2009) and contains trypsin inhibitors (3.6–7.6 units/mg protein) and tannins (1.28–3.9 mg/g; Hefnawy, 2011). Inactivity of trypsin and chymotrypsin enzymes causes difficulties in lysis proteins into small peptides and eventually affects the release of amino acids from small peptides. Tannins are phenolic inhibitors that bind to proteins via Lys or Met cross-links (Davis, 1981) and make insoluble complexes with carbohydrates (Reddy et al., 1985). In lentils, trypsin and chymotrypsin inhibitors and phytic acids are present in seed cotyledons, whereas tannins are concentrated mainly in the seed coat (Dueñas et al., 2002). Different food processing methods, including dehulling and cooking, are recommended to reduce these antinutritional properties (Acquah et al., 2021). Dehulling effectively reduces the tannins by removing the seed coat (Goyal et al., 2009). In pulses, other common processing treatments are soaking, hydrothermal treatments (cooking and roasting), fermentation, and irradiation (Acquah et al., 2021). Soaking reduces trypsin and chymotrypsin inhibitors, phytic acids, and tannins in lentils depending on the soaking time (Shi et al., 2017). Thermal methods are recommended for denaturing trypsin and chymotrypsin inhibitors and removing tannin in lentils (Hefnawy, 2011). Fermentation and irradiation are alternate methods to reduce antinutritional compounds (Siddhuraju et al., 2002; Maleki and Razavi, 2021) but have not been widely studied in pulses.
Pulse breeding programs focus on meeting the world’s food demand and ensuring global food security. The primary objectives of these breeding programs are to increase the yield by efficient selection from available germplasm, introduce hybrid lines, cross contrasting lines to exploit heterosis, develop biotic and abiotic stress-tolerant cultivars, and induce mutations to generate novel variability with molecular and genomic techniques. Today, most conventional pulse breeding programs employ molecular markers for traits of interest. Genetic engineering technology has demonstrated remarkable potential to modify plants for specific breeding objectives. Thereby, technological advancement has broadened the scope of plant breeding to enable special-purpose breeding programs such as nutritional quality improvement programs or nutritional breeding (Kumar et al., 2020).
Conventional breeding approaches focus on improving highly heritable traits governed by a few genes. Quantitative traits with low heritability and high environmental effects, such as protein and other nutritional quality traits, do not significantly respond to selection by conventional breeding methods. In crop plants, including pulses, protein concentration negatively correlates with yield (Qureshi et al., 2013); therefore, selecting either trait negatively affects the other. For this reason, conventional approaches, such as mass selection, pedigree method, and bulk method, face challenges for protein quality improvement, but adding genetic markers into the breeding pipeline is possible. A comprehensive study comparing relative protein concentration among different lentil species identified a high protein accession, ILWL 47, belonging to L. ervoides (Bhatty, 1986). Lentil cultivar., IC317520, was identified as a high protein, sugar, and starch cultivar (Tripathi et al., 2019). The identified candidates can improve protein content in cultivated lentils by hybridization-based breeding methods.
Compared to selection and hybridization-based methods, mutation breeding has improved legume protein. A mutant lentil variety, NIA-MASOOR-5, with increased protein concentration, high yield, and disease resistance was created by gamma irradiation of M-85 as a parent and released in Pakistan (Ali and Shaikh, 2007). Mutation using gamma radiation has increased protein levels in mutants obtained from Chiang Mai 60, SSRSN35-19-4, and EHP 275 cultivars of soybean (Yathaputanon et al., 2009). Some high-protein and low-fiber mutants were identified from gamma ray-irradiated and ethyl methanesulfonate (EMS)-treated Himso 1563 and TS 82 cultivars in soybean (Kavithamani et al., 2010). EMS also induced beneficial mutations for protein and oil content improvement in Huayu 22 and Yueyou 45 cultivars of peanut (Chen et al., 2020). A high-yielding and high-protein chickpea mutant variety, Hyprosola or Faridpur-1, was also developed by gamma irradiation in Bangladesh (Oram et al., 1987). TAEK-SAGEL is another gamma radiation-derived, high-protein mutant variety of chickpea released in Turkey (Saǧel et al., 2009). Such landmark achievements of mutation breeding in pulse crops, including lentils on a commercial scale, demonstrate the success of this method for improving quality traits.
Genomic-assisted breeding demonstrates the broad potential for improving quantitative traits, which are highly complex, controlled by many genes, and environmentally influenced (Kumar et al., 2016a). The current genomic toolbox for breeding includes genetic marker development, linkage map construction, identifying QTL and alien introgressions, candidate gene discovery, diversity analysis, genome sequencing, and pangenome construction. The use of molecular markers to gear up genomic developments in lentils for various traits has been reviewed widely (Kumar et al., 2015). Several legume crops, including dry pea (Pisum sativum L.), soybean, and chickpea, have been broadly investigated for use in genomic-assisted breeding to identify putative genomic regions governing seed protein concentration. The QTL mapping approach in dry pea revealed three genes regulating protein concentration using a linkage map of 207 markers (AFLP, RAPD, and STS markers; Tar’an et al., 2004). Another similar mapping study in dry pea using 204 markers (morphological, isozyme, AFLP, ISSR, STS, CAPS, and RAPD) identified genomic regions for seed protein concentration (Irzykowska and Wolko, 2004). Several other studies using genomic-assisted breeding in dry pea identified protein concentration-related genes (Tayeh et al., 2015). However, these studies are limited in the number of dry pea accessions used in each study and the genome-wide comparisons. Furthermore, a restriction-site associated DNA sequencing (RAD-seq) approach identified 47,472 SNP markers in a soybean RIL population (Liu et al., 2017), and several genes for the seed protein in soybean were found using transcriptome analysis, QTL mapping, and the genome-wide association study (GWAS) approach (Patil et al., 2017). A gene controlling seed size, weight, and composition of amino acids in total protein concentration were characterized in model legume Medicago trunculata and soybean using PCR-based markers and transcriptome profiling (Ge et al., 2016). Likewise, extensive studies in soybean have also identified several seed protein genes by exploiting genomic breeding approaches (Brummer et al., 1997; Sebolt et al., 2000; Chapman et al., 2003; Chung et al., 2003; Liang et al., 2010; Van and Mchale, 2017; Li et al., 2018; Huang et al., 2020). A high-throughput genotyping technology study identified 16,376 SNPs and revealed seven major genes for seed protein through a GWAS in 336 desi and Kabuli chickpea accessions (Upadhyaya et al., 2016). Such studies in legume crops demonstrate the success of marker-based genomic tools for improving protein concentration and quality. However, marker-based genomic-assisted studies identifying genic regions associated with seed protein content and quality have not been reported in lentils so far.
Genetic engineering technology has provided other insights to improve protein concentration in legumes. Protocols have been designed to develop transgenic lines in chickpea (Fontana et al., 1993), common bean (Russell et al., 1993), lupin (Molvig et al., 1997), peanuts (Brar et al., 1994), pea (Schroeder et al., 1993) and soybean (Hinchee et al., 1988). Several research groups have developed transgenic soybean lines with increased S-containing amino acids (Falco et al., 1995; Dinkins et al., 2001; Guo et al., 2020). Likewise, transformation studies to improve seed protein concentration in broad bean (Montamat et al., 1999), dry pea (Tegeder et al., 2007), and French bean (Tan et al., 2008) have also been reported. Recently, the genome-editing tool CRISPR/Cas 9 has emerged as a revolutionary approach to improving staple food crops, but this approach is not widespread in pulses except in soybean.
Most lentil breeding programs worldwide focus on yield improvement, disease resistance, biotic/abiotic stress tolerance, and germplasm diversity. Lentils are a nutrient-dense superfood to combat malnutrition and non-communicable diseases. As such, lentil protein quality has recently emerged as a target trait for lentil breeding programs due to the increased demand for plant-based protein. Conventional breeding is progressing for lentil crop nutritional improvement, but other genomic approaches are essential to speed up the breeding process due to the quantitative nature of these traits. Genome-wide association studies with conventional plant breeding approaches are appropriate for improving the genetic gain of quantitative traits by increasing selection accuracy through indirect selection (Rutkoski, 2019). For example, the genetic gain for lentil protein concentration can be achieved by selecting diverse parents, increasing the selection intensity, accuracy and reducing the selection cycle duration by increasing the number of generations per year. Conventional methods like pedigree, bulk, and mutation breeding can develop new breeding material using wild species, cultivars, landraces, advanced/elite breeding lines, and genetic stocks (Figure 2). These breeding methods will generate broadly diversified germplasm used for phenotyping and genotyping platforms to enhance selection accuracy (Xu et al., 2017). However, these conventional methods do not increase the selection intensity due to low heritability, slow progression, and visual phenotypic selection (Cobb et al., 2019). Combining genomic-assisted breeding with rapid generation methods such as single-seed descent, speed breeding, and double haploid production will enhance selection intensity and shorten the selection cycle, resulting in increased genetic gain over time (Cobb et al., 2019; Figure 3). Future lentil breeding efforts should focus on the rapid diversification and evaluation of lentil germplasm for protein quality through conventional breeding approaches. The development and adoption of genomic resources and tools such as genetic engineering or genome editing may also contribute to the pace of conventional breeding in lentils and eventually lead to breakthroughs in lentil protein improvement programs to ensure nutritional security and improve human health.
SS is a doctoral student under the supervision of DT who drafted the paper objectives, wrote the first draft, revised and edited the final version of this paper. JLB, PT, and SK edited/reviewed the final version and provided revisions and edits constructively. DT supervised SS and designed the objectives with SS, wrote parts of the paper, edited and revised the last version. All authors contributed to the article and approved the submitted version.
Funding support for this project was provided by the Organic Agriculture Research and Extension Initiative (OREI; award no. 2018-51300-28431/proposal no. 2018-02799; and award no. 2021-51300-34805/proposal no. 2021-02927) of the United States Department of Agriculture, National Institute of Food and Agriculture (DT, LB), and the USDA National Institute of Food and Agriculture, [Hatch] project [1022664] (DT); the Good Food Institute (DT); and the FoodShot Global. Its contents are solely the responsibility of the authors and do not necessarily represent the official views of the USDA.
The authors declare that the research was conducted in the absence of any commercial or financial relationships that could be construed as a potential conflict of interest.
All claims expressed in this article are solely those of the authors and do not necessarily represent those of their affiliated organizations, or those of the publisher, the editors and the reviewers. Any product that may be evaluated in this article, or claim that may be made by its manufacturer, is not guaranteed or endorsed by the publisher.
Ackroyd, H., and Hopkins, F. G. (1916). Feeding experiments with deficiencies in the amino-acid supply: arginine and histidine as possible precursors of purines. Biochem. J. 10, 551–576. doi: 10.1042/bj0100551
Acquah, C., Ohemeng-Boahen, G., Power, K. A., and Tosh, S. M. (2021). The effect of processing on bioactive compounds and nutritional qualities of pulses in meeting the sustainable development goal 2. Front. Sustainable Food Syst. 5:681662. doi: 10.3389/fsufs.2021.681662
Aldemir, S., Ateş, D., Temel, H. Y., Yağmur, B., Alsaleh, A., Kahriman, A., et al. (2017). QTLs for iron concentration in seeds of the cultivated lentil (Lens culinaris Medic.) via genotyping by sequencing. Turk. J. Agric. For. 41, 243–255.
Alghamdi, S. S., Khan, A. M., Ammar, M. H., El-Harty, E., Migdadi, H. M., El-Khalik, S., et al. (2014). Phenological, nutritional and molecular diversity assessment among 35 introduced lentil (Lens culinaris Medik.) genotypes grown in Saudi Arabia. Int. J. Mol. Sci. 15, 277–295. doi: 10.3390/ijms15010277
Ali, J. A. F., and Shaikh, N. A. (2007). Genetic exploitation of lentil through induced mutations. Pak. J. Bot. 39, 2379–2388.
Arabidopsis Genome Initiative (2000). Analysis of the genome sequence of the flowering plant Arabidopsis thaliana. Nature 408, 796–815. doi: 10.1038/35048692
Asif, M., Rooney, L. W., Ali, R., and Riaz, M. N. (2013). Application and opportunities of pulses in food system: a review. Crit. Rev. Food Sci. Nutr. 53, 1168–1179. doi: 10.1080/10408398.2011.574804
Ates, D., Sever, T., Aldemir, S., Yagmur, B., Temel, H. Y., Kaya, H. B., et al. (2016). Identification QTLs controlling genes for se uptake in lentil seeds. PLoS One 11:e0149210. doi: 10.1371/journal.pone.0149210
Bhatty, R. S. (1982). Albumin proteins of eight edible grain legume species. Electrophoretic patterns and amino acid composition. J. Agric. Food Chem. 30, 620–622. doi: 10.1021/jf00111a057
Bhatty, R. S. (1986). Protein subunits and amino acid composition of wild lentil. Phytochemistry 25, 641–644. doi: 10.1016/0031-9422(86)88015-3
Bhatty, R. S., and Slinkard, A. E. (1979). Composition, starch properties and protein quality of lentils. Can. Inst. Food Sc. Technol. J. 12, 88–92. doi: 10.1016/s0315-5463(79)73062-8
Borisjuk, N., Kishchenko, O., Eliby, S., Schramm, C., Anderson, P., Jatayev, S., et al. (2019). Genetic modification for wheat improvement: from transgenesis to genome editing. Biomed. Res. Int. 2019, 1–18. doi: 10.1155/2019/6216304
Branca, F., Lartey, A., Oenema, S., Aguayo, V., Stordalen, G. A., Richardson, R., et al. (2019). Transforming the food system to fight non-communicable diseases. BMJ 364:l296. doi: 10.1136/bmj.l296
Brar, G. S., Cohen, B. A., Vick, C. L., and Johnson, G. W. (1994). Recovery of transgenic peanut (Arachis hypogaea L.) plants from elite cultivars utilizing ACCELL® technology. Plant J. 55, 745–753. doi: 10.1111/j.1365-313X.1994.00745.x
Brummer, E. C., Graef, G. L., Orf, J., Wilcox, J. R., and Shoemaker, R. C. (1997). Mapping QTL for seed protein and oil content in eight soybean populations. Crop Sci. 37, 370–378. doi: 10.2135/cropsci1997.0011183X003700020011x
Buchanan, J. T., Simpson, A. J., Aziz, R. K., Liu, G. Y., Kristian, S. A., Kotb, M., et al. (2006). DNase expression allows the pathogen group a streptococcus to escape killing in neutrophil extracellular traps. Curr. Biol. 16, 396–400. doi: 10.1016/j.cub.2005.12.039
Calder, P. C. (1995). Fuel utilization by cells of the immune system. Proc. Nutr. Soc. 54, 65–82. doi: 10.1079/pns19950038
Calder, P. C. (2006). Branched-chain amino acids and immunity. J. Nutr. 136, 288S–293S. doi: 10.1093/jn/136.1.288S
Chapman, A., Pantalone, V. R., Ustun, A., Allen, F. L., Landau-Ellis, D., Trigiano, R. N., et al. (2003). Quantitative trait loci for agronomic and seed quality traits in an F 2 and F 4:6 soybean population. Euphytica 129, 387–393. doi: 10.1023/A:1022282726117
Chen, T., Huang, L., Wang, M., Huang, Y., Zeng, R., Wang, X., et al. (2020). Ethyl methyl sulfonate-induced mutagenesis and its effects on peanut agronomic, yield and quality traits. Agronomy 10:655. doi: 10.3390/agronomy10050655
Chen, P., Shen, Z., Ming, L., Li, Y., Dan, W., Lou, G., et al. (2018). Genetic basis of variation in rice seed storage protein (albumin, globulin, Prolamin, and Glutelin) content revealed by genome-wide association analysis. Front. Plant Sci. 9:612. doi: 10.3389/fpls.2018.00612
Chung, H. L., Babka, G. L., Graef, P. E., Staswick, D. J., Lee, P. B., Cregan, R. C., et al. (2003). The seed protein, oil, and yield QTL on soybean linkage group I. Crop Sci. 43, 1053–1067. doi: 10.2135/cropsci2003.1053
Cobb, J. N., Juma, R. U., Biswas, P. S., Arbelaez, J. D., Rutkoski, J., Atlin, G., et al. (2019). Enhancing the rate of genetic gain in public-sector plant breeding programs: lessons from the breeder’s equation. Theor. Appl. Genet. 132, 627–645. doi: 10.1007/s00122-019-03317-0
Craciun, A., Jacobs, M., and Vauterin, M. (2000). Arabidopsis loss-of-function mutant in the lysine pathway points out complex regulation mechanisms. FEBS Lett. 487, 234–238. doi: 10.1016/s0014-5793(00)02303-6
Cubero, J. (1981). Origin, taxonomy and domestication. in Lentils, ed. H.G.C Webb Slough, UK: Commonwealth Agricultural Bureau, 15–38.
Danielson, C. E. (1950). An electrophoretic investigation of vicilin and legumin from seeds of peas. Acta Chem. Scand. 4, 762–771. doi: 10.3891/acta.chem.scand.04-0762
Davis, K. R. (1981). Effect of processing on composition and Tetrahymena relative nutritive value on green and yellow peas, lentils and white pea beans. Cereal Chem. 58, 454–460.
Dinkins, R. D., Srinivasa Reddy, M. S., Meurer, C. A., Yan, B., Trick, H., Thibaud-Nissen, F., et al. (2001). Increased sulfur amino acids in soybean plants overexpressing the maize 15 kDa zein protein. In Vitro Cell Dev. Biol. Plant 37, 742–747. doi: 10.1007/s11627-001-0123-x
Dudareva, N., Maeda, H., and Yoo, H. (2011). Prephenate aminotransferase directs plant phenylalanine biosynthesis via arogenate. Nat. Chem. Biol. 7, 19–21. doi: 10.1038/nchembio.485
Dueñas, M., Hernández, T., and Estrella, I. (2002). Phenolic composition of the cotyledon and the seed coat of lentils (Lens culinaris L.). Eur. Food Res. Technol. 215, 478–483. doi: 10.1007/s00217-002-0603-1
Duranti, M. (2006). Grain legume proteins and nutraceutical properties. Fitoterapia 77, 67–82. doi: 10.1016/j.fitote.2005.11.008
Duranti, M., and Gius, C. (1997). Legume seeds: protein content and nutritional value. Field Crop Res. 53, 31–45. doi: 10.1016/s0378-4290(97)00021-x
Erskine, W., Williams, P. C., and Nakkoul, H. (1985). Genetic and environmental variation in the seed size, protein, yield, and cooking quality of lentils. Field Crops Res. 12, 153–161. doi: 10.1016/0378-4290(85)90061-9
Falco, S. C., Guida, T., Locke, M., Mauvais, J., Sanders, C., Ward, R. T., et al. (1995). Transgenic canola and soybean seeds with increased lysine. Biotechnology 13, 577–582. doi: 10.1038/nbt0695-577
FAOSTAT (2021). Available at: https://www.fao.org/faostat/en/#compare (Accessed November 20, 2021).
Ferguson, M. E., Maxted, N., Van Slageren, M., and Robertson, L. D. (2000). A re-assessment of the taxonomy of Lens Mill. (Leguminosae, Papilionoideae, Vicieae). Bot. J. Linn Soc. 133, 41–59. doi: 10.1006/bojl.1999.0319
Fontana, G. S., Santini, L., Caretto, S., Frugis, G., and Mariotti, D. (1993). Genetic transformation in the grain legume Cicer arietinum L. (chickpea). Plant Cell Rep. 12, 194–198. doi: 10.1007/BF00237052
Forde, B. G., and Lea, P. J. (2007). Glutamate in plants: metabolism, regulation, and signalling. J. Exp. Bot. 58, 2339–2358. doi: 10.1093/jxb/erm121
Fürst, P., and Young, V. R. (eds.) (2000). Proteins, Peptides, and Amino Acids in Enteral Nutrition. Vol. 3. Karger Medical and Scientific Publishers.
Galili, G. (2011). The aspartate-family pathway of plants. Plant Signaling Behav. 6, 192–195. doi: 10.4161/psb.6.2.14425
Gaufichon, L., Reisdorf-Cren, M., Rothstein, S. J., Chardon, F., and Suzuki, A. (2010). Biological functions of asparagine synthetase in plants. Plant Sci. 179, 141–153. doi: 10.1016/j.plantsci.2010.04.010
Gaufichon, L., Rothstein, S. J., and Suzuki, A. (2016). Asparagine metabolic pathways in Arabidopsis. Plant Cell Physiol. 57, 675–689. doi: 10.1093/pcp/pcv184
Gautam, N. K., Bhardwaj, R., Yadav, S., Suneja, P., Tripathi, K., and Ram, B. (2018). Identification of lentil (Lens culinaris Medik.) germplasm rich in protein and amino acids for utilization in crop improvement. Ind. J. Genet. 78:9. doi: 10.31742/IJGPB.78.4.9
Ge, L., Yu, J., Wang, H., Luth, D., Bai, G., Wang, K., et al. (2016). Increasing seed size and quality by manipulating BIG SEEDS1 in legume species. Proc. Natl. Acad. Sci. U. S. A. 113, 12414–12419. doi: 10.1073/pnas.1611763113
Gibson, G. R., Hutkins, R., Sanders, M. E., Prescott, S. L., Reimer, R. A., Salminen, S. J., et al. (2017). Expert consensus document: The International Scientific Association for Probiotics and Prebiotics (ISAPP) consensus statement on the definition and scope of prebiotics. Nat. Rev. Gastroenterol. Hepatol. 14, 491–502. doi: 10.1038/nrgastro.2017.75
Goyal, R. K., Vishwakarma, R. K., and Wanjari, O. D. (2009). Optimization of process parameters and mathematical modelling for dehulling of pigeonpea. Int. J. Food Sci. Tech. 44, 36–41. doi: 10.1111/j.1365-2621.2007.01630.x
Grusak, M. A. (2009). “Nutritional and health-beneficial quality” in The Lentil: Botany, Production and Uses. eds. W. Erskine, F. J. Muehlbauer, A. Sarker, and B. Sharma (Cambridge, UK: CAB International), 368–390.
Guo, C., Liu, X., Chen, L., Cai, Y., Yao, W., Yuan, S., et al. (2020). Elevated methionine content in soybean seed by overexpressing maize β-zein protein. Oil Crop Sci. 5, 11–16. doi: 10.1016/j.ocsci.2020.03.004
Han, M., Zhang, C., Suglo, P., Sun, S., Wang, M., and Su, T. (2021). L-aspartate: an essential metabolite for plant growth and stress acclimation. Molecules 26:1887. doi: 10.3390/molecules26071887
Hefnawy, T. H. (2011). Effect of processing methods on nutritional composition and antinutritional factors in lentils (Lens culinaris). Ann. Agric. Sci. 56, 57–61. doi: 10.1016/j.aoas.2011.07.001
Heuzé, V., Tran, G., Sauvant, D., Bastianelli, D., and Lebas, F. (2021). Lentil (Lens culinaris). Feedipedia, a programme by INRAE, CIRAD, AFZ and FAO. 18. Available at: https://www.feedipedia.org/node/284 (Accessed December 15, 2021).
Hinchee, M. A. W., Connor-Ward, D. V., Newell, C. A., McDonnell, R. E., Sato, S. J., Gasser, C. S., et al. (1988). Production of transgenic soybean plants using agrobacterium-mediated DNA transfer. Bio/technology 6, 915–922. doi: 10.1038/nbt0888-915
Howarth, J. R., Roberts, M. A., and Wray, J. L. (1997). Cysteine biosynthesis in higher plants: a new member of the Arabidopsis thaliana serine acetyltransferase small gene-family obtained by functional complementation of an Escherichia coli cysteine auxotroph. Biochim. Biophys. Acta 1350, 123–127. doi: 10.1016/s0167-4781(96)00213-8
Huang, J., Ma, Q., Cai, Z., Xia, Q., Li, S., Jia, J., et al. (2020). Identification and mapping of stable QTLs for seed oil and protein content in soybean [Glycine max (L.) Merr.]. J. Agric. Food Chem. 68, 6448–6460. doi: 10.1021/acs.jafc.0c01271
Iqbal, A., Khalil, I. A., Ateeq, N., and Sayyar Khan, M. (2006). Nutritional quality of important food legumes. Food Chem. 97, 331–335. doi: 10.1016/j.foodchem.2005.05.011
Irzykowska, L., and Wolko, B. (2004). Interval mapping of QTLs controlling yield-related traits and seed protein content in Pisum sativum. J. Appl. Genet. 45, 297–306.
Jasinski, S., Lécureuil, A., Durandet, M., Bernard-Moulin, P., and Guerche, P. (2016). Arabidopsis seed content QTL mapping using high-throughput phenotyping: the assets of near infrared spectroscopy. Front. Plant Sci. 7:1682. doi: 10.3389/fpls.2016.01682
Kahraman, A. (2018). Sustainable food systems: relations amongst nutritional components in chickpea (Cicer arietinum L.). Selcuk J. Agric. Food Sci. 32, 458–461. doi: 10.15316/SJAFS.2018.123
Kavithamani, D., Kalamani, A., Vanniarajan, C., and Uma, D. (2010). Development of new vegetable soybean (Glycine max L. Merill) mutants with high protein and less fibre content. Electron. J. Plant Breed. 1, 1060–1065.
Khazaei, H., Subedi, M., Nickerson, M., Martínez-Villaluenga, C., Frias, J., and Vandenberg, A. (2019). Seed protein of lentils: current status, progress, and food applications. Foods 8:391. doi: 10.3390/foods8090391
Kim, S. W., Mateo, R. D., Yin, Y., and Wu, G. (2007). Functional amino acids and fatty acids for enhancing production performance of sows and piglets. Asian-Australas. J. Anim. Sci. 20, 295–306. doi: 10.5713/ajas.2007.295
Knill, T., Reichelt, M., Paetz, C., Gershenzon, J., and Binder, S. (2009). Arabidopsis thaliana encodes a bacterial-type heterodimeric isopropylmalate isomerase involved in both Leu biosynthesis and the met chain elongation pathway of glucosinolate formation. Plant Mol. Biol. 71, 227–239. doi: 10.1007/s11103-009-9519-5
Kouzarides, T. (2007). Chromatin modifications and their function. Cell 128, 693–705. doi: 10.1016/j.cell.2007.02.005
Kumar, S., Gupta, P., Choukri, H., and Siddique, K. H. M. (2020). “Efficient breeding of pulse crops” in Accelerated Plant Breeding. Vol. 3. eds. S. S. Gosal and S. H. Wani (Cham: Springer International Publishing), 1–30.
Kumar, J., Gupta, D. S., Kumar, S., Gupta, S., and Singh, N. P. (2016a). Current knowledge on genetic biofortification in lentil. J. Agric. Food Chem. 64, 6383–6396. doi: 10.1021/acs.jafc.6b02171
Kumar, S., Rajendran, K., Kumar, J., Hamwieh, A., and Baum, M. (2015). Current knowledge in lentil genomics and its application for crop improvement. Front. Plant Sci. 6:78. doi: 10.3389/fpls.2015.00078
Kumar, H., Singh, A., Jain, N., Kumari, J., Singh, A. M., Singh, D., et al. (2014). Characterization of grain iron and zinc in lentil (Lens culinaris Medikus culinaris) and analysis of their genetic diversity using SSR markers. Aust. J. Crop. Sci. 8, 1005–1012.
Kumar, J., Singh, J., Kanaujia, R., and Gupta, S. (2016b). Protein content in wild and cultivated taxa of lentil (Lens culinaris ssp. culinaris Medikus). Indian J. Genet. Plant Breed. 76:631. doi: 10.5958/0975-6906.2016.00078.X
Lee, M. L., Fung, S., Chung, I., Pailoor, J., Cheah, S. H., and Tan, N. (2014). King cobra (Ophiophagus hannah) venom l-amino acid oxidase induces apoptosis in PC-3 cells and suppresses PC-3 solid tumor growth in a tumor xenograft mouse model. Int. J. Med. Sci. 11, 593–601. doi: 10.7150/ijms.8096
Less, H., and Galili, G. (2009). Coordinations between gene modules control the operation of plant amino acid metabolic networks. BMC Syst. Biol. 3:14. doi: 10.1186/1752-0509-3-14
Li, Y., Reif, J. C., Hong, H., Li, H., Liu, Z., Ma, Y., et al. (2018). Genome-wide association mapping of QTL underlying seed oil and protein contents of a diverse panel of soybean accessions. Plant Sci. 266, 95–101. doi: 10.1016/j.plantsci.2017.04.013
Li, P., Yin, Y., Li, D., Woo Kim, S., and Wu, G. (2007). Amino acids and immune function. Br. J. Nutr. 98, 237–252. doi: 10.1017/S000711450769936X
Liang, H., Yu, Y., Wang, S., Lian, Y., Wang, T., Wei, Y., et al. (2010). QTL mapping of Isoflavone, oil and protein contents in soybean (Glycine max L. Merr.). Agric. Sci. China 9, 1108–1116. doi: 10.1016/s1671-2927(09)60197-8
Liepman, A. H., and Olsen, L. J. (2004). Genomic analysis of aminotransferases in Arabidopsis thaliana. Crit. Rev. Plant Sci. 23, 73–89. doi: 10.1080/07352680490273419
Liu, N., Li, M., Hu, X., Ma, Q., Mu, Y., Tan, Z., et al. (2017). Construction of high-density genetic map and QTL mapping of yield-related and two quality traits in soybean RILs population by RAD-sequencing. BMC Genomics 18:466. doi: 10.1186/s12864-017-3854-8
Lowe, N. M. (2021). The global challenge of hidden hunger: perspectives from the field. Proc. Nutr. Soc. 80, 283–289. doi: 10.1017/S0029665121000902
Majumdar, R., Barchi, B., Turlapati, S. A., Gagne, M., Minocha, R., Long, S., et al. (2016). Glutamate, ornithine, arginine, proline, and polyamine metabolic interactions: the pathway is regulated at the post-transcriptional level. Front. Plant Sci. 7:78. doi: 10.3389/fpls.2016.00078
Maleki, S., and Razavi, S. H. (2021). Pulses' germination and fermentation: two bioprocessing against hypertension by releasing ACE inhibitory peptides. Crit. Rev. Food Sci. Nutr. 61, 2876–2893. doi: 10.1080/10408398.2020.1789551
Mann, G., Diffey, S., Cullis, B., Azanza, F., Martin, D., Kelly, A., et al. (2009). Genetic control of wheat quality: interactions between chromosomal regions determining protein content and composition, dough rheology, and sponge and dough baking properties. Theor. Appl. Genet. 118, 1519–1537. doi: 10.1007/s00122-009-1000-y
McKnight, J. R., Satterfield, M. C., Jobgen, W. S., Smith, S. B., Spencer, T. E., Meininger, C. J., et al. (2010). Beneficial effects of L-arginine on reducing obesity: potential mechanisms and important implications for human health. Amino Acids 39, 349–357. doi: 10.1007/s00726-010-0598-z
Molvig, L., Tabe, L. M., Eggum, B. O., Moore, A. E., Craig, S., Spencer, D., et al. (1997). Enhanced methionine levels and increased nutritive value of seeds of transgenic lupins (Lupinus angustifolius L.) expressing a sunflower seed albumin gene. Proc. Natl. Acad. Sci. U. S. A 94, 8393–839883.
Montamat, F., Maurousset, L., Tegeder, M., Frommer, W., and Delrot, S. (1999). Cloning and expression of amino acid transporters from broad bean. Plant Mol. Biol. 41, 259–268. doi: 10.1023/a:1006321221368
Nishimura, J., Masaki, T., Arakawa, M., Seike, M., and Yoshimatsu, H. (2010). Isoleucine prevents the accumulation of tissue triglycerides and upregulates the expression of PPARalpha and uncoupling protein in diet-induced obese mice. J. Nutr. 140, 496–500. doi: 10.3945/jn.109.108977
Olsen, M. S., and Phillips, R. L. (2009). “Molecular genetic improvement of protein quality in maize,” in Impacts of Agriculture on Human Health And Nutrition. eds. I. Cakmak and R. M. Welch (EOLSS Online Publications), 1–20.
Oommen, A. M., Griffin, J. B., Sarath, G., and Zempleni, J. (2005). Roles for nutrients in epigenetic events. J. Nutr. Biochem. 16, 74–77. doi: 10.1016/j.jnutbio.2004.08.004
Oram, R. N., Shaikh, M. A. Q., Zaman, K. M. S., and Brown, A. H. D. (1987). Isozyme similarity and genetic differences in morphology between hyprosola, a high yielding, high protein mutant of chickpea (Cicer arietinum L.) and its parental cultivar. Environ. Exp. Bot. 27, 455–462. doi: 10.1016/0098-8472(87)90026-8
Osborne, T. B., and Campbell, G. F. (1898). PROTEIDS of the LENTIL.1. J. Am. Chem. Soc. 20, 362–375. doi: 10.1021/ja02067a007
Osborne, T. B., and Mendel, L. B. (1914). Amino-acids in nutrition and growth. J. Biol. Chem. 12, 484–485. doi: 10.1080/07315724.1993.10718340
Parthasarathy, A., Cross, P. J., Dobson, R. C. J., Adams, L. E., Savka, M. A., and Hudson, A. O. (2018). A three-ring circus: metabolism of the three proteogenic aromatic amino acids and their role in the health of plants and animals. Front. Mol. Biosci. 5:29. doi: 10.3389/fmolb.2018.00029
Parthasarathy, A., Savka, M. A., and Hudson, A. O. (2019). The synthesis and role of β-alanine in plants. Front. Plant Sci. 10:921. doi: 10.3389/fpls.2019.00921
Patil, G., Mian, R., Vuong, T., Pantalone, V., Song, Q., Chen, P., et al. (2017). Molecular mapping and genomics of soybean seed protein: a review and perspective for the future. Theor. Appl. Genet. 130, 1975–1991. doi: 10.1007/s00122-017-2955-8
Phua, C. S., Vejayan, J., Ambu, S., Ponnudurai, G., and Gorajana, A. (2012). Purification and antibacterial activities of an l-venom amino acid oxidase from king cobra (Ophiophagus hannah). J. Venomous Anim. Toxins Incl. Trop. Dis. 18, 198–207. doi: 10.1590/S1678-91992012000200010
Podder, R., Glahn, R. P., and Vandenberg, A. (2020). Iron- and zinc-fortified lentil (lens culinaris medik.) demonstrate enhanced and stable iron bioavailability after storage. Front. Nutr. 7:614812. doi: 10.3389/fnut.2020.614812
Qureshi, A., Wani, S., Lone, A., Dar, Z., Wani, S., and Nehvi, F. (2013). “Breeding for quality traits in grain legumes,” in Conventional and non-conventional interventions in crop improvement. 1st Edn. eds. C. Malik, G. Sanghera, and S. Wani (New Delhi: M D Publishers).
Rasheed, N., Maqsood, M. A., Aziz, T., and Jabbar, A. (2020). Characterizing lentil Germplasm for zinc biofortification and high grain output. J. Soil Sci. Plant Nutr. 20, 1336–1349. doi: 10.1007/s42729-020-00216-y
Reddy, N. R., Pierson, M. D., Sathe, S. K., and Salunkhe, D. K. (1985). Dry bean tannins: a review of nutritional implications. J. Am. Oil Chem. Soc. 62, 541–549. doi: 10.1007/BF02542329
Reeds, P. J. (2000). Dispensable and indispensable amino acids for humans. J. Nutr. 130, 1835S–1840S. doi: 10.1093/jn/130.7.1835S
Rees, J. D., Ingle, R. A., and Smith, J. A. C. (2009). Relative contributions of nine genes in the pathway of histidine biosynthesis to the control of free histidine concentrations inArabidopsis thaliana. Plant Biotechnol. J. 7, 499–511. doi: 10.1111/j.1467-7652.2009.00419.x
Rose, W. C., and Cox, G. J. (1924). The relation of arginine and histidine to growth. J. Biol. Chem. 61, 747–773. doi: 10.1016/s0021-9258(18)85123-7
Rose, W. C., Haines, W. J., and Johnson, J. E. (1947). The rôle of the amino acids in human nutrition. J. Biol. Chem. 146, 683–684. doi: 10.1016/s0021-9258(18)44994-0
Rozan, P., Kuo, Y., and Lambein, F. (2001). Amino acids in seeds and seedlings of the genus lens. Phytochemistry 58, 281–289. doi: 10.1016/s0031-9422(01)00200-x
Russell, D. R., Wallace, K. M., Bathe, J. H., Martinell, B. J., and McCabe, D. E. (1993). Stable transformation of Phaseolus vulgaris via electric-discharge mediated particle acceleration. Plant Cell Rep. 12, 165–169. doi: 10.1007/BF00239099
Rutkoski, J. E. (2019). A practical guide to genetic gain. Adv. Agron. 157, 217–249. doi: 10.1016/bs.agron.2019.05.001
Saǧel, Z., Tutluer, M. I., Peşkircioǧlu, H., Kantoǧlu, K. Y., and Kunter, B. (2009). “The improvement of TAEK-sagel chickpea (Cicer arietinum L.) mutant variety in Turkey” in Induced Plant Mutations in the Genomics Era. ed. Q. Y. Shu (Rome: FAO), 319–321.
Saint-Clair, P. M. (1972). Responses of lens Esculenta Moench to controlled environmental factors. Wangeningen, Netherlands: Wangeningen University
Sales, M. P., Gerhardt, I. R., Grossi-de-Sá, M. F., and Xavier-Filho, J. (2000). Do legume storage proteins play a role in defending seeds against Bruchids? Plant Physiol. 124, 515–522. doi: 10.1104/pp.124.2.515
Sarrobert, C., Thibaud, M. C., Contard-David, P., Gineste, S., Bechtold, N., Robaglia, C., et al. (2000). Identification of an Arabidopsis thaliana mutant accumulating threonine resulting from mutation in a new dihydrodipicolinate synthase gene. Plant J. 24, 357–368. doi: 10.1046/j.1365-313x.2000.00884.x
Sayeed, S., and Njaa, L. R. (1985). Effect of a Bangladeshi home-cooking procedure on the amino acid content, trypsin inhibitor activity and in vitro digestibility of some legume seeds. Qualitas plantarum. Plant Foods Human Nutr. 354, 35, 379–388. doi: 10.1007/BF01091783
Schroeder, H. E., Schotz, A. H., Wardley-Richardson, T., Spencer, D., and Higgins, T. (1993). Transformation and regeneration of two cultivars of pea (Pisum sativum L.). Plant Physiol. 101, 751–757. 10.1104/pp.101.3.751
Scippa, G. S., Rocco, M., Ialicicco, M., Trupiano, D., Viscosi, V., Di Michele, M., et al. (2010). The proteome of lentil (Lens culinaris Medik.) seeds: discriminating between landraces. Electrophoresis 31, 497–506. doi: 10.1002/elps.200900459
Sebolt, A. M., Shoemaker, R. I., and Diers, R. W. (2000). Analysis of a quantitative trait locus allele from wild soybean that increases seed protein concentration in soybean. Crop Sci. 40, 1438–1444. doi: 10.2135/cropsci2000.4051438x
Semba, R. D. (2016). The rise and fall of protein malnutrition in global health. Ann. Nutr. Metab. 69, 79–88. doi: 10.1159/000449175
Sen Gupta, D., Thavarajah, D., Knutson, P., Thavarajah, P., McGee, R. J., Coyne, C. J., et al. (2013). Lentils (Lens culinaris L.), a rich source of Folates. J. Agric.Food Chem. 61, 7794–7799. doi: 10.1021/jf401891p
Shekib, L. A. H., Zoueil, M. E., Youssef, M. M., and Mohamed, M. S. (1986). Amino acid composition and In vitro digestibility of lentil and rice proteins and their mixture (Koshary). Food Chem. 20, 61–67. doi: 10.1016/0308-8146(86)90167-6
Shi, L., Mu, K., Arntfield, S. D., and Nickerson, M. T. (2017). Changes in levels of enzyme inhibitors during soaking and cooking for pulses available in Canada. J. Food Sci. Technol. 54, 1014–1022. doi: 10.1007/s13197-017-2519-6
Siddhuraju, P., Osoniyi, O., Makkar, H. P. S., and Becker, K. (2002). Effect of soaking and ionising radiation on various antinutritional factors of seeds from different species of an unconventional legume, Sesbania and a common legume, green gram (Vigna radiata). Food Chem. 79, 273–281. doi: 10.1016/S0308-8146(02)00140-1
Singh, B. K., and Shaner, D. L. (1995). Biosynthesis of branched chain amino acids: from test tube to field. Plant Cell 7, 935–944. doi: 10.1105/tpc.7.7.935
Stoddard, F. L., Marshall, D. R., and Ali, S. M. (1993). Variability in grain protein concentration of peas and lentils grown in Australia. Aust. J. Agric. Res. 44:1415. doi: 10.1071/AR9931415
Sulieman, M. A., Amro, B. H., Gamaa, A. O., Mohamed, M. E. T., Elhadi, A. I. E. K., Abdullahi, H. E. T., et al. (2008). Changes in total protein digestibility, fractions content and structure during cooking of lentil cultivars. Pak. J. Nutr. 7, 801–805. doi: 10.3923/pjn.2008.801.805
Tahir, M., Lindeboom, N., Båga, M., Vandenberg, A., and Chibbar, R. (2011). Composition and correlation between major seed constituents in selected lentil (Lens culinaris. Medik) genotypes. Can. J. Plant Sci. 91, 825–835. doi: 10.4141/cjps2011-010
Tan, Q., Grennan, A. K., Pélissier, H. C., Rentsch, D., and Tegeder, M. (2008). Characterization and expression of French bean amino acid transporter PvAAP1. Plant Sci. 174, 348–356. doi: 10.1016/j.plantsci.2007.12.008
Tar’an, B., Warkentin, T., Somers, D. J., Miranda, D., Vandenberg, A., Blade, S., et al. (2004). Identification of quantitative trait loci for grain yield, seed protein concentration and maturity in field pea (Pisum sativum L.). Euphytica 136, 297–306. doi: 10.1023/B:EUPH.0000032721.03075.a0
Tayeh, N., Aubert, G., Pilet-Nayel, M., Lejeune-Hénaut, I., Warkentin, T. D., and Burstin, J. (2015). Genomic tools in pea breeding programs: status and perspectives. Front. Plant Sci. 6:1037. doi: 10.3389/fpls.2015.01037
Tegeder, M., Tan, Q., Grennan, A. K., and Patrick, J. W. (2007). Amino acid transporter expression and localisation studies in pea (Pisum sativum). Funct. Plant Biol. 34, 1019–1028. doi: 10.1071/FP07107
Thavarajah, D., Thavarajah, P., Sarker, A., Materne, M., Vandemark, G., Shrestha, R., et al. (2011). A global survey of effects of genotype and environment on selenium concentration in lentils (Lens culinaris L.): implications for nutritional fortification strategies. Food Chem. 125, 72–76. doi: 10.1016/j.foodchem.2010.08.038
Thavarajah, P., Thavarajah, D., and Vandenberg, A. (2009). Low phytic acid lentils (Lens culinaris L.): a potential solution for increased micronutrient bioavailability. J. Agric. Food Chem. 57, 9044–9049. doi: 10.1021/jf901636p
Tripathi, K., Gore, P. G., Pandey, A., Bhardwaj, R., Singh, N., Chawla, G., et al. (2019). Seed morphology, quality traits and imbibition behaviour study of atypical lentil (Lens culinaris Medik.) from Rajasthan, India. Genet. Resour. Crop. Evol. 66, 697–706. doi: 10.1007/s10722-019-00745-1
Tzin, V., and Galili, G. (2010). The biosynthetic pathways for Shikimate and aromatic amino acids in Arabidopsis thaliana. Arabidopsis Book 8, e0132. doi: 10.1199/tab.0132
Upadhyaya, H. D., Bajaj, D., Narnoliya, L., Das, S., Kumar, V., Gowda, C. L. L., et al. (2016). Genome-wide scans for delineation of candidate genes regulating seed-protein content in chickpea. Front. Plant Sci. 7:302. doi: 10.3389/fpls.2016.00302
Van, K., and Mchale, L. K. (2017). Meta-analyses of QTLs associated with protein and oil contents and compositions in soybean [Glycine max (L.) Merr.] seed. IJMS 18:1180. doi: 10.3390/ijms18061180
van Vliet, D., Derks, T. G. J., van Rijn, M., de Groot, M. J., MacDonald, A., Heiner-Fokkema, M. R., et al. (2014). Single amino acid supplementation in aminoacidopathies: a systematic review. Orphanet J. Rare Dis. 9:7. doi: 10.1186/1750-1172-9-7
Vauterin, M., Frankard, V., and Jacobs, M. (1999). The Arabidopsis thaliana dhdps gene encoding dihydrodipicolinate synthase, key enzyme of lysine biosynthesis, is expressed in a cell-specific manner. Plant Mol. Biol. 39, 695–708. doi: 10.1023/a:1006132428623
Vauterin, M., and Jacobs, M. (1994). Isolation of a poplar and an Arabidopsis thaliana dihydrodipicolinate synthase cDNA clone. Plant Mol. Biol. 25, 545–550. doi: 10.1007/bf00043882
Vidal-Valverde, C., Frias, J., Estrella, I., Gorospe, M. J., Ruiz, R., and Bacon, J. (1994). Effect of processing on some antinutritional factors of lentils. J. Agric. Food Chem. 42, 2291–2295. doi: 10.1021/jf00046a039
Wang, J., Chen, L., Li, P., Li, X., Zhou, H., Wang, F., et al. (2008). Gene expression is altered in piglet small intestine by weaning and dietary glutamine supplementation. J. Nutr. 138, 1025–1032. doi: 10.1093/jn/138.6.1025
Wang, N., and Daun, J. K. (2006). Effects of variety and crude protein content on nutrients and antinutrients in lentils (Lens culinaris). Food Chem. 95, 493–502. doi: 10.1016/j.foodchem.2005.02.001
Wirtz, M., Droux, M., and Hell, R. (2004). O-acetylserine (thiol) lyase: an enigmatic enzyme of plant cysteine biosynthesis revisited in Arabidopsis thaliana. J. Exp. Bot. 55, 1785–1798. doi: 10.1093/jxb/erh201
Wong, M. M. L., Gujaria-Verma, N., Ramsay, L., Yuan, H. Y., Caron, C., Diapari, M., et al. (2015). Classification and characterization of species within the genus lens using genotyping-by-sequencing (GBS). PLoS One 10:e0122025. doi: 10.1371/journal.pone.0122025
World Health Organization. (2019). Non communicable diseases. Available at: https://www.who.int/gho/ncd/mortality_morbidity/en/ (Accessed December 05, 2021).
Wu, G. (2010). Functional amino acids in growth, reproduction, and health. Adv. Nutr. 1, 31–37. doi: 10.3945/an.110.1008
Xing, A., and Last, R. L. (2017). A regulatory hierarchy of the Arabidopsis branched-chain amino acid metabolic network. Plant Cell 29, 1480–1499. doi: 10.1105/tpc.17.00186
Xu, Y., Li, P., Zou, C., Lu, Y., Xie, C., Zhang, X., et al. (2017). Enhancing genetic gain in the era of molecular breeding. J. Exp. Bot. 68, 2641–2666. doi: 10.1093/jxb/erx135
Yathaputanon, C., Bunyarut, J., Kumsueb, B., Malipan, A., and Srisombun, S.. (2009). Protein Content in High-Protein Soybean Mutants in Thailand. Food and Agriculture Organization of the United Nations (FAO) : Rome: FAO.
Zaccardelli, M., Lupo, F., Piergiovanni, A. R., Laghetti, G., Sonnante, G., Daminati, M. G., et al. (2012). Characterization of Italian lentil (Lens culinaris Medik.) germplasm by agronomic traits, biochemical and molecular markers genet. Resour. Crop Evol. 59, 727–738. doi: 10.1007/s10722-011-9714-5
Zhang, X., Wang, W., Guo, N., Zhang, Y., Bu, Y., Zhao, J., et al. (2018). Combining QTL-seq and linkage mapping to fine map a wild soybean allele characteristic of greater plant height. BMC Genomics 19, 226. doi: 10.1186/s12864-018-4582-4
Keywords: Lentil (Lens culinaris L.), protein, biofortification, amino acids, protein quality, food secuity
Citation: Salaria S, Boatwright JL, Thavarajah P, Kumar S and Thavarajah D (2022) Protein Biofortification in Lentils (Lens culinaris Medik.) Toward Human Health. Front. Plant Sci. 13:869713. doi: 10.3389/fpls.2022.869713
Received: 04 February 2022; Accepted: 14 March 2022;
Published: 05 April 2022.
Edited by:
Eric Von Wettberg, University of Vermont, United StatesReviewed by:
Melike Bakir, Erciyes University, TurkeyCopyright © 2022 Salaria, Boatwright, Thavarajah, Kumar and Thavarajah. This is an open-access article distributed under the terms of the Creative Commons Attribution License (CC BY). The use, distribution or reproduction in other forums is permitted, provided the original author(s) and the copyright owner(s) are credited and that the original publication in this journal is cited, in accordance with accepted academic practice. No use, distribution or reproduction is permitted which does not comply with these terms.
*Correspondence: Dil Thavarajah, ZHRoYXZhckBjbGVtc29uLmVkdQ==
Disclaimer: All claims expressed in this article are solely those of the authors and do not necessarily represent those of their affiliated organizations, or those of the publisher, the editors and the reviewers. Any product that may be evaluated in this article or claim that may be made by its manufacturer is not guaranteed or endorsed by the publisher.
Research integrity at Frontiers
Learn more about the work of our research integrity team to safeguard the quality of each article we publish.