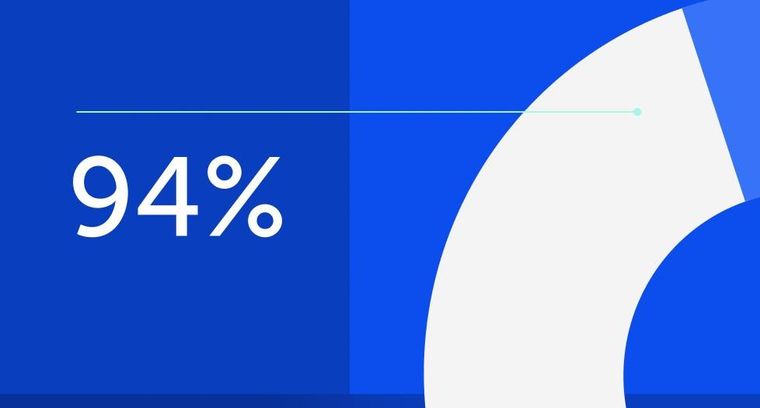
94% of researchers rate our articles as excellent or good
Learn more about the work of our research integrity team to safeguard the quality of each article we publish.
Find out more
REVIEW article
Front. Plant Sci., 10 June 2022
Sec. Plant Abiotic Stress
Volume 13 - 2022 | https://doi.org/10.3389/fpls.2022.867531
This article is part of the Research TopicPlants for Future Climate: Responses and Adaptations to Combined, Multifactorial, and Sequential StressesView all 8 articles
Proline is a proteinogenic amino acid synthesized from glutamate and ornithine. Pyrroline-5-carboxylate synthetase and pyrroline-5-carboxylate reductase are the two key enzymes involved in proline synthesis from glutamate. On the other hand, ornithine-δ-aminotransferase converts ornithine to pyrroline 5-carboxylate (P5C), an intermediate in the synthesis of proline as well as glutamate. Both proline dehydrogenase and P5C dehydrogenase convert proline back to glutamate. Proline accumulation is widespread in response to environmental challenges such as high temperatures, and it is known to defend plants against unpropitious situations promoting plant growth and flowering. While proline accumulation is positively correlated with heat stress tolerance in some crops, it has detrimental consequences in others. Although it has been established that proline is a key osmolyte, its exact physiological function during heat stress and plant ontogeny remains unknown. Emerging evidence pointed out its role as an overriding molecule in alleviating high temperature stress (HTS) by quenching singlet oxygen and superoxide radicals. Proline cycle acts as a shuttle and the redox couple (NAD+/NADH, NADP+/NADPH) appears to be highly crucial for energy transfer among different cellular compartments during plant development, exposure to HTS conditions and also during the recovery of stress. In this review, the progress made in recent years regarding its involvement in heat stress tolerance is highlighted.
Climate change is on the horizon, and its implications will have a significant footprint on crop productivity and consequently food and nutritional security. Crop plants’ physiological and metabolic activities are radically altered not just by rising day temperatures, but also due to elevated night temperatures. All of the important food crops, such as grains and legumes are prone to high temperature stress (HTS) resulting in an overall reduction in yields (Lobell and Field, 2007; Battisti and Naylor, 2009; Lobell et al., 2011; Xu et al., 2020). HTS negatively affects spikelet fertility, panicle and grain numbers, grain filling, seed size, and grain quality (Peng et al., 2004; Bahuguna et al., 2017; Cheabu et al., 2018; Impa et al., 2019; Dawood et al., 2020). In addition to the yield formation factors altered by HTS, various physiological changes such as decreased photosynthetic efficiency, and formation of reactive oxygen species (ROS) leading to oxidative stress particularly in the chloroplasts occurs with a consequence of membrane damage, and onset of leaf senescence (Fatma et al., 2021). To generate climate resilient crops, it is necessary to understand the underlying physiological and biochemical mechanisms associated with HTS.
Touted as a multifunctional amino acid, proline plays vital functions in plant abiotic stress tolerance, including elevated temperature stress (Kishor et al., 1995, 2005, 2015; de Ronde et al., 2000; Kishor and Sreenivasulu, 2014; Mattioli et al., 2020; Maria et al., 2021). De novo accumulation of proline was noticed in barley, radish (Chu et al., 1974), leaves of tomato (Rivero et al., 2004), tobacco (Cvikrova et al., 2012), and heat-tolerant varieties of lettuce and wheat in response to heat stress (Han et al., 2013; Djukic et al., 2021). Accumulation of proline has also been found under heat stress in wide array of taxa (Szabados and Savouré, 2010; Pospisilova et al., 2011; Kaur and Asthir, 2015; Harsh et al., 2016; Per et al., 2017). Pyrroline-5-carboxylate synthetase (P5CS) and pyrroline-5-carboxylate reductase (P5CR) are the first two enzymes involved in proline biosynthesis catalyzing glutamate to proline via pyrroline-5-carboxylate (P5C; Figure 1). P5CS overexpression in transgenic sugarcane resulted in proline buildup under water deficit conditions, which protected chlorophyll and photosystem II (PSII; Molinari et al., 2007). Ahammed et al. (2020) demonstrated that transcription factor SlWRKY81 represses SlP5CS1 transcription and drought stress tolerance in tomatoes. On the other hand, SlWRKY81-silenced transgenics showed increased proline biosynthesis and drought stress tolerance.
Figure 1. Proline is synthesized from glutamate in cytosol by P5CS1/P5CS2 enzymes and these reactions take place in cytosol (indicated in yellow shade). Oxidation of proline takes place by ProDH and P5CDH enzymes in mitochondria (indicated in green shade). While proline synthesis consumes NAD(P)H and ATP in cytosol, its degradation releases FADH2 and NADH in mitochondria. It is not clearly known what transports arginine and ornithine from cytoplasm into mitochondria. But, it is predicted that arginine and ornithine, the two polyamines are transported into mitochondria from cytosol and back by basic amino acid carriers (BAC2). While arginine gets converted to ornithine, ornithine is catalyzed by ornithine δ-amino transferase (OAT) to pyrroline-5-carboxylate (P5C), an overriding intermediate in proline cycle. Note that the release of NAD(P)H is connected to pentose phosphate pathway (PPP pathway) in mitochondria. Conversion of P5C to proline and proline to P5C changes the NADP+/NADPH ratio in the cells. This leads to the modulation of phosphoribosyl pyrophosphate (PRPP) synthesis besides nucleotide biosynthesis via the PPP pathway.
Proline in transgenics has been found to act as a component of antioxidative defense systems, and not for osmotic adjustment (Molinari et al., 2007). Congruently, P5CR in soybean increased the tolerance to heat in Arabidopsis thaliana (de Ronde et al., 2004). Existing evidence points out that proline might play a role during osmotic adjustment under heat stress as well as in scavenging the ROS (Kishor et al., 1995; Szabados and Savouré, 2010; Anjaneyulu et al., 2014; Reddy et al., 2015; Signorelli et al., 2015). Experiments carried out by Signorelli et al. (2016) unequivocally demonstrate that proline is not a direct scavenger of peroxynitrite, superoxide, nitric oxide (NO), and nitrogen dioxide (NO2). Contrarily, Hua et al. (2001) and Rizhsky et al. (2004) found no proline accumulation under HTS. Likewise, proline content has been reported to decrease in wheat seedlings during heat stress (Song et al., 2005), inferring that proline accumulation under heat stress is not ubiquitous. In support of this hypothesis, HTS does not trigger the accumulation of proline in A. thaliana, and its synthesis literally showed inimical effect (Lv et al., 2011). In contrast, exogenously applied 1 to 5 μM proline protected the Arabidopsis plants exposed to 50°C for 10 min (Chua et al., 2020). Foliar application of 5 and 10 mM proline helped in heat stress tolerance in Capsicum frutescens exposed to 40°C/32°C day/night for 30-days and also recovery (Akram et al., 2021). Further, 30 mM foliar application of proline resulted in the alleviation of heat stress in rice seedlings exposed to 34 to 36°C (Table 1). Improved antioxidant defense is the hallmark in all these species implying that proline alleviates heat stress through antioxidant defense (Hanif et al., 2021). A list of plants exposed to heat stress and their alleviation by proline and antioxidant defense is shown in Table 1. However, the amount of proline accumulated and the ability to combat heat stress is affected by the nitrogen availability (Rivero et al., 2004). With these contradictory reports, the feasibility of designing crop plants targeting proline biosynthetic pathway genes for heat stress tolerance is still being debated (Verslues and Sharma, 2010; Bhaskara et al., 2015). This difference in proline buildup could be attributed to (a) overexpression of genes involved in proline biosynthesis not only from glutamate but also from ornithine, and (b) proline homeostasis achieved through activation of the proline catabolic pathways. HTS suppressed the expression of proline dehydrogenase (OsProDH) in rice (Guo et al., 2020). It appears that proline accumulation quenches the ROS, thereby imparting thermal tolerance. Impaired insulin/IGF1 signaling extends lifespan in worms by boosting proline degradation to induce a transient ROS signal (Zarse et al., 2012), tumor suppression and cell survival (Liu et al., 2006; Phang, 2019), and hypersensitive response in plants (Miller et al., 2009). ProDH generates ROS for signaling, but the threshold levels of catabolic activities that switch survival pathways to cellular apoptosis remain unknown and appear to be an emerging issue. Besides ROS, other players associated with proline metabolic signaling look ambiguous, and a matter in question. To understand the role of biosynthetic and catabolic pathway genes/proteins during heat stress, it is imperative to have a comprehensive idea about their regulatory networks. In this review article, we discuss the role of proline biosynthetic and catabolic pathways to heat response and how the conversion of glutamate to proline and proline to glutamate shuttles the reducing power. Further, we emphasize the importance of proline in radical scavenging and redox potential thereby conferring heat stress tolerance.
The regulatory roles of proline and proline cycle in heat stress have been emphasized by Iqbal et al. (2019), Zheng et al. (2021), and Lehr et al. (2022). Through diverse molecular methods, key genes involved in proline biosynthesis and degradation (proline cycle; Figure 1) have been identified in plants (Deuschle et al., 2001). The enzymes P5CS1 and P5CS2 (EC 2.7.2.11, two isoforms) utilize either NADH or NADPH (Chen et al., 2006; Forlani et al., 2017) and ATP to produce P5C and their activities are subjected to feedback inhibition by proline, other amino acids and cofactors (Sabbioni et al., 2021). Millimolar levels of proline have been found inhibitory to rice P5CS2 enzyme. Similarly, analogs of proline like azetidine-2-carboxylate, pipecolate, hydroxyproline, phosphonoproline displayed the ability to bind to the enzyme rice P5CS2 and inhibit the activity aside cofactors such as NAD+ and ADP (Sabbioni et al., 2021). These results infer that structural analogs of proline inhibit the P5CS2 enzyme like that of proline feed-back inhibition. Such data on other enzymes like P5CS1, P5CR, ProDH1, ProDH2, and P5C dehydrogenase (P5CDH) are lacking, but the data are critical for better understanding. The findings of Sabbioni et al. (2021) substantiate the influence of redox status of the cell and availability of nitrogen/cofactors like NAD+ on proline production.
While P5CS1 is required for the synthesis and accumulation of proline under stress treatments, P5CS2 is indispensable for embryo and seedling development (Funck et al., 2020). Evidence has also been presented that both P5CS1 and P5CS2 participate in the synthesis of proline (Funck et al., 2020). Initially, it was predicted that P5CS1 protein is localized in plastids and P5CS2 in cytoplasm (Szekely et al., 2008). Subsequent experiments with fluorescence imaging technique showed that both P5CS1 and P5CS2 are located in cytosol in Arabidopsis, and plastids do not contribute to the synthesis of proline (Funck et al., 2020). Thus, subcellular localization of P5CS1 is still enigmatic in plants. Possibly, its localization may depend upon the growth or stress conditions that the plants are undergoing. In line with this, p5cs1 mutants of A. thaliana accumulate ROS due to upregulation of genes implicated in ROS but not the overexpressed lines (Szekely et al., 2008; Shinde et al., 2016). It has been shown that alternative splicing of AtP5CS1 results in natural variation in the content of proline and climate adaptation (Kesari et al., 2012) implaying the importance of P5CS during stress. With regard to redox under abiotic stress conditions, Shinde et al. (2016) have noticed an interconnection between proline and lipid metabolism which may help in buffering cellular redox status. On the other hand, P5CR (EC 1.5.1.2, four isoforms in all including bacterial sources) uses either NADH or NADPH as the electron donor with contrasting affinities and maximum reaction rates (Forlani et al., 2017). P5CR is mostly localized in cytosol in A. thaliana (Funck et al., 2012). Activity of P5CS was inhibited by cations like Na+, Mg2+, and Ca2+, anions such as Cl– promoted it (Sabbioni et al., 2021). Interestingly, in A. thaliana, stimulation or inhibition by chloride ions and feedback regulation by proline depends on whether NADPH or NADH acts as co-substrate (Giberti et al., 2014). This infers that activities of P5CS and P5CR lower the concentrations of NADPH/NADP+ ratio in the cytosol (Liang et al., 2013; Zheng et al., 2021, Lehr et al., 2022). While proline suppressed the activity of only NADH-dependent enzymes, salt stimulated the NADPH-dependent reaction (Giberti et al., 2014). Further, the catabolic pathway enzymes like ProDH (EC 1.5.5.2) and pyrroline-5-carboxylate dehydrogenase (P5CDH, EC 1.2.1.88) are also dependent on FAD and NAD(P) for their activities, respectively. While ProDH is localized on the matrix side of the mitochondrial inner membrane (Cabassa-Hourton et al., 2016), P5CDH (single copy in Arabidopsis) has been found in the mitochondrial inner membrane in maize.
The enzymes ProDH and P5CDH were found to interact with drought and freezing responsive gene 1 (DFR1) protein physically. DFR1 protein mediates the inhibition of proline degradation and modulates drought and freezing tolerance (Ren et al., 2018). P5CDH is also crucial to degrade the toxic effects of proline in plants or its intermediate glutamic semialdehyde/P5C to glutamate releasing the reducing power in mitochondria. In plants, proline-P5C cycle generates ROS which can trigger cell apoptosis and prevent pathogenesis. Such proline/P5C toxicity could be because of an overflow of electrons in the mitochondrial electron transport chain (mETC). The increase in ROS was caused by inhibiting P5CDH or yeast mutants lacking this enzyme, demonstrating that P5C functions as a stress response regulator and that its levels must be strictly maintained (Deuschle et al., 2001; Zheng et al., 2021). Thus, the redox state, as well as chloride and proline concentrations in the cytosol, govern all enzymatic activities of the proline cycle in a complicated way. Since proline metabolism is linked to cellular compartments like mitochondria and energetics, it takes part in NAD(P)+/NAD(P)H homeostasis during the growth of plants and when exposed to the stress conditions or relieved from it. A look at the expression of the genes involved in proline cycle and the transporters (eight identified in Arabidopsis) infers that the genes are up- and down-regulated by HTS (Figure 2). Surprisingly, more than P5CS, P5CR gene was upregulated under HTS indicating its importance during short- and long-term exposure to temperature stress. Ornithineδ-aminotransferase (OAT) also was expressed but under long-term exposure, while its expression was not altered under short-term treatments.
Figure 2. Expression of proline biosynthetic pathway genes (anabolic and catabolic) both from glutamate and ornithine and proline transporters (ProT) under diverse temperature regimes in Arabidopsis thaliana.
Once the stress is relieved, proline is transported to mitochondria either through proline symporter or a proline/glutamate antiporter to produce energy [FADH2 and NAD(P)H] by ProDH, and P5CDH, respectively, and release glutamate (Di Martino et al., 2006). But the genes that encode these transporters have not yet been identified in plants. It appears now that glutamate released into mitochondria is transported back to cytosol via a novel glutamate transporter “a bout de souffle” (BOU) and uncoupling proteins1 and 2 which also act as transporters of aspartate and dicarboxylates (Monne et al., 2018; Porcelli et al., 2018). Shuttling of proline and glutamate and also the reducing power between the cellular compartments like cytosol and mitochondria is vital for maintaining proper ratio of NAD(P): NAD(P)H in the cellular system. In contrast, ProDH was consistent in its expression especially at 3 + 3-h-long temperature stress exposure, but not P5CDH barring a moderate expression at 3 h/4 h treatments (Figure 2). Though transcript levels of OsProDH were high in root and leaf blade, heat stress reduced the transcript levels of OsProDH (Guo et al., 2020). Expression of the ProDH1 and ProDH2 genes has been found upregulated during plant senescence (Launay et al., 2019). This may help to reduce the toxic levels of proline/P5C on one hand and on the other, aid to generate energy (Launay et al., 2019). These results distinctly demonstrate that proline oxidation fuels mitochondrial respiration during senescence which is essential for maintaining NADP+ and NADPH ratio in the cells. Proline cycle is therefore indispensable in the cells for maintaining proper cellular redox. Taking cognizance of these studies, breeding strategies must be developed to enhance HTS tolerance by manipulating proline metabolism.
Though proline biosynthesis occurs in cytosol and chloroplasts (Szabados and Savouré, 2010), proline is detected both in xylem and phloem (Lehmann et al., 2010), inferring its transport in different tissues. While Girousse et al. (1996) noticed water stress-stimulated transport of proline to long distances via phloem in alfalfa, Mattioli et al. (2020) demonstrated its accumulation in pollen under various abiotic stress conditions, so, its transport to pollen grains is essential for the pollen fertility, thereby limiting the seed loss. Proline porters also act as porters of betaine and γ-aminobutyric acid in A. thaliana (Breitkreuz et al., 1999). In Arabidopsis thaliana, the expression of proline transporter 3 (AtProT3) was steady under both short-term and long-term exposure to temperature stress compared to AtProT1 and AtProT2 (Figure 2). However, the functions of other ProTs under heat stress conditions are unclear. These studies clearly point out that along with the proline cycle genes, transporters are also vital for stress tolerance, under normal as well as environmental stimuli. There is also a debate about whether proline buildup is more important to confer short-term stress tolerance or attaining proline homeostasis through the activation of a catabolic pathway to meet the energy and redox potential in driving plant development under prolonged stress (Kishor and Sreenivasulu, 2014).
Proline is synthesized not only from glutamate but also from ornithine (derived from arginine) by OAT (Chalecka et al., 2021). Shin et al. (2016) have presented evidence that ornithine, rather than glutamate pathway is the major route for the synthesis of proline both during growth resumption and HTS in Prunus persica. Production of proline from ornithine involves P5C, the intermediate in the cycle. But, Funck et al. (2008) and Winter et al. (2015) showed that P5C derived from ornithine is utilized for generation of glutamate via P5CDH rather than proline via P5CR. This may perhaps depend on cellular status of proline or glutamate levels and also stress conditions. If the levels of P5C are higher, which are toxic to the cells, it may get converted to glutamate. So, P5C is at a central point to deal with the situation depending on cellular conditions (Anwar et al., 2018; Chalecka et al., 2021). It has also been pointed out that proline regulates the function of mitochondria influencing the death of cells when biotrophic and necrotrophic pathogens invade and in cancer state (Rizzi et al., 2016; Phang, 2019). Given the adage that the OAT is an important stress associated protein involved in proline/glutamate biosynthesis, it has been sought to identify the interacting partners of the protein (Figures 3A,B), which are largely co-expressed with a few of the experimentally determined genes (indicated in yellow background in Supplementary Table 1). We explored important nodes/hubs from the interaction using stress as a topological algorithm from Cytohubba app (Chin et al., 2014) of cytoscape. Highly ranked proteins have been identified during mining (Figures 3A,B) from stress using the Edge Percolated Component, Maximum Neighborhood Component, Betweenness and Closeness Centralities, Density of Maximum Neighborhood Component, Maximal Clique Centrality, and centralities based on shortest paths, such as Bottleneck, and radiality. Network hub indicates that P5CS1 is also one of the key interaction partners of OAT under stress. An attempt was made to unravel the connections between OAT protein and lncRNA targets under stress, but apparently none of the bonafide genuine possibilities were uncovered. This systems biology prediction is in agreement with the supported hypothesis that with an increased OAT, there is an alteration in stress-regulated P5CS1 levels.
Figure 3. (A) Protein interaction networks generated by genemania.org taking OAT and its interacting partners. (B) Cytohubba prediction of OAT with ranked genes. Brighter the node, larger is the adherence of accumulated stress protein.
High temperature stress causes many changes in plant growth, development, leaf senescence, discoloration of leaves (Vollenweider and Gunthardt-Goerg, 2005), degradation of chlorophyll a and b (Jahan et al., 2021), and decline in quantum efficiency. Functional aspects of chloroplasts during programmed cell death (PCD) are mostly obscure. Zavafer et al. (2020) noticed that chloroplasts integrity is collapsed which leads to PCD promoted by long chain bases or ceramides under HTS. In response to HTS, metabolic reprogramming such as breakdown of chlorophyll, production of ROS and alterations in carbon metabolism occur. Such an alteration in metabolic programming is perhaps essential during stress acclimation. Chloroplasts play a crucial role in inducing the expression of nuclear heat-response genes under HTS response (Hu et al., 2020). Light intensity inhibits chlorophyll synthesis especially if ABA levels are low or poor like in nced3nced5 mutants (Huang et al., 2019). High light and HTS produce ROS in the thylakoid which play vital roles as signal transducers. ROS generated during light and HTS provide cells with vital information on the current status of abiotic stress (Foyer and Noctor, 2016; Foyer et al., 2017). Heat stress causes reduced leaf nitrogen concentrations, protein damage, oxidative stress, and associated membrane damage, limiting plant growth and productivity (Wahid et al., 2007; Fahad et al., 2017). Temperature stress lowers chlorophyll biosynthesis due to decreased chlorophyll biosynthetic pathway enzymes such as 5-aminolevulinate dehydratase (Dutta et al., 2009), which could be linked to oxidative damage (Guo et al., 2006; Mohanty et al., 2006). Proline is associated with the protection of thylakoid membranes against free radical-induced photodamage (Alia et al., 1997). Interestingly, reduced ratio between chlorophyll to carotenoid was recorded in tomato and sugarcane plants that are heat tolerant (Camejo et al., 2005; Huang et al., 2019) inferring that pigment (chlorophyll to carotenoid) ratio is implicated in temperature stress tolerance. PSII is highly temperature sensitive and its activity is either partially or totally impaired depending upon the duration and intensity of heat stress (Camejo et al., 2005; Huang et al., 2019). High day and night temperatures perturb the photosynthetic activity in rice cultivars (Fahad et al., 2016). Glycine max transgenic lines containing P5CR gene (sense and antisense) were used to study the effect of heat stress. While sense plants reveal elevated proline alongside high NADP+ levels with no symptoms of temperature stress, antisense plants showed lower levels of NADP+ with severe symptoms (de Ronde et al., 2004). These results corroborate what has been noticed in the heat map (Figure 2). NADP+ concentrations reached normal levels faster in sense plants during rewarming from stress in comparison with antisense plants. Furthermore, after photoinhibition or repeated heating in all plants, the oxygen evolving complex, D1 and D2 proteins dissociate, disrupting electron transfer to the acceptor molecule PSII and reducing RuBP regeneration (Sharkova, 2001; Wise et al., 2004; Toth et al., 2005). Thus electron transport is the functional limitation of photosynthesis at higher temperatures. Plants cope better with heat stress, where proline supplies electrons to PSII (Moustakas et al., 2011; Oukarroum et al., 2012). This type of electron transport in PSII keeps NADPH levels normal and protects the PSII from additional damage (Oukarroum et al., 2012). When plants are relieved from stress, proline is catabolized releasing high concentrations of NADP+ which would be used for accepting the electrons in PSII which may ameliorate the effect of uncoupling of the oxygen evolving complex (de Ronde et al., 2004). Thus, proline plays a key role in protecting the PSII and other events related to photosynthetic machinery and its activity under HTS.
Interestingly, heat-stressed A. thaliana plants that were pre-treated with Nostoc muscorum displayed lower PCD in root hairs in comparison with untreated seedlings. By suppressing PCD but not necrosis, cyanobacteria (N. muscorum)-derived proline enhanced the heat stress tolerance in A. thaliana root hairs (Chua et al., 2020). Also, exogenous supply of proline mimicked comparable PCD suppression levels like that of N. muscorum (Chua et al., 2020). Further, proline transporter mutants like lht1, aap1, and atprot1-1:atprot2-3:atprot3-2 were used to find out if proline is playing any active role during heat stress. Suppression of PCD under heat stress in lht1 and aap1 mutants was reduced markedly when 5 μM levels of proline were supplied exogenously. When the three mutants were pre-treated with cyanobacteria, PCD levels were comparatively enhanced (Chua et al., 2020). Thus, these experiments clearly reveal that Nostoc-derived proline protects the plants by interfering with PCD activity. Nevertheless, such mechanisms need to be studied in depth to understand the implication of proline in preventing PCD of plants under HTS.
Under normal conditions, equilibrium between the production of ROS and antioxidant defense system is maintained, which is perturbed under abiotic stress conditions including HTS. HTS leads to the high accumulation of ROS and oxidative stress in plants. But the production of ROS and their detoxification by both enzymatic and non-enzymatic machinery must be maintained in such adverse environmental conditions (Hasanuzzaman et al., 2020), though our understanding about ROS signaling is limited. Ectopic overexpression of Stipa P5CS gene (StP5CS) in A. thaliana resulted in higher survival rates of plants under drought stress with less membrane damage and superior antioxidant machinery (Yang et al., 2021). HTS elevated the expression of P5CS in addition to heat shock protein (HSP), and manganese superoxide dismutase 1 (MSD1) in rice (Nahar et al., 2022). Their data display that the responses of plants to salt, heat and a combination of salt + heat are unique, but complex with many molecular network modules. Clearly, mitigation of salt and heat stresses were observed with improved antioxidant enzyme activities and elevated expression of P5CS gene and subsequent proline accumulation. Foyer et al. (2017) has proposed that oxidative stress must be viewed as a signaling mechanism rather than damage to the cells.
Reactive oxygen species such as hydroxyl radical, hydrogen peroxide, superoxide, and singlet oxygen are produced due to environmental conditions including heat stress as pointed out. ROS can denature proteins, lipids and many macromolecules and inactivate photosynthetic machinery. But, enzymatic and non-enzymatic (ascorbate, a-tocopherol, glutathione, etc.) machinery exists to remove the ROS from the system. Again, the putative role of proline as an antioxidative molecule has sparked debate. Proline is thought to be a ROS or hydroxyl radical quencher (Matysik et al., 2002; Signorelli et al., 2014, 2015). It is known that proline reduces or eliminates the levels of hydroxyl radical and hydrogen peroxide in vivo in non-transgenic tobacco and improves the antioxidative enzymatic activities in transgenic Sorghum bicolor plants under stress (Banu et al., 2010; Reddy et al., 2015) and also increases glutathione under drought and heat stresses in G. max (Kocsy et al., 2005). The initial evidence points out that proline cannot totally quench or scavenge singlet oxygen, and does not interact with superoxide radical, nitric oxide, peroxynitrite, and nitrogen dioxide (Signorelli et al., 2013, 2016; Signorelli, 2016). Rehman et al. (2021) used a variety of techniques, including electron paramagnetic resonance spin trapping with 2,2,6,6-tetramethyl-4-piperidone (TEMPD), fluorescence probing with singlet oxygen sensor green (SOSG), and oxygen uptake in isolated thylakoids, to demonstrate that proline quenches both singlet oxygen and superoxide radical in vitro via an electron transfer reaction. Their experiments demonstrate that singlet oxygen-scavenging capacity of proline reaches up to two thirds that of α-tocopherol and significantly superior or the same that of ascorbate (Rehman et al., 2021). Further, Aswani et al. (2019) have shown that proline plays a physiological role in singlet oxygen detoxification in the leaves of Pisum sativum exposed to methyl viologen that generates large amounts of singlet oxygen under light conditions. Proline makes physiologically meaningful contributions to ROS (singlet oxygen) quenching, and may operate as a supplement to other non-enzymatic ROS quenchers in stressful settings, according to these investigations.
It has been demonstrated that P5C-proline cycles operative in different cellular compartments are associated with signaling events and modulation of intracellular redox potential (Hare and Cress, 1997; Miller et al., 2009). Redox homeostasis is essential during photosynthesis especially under the conditions of climate change to promote plant development (Scheibe et al., 2005). Proline metabolism is compartmentalized mostly in chloroplasts, mitochondria and cytoplasm implying that reducing equivalents are concentrated in these compartments (Lunn, 2006). Regulation of energy in the form of ATP, and reducing power (NADH/NADPH), its homeostasis under heat stress requires proper channelization and utilization wherever necessary (Foyer and Noctor, 2020). Dark CO2 fixation is affected under heat stress since stomata are closed and chlorophyll molecules are degraded leading to reduced generation of reducing power in chloroplasts. Since carbohydrate supply is limited under heat stress, concomitant decrease in ATP and NADPH generation is generally noticed in mitochondria. Therefore, very tight spatiotemporal regulation and supply of energy at different cellular compartments is necessary under heat stress. While NAD+ is synthesized in the cytoplasm, it must be translocated into chloroplasts and mitochondria via NAD+ carrier proteins NDT1 and NDT2 localized in plastid and mitochondrial membranes (Palmieri et al., 2009). NDT1, the mitochondrial transporter, appears to play a crucial role in cellular NAD+ homeostasis in A. thaliana (de Souza Chaves et al., 2019). However, the redox couples (NAD+/NADH and NADP+/NADPH) produced by calmodulin-dependent NAD+ kinase (Gakiere et al., 2018; Dell’Aglio et al., 2019) are not permeable to organellar membranes and must be transported across subcellular compartments by shuttle systems or metabolic valves. Under abiotic stress conditions, the glycerol-3-phosphate, malate-aspartate shuttle for NADH pools, and malate-oxaloacetate shuttle for NADPH pools transport reducing equivalents from subcellular compartments into the cytoplasm and back, maintaining redox and energy homeostasis. As part of proline cycle, P5CS and P5CR convert glutamate to proline using NAD(P)H, as an energy source; while ProDH and P5CDH in the mitochondria accelerate the conversion back to glutamate, lowering both FAD+ and NAD+ molecules (Liang et al., 2013). This cycle thus involves NAD(P)+/NAD(P)H between cytoplasm and mitochondria during plant development and also during abiotic stress including heat stress. Arabidopsis p5cs1 mutants exhibit sensitivity to salt stress, accumulate ROS with implications in redox metabolism at subcellular compartments like chloroplasts and mitochondria (Szekely et al., 2008; Shinde et al., 2016). Ruszkowski et al. (2015) and Shinde et al. (2016) noticed that proline metabolism helps in maintaining cellular redox under stress conditions. Proline and ascorbate pathways have been found to act synchronously to maintain cellular redox homeostasis in tomato (Lopez-Delacalle et al., 2021; Table 1). Interestingly, it has been found that NADP+ suppresses the P5CR expression, but not NADPH-dependent reactions (Giberti et al., 2014). Further, NADH-dependent P5CR activity is reduced if proline is accumulated in excess. Thus, proline cycle helps in the production of FADH2 and NADH which might impact the electron transport in mitochondria alongside the production of ROS. Huang et al. (2013) also reported the discovery of succinate dehydrogenase (SDH) assembly factor 2 (SDHAF2), a protein that co-expresses with SDH1-1 and is required for the insertion of FAD+ into SDH component or mitochondrial complex II, as well as proper root elongation in A. thaliana. Further, it has been shown that proline biosynthesis might act as a redox vent even in mammals (Schworer et al., 2020).
Under stress conditions, proline accumulation assists in plant growth. But application of proline under normal conditions inhibits plant growth (Han et al., 2021). If proline is supplied externally under normal conditions, degradation of excess proline induces mitochondrial ROS due to electron overflow in mETC which might contribute to the proline toxicity and inhibition of plant growth. A mitochondrial truncated matrix protein (SSR1) with a tetratricopeptide repeat domain has been shown by Han et al. (2021) to be involved in maintaining the function of mETC in proline hypersensitive phenotype of A. thaliana. When the ssr1-1 mutant was given proline, accumulation of ROS was dramatically increased due to the increased activity of ProDH, resulting in proline breakdown and triggering of apoptosis (White et al., 2007; Pandhare et al., 2009). Han et al. (2021) pointed out that treating plants with proline under normal conditions might lead to higher mitochondrial ROS, lower ATP content, decreased mETC complex I and II. These results imply that SSR1 is associated in maintaining mETC at optimum levels to mitigate proline toxicity under normal conditions. Because ProDH binds to the coenzyme Q, superoxide radicals may not be formed during proline oxidation by ProDH at complex II, as they are produced at complex III (Goncalves et al., 2014; Hancock et al., 2016). Furthermore, polymorphisms in mitochondrial DNA were linked to two genes that encode NADH dehydrogenase subunits. These findings show that proline accumulation and cellular redox are carefully regulated, with proline breakdown being the most important factor in stress tolerance (Atkin and Macherel, 2009; Sharma et al., 2011). Aside from providing energy in the form of FADH2 and NADPH, proline degradation impacts oxidative stress resistance. Zhang et al. (2015) characterized oxidative stress resistance of putA (contains both ProDH and P5CDH domains) mutant strains of E. coli. These experiments revealed that putA mutants are sensitive to oxidative stress compared to the wild-type strain. Thus, proline serves as an important molecule in oxidative stress resistance.
Proline degradation is equally essential for supplying energy under long term stress and when the plants are relieved from stress. Proline is synthesized in chloroplasts/cytoplasm, but transported through proline porters to the root and shoot tips where it supplies energy by oxidation in mitochondria. During senescence, degradation of Calvin cycle enzymes occurs, therefore, NADPH levels are reduced in the senescing leaves. The accumulated leaf proline activates ProDH leading to higher activity of ProDH1 and ProDH2 under senescing conditions (Launay et al., 2019; Dellero et al., 2020). Thus, oxidation of proline during heat stress and also during stress release is an important additional source of energy besides replenishment of NADP+/NADPH ratio in the cells. Besides ProDH, P5CDH also contributes to the alleviation of oxidative stress. Mutants of p5cdh accumulate ROS when proline was supplied exogenously (Deuschle et al., 2004; Miller et al., 2009). The results reveal that P5CDH and the dynamics proline cycle are important for maintaining ROS homeostasis.
Thioredoxin (Trx) is a ubiquitous thiol-disulfide reductase and the master regulator protein of the tricarboxylic cycle in plant mitochondria, and located both in mitochondria and cytosol. Trx regulates SDH, fumerase and ATP-citrate lyase by modulating thiol redox status, and thus the carbon flux. The NADPH produced by dehydrogenases including P5CDH can be utilized in glutathione reductase for protection against oxidative stress and by thioredoxin reductase in the regulation of metabolic pathways (Moller and Rasmusson, 1998). In other words, proline catabolism plays a role since Trx makes use of NADPH through NADPH-thioredoxin reductase enzyme (NTR; Geigenberger et al., 2017). In comparison to wild-type plants, trxo1 mutant and ntra, ntrb double mutants amass less proline but more glutamate and malate (Daloso et al., 2015; Geigenberger et al., 2017). Malate serves as a source of NADH/NADPH in the TCA cycle under stress conditions. Accumulation of proline was curtailed in the mutant trx1 under drought stress conditions (Verslues et al., 2014). Trxs not only transmit the light signal from chloroplasts to mitochondria, but also moderate the enzymes of the proline cycle. Importantly, when chloroplasts are inactive during night time, proline degradation may release energy (FADH2 and NADPH) and redox power to the cells. Therefore, proline degradation and the energy released thereof is imperative unequivocally once the plants are relieved from stress. Plants accumulate 56-times more amounts of proline in flowers than the leaves (Schwacke et al., 1999; Kishor and Sreenivasulu, 2014). Proline also accumulates significantly in high concentrations in developing microspores from local synthesis and accounts for 70% of the total free amino acids. Proline has been found essential for pollen fertility and improved yield stability under salt stress in A. thaliana (Mattioli et al., 2018, 2020). Further, tomato proline transporter LeProT1 is expressed in the germinating pollen tube and supplies energy during the growth of the pollen tube (Mattioli et al., 2018).
High temperature stress activates the accumulation of proline and polyamines (Chakraborty and Tongden, 2005; Cvikrova et al., 2012). Anabolism and catabolism of polyamines have been noticed in plant leaves exposed to heat stress (Walter, 2003). Proline and polyamines play a role in osmotic adjustment as well as in scavenging ROS (Bouchereau et al., 1999). The effect of heat stress on the accumulation of polyamines and proline in transgenic tobacco plants (overexpressing P5CSF129A gene with no feedback regulation of P5CS enzyme) that overproduce proline in lower, and upper leaves, and roots was recorded (Cvikrova et al., 2012). Genes that encode proline biosynthetic pathway enzymes confer salt stress tolerance in Panicum virgatum in cooperation with polyamines metabolism (Guan et al., 2020). Proline accumulated after 6-h of heat stress treatment in the lower leaves. After 2-h of exposure to 40°C, wild-type tobacco plants accumulated more proline than transgenics, but after a 2-h lag period, transgenics accumulated putrescine, spermidine, norspermidine, and spermine, with matching increases in enzyme activity (Cvikrova et al., 2012). During the initial heat stress, polyamine oxidase was elevated in the roots of wild-type and transgenic plants, but it was unexpectedly reduced in the leaves of transgenics. Decrease in the activity of ornithine decarboxylase and increase in the diamine oxidase in the leaves and roots were recorded in transgenics (Cvikrova et al., 2012). In addition, the results obtained by Cheng et al. (2009) in tomato infer that both spermine and spermidine play indispensable roles in heat stress tolerance. High levels of proline in transgenics also appear to play a positive effect on heat stress tolerance, since degradation products of proline aid in the biosynthesis of polyamines in the early stage of exposure to heat (Cvikrova et al., 2012). Changes in the accumulation of proline and its metabolism have been found regulated depending on the age of the leaf in pea plants exposed to metal stress, independent of ABA signals (Zdunek-Zastocka et al., 2021). Such an accumulation of proline in the leaves may help in osmotic adjustment during stress.
Stress responses in plants are highly dynamic and often involve a complex cross-talk between gene expressions, and a unique reprogramming at the metabolic, and phenotypic levels. It is known that nitrogen source increases proline quantity, however, the mechanistic explanation is mostly imprecise. Water and heat stresses trigger sucrose synthases (SUS1 and SUS4), glucan, water dikinase 2 (GWD2) as well as P5CS1. Gurrieri et al. (2020) have shown that wild-type plants and loss-of-function mutants show no differences in transitory starch and cell wall carbohydrates and in the total amino acid content under water deficit conditions. But water-soluble sugars and proline contents decline in mutants in comparison with wild-type plants. Their results indicate that GWD2 strengthens the involvement of SUS1 concerning osmotic stress and higher contribution of soluble sugars than proline in osmotic adjustment under drought stress (Gurrieri et al., 2020). Also, a putative interaction between proline and soluble sugars has been noticed (Gurrieri et al., 2020). However, the nature of the interaction between soluble sugars and proline is yet to be ascertained. Proline concentration in phloem exudates, uptake of nitrogen and absorbed nitrogen from the soil has been determined under water deficit conditions in Trifolium repens (Lee et al., 2009). Under drought stress, proline content in phloem exudates has been found enhanced, with a concomitant decrease in nitrate reductase activity in roots. The results prove that proline transport to roots via phloem was enhanced due to drought stress conditions which are governing the uptake and assimilation of newly absorbed nitrogen (Lee et al., 2009). However, if such a link exists or not between high day and high night temperature stresses, proline transport to phloem and nitrogen nutrition is largely elusive. Exogenous supply of proline reduces the levels of malonaldehyde and H2O2 under HTS in tomato, it improves the water use efficiency, fruit quality attributes like acidity and total soluble solids, and final yields (Tonhati et al., 2020). It emerges therefore, that proline alleviates HTS by improving the oxidative stress damage. Lopez-Delacalle et al. (2021) noticed that proline and ascorbate pathways act highly in a synchronous fashion to maintain cellular redox homeostasis under salt and heat combination stresses in tomato. They identified the transcription factor families like the basic leucine zipper domain (bZIP), zinc finger cysteine-2/histidine-2 (C2H2) and trihelix as modulators of the up-regulated genes when salt and heat stresses were given in combination. Rivero et al. (2004) studied the effect of different nitrogen sources (NO3– or NH4+) on heat stress tolerance due to the accumulation of proline in tomato leaves. They noticed that proline has accumulated due to increased P5CS and OAT expressions with a concomitant decrease in ProDH and P5CDH. In NH4+ fed-plants, at 35°C, proline and biomass of plants was higher in comparison with NO3– fed-plants. Tomato plants fed with NH4+ as a source of nitrogen unveil higher temperature tolerance (35°C) than the plants that were fed with NO3– (Rivero et al., 2004). Such a tolerance was correlated to the higher accumulation of proline when NH4+ was fed to the plants than nitrate (Rivero et al., 2004). The experiments point out that the source of nitrogen is crucial for proline accumulation under high temperature and in imparting tolerance to HTS. In line with this, Dellero et al. (2020) noticed downregulation of three P5CS1 genes in source leaves with reduced commitment of nitrogen source toward proline biosynthesis in Brassica napus. Contrary to this, ProDH genes were upregulated by carbon starvation (dark-induced senescence) compared to early senescing leaves. It is evident that besides nitrogen status, dark to light transition and stress response jointly modulate the differential expression of P5CS and ProDH genes associated with proline synthesis and degradation.
Besides genetic engineering technologies, editing of one or more genes through multiplex-multigene CRISPR/Cas9 system is an encouraging approach for generating crop plants tolerant to abiotic stresses including HTS. While P5CS and P5CR are important positive regulators in proline biosynthesis, ProDH is the rate limiting step in its degradation. Both P5CS and P5CR have not been subjected to editing, but are promising candidate genes. Transcript levels of OsProDH were higher in roots and leaf blade, and HTS repressed the expression of ProDH in rice (Guo et al., 2020). While overexpression of OsProDH (single copy gene in rice) decreased proline quantity, a knockout mutant (created using CRISPR/Cas9) increased the proline content (Guo et al., 2020). CRISPR-edited lines displayed better resistance in comparison with wild-type rice plants. These results imply that OsProDH negatively modulates heat stress tolerance in rice seedlings by regulating proline catabolism (Guo et al., 2020). Knockout mutants accumulate less H2O2 than the overexpressed lines. It appears therefore, that proline accumulation quenches the ROS, thereby imparts thermal tolerance. Paralogous genes must be kept in mind which may get targeted if not protected carefully (Saikia et al., 2020). Care must therefore be exercised while selecting target genes through pre-CRISPR analysis for multiplex-multigene editing while making efforts to manipulate proline metabolism. The gene editing procedures including TALENS/ZFNs or CRISPR/Cas9-based multiplexed editing have shown great promise in deletion of candidate motifs associated with stress tolerant varieties in tomato (Tran et al., 2021). To develop climate resilient heat tolerance lines, undertaking precision genome editing of proline biosynthesis and degradation targets is necessary to attain redox, energy homeostasis and to scavenge ROS. Although Tran et al. (2021) have attempted to understand this in the manipulation of hybrid proline-rich protein 1 (HyPRP1) domains, engineering such domains for coping up with climate changes needs to be further heralded.
Despite being a proteinogenic amino acid, proline has a wide range of activities in plants, as an osmoprotectant for osmotic adjustment under conditions of salt, drought and temperature stresses. Recent evidence surmises that proline reduces the production of ROS, through quenching singlet oxygen and superoxide radicals in thylakoids. It is widely acknowledged that proving this evidence in whole plants is challenging. As a result, much more research is needed to show that proline is a good quencher matching to the properties of non-enzymatic antioxidant compounds. Proline cycle acts as a shuttle to transport redox couples from mitochondria to cytoplasm and back. Both synthesis and degradation of proline appear to be highly critical during plant growth and stress conditions. The enzymes of the biosynthetic pathway have not been completely characterized under diverse abiotic stress conditions and more studies on protein-protein interactions, membrane transporters are necessary. Enzymes involved in proline catabolism interact with Trx, but other interacting partners need to be uncovered to understand stress response. Recent findings filled the gaps in our understanding of proline cycle and production of ROS and their homeostasis in attaining heat tolerance. Proline catabolism has been implicated in cancer biology in humans and abiotic stress tolerance in plants. Therefore, understanding the biochemical mechanisms of how proline improves the stress tolerance in plants and also human health is vital for us. We need to target to increase proline under short-term stress to quench ROS and to attain proline homeostasis under prolonged stress and then yield stability through a balanced redox potential. But it appears that proline metabolism can only be an adjunctive target for generating heat stress tolerant plants.
PBKK and NS conceived the idea and wrote a major part of the review article. PBKK, PS, PR, and NS analyzed the data, interpreted, and wrote the manuscript. NS has generated the heat map. PS has analyzed the bioinformatics data, created the figures, and interpreted the results. All authors have critically analyzed the manuscript, read and approved it.
This work was supported by the financial support from the German Federal Ministry for Economic Cooperation and Development (BMZ) commissioned and administered through the Deutsche Gesellschaft für Internationale Zusammenarbeit (GIZ) Fund for International Agricultural Research (FIA), Grant No: 81206686 and the Bill and Melinda Gates Foundation AGGRi Alliance (Grant No. OPP1194925), and Stress Tolerant Rice for Africa and South Asia (STRASA) Phase III (Grant No. OPP1088843).
The authors declare that the research was conducted in the absence of any commercial or financial relationships that could be construed as a potential conflict of interest.
All claims expressed in this article are solely those of the authors and do not necessarily represent those of their affiliated organizations, or those of the publisher, the editors and the reviewers. Any product that may be evaluated in this article, or claim that may be made by its manufacturer, is not guaranteed or endorsed by the publisher.
Figure 1 is revised by Rhowell N. Tiozon, to ensure good visuals and smooth reading. We gratefully acknowledge his support and help.
The Supplementary Material for this article can be found online at: https://www.frontiersin.org/articles/10.3389/fpls.2022.867531/full#supplementary-material
Ahammed, G. L., Li, X., Wan, H., Zhou, G., and Cheng, Y. (2020). SlWRKY81 reduces drought tolerance by attenuating proline biosynthesis in tomato. Scientia Horticult. 270:109444. doi: 10.1016/j.scienta.2020.109444
Ahmed, J. U., and Hasan, M. A. (2011). Evaluation of seedling proline content of wheat genotypes in relation to heat tolerance. Bangladesh J. Bot. 40, 17–22. doi: 10.3329/bjb.v40i1.7991
Akram, S., Ayyub, C. M., Shahzad, M., and Shahzad, A. (2021). Role of proline in mitigating the deleterious effects of heat stress in chillies. Serbian J. Agricult. Sci. 70, 28–35. doi: 10.2478/contagri-2021-0006
Alia, Saradhi, P. P., and Mohanty, P. (1997). Involvement of proline in protecting thylakoid membranes against free radical-induced photodamage. J. Photochem. Photobiol. B Biol. 38, 253–257. doi: 10.1016/S1011-1344(96)07470-2
Anjaneyulu, E., Reddy, P. S., Sunita, M. S., Kishor, P. B. K., and Balaji, M. (2014). Salt tolerance and activity of antioxidative enzymes of transgenic finger millet overexpressing a vacuolar H+-pyrophosphatase gene (SbVPPase) from Sorghum bicolor. J. Plant Physiol. 171, 789–798. doi: 10.1016/j.jplph.2014.02.001
Anwar, A., She, M., Wang, K., Riaz, B., and Ye, X. (2018). Biological roles of ornithine aminotransferase (OAT) in plant stress tolerance: present progress and future perspectives. Int. J. Mol. Sci. 19:3681. doi: 10.3390/ijms19113681
Aswani, V., Rajsheel, P., Bapatla, R. B., Sunil, B., and Raghavendra, A. S. (2019). Oxidative stress induced in chloroplasts or mitochondria promotes proline accumulation in leaves of pea (Pisum sativum): another example of chloroplast-mitochondria interactions. Protoplasma 256, 449–457. doi: 10.1007/s00709-018-1306-1
Atkin, O. K., and Macherel, D. (2009). The crucial role of plant mitochondria in orchestrating drought tolerance. Ann. Bot. 103, 581–597. doi: 10.1093/aob/mcn094
Bahuguna, R. N., Celymar, A. S., Shi, W., and Jagadish, S. V. K. (2017). Post-flowering night respiration and altered sink activity account for high night temperature-induced grain yield and quality loss in rice (Oryza sativa L.). Physiol. Plant 159, 59–73. doi: 10.1111/ppl.12485
Banu, A. N., Hoque, A., Watanabe-Sugimoto, M., Islam, M. M., Uraji, M., Matsuoka, K., et al. (2010). Proline and glycinebetaine ameliorated NaCl stress via scavenging of hydrogen peroxide and methylglyoxal but not superoxide or nitric oxide in tobacco cultured cells. Biosci. Biotechnol. Biochem. 74, 2043–2049. doi: 10.1271/bbb.100334
Battisti, D. S., and Naylor, R. L. (2009). Historical warnings of future food insecurity with unprecedented seasonal heat. Science 323, 240–244. doi: 10.1126/science.1164363
Bhaskara, G. B., Yang, T. H., and Verslues, P. E. (2015). Dynamic proline metabolism: importance and regulation in water limited environments. Front. Plant Sci. 6:484. doi: 10.3389/fpls.2015.00484
Bouchereau, A., Aziz, A., Lahrer, F., and Martin-Tanguy, J. (1999). Polyamines and environmental challenges: recent development. Plant Sci. 140, 103–125. doi: 10.1016/S0168-9452(98)00218-0
Breitkreuz, K. E., Shelp, B. J., Fischer, W. N., Schwacke, R., and Rentsch, D. (1999). Identification and characterization of GABA, proline and quaternary ammonium compound transporters from Arabidopsis thaliana. FEBS Lett. 450, 280–284. doi: 10.1016/S0014-5793(99)00516-5
Cabassa-Hourton, C., Schertl, P., and Bordenave-Jacquemin, M. (2016). Proteomic and functional analysis of proline dehydrogenase 1 link proline catabolism to mitochondrial electron transport in Arabidopsis thaliana. Biochem. J. 473, 2623–2634. doi: 10.1042/BCJ20160314
Camejo, D., Rodriguez, P., Morales, M. A., Dell’amico, J. M., Torrecillas, A., and Alarcon, J. J. (2005). High temperature effects on photosynthetic activity of two tomato cultivars with different heat susceptibility. J. Plant Physiol. 162, 281–289. doi: 10.1016/j.jplph.2004.07.014
Chakraborty, U., and Tongden, C. (2005). Evaluation of heat acclimation and salicylic acid treatments as potent inducers of thermotolerance in Cicer arietinum L. Curr. Sci. 89, 384–389.
Chalecka, M., Kazberuk, A., Palka, J., and Surazynski, A. (2021). P5C as an interface of proline interconvertible amino acids and its role in regulation of cell survival and apoptosis. Int. J. Mol. Sci. 22:11763. doi: 10.3390/ijms222111763
Cheabu, S., Moung-Ngam, P., Arikit, S., Vanavichit, A., and Malumpong, C. (2018). Effects of heat stress at vegetative and reproductive stages on spikelet fertility. Rice Sci. 25, 218–226. doi: 10.1016/j.rsci.2018.06.005
Chen, M., Cao, J., Zheng, C., and Liu, Q. (2006). Directed evolution of an artificial bifunctional enzyme, γ-glutamyl kinase/γ-glutamyl phosphate reductase, for improved osmotic tolerance of Escherichia coli transformants. FEMS Microbiol. Lett. 263, 41–47. doi: 10.1111/j.1574-6968.2006.00397.x
Cheng, L., Zou, Y. J., Ding, S. L., Zhang, J. J., Yu, X. L., Cao, J. S., et al. (2009). Polyamine accumulation in transgenic tomato enhances the tolerance to high temperature stress. J. Integr. Plant Biol. 51, 489–499. doi: 10.1111/j.1744-7909.2009.00816.x
Chin, C. H., Chen, S. H., Wu, H. H., Ho, C. W., Ko, M. T., and Lin, C. Y. (2014). cytoHubba: identifying hub objects and subnetworks from complex interactome. BMC Syst. Biol. 8(Suppl. 4):S11. doi: 10.1186/1752-0509-8-S4-S11
Chu, T. M., Aspinall, D., and Paleg, L. G. (1974). Stress metabolism. VI. Temperature stress and the accumulation of proline in barley and radish. Aust. J. Plant Physiol. 1, 87–97. doi: 10.1071/pp9740087
Chua, A., Sherwood, O. L., Fitzhenry, L., Ng, C. K. Y., McCabe, P. F., and Daly, C. T. (2020). Cyanobacteria-derived proline increases stress tolerance in Arabidopsis thaliana root hairs by suppressing programmed cell death. Front. Plant Sci. 11:490075. doi: 10.3389/fpls.2020.490075
Cvikrova, M., Gemperlova, L., Dobra, J., Martincova, O., Prasil, I. T., Gubis, J., et al. (2012). Effect of heat stress on polyamine metabolism in proline-over-producing tobacco plants. Plant Sci. 182, 49–58. doi: 10.1016/j.plantsci.2011.01.016
Daloso, D. M., Müller, K., Obata, T., Florian, A., Tohge, T., and Bottcher, A. (2015). Thioredoxin, a master regulator of the tricarboxylic acid cycle in plant mitochondria. Proc. Nat. Acad. Sci. U.S.A. 112, E1392–E1400. doi: 10.1073/pnas.1424840112
Dawood, M. F. A., Moursi, Y. S., Amro, A., Baenziger, P. S., and Sallam, A. (2020). Investigation of heat-induced changes in the grain yield and grains metabolites, with molecular insights on the candidate genes in barley. Agronomy 10:1730. doi: 10.3390/agronomy10111730
de Ronde, J. A., Cress, W. A., Krüger, G. H. J., Strasser, R. J., and Van Staden, J. (2004). Photosynthetic response of transgenic soybean plants, containing an Arabidopsis P5CR gene, during heat and drought stress. J. Plant Physiol. 161, 1211–1224. doi: 10.1016/j.jplph.2004.01.014
de Ronde, J. A., Cress, W. A., and Van Staden, J. (2000). Interaction of osmotic and temperature stress on transgenic soybean. South Afr. J. Bot. 67, 655–660. doi: 10.1016/s0254-6299(15)31196-0
de Souza Chaves, I., Feitosa-Araújo, E., Florian, A., Medeiros, D. B., da Fonseca-Pereira, P., Charton, L., et al. (2019). The mitochondrial NAD+ transporter (NDT1) plays important roles in cellular NAD+ homeostasis in Arabidopsis thaliana. Plant J. 100, 487–504. doi: 10.1111/tpj.14452
Dell’Aglio, E., Giustini, C., Kraut, A., Couté, Y., Costa, A., Decros, G., et al. (2019). Identification of the Arabidopsis calmodulin-dependent NAD+ kinase that sustains the elicitor induced oxidative burst. Plant Physiol. 181, 1449–1458. doi: 10.1104/pp.19.00912
Dellero, Y., Clouet, V., Marnet, N., Pellizzaro, A., Dechaumet, S., Niogret, M. F., et al. (2020). Leaf status and environmental signals jointly regulate proline metabolism in winter oilseed rape. J. Exp. Bot. 71, 2098–2111. doi: 10.1093/jxb/erz538
Deuschle, K., Funck, D., Forlani, G., Stransky, H., Biehl, A., Leister, D., et al. (2004). The role of delta1-pyrroline-5-carboxylate dehydrogenase in proline degradation. Plant Cell 16, 3413–3425. doi: 10.1105/tpc.104.023622
Deuschle, K., Funck, D., Hellmann, H., Daschner, K., Binder, S., and Frommer, W. B. (2001). A nuclear gene encoding mitochondrial Δ1-pyrroline-5-carboxylate dehydrogenase and its potential role in protection from proline toxicity. Plant J. 27, 345–356. doi: 10.1046/j.1365-313x.2001.01101.x
Di Martino, C., Pizzuto, R., Pallotta, M. L., De Santis, A., and Passarella, S. (2006). Mitochondrial transport in proline catabolism in plants: the existence of two separate translocators in mitochondria isolated from durum wheat seedlings. Planta 223, 1123–1133. doi: 10.1007/s00425-005-0166-z
Djukic, N., Markovic, S. M., Mastilovic, J. S., and Simovic, P. (2021). Differences in proline accumulation between wheat varieties in response to heat stress. Botanica Serbica 45, 61–69. doi: 10.2298/BOTSERB2101061D
Dutta, S., Mohanty, S., and Tripathy, B. C. (2009). Role of temperature stress on chloroplast biogenesis and protein import in pea. Plant Physiol. 150, 1050–1061. doi: 10.1104/pp.109.137265
Fahad, S., Bajwa, A. A., Nazir, U., Anjum, S. A., Farooq, A., Zohaib, A., et al. (2017). Crop production under drought and heat stress: plant responses and management options. Front. Plant Sci. 8:1147. doi: 10.3389/fpls.2017.01147
Fahad, S., Hussain, S., Saud, S., Hassan, S., Tanveer, M., Ihsan, M. Z., et al. (2016). A combined application of biochar and phosphorus alleviates heat-induced adversities on physiological, agronomical and quality attributes of rice. Plant Physiol. Biochem. 103, 191–198. doi: 10.1016/j.plaphy.2016.03.001
Fatma, M., Iqbal, N., Sehar, Z., Alyemeni, M. N., Kaushik, P., Khan, N. A., et al. (2021). Methyl jasmonate protects the PS II system by maintaining the stability of chloroplast D1 protein and accelerating enzymatic antioxidants in heat-stressed wheat plants. Antioxidants 10:1216. doi: 10.3390/antiox10081216
Forlani, G., Nocek, B., Chakravarthy, S., and Joachimiak, A. (2017). Functional characterization of four putative δ1-pyrroline-5-carboxylate reductases from Bacillus subtilis. Front. Microbiol. 8:1442. doi: 10.3389/fmicb.2017.01442
Foyer, C. H., and Noctor, G. (2016). Stress-triggered redox signalling: what’s in pROSpect? Plant Cell Environ. 39, 951–964. doi: 10.1111/pce.12621
Foyer, C. H., and Noctor, G. (2020). Redox homeostasis and signaling in a higher-CO2 world. Annu. Rev. Plant Biol. 71, 157–182. doi: 10.1146/annurev-arplant-050718-095955
Foyer, C. H., Ruban, A. V., and Noctor, G. (2017). Viewing oxidative stress through the lens of oxidative signalling rather than damage. Biochem. J. 474, 877–883. doi: 10.1042/BCJ20160814
Funck, D., Baumgarten, L., Stift, M., von Wiren, N., and Schönemann, L. (2020). Differential contribution of P5CS isoforms to stress tolerance in Arabidopsis. Front. Plant Sci. 11:565134. doi: 10.3389/fpls.2020.565134
Funck, D., Stadelhofer, B., and Koch, W. (2008). Ornithine-delta-aminotransferase is essential for arginine catabolism but not for proline biosynthesis. BMC Plant Biol. 8:40. doi: 10.1186/1471-2229-8-40
Funck, D., Winter, G., Baumgarten, L., and Forlani, G. (2012). Requirement of proline synthesis during Arabidopsis reproductive development. BMC Plant Biol. 12:191. doi: 10.1186/1471-2229-12-191
Gakiere, B., Hao, J., de Bont, L., Pétriacq, P., Nunes-Nesi, A., and Fernie, A. R. (2018). NAD+ biosynthesis and signaling in plants. Crit. Rev. Plant Sci. 37, 259–307. doi: 10.1080/07352689.2018.1505591
Geigenberger, P., Thormählen, I., Daloso, D. M., and Fernie, A. R. (2017). The unprecedented versatility of the plant- thioredoxin system. Trends Plant Sci. 22, 249–262. doi: 10.1016/j.tplants.2016.12.008
Giberti, S., Funck, D., and Forlani, G. (2014). Δ1-pyrroline-5-carboxylate reductase from Arabidopsis thaliana: stimulation or inhibition by chloride ions and feedback regulation by proline depend on whether NADPH or NADH acts as co-substrate. New Phytol. 202, 911–919. doi: 10.1111/nph.12701
Girousse, C., Bournoville, R., and Bonnemain, J. L. (1996). Water deficit-induced changes in concentrations in proline and some other amino acids in the phloem sap of alfalfa. Plant Physiol. 111, 109–113. doi: 10.1104/pp.111.1.109
Goncalves, R. L., Rothschild, D. E., Quinlan, C. L., Scott, G. K., Benz, C. C., and Brand, M. D. (2014). Sources of superoxide/H2O2 during mitochondrial proline oxidation. Redox Biol. 2, 901–909. doi: 10.1016/j.redox.2014.07.003
Gosavi, G., Jadhav, A., Kale, A. A., Gadakh, S., Pawar, B., and Chimote, V. (2014). Effect of heat stress on proline, chlorophyll content, heat shock proteins and antioxidant enzyme activity in sorghum (Sorghum bicolor) at seedlings stage. Ind. J. Biotech. 13, 356–363.
Guan, C., Cui, X., Liu, H., Li, X., Li, M., and Zhang, Y. (2020). Proline biosynthesis enzyme genes confer salt tolerance to switchgrass (Panicum virgatum L.) in cooperation with polyamines metabolism. Front. Plant Sci. 11:46. doi: 10.3389/fpls.2020.00046
Guo, M., Zhang, X., and Liu, J. (2020). OsProDH negatively regulates thermotolerance in rice by modulating proline metabolism and reactive oxygen species scavenging. Rice 13:61. doi: 10.1186/s12284-020-00422-3
Guo, Y. P., Zhou, H. F., and Zhang, L. C. (2006). Photosynthetic characteristics and protective mechanisms against photooxidation during high temperature stress in two citrus species. Sci. Hortic. 108, 260–267. doi: 10.1016/j.scienta.2006.01.029
Gur, A., Demirel, U., Ozden, M., Kahraman, A., and Çopur, O. (2010). Diurnal gradual heat stress affects antioxidant enzymes, proline accumulation and some physiological components in cotton (Gossypium hirsutum L.). Afr. J. Biotechnol. 9, 1008–1015. doi: 10.5897/AJB09.1590
Gurrieri, L., Merico, M., Trost, P., Forlani, G., and Sparla, F. (2020). Impact of drought on soluble sugars and free proline content in selected Arabidopsis mutants. Biology 9:367. doi: 10.3390/biology9110367
Han, H. L., Liu, J., Feng, X. J., Zhang, M., Lin, Q. F., Wang, T., et al. (2021). SSR1 is involved in maintaining the function of mitochondria electron transport chain and iron homeostasis upon proline treatment in Arabidopsis. J. Plant Physiol. 256:153325. doi: 10.1016/j.jplph.2020.153325
Han, Y., Fan, S., Zhang, Q., and Wang, Y. (2013). Increased proline content was observed in leaf of lettuce seedlings under heat stress but the increase in heat-tolerant varieties was significantly higher than the non-heat tolerant varieties. Agric. Sci. 4, 112–115. doi: 10.4236/as.2013.45B021
Hancock, C. N., Liu, W., Alvord, W. G., and Phang, J. M. (2016). Co-regulation of mitochondrial respiration by proline dehydrogenase/oxidase and succinate. Amino Acids 48, 859–872. doi: 10.1007/s00726-015-2134-7
Hanif, S., Saleem, M., Sarwar, M., Irshad, M., Shakoor, A., Wahid, M., et al. (2021). Biochemically triggered heat and drought stress tolerance in rice by proline application. J. Plant Growth Reg. 40, 305–312. doi: 10.1007/s00344-020-10095-3
Hare, P. D., and Cress, W. A. (1997). Metabolic implications of stress-induced proline accumulation in plants. Plant Growth Reg. 21, 79–102. doi: 10.1023/A:1005703923347
Harsh, A., Sharma, Y. K., Joshi, U., Rampuria, S., Singh, G., Kumar, S., et al. (2016). Effect of short-term heat stress on total sugars, proline and some antioxidant enzymes in moth bean (Vigna aconitifolia). Ann. Agricult. Sci. 61, 57–64. doi: 10.1016/j.aoas.2016.02.001
Hasanuzzaman, M., Bhuyan, M. H. M. B., Zulfiqar, F., Raza, A., Mohsin, S. M., Mahmud, J. A., et al. (2020). Reactive oxygen species and antioxidant defense in plants under abiotic stress: revisiting the crucial role of a universal defense regulator. Antioxidants 9:681. doi: 10.3390/antiox9080681
Hossain, M. M., Takeda, H., and Senboku, T. (1995). Proline content in Brassica under high temperature stress. JIRCAS J. 2, 87–93.
Hu, S., Ding, Y., and Zhu, C. (2020). Sensitivity and responses of chloroplasts to heat stress in plants. Front. Plant Sci. 11:375. doi: 10.3389/fpls.2020.00375
Hua, X. J., Van de Cotte, B., Van Montagu, M., and Verbruggen, N. (2001). The 5’ untranslated region of the At-P5CR gene is involved in both transcriptional and post-transcriptional regulation. Plant J. 26, 157–169. doi: 10.1046/j.1365-313x.2001.01020.x
Huang, J., Zhao, X., and Chory, J. (2019). The Arabidopsis transcriptome responds specifically and dynamically to high light stress. Cell Rep. 29, 4186–4199. doi: 10.1016/j.celrep.2019.11.051
Huang, S., Taylor, N. L., Ströher, E., Fenske, R., and Millar, A. H. (2013). Succinate dehydrogenase assembly factor 2 is needed for assembly and activity of mitochondrial complex II and for normal root elongation in Arabidopsis. Plant J. 73, 429–441. doi: 10.1111/tpj.12041
Impa, S. M., Sunoj, V. S. J., Krassovskaya, I., Bheemanahalli, R., Obata, T., and Jagadish, S. V. K. (2019). Carbon balance and source-sink metabolic changes in winter wheat exposed to high night-time temperature. Plant Cell Environ. 42, 1233–1246. doi: 10.1111/pce.13488
Iqbal, N., Fatma, M., Khan, N. A., and Umar, S. (2019). “Regulatory role of proline in heat stress tolerance: modulation by salicylic acid,” in Plant Signaling Molecules, eds M. I. R. Khan, P. S. Reddy, A. Ferrante, and N. A. Khan (Sawston: Woodhead Publishing), 437–448. doi: 10.1016/b978-0-12-816451-8.00027-7
Jahan, M. S., Guo, S., Sun, J., Shu, S., Wang, Y., El-Yazied, A. A., et al. (2021). Melatonin-mediated photosynthetic performance of tomato seedlings under high-temperature stress. Plant Physiol. Biochem. 167, 309–320. doi: 10.1016/j.plaphy.2021.08.002
Kaur, G., and Asthir, B. (2015). Proline: a key player in plant abiotic stress tolerance. Biol. Plant 59, 609–619. doi: 10.1007/s10535-015-0549-3
Kaushal, N., Gupta, K., Bhandhari, K., Kumar, S., Thakur, P., and Nayyar, H. (2011). Proline induces heat tolerance in chickpea (Cicer arietinum L.) plants by protecting vital enzymes of carbon and antioxidative metabolism. Physiol. Mol. Biol. Plants 17, 203–213. doi: 10.1007/s12298-011-0078-2
Kesari, R., Lasky, J. R., Villamor, J. G., Des Marais, D. L., Chen, Y. J., Liu, T. W., et al. (2012). Intron-mediated alternative splicing of Arabidopsis P5CS1 and its association with natural variation in proline and climate adaptation. Proc. Natl. Acad. Sci. U.S.A. 109, 9197–9202. doi: 10.1073/pnas.1203433109
Khan, M. A., Asaf, S., Khan, A. L., Jan, R., Kang, S. M., Kim, K. M., et al. (2020). Thermotolerance effect of plant growth-promoting Bacillus cereus SA1 on soybean during heat stress. BMC Microbiol. 20:175. doi: 10.1186/s12866-020-01822-7
Kishor, P. B. K., Hima Kumari, P., Sunita, M. S. L., and Sreenivasulu, N. (2015). Role of proline in cell wall synthesis and plant development and its implications in plant ontogeny. Front. Plant Sci. 6:544. doi: 10.3389/fpls.2015.00544
Kishor, P. B. K., Hong, Z., Miao, C. H., Hu, C. A. A., and Verma, D. P. S. (1995). Overexpression of [delta]-pyrroline-5-carboxylate synthetase increases proline production and confers osmotolerance in transgenic plants. Plant Physiol. 108, 1387–1394. doi: 10.1104/pp.108.4.1387
Kishor, P. B. K., Sangam, S., Amrutha, R. N., Laxmi, P. S., Naidu, K. R., Rao, K. R. S. S., et al. (2005). Regulation of proline biosynthesis, degradation, uptake and transport in higher plants: its implications in plant growth and abiotic stress tolerance. Curr. Sci. 88, 424–438.
Kishor, P. B. K., and Sreenivasulu, N. (2014). Is proline accumulation per se correlated with stress tolerance or is proline homeostasis a more critical issue? Plant Cell Environ. 37, 300–311. doi: 10.1111/pce.12157
Kocsy, G., Laurie, R., Szalai, G., Szilagyi, V., Simon-Sarkadi, L., Galiba, G., et al. (2005). Genetic manipulation of proline levels affects antioxidants in soybean subjected to simultaneous drought and heat stresses. Physiol. Plant. 124, 227–235. doi: 10.1111/j.1399-3054.2005.00504.x
Launay, A., Cabassa-Hourton, C., Eubel, H., Maldiney, R., Guivarc’h, A., Crilat, E., et al. (2019). Proline oxidation fuels mitochondrial respiration during dark-induced leaf senescence in Arabidopsis thaliana. J. Exp. Bot. 70, 6203–6214. doi: 10.1093/jxb/erz351
Lee, B. R., Jin, Y. L., Avice, J. C., Cliquet, J. B., Ourry, A., and Kim, T. H. (2009). Increased proline loading to phloem and its effects on nitrogen uptake and assimilation in water-stressed white clover (Trifolium repens). New Phytol. 182, 654–663. doi: 10.1111/j.1469-8137.2009.02795.x
Lehmann, S., Funck, D., Szabados, L., and Rentsch, D. (2010). Proline metabolism and transport in plant development. Amino Acids 39, 949–962. doi: 10.1007/s00726-010-0525-3
Lehr, P., Hernández-Montes, E., Ludwig-Müller, J., Keller, M., and Zörb, C. (2022). Abscisic acid and proline are not equivalent markers for heat, drought and combined stress in grapevines. Aust. J. Grape Wine Res. 28, 119–130. doi: 10.1111/ajgw.12523
Liang, X., Zhang, L., Natarajan, S. K., and Becker, D. F. (2013). Proline mechanisms of stress survival. Antioxid. Redox Signal. 19, 998–1011. doi: 10.1089/ars.2012.5074
Liu, Y., Borchert, G. L., Surazynski, A., Hu, C. A., and Phang, J. M. (2006). Proline oxidase activates both intrinsic and extrinsic pathways for apoptosis: the role of ROS/superoxides, NFAT and MEK/ERK signaling. Oncogene 25, 5640–5647. doi: 10.1038/sj.onc.1209564
Lobell, D. B., and Field, C. B. (2007). Global scale climate-crop yield relationships and the impacts of recent warming. Environ. Res. Lett. 2:014002. doi: 10.1088/1748-9326/2/1/014002
Lobell, D. B., Schlenker, W., and Costa-Roberts, J. (2011). Climate trends and global crop production since 1980. Science 333, 616–620. doi: 10.1126/science.1204531
Lopez-Delacalle, M., Silva, C. J., Mestre, T. C., Martinez, V., Blanco-Ulate, B., and Rivero, R. M. (2021). Synchronization of proline, ascorbate and oxidative stress pathways under the combination of salinity and heat in tomato plants. Env. Exp. Bot. 183:104351. doi: 10.1016/j.envexpbot.2020.104351
Lunn, J. E. (2006). Compartmentation in plant metabolism. J. Exp. Bot. 58, 35–47. doi: 10.1093/jxb/erl134
Lv, W. T., Lin, B., Zhang, M., and Hua, X. G. (2011). Proline accumulation is inhibitory to Arabidopsis seedlings during heat stress. Plant Physiol. 156, 1921–1933. doi: 10.1104/pp.111.175810
Maria, E. A., Savouré, A., and Szabados, L. (2021). Proline metabolism as regulatory hub. Trends Plant Sci. 27, 39–55. doi: 10.1016/j.tplants.2021.07.009
Mattioli, R., Biancucci, M., El Shall, A., Mosca, L., Costantino, P., Funck, D., et al. (2018). Proline synthesis in developing microspores is required for pollen development and fertility. BMC Plant Biol. 18:356. doi: 10.1186/s12870-018-1571-3
Mattioli, R., Palombi, N., Funck, D., and Trovato, M. (2020). Proline accumulation in pollen grains as potential target for improved yield stability under salt stress. Front. Plant Sci. 11:582877. doi: 10.3389/fpls.2020.58287
Matysik, J., Alia, A., Bhalu, B., and Mohanty, P. (2002). Molecular mechanisms of quenching of reactive oxygen species by proline under stress in plants. Curr. Sci. 82, 525–532. doi: 10.1111/ppl.12563
Miller, G., Honig, A., Stein, H., Suzuki, N., Mittler, R., and Zilberstein, A. (2009). Unraveling delta1-pyrroline-5-carboxylate-proline cycle in plants by uncoupled expression of proline oxidation enzymes. J. Biol. Chem. 284, 26482–26492. doi: 10.1074/jbc.M109.009340
Mishra, D., Shekhar, S., Agrawal, L., Chakraborty, S., and Chakraborty, N. (2017). Cultivar-specific high temperature stress responses in bread wheat (Triticum aestivum L.) associated with physicochemical traits and defense pathways. Food Chem. 221, 1077–1087. doi: 10.1016/j.foodchem.2016.11.053
Mohanty, S., Baishna, B. G., and Tripathy, C. (2006). Light and dark modulation of chlorophyll biosynthetic genes in response to temperature. Planta 224, 692–699. doi: 10.1007/s00425-006-0248-6
Molinari, H. B. C., Daros, E., Marur, C. J., and Vieira, L. G. E. (2007). Evaluation of the stress-inducible production of proline in transgenic sugarcane (Saccharum spp.): osmotic adjustment, chlorophyll fluorescence and oxidative stress. Physiol. Plant. 130, 218–229. doi: 10.1111/j.1399-3054.2007.00909.x
Moller, I. M., and Rasmusson, A. G. (1998). The role of NADP in the mitochondrial matrix. Trends Plant Sci. 3, 21–27. doi: 10.1016/S1360-1385(97)01156-4
Monne, M., Daddabbo, L., Gagneul, D., Obata, T., Hielscher, B., Palmieri, L., et al. (2018). Uncoupling proteins1 and 2 (UCP1 and UCP2) from Arabidopsis thaliana are mitochondrial transporters of aspartate, glutamate, and dicarboxylates. J. Biol. Chem. 293, 4213–4227. doi: 10.1074/jbc.RA117.000771
Moustakas, M., Sperdouli, I., Kouna, T., Antonopoulou, C., and Therios, I. (2011). Exogenous proline induces soluble sugar accumulation and alleviates drought stress effects on photosystem II functioning of Arabidopsis thaliana leaves. Plant Growth Reg. 65:315. doi: 10.1007/s10725-011-9604-z
Nahar, L., Aycan, M., Hanamata, S., Baslam, M., and Mitsui, T. (2022). Impact of single and combined salinity and high-temperature stresses on agro-physiological, biochemical, and transcriptional responses in rice and stress-release. Plants (Basel) 11:501. doi: 10.3390/plants11040501
Nesterenko, O., and Rashydov, N. (2018). Features of the proline synthesis of pea seedlings independent of salt and hyperthermia treatment coupled with ionizing radiation. Int. J. Sec. Metabol. 5, 94–108. doi: 10.21448/ijsm.407285
Oukarroum, A., Madidi, S. E., and Strasser, R. J. (2012). Exogenous glycine betaine and proline play a protective role in heat-stressed barley leaves (Hordeum vulgare L.): a chlorophyll a fluorescence study. Plant Biosyst. Int. J. Dealing Aspects Plant Biol. 146, 1037–1043. doi: 10.1080/11263504.2012.697493
Palmieri, F., Rieder, B., Ventrella, A., Blanco, E., Do, P. T., Nunes-Nesi, A., et al. (2009). Molecular identification and functional characterization of Arabidopsis thaliana mitochondrial and chloroplastic NAD+ carrier proteins. J. Biol. Chem. 284, 31249–31259. doi: 10.1074/jbc.M109.041830
Pandhare, J., Donald, S. P., Cooper, S. K., and Phang, J. M. (2009). Regulation and function of proline oxidase under nutrient stress. J. Cellular Biochem. 107, 759–768. doi: 10.1002/jcb.22174
Peng, S., Huang, J., Sheehy, J. E., Laza, R. C., Visperas, R. M., Zhong, X., et al. (2004). Rice yields decline with higher night temperature from global warming. Proc. Natl. Acad. Sci. U.S.A. 101, 9971–9975. doi: 10.1073/pnas.0403720101
Per, T. S., Khan, N. A., Reddy, P. S. B., Masood, A., Hasanuzzaman, M., Khan, M. I. R., et al. (2017). Approaches in modulating proline metabolism in plants for salt and drought stress tolerance: phytohormones, mineral nutrients and transgenics. Plant Physiol. Biochem. 115, 126–140. doi: 10.1016/j.plaphy.2017.03.018
Phang, J. M. (2019). Proline metabolism in cell regulation and cancer biology: recent advances and hypotheses. Antioxid. Redox Signal. 30, 635–649. doi: 10.1089/ars.2017.7350
Porcelli, V., Vozza, A., Calcagnile, V., Gorgoglione, R., Arrigoni, R., Fontanesi, F., et al. (2018). Molecular identification and functional characterization of a novel glutamate transporter in yeast and plant mitochondria. Biochim. Biophys. Acta Bioenerget. 1859, 1249–1258. doi: 10.1016/j.bbabio.2018.08.001
Pospisilova, J., Haisel, D., and Vankova, R. (2011). Response of transgenic tobacco plants with increased proline content to drought and/or heat stress. Am. J. Plant Sci. 2, 318–324. doi: 10.4236/ajps.2011.23036
Rajametov, S. N., Yang, E. Y., Cho, M. C., Chae, S. Y., Jeong, H. B., Chae, W. B., et al. (2021). Heat-tolerant hot pepper exhibits constant photosynthesis via increased transpiration rate, high proline content and fast recovery in heat stress condition. Sci. Rep. 11:14328. doi: 10.1038/s41598-021-93697-5
Rasheed, R., Wahid, A., Farooq, M., Hussain, I., and Basra, S. (2011). Role of proline and glycinebetaine pretreatments in improving heat tolerance of sprouting sugarcane (Saccharum sp.) buds. Plant Growth Reg. 65, 35–45. doi: 10.1007/s10725-011-9572-3
Reddy, P. S., Jogeswar, G., Rasineni, G. K., Maheswari, M., Reddy, A. R., Varshney, R. K., et al. (2015). Proline over-accumulation alleviates salt stress and protects photosynthetic and antioxidant enzyme activities in transgenic sorghum [Sorghum bicolor (L.) Moench]. Plant Physiol. Biochem. 94, 104–113. doi: 10.1016/j.plaphy.2015.05.014
Rehman, A. U., Bashir, F., Ayaydin, F., Kota, Z., Pali, T., and Vass, I. (2021). Proline is a quencher of singlet oxygen and superoxide both in in vitro systems and isolated thylakoids. Physiol. Plant. 172, 7–18. doi: 10.1111/ppl.13265
Ren, Y., Miao, M., Meng, Y., Cao, J., Fan, T., Yue, J., et al. (2018). DFR1-mediated inhibition of proline degradation pathway regulates drought and freezing tolerance in Arabidopsis. Cell Rep. 23, 3960–3974. doi: 10.1016/j.celrep.2018.04.011
Rivero, R. M., Mestre, T. C., Mittler, R., Rubio, F., Garcia-Sanchez, F., and Martinez, V. (2014). The combined effect of salinity and heat reveals a specific physiological, biochemical and molecular response in tomato plants. Plant Cell Environ. 37, 1059–1073. doi: 10.1111/pce.12199
Rivero, R. M., Ruiz, J. M., and Romero, L. (2004). Importance of N source on heat stress tolerance due to the accumulation of proline and quaternary ammonium compounds in tomato plants. Plant Biol. 6, 702–707. doi: 10.1055/s-2004-821293
Rizhsky, L., Liang, H. J., Shuman, J., Shulaev, V., Davletova, S., and Mittler, R. (2004). When defense pathways collide: the response of Arabidopsis to a combination of drought and heat stress. Plant Physiol. 134, 1683–1696. doi: 10.1104/pp.103.033431
Rizzi, Y. S., Cecchini, N. M., Fabro, G., and Alvarez, M. E. (2016). Differential control and function of Arabidopsis ProDH1 and ProDH2 genes on infection with biotrophic and necrotrophic pathogens. Mol. Plant Pathol. 18, 1164–1174. doi: 10.1111/mpp.12470
Ruszkowski, M., Nocek, B., Forlani, G., and Dauter, Z. (2015). The structure of Medicago truncatula Δ1-pyrroline-5-carboxylate reductase provides new insights into regulation of proline biosynthesis in plants. Front. Plant Sci. 6:869. doi: 10.3389/fpls.2015.00869
Sabbioni, G., Funck, D., and Forlani, G. (2021). Enzymology and regulation of δ1-pyrroline-5-carboxylate synthetase 2 from rice. Front. Plant Sci. 12:672702. doi: 10.3389/fpls.2021.672702
Saikia, B., Singh, S., Debbarma, J., Velmurugan, N., Dekaboruah, H., Arunkumar, K. P., et al. (2020). Multigene CRISPR/Cas9 genome editing of hybrid proline rich proteins (HyPRPs) for sustainable multi-stress tolerance in crops: the review of a promising approach. Physiol. Mol. Biol. Plants Int. J. Funct. Plant Biol. 26, 857–869. doi: 10.1007/s12298-020-00782-6
Sattar, A., Sher, A., Ijaz, M., Ul-Allah, S., Rizwan, M. S., Hussain, M., et al. (2020). Terminal drought and heat stress alter physiological and biochemical attributes in flag leaf of bread wheat. PLoS One 15:e0232974. doi: 10.1371/journal.pone.0232974
Scheibe, R., Backhausen, J. E., Emmerlich, V., and Holtgrefe, S. (2005). Strategies to maintain redox homeostasis during photosynthesis under changing conditions. J. Exp. Bot. 56, 1481–1489. doi: 10.1093/jxb/eri181
Schwacke, R., Grallath, S., Breitkreuz, K. E., Stransky, E., Stransky, H., Frommer, W. B., et al. (1999). LeProT1, a transporter for proline, glycine betaine, and γ-amino butyric acid in tomato pollen. Plant Cell 11, 377–392. doi: 10.1105/tpc.11.3.377
Schworer, S., Berisa, M., Violante, S., Qin, W., Zhu, J., Hendrickson, R. C., et al. (2020). Proline biosynthesis is a vent for TGFβ-induced mitochondrial redox stress. EMBO J. 39:e103334. doi: 10.15252/embj.2019103334
Sharkova, V. E. (2001). The Effect of heat shock on the capacity of wheat plants to restore their photosynthetic electron transport after photoinhibition or repeated heating. Russ. J. Plant Physiol. 48, 793–797. doi: 10.1023/A:1012564709996
Sharma, S., Villamor, J. G., and Verslues, P. E. (2011). Essential role of tissue specific proline synthesis and catabolism in growth and redox balance at low water potential. Plant Physiol. 157, 292–304. doi: 10.1104/pp.111.183210
Shin, H., Oh, S., Arora, R., and Kim, D. (2016). Proline accumulation in response to high temperature in winter-acclimated shoots of Prunus persica: a response associated with growth resumption or heat stress? Can. J. Plant Sci. 96, 630–638. doi: 10.1139/cjps-2015-0372
Shinde, S., Villamor, J. G., Lin, W., Sharma, S., and Verslues, P. E. (2016). Proline coordination with fatty acid synthesis and redox metabolism of chloroplast and mitochondria. Plant Physiol. 172, 1074–1088. doi: 10.1104/pp.16.01097
Signorelli, S. (2016). The fermentation analogy: a point of view for understanding the intriguing role of proline accumulation in stressed plants. Front. Plant Sci. 7:1339. doi: 10.3389/fpls.2016.01339
Signorelli, S., Arellano, J. B., Melø, T. B., Borsani, O., and Monza, J. (2013). Proline does not quench singlet oxygen: evidence to reconsider its protective role in plants. Plant Physiol. Biochem. 64, 80–83. doi: 10.1016/j.plaphy.2012.12.017
Signorelli, S., Coitiño, E. L., Borsani, O., and Monza, J. (2014). Molecular mechanisms for the reaction between ⋅OH radicals and proline: insights on the role as reactive oxygen species scavenger in plant stress. J. Phys. Chem. B 118, 37–47. doi: 10.1021/jp407773u
Signorelli, S., Dans, P. D., Coitiño, E. L., Borsani, O., and Monza, J. (2015). Connecting proline and γ-amino butyric acid in stressed plants through non-enzymatic reactions. PLoS One 10:e0115349. doi: 10.1371/journal.pone.0115349
Signorelli, S., Imparatta, C., Rodríguez-Ruiz, M., Borsani, O., Corpas, F. J., and Monza, J. (2016). In vivo and in vitro approaches demonstrate proline is not directly involved in the protection against superoxide, nitric oxide, nitrogen dioxide and peroxynitrite. Funct. Plant Biol. 43, 870–879. doi: 10.1071/fp16060
Song, S. Q., Lei, Y. B., and Tian, X. R. (2005). Proline metabolism and cross tolerance to salinity and heat stress in germinating wheat seeds. Russ. J. Plant Physiol. 52, 793–800. doi: 10.1007/s11183-005-0117-3
Szabados, L., and Savouré, A. (2010). Proline: a multifunctional amino acid. Trends Plant Sci. 15, 89–97. doi: 10.1016/j.tplants.2009.11.009
Szekely, G., Abrahám, E., Cséplo, A., Rigó, G., Zsigmond, L., Csiszár, J., et al. (2008). Duplicated P5CS genes of Arabidopsis play distinct roles in stress regulation and developmental control of proline biosynthesis. Plant J. 53, 11–28. doi: 10.1111/j.1365-313X.2007.03318.x
Tonhati, R., Mello, S. C., Momesso, P., and Pedroso, R. M. (2020). L-proline alleviates heat stress of tomato plants grown under protected environment. Sci. Horticult. 268:109370. doi: 10.1016/j.scienta.2020.109370
Toth, S. Z., Schansker, G., Kissimon, J., Kovacs, L., Garab, G., and Strasser, R. J. (2005). Biophysical studies of photosystem II-related recovery processes after a heat pulse in barley seedlings (Hordeum vulgare L.). J. Plant Physiol. 162, 181–194. doi: 10.1016/j.jplph.2004.06.010
Tran, M. T., Doan, D. T. H., Kim, J., Song, Y. J., Sung, Y. W., Das, S., et al. (2021). CRISPR/Cas9-based precise excision of SlHyPRP1 domain(s) to obtain salt stress-tolerant tomato. Plant Cell Rep. 40, 999–1011. doi: 10.1007/s00299-020-02622-z
Verslues, P. E., Lasky, J. R., Juenger, T. E., Liu, T. W., and Kumar, M. N. (2014). Genome-wide association mapping combined with reverse genetics identifies new effectors of low water potential-induced proline accumulation in Arabidopsis. Plant Physiol. 164, 144–159. doi: 10.1104/pp.113.224014
Verslues, P. E., and Sharma, S. (2010). Proline metabolism and its implications for plant-environment interaction. Arabidopsis Book 8:e0140. doi: 10.1199/tab.0140
Vollenweider, P., and Gunthardt-Goerg, M. S. (2005). Diagnosis of abiotic and biotic stress factors using the visible symptoms in foliage. Environ. Pollut. 137, 455–465. doi: 10.1016/j.envpol.2005.01.032
Wahid, A., Gelani, S., Ashraf, M., and Foolad, M. R. (2007). Heat tolerance in plants: an overview. Environ. Exp. Bot. 61, 199–223. doi: 10.1016/j.envexpbot.2007.05.011
Walter, D. R. (2003). Resistance to plant pathogens; possible role of free polyamines and polyamine catabolism. New Phytol. 159, 109–115. doi: 10.1046/j.1469-8137.2003.00802.x
Wang, J., Yuan, B., Xu, Y., and Huang, B. (2018). Differential responses of amino acids and soluble proteins to heat stress associated with genetic variations in heat tolerance for hard fescue. J. Am. Soc. Horticult. Sci. 143, 45–55. doi: 10.21273/JASHS04246-17
White, T. A., Krishnan, N., Becker, D. F., and Tanner, J. J. (2007). Structure and kinetics of monofunctional proline dehydrogenase from Thermus thermophilus. J. Biol. Chem. 282, 14316–14327. doi: 10.1074/jbc.M700912200
Winter, G., Todd, C., Trovato, M., Forlani, G., and Funck, D. (2015). Physiological implications of arginine metabolism in plants. Front. Plant Sci. 6:534. doi: 10.3389/fpls.2015.00534
Wise, R. R., Olson, A. J., Schrader, S. M., and Sharkey, T. D. (2004). Electron transport is the functional limitation of photosynthesis in field-grown Pima cotton plants at high temperature. Plant Cell Environ. 27, 717–724. doi: 10.1111/j.1365-3040.2004.01171.x
Xu, J., Henry, A., and Sreenivasulu, N. (2020). Rice yield formation under high day and night temperatures-A prerequisite to ensure future food security. Plant Cell Environ. 43, 1595–1608. doi: 10.1111/pce.13748
Yang, D., Ni, R., Yang, S., Pu, Y., Qian, M., Yang, Y., et al. (2021). Functional characterization of the Stipa purpurea P5CS gene under drought stress conditions. Int. J. Mol. Sci. 22:9599. doi: 10.3390/ijms22179599
Zarse, K., Schmeisser, S., Groth, M., Priebe, S., Beuster, G., Kuhlow, D., et al. (2012). Impaired insulin/IGF1 signaling extends life span by promoting mitochondrial L-proline catabolism to induce a transient ROS signal. Cell Metab. 15, 451–465. doi: 10.1016/j.cmet.2012.02.013
Zavafer, A., González-Solís, A., Palacios-Bahena, S., Saucedo-Garcia, M., de Aquino, C. T., Vazquez-Santana, S., et al. (2020). Organized disassembly of photosynthesis during programmed cell death mediated by long chain bases. Sci. Rep. 10:10360. doi: 10.1038/s41598-020-65186-8
Zdunek-Zastocka, E., Grabowska, A., Michniewska, B., and Orzechowski, S. (2021). Proline concentration and its metabolism are regulated in a leaf age dependent manner but not by abscisic acid in pea plants exposed to cadmium stress. Cells 10:946. doi: 10.3390/cells10040946
Zhang, L., Alfano, J. R., and Becker, D. F. (2015). Proline metabolism increases katG expression and oxidative stress resistance in Escherichia coli. J. Bacteriol. 197, 431–440. doi: 10.1128/JB.02282-14
Keywords: heat stress, proline cycle, radical scavenging, reactive oxygen species, redox couple
Citation: Kavi Kishor PB, Suravajhala P, Rathnagiri P and Sreenivasulu N (2022) Intriguing Role of Proline in Redox Potential Conferring High Temperature Stress Tolerance. Front. Plant Sci. 13:867531. doi: 10.3389/fpls.2022.867531
Received: 01 February 2022; Accepted: 21 April 2022;
Published: 10 June 2022.
Edited by:
Ashish Kumar Srivastava, Bhabha Atomic Research Centre (BARC), IndiaReviewed by:
Sudhakar Reddy Palakolanu, International Crops Research Institute for the Semi-Arid Tropics (ICRISAT), IndiaCopyright © 2022 Kavi Kishor, Suravajhala, Rathnagiri and Sreenivasulu. This is an open-access article distributed under the terms of the Creative Commons Attribution License (CC BY). The use, distribution or reproduction in other forums is permitted, provided the original author(s) and the copyright owner(s) are credited and that the original publication in this journal is cited, in accordance with accepted academic practice. No use, distribution or reproduction is permitted which does not comply with these terms.
*Correspondence: P. B. Kavi Kishor, cGJrYXZpQHlhaG9vLmNvbQ==; Nese Sreenivasulu, bi5zcmVlbml2YXN1bHVAaXJyaS5vcmc=
†ORCID: Prashanth Suravajhala, orcid.org/0000-0002-8535-278X
Disclaimer: All claims expressed in this article are solely those of the authors and do not necessarily represent those of their affiliated organizations, or those of the publisher, the editors and the reviewers. Any product that may be evaluated in this article or claim that may be made by its manufacturer is not guaranteed or endorsed by the publisher.
Research integrity at Frontiers
Learn more about the work of our research integrity team to safeguard the quality of each article we publish.