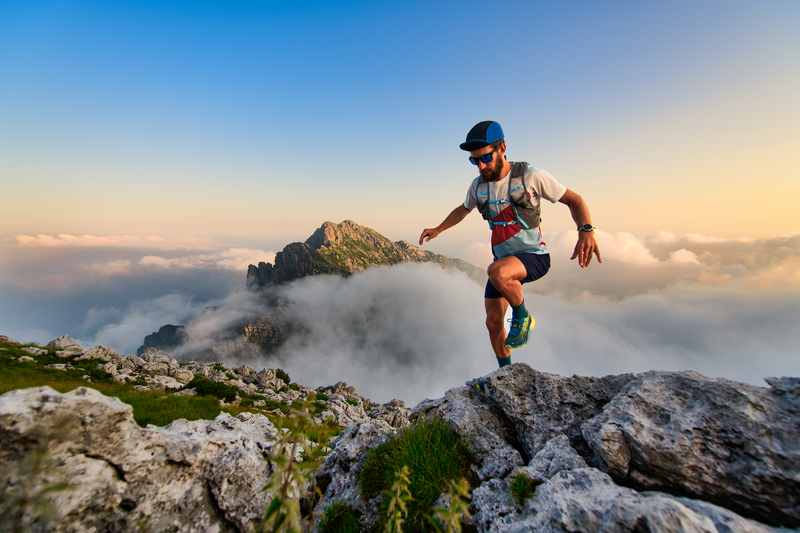
95% of researchers rate our articles as excellent or good
Learn more about the work of our research integrity team to safeguard the quality of each article we publish.
Find out more
REVIEW article
Front. Plant Sci. , 13 May 2022
Sec. Plant Physiology
Volume 13 - 2022 | https://doi.org/10.3389/fpls.2022.866409
This article is part of the Research Topic Abiotic Stress Signaling in Plants: Functional Genomic Intervention, Volume II View all 18 articles
Plants can adapt to different environmental conditions and can survive even under very harsh conditions. They have developed elaborate networks of receptors and signaling components, which modulate their biochemistry and physiology by regulating the genetic information. Plants also have the abilities to transmit information between their different parts to ensure a holistic response to any adverse environmental challenge. One such phenomenon that has received greater attention in recent years is called stress priming. Any milder exposure to stress is used by plants to prime themselves by modifying various cellular and molecular parameters. These changes seem to stay as memory and prepare the plants to better tolerate subsequent exposure to severe stress. In this review, we have discussed the various ways in which plants can be primed and illustrate the biochemical and molecular changes, including chromatin modification leading to stress memory, with major focus on thermo-priming. Alteration in various hormones and their subsequent role during and after priming under various stress conditions imposed by changing climate conditions are also discussed.
Plants being sessile by nature are perpetually manifested with various adverse abiotic and biotic conditions. These unfavorable conditions usually occur together; concurrently or sequentially and negatively impact plant growth, ultimately leading to loss of crop yields (Pandey et al., 2017; Hilker and Schmülling, 2019). Plants possess efficient signaling networks and a sophisticated innate immune system, which enables them to endure a combination of diverse stresses. They seem to have well-defined mechanisms and strategies to remember the impeding stresses by inducing “stress memory” (Martinez-Medina et al., 2016; Hossain et al., 2020).
The concept of memory in relation to plant biology was first indicated by Charles Darwin while analyzing the Venus flytrap (Dionaea muscipula), for its ability to recognize and grasp insects (Darwin and Darwin, 1880). The term “memory” has been used to describe processes used for acquiring, storing, retaining and retrieving information. In humans and animals, it has been mainly linked with neuronal communications. In case of plants, however, “memory” has been used to describe three behaviors namely, keeping time, chemical talks within self and cross-talks with surroundings (Leopold, 2014).
The work on the retention of memory was demonstrated by Gagliano and her colleagues by using a potted touch-me-not plant (Mimosa pudica) (Gagliano et al., 2018). The beneficial adaptable behaviors in plants were considered to be similar to non-conscious, involuntary primitive qualities. Prof. Rainer Hedrich and his team elucidated that various action potentials elicited by an insect in insectivorous plants could be effectively mimicked by electric pulses. The pulses appraised and forewarned the plants with regards to the size of the prey and constitution of the nutrients (Böhm et al., 2016). These experiments demonstrated that plants could learn in terms of counting, memorizing and interpreting the action potentials evoked due to mechanical stimuli and further interpreting these stimuli to effectuate gene expression (Scherzer et al., 2017).
The ability of plants to retain memory in response to milder impact of various biotic and abiotic challenges is called stress priming. In this review we will discuss the biochemical and molecular events induced during stress priming, which may be the cause or effect for the retention of memory. This stress memory can even be passed on to the next generation (Bruce et al., 2007). These changes may eventually help the plants to ward off the negative impact of subsequent severe stress. The descriptions mainly include examples from thermo-priming but examples from other stress priming events are also discussed to better explain the cellular molecular mechanisms.
Stress can appear at any stage during the development of a plant and it affects essential processes like photosynthesis, membrane stability, gene expression, RNA splicing, protein synthesis, etc (Sah et al., 2016). When exposed to stress plants undergo several changes, which eventually allow them to tolerate stress and survive. When plants are subjected to a mild or sub-lethal stress treatment and permitted to regain, they form a “memory” which ensures that future stress stimuli are not as detrimental as the severe stress given at the first time, even in sensitive plants (Mittler et al., 2012; Crisp et al., 2016). The “memory” response forms the basis of the process of stress priming to facilitate protection of plants upon exposure to subsequent harsh stress. Stress priming is also referred to as stress hardening, stress training, or stress conditioning. Priming has attracted intensive research over the last decade as primed plants show better adaptability and improved stress tolerance. Recent research interests are now focused on understanding and developing stress priming as a viable technique for improving plant tolerance to diverse stresses (Balmer et al., 2015; Martinez-Medina et al., 2016; Xiao et al., 2017; Liu H. et al., 2021).
There are several reports to support that an earlier exposure to a modest high temperature stress (HTS) eliciting stimulus can prime a plant to acclimate to a further exposure of same or different stress (Table 1). Alexandrov (1956) first used the term “heat hardening” to describe this occurrence and he observed that hardening time and temperature are inversely proportional. Likewise, thermo-tolerance can be induced in plants after priming with other biotic or abiotic stress (Table 1). Plants can also be primed for a better response to unfavorable conditions by treatment with specific chemicals like salicylic acid (SA) or JA (Aranega-Bou et al., 2014; Conrath et al., 2015), herbivory and by colonization with microbes like rhizobacterium species (Conrath, 2011; Pieterse et al., 2014; Liu and Avramova, 2016).
Table 1. Thermo-priming for enhancing plant tolerance to high temperature and other abiotic stresses.
The effect of priming can be short-term or long-term and the process can be achieved by exposing plants at three different developmental stages to different abiotic and/or biotic elicitors:
(a) Priming of seeds helps to evade diverse stresses at the time of germination by inducing cross-stress tolerance. Seed priming accelerated germination and emergence to allow seedlings to escape abiotic stress such as HTS (Banerjee and Roychoudhury, 2020; Kumari et al., 2021).
(b) Priming at the post-embryonic stage increases the likelihood of the plant for surviving through the environmental changes. Its effects are mainly observed in the existing generation so it is also categorized as intergenerational, mitotic or somatic stress memory. Yucel et al. (1992) demonstrated that pre-heat treated wheat seedlings displayed thermal tolerance to HTS and showed reduced loss in photosynthetic capacity.
(c) Priming of parental population, such that the effects are transmitted to progenies constitute trans-generational priming. Its effects influence the future generations so it is also categorized as meiotic stress memory (Munné-Bosch and Alegre, 2013). In trans-generational stress priming, the memory for response to environmental stresses are likely to be packaged into seeds along with other resources and these provide crucial information to the new seedling during germination and beginning of plant development (Herman and Sultan, 2011). Studies in Brassica rapa indicated that changes in the profiles of non-coding RNA due to HTS could be transmitted to the next generation (Bilichak, 2015). The effect of HTS on seed germination and seed vigor in the progeny from thermo-primed parents has been studied in wheat (Liu, 2020; Zhou et al., 2020).
Initially it was proposed that memory of biotic priming is conferred trans-generationally to protect the progeny against recurring biotic stresses (Luna et al., 2012), but soon it was discovered that memory of abiotic stresses is also passed on to the next generations.
Priming can also be categorized based on the nature of the stress signals into cis- and trans- priming. When the eliciting stimulus and the response-triggering stress are of the same nature it is termed as “cis-priming.” If the eliciting stimulus is different from the response-triggering stress it is termed as “trans-priming” (Hilker et al., 2016).
Priming with a particular biotic or abiotic stress can stimulate plant tolerance to subsequent occurrence of same stress and such examples are categorized as cis-priming. It was unequivocally demonstrated using different plants that priming with high temperatures could help in increasing thermo-tolerance to subsequent HTS (Wang et al., 2014a; Zhang et al., 2016b). The preservation of thermo-memory involved expression of specific genes and epigenetic markings (Shi et al., 2016). In wheat seedlings, thermo-priming for two consecutive days with temperature which was 8°C higher than day/night temperatures, increased the tolerance of mature plants to subsequent HTS occurring after anthesis. The primed plants displayed enhanced photosynthetic capacity, higher scavenging capacity of reactive oxygen species (ROS) and greater grain starch accumulation, as compared to plants, which were not primed (Wang X. et al., 2012). These traits were attributed to the up regulation of expression of antioxidant genes in the primed plants (Wang X. et al., 2012; Wang et al., 2014a). High temperature exposure to 10 days old Arabidopsis thaliana induced attenuated memory, early flowering and post-transcription gene silencing in the next generation (Suter and Widmer, 2013; Liu J. et al., 2019). Pre-exposure to sub-lethal high temperatures boosted thermo-tolerance of rice plants and their progenies at the vegetative stage (Shi et al., 2016).
Similarly, priming plants with low temperatures was shown to successfully boost their tolerance to cold stress (Li X. et al., 2014). Seven days pre-exposure of wheat plants at tillering stage to a temperature which was 5°C less than surrounding environmental temperature (10°C), could relieve the damaging impact of low temperature (14°C) stress (Li X. et al., 2014). Cold priming resulted in accumulation of metabolites like sucrose, proline and other osmolytes thereby contributing to the stability of cellular membranes against cold stress (Iba, 2002). It also induced the expression of genes encoding antioxidant enzymes, electron transport chain during photosynthesis, synthesis of chlorophyll and starch thereby ameliorating cell membrane damage and protecting photosynthesis apparatus (Byun et al., 2014; Li X. et al., 2014).
trans-priming includes all cases where pre-exposure to a particular biotic or abiotic stress can stimulate plant tolerance to subsequent occurrence of another stress. For instance, drought priming wheat plants at the stem elongation stage induced cross-tolerance to HTS at grain filling stage and reduced yield loss (Wang et al., 2015). Drought primed plants exhibited increase in rate of carboxylation and photosynthesis and reduction in rate of energy loss under HTS (Wang et al., 2015). Both drought primed and unstressed control wheat plants showed similar levels of total protein, glutenin macropolymers and high molecular weight glutenin subunits, which suggested that drought priming protected the grain quality under HTS (Zhang et al., 2013a). Similarly, intermediate drought stress during early seedling stage was found to reduce the damage of subsequent cold stress in wheat by activation of antioxidant enzymes and maintenance of photosynthesis (Li X. et al., 2015). In wheat, pre-treatment of the parent plants with drought or HTS during grain filling imparted trans-generational cross-tolerance to their offspring against HTS at post-anthesis stage (Wang X. et al., 2018). The progenies showed higher grain yield, better maintenance of leaf photosynthesis, enhanced activities of antioxidant enzymes and reduced cell membrane damage (Wang et al., 2016). Drought primed plant progenies also showed cross tolerance to HTS during grain filling due to increased in rate of photosynthesis, reduction of membrane damage and increase in grain yield (Zhang et al., 2016a). The imprint of trans-priming of stress tolerance was also observed in rice, tobacco, radish and alfalfa (Bruce et al., 2007; Choi and Sano, 2007; Vu et al., 2015).
Trans-priming may be facilitated as similar or overlapping signaling pathways are involved in the perception and response to most abiotic stresses in plant (Zhu, 2016). HTS resulted in acquired resistance against the heavy metals such as cadmium, aluminum, iron and copper (Orzech and Burke, 1988). It was shown that HTS treatment increased the production of antioxidant enzymes in plants, which helped to regulate the ROS production triggered by metals (Kochhar and Kochhar, 2005; Hsu and Kao, 2007). Thermo-priming could enhance the preservation of many fruits, particularly those from tropical or subtropical locations, prior to exposure to low temperatures. Response to thermo-priming was associated with accumulation of heat shock proteins (HSPs: HSP70 and HSP79), small HSPs (sHSP22, sHSP18.1, sHSP18.2) increase in antioxidant enzymes (APX, catalase), maintenance of ultrastructure, reduction of membrane leakage and lipid peroxidation. These processes were also associated with providing chilling tolerance (Lurie and Klein, 1991; Jennings and Saltveit, 1994; Collins et al., 1995; Sabehat et al., 1996; Sato et al., 2001; Rozenzvieg et al., 2004; Zhang et al., 2005). A variety of plants could develop cross-tolerance to salt stress after being pre-exposed to HTS. The thermo-memory prior to salt stress caused an increase in H2O2, which resulted in enhanced survival and decreased damage when later exposed to NaCl (Gong et al., 2001). Heat pre-treatment was also shown to increase salinity tolerance by improving antioxidant activity and glyoxalase metabolism in mustard, tobacco and cashew plants (Harrington and Alm, 1988; Hossain et al., 2013b; Pérez-Salamó et al., 2014).
Priming seeds with hormone solutions, bacteria and chemicals is also known to substantially improve seedling emergence, seed germination and plant growth under HTS (Hasanuzzaman and Fotopoulos, 2019). Seed priming with combination of ascorbic acid and SA improved rice growth under HTS (Kata et al., 2014). Priming seeds with bacteria such as Bacillus spp. and Azospirillum spp. improved tolerance against HTS by reducing ROS production (Abd El-Daim et al., 2014). Wheat seeds inoculated with rhizobacteria substantially increased thermo-tolerance of the seedlings (Yang et al., 2009; Anderson and Habiger, 2012). The endophytic bacteria present inside plant tissues have also been implicated in priming process to promote plant growth (Singh et al., 2021). Recently it was reported that colonization by Enterobacter sp. SA187, isolated from root nodules of the desert plant Indigofera argentea lead to HTS tolerance in wheat seedlings under field conditions (Shekhawat et al., 2021). Similarly, endophytic fungus, Paecilomyces formosus (Waqas et al., 2015) and arbuscular mycorrhizal fungi (AMF) (Begum et al., 2019) were shown to improve plant growth under HTS. AMF improved growth by enhancing uptake of water and nutrients, increasing the activity of antioxidant enzymes and improving the rates of photosynthesis (Zhu et al., 2011; Maya and Matsubara, 2013).
Priming of plants by foliar spray of various micronutrients also enhanced their performance under HTS. The foliar spray of boron and iron was effective in attenuating both HTS and drought stress by securing high water content (Kumari et al., 2019). Selenium acts as an osmo-protectant by improving membrane permeability and activities of anti-oxidant system under HTS (Djanaguiraman et al., 2010). Exogenously applied proline protected carbon metabolism and antioxidant enzymes in chickpea and thereby provided better tolerance to HTS (Kaushal et al., 2011).
The integration of priming memory in plants with different molecular techniques may have a major impact on vigor. Although common stress response mechanisms have been shown to provide cross-tolerance to a variety of abiotic and biotic stresses, different plant species have varied abiotic stress sensitivities and the mechanisms used to protect against stress and the cross-talk between these stresses may be species dependent.
The response of plants to stress depends on their ability to rapidly transmit the perceived signals for initiating appropriate physiological, biochemical and molecular adjustments (Hasanuzzaman et al., 2013; Kollist et al., 2019). The stress signals get amplified through various second messengers like calcium ions (Ca2+) and ROS to regulate physiological and developmental responses. This leads to rapid alterations in the genetic machinery, which is achieved through a network of transcription factors (TFs) and microRNAs (miRNAs) (Kinoshita and Seki, 2014).
The same molecular pathways also conferred memory during priming by triggering an early, appropriate and successful acclimation response (Bruce et al., 2007; Conrath, 2011; Walter et al., 2011; Kollist et al., 2019). The activation of basal cellular processes possibly ensured increased capacity to tolerate future stress and maintain complete fitness of the plant (Li S. et al., 2014). The hypothesis thus proposed, suggested that the rapid stress response phase in plants could serve as an intermediate stage in which generic morphological, biochemical and metabolic changes occurred to effectively counteract the detrimental effects of subsequent stress (Figure 1). These processes might involve some overlap with the responses activated during later stages of stress. Together they help in preventing irreversible damage and pre-inducing the stress response cascades. It is possible that a cellular feedback mechanism from the early stress response of plants provides memory by modifying or altering the later plant response during re-occurring stress events (Kollist et al., 2019).
Figure 1. Schematic representation to show the molecular networks operative during thermo-priming in plants. Plants under HTS perform better when primed with a short exposure to high temperature. Priming leads to changes in the physiological, biochemical and molecular framework. The molecular mechanisms responsible for thermo-tolerance and HTS memory are indicated below. Temperature priming activates the hormonal networks and ROS, which transmit the signals through the Ca2+ dependent pathways and/or MPKs to regulate HTS-responsive gene expression. The Ca2+ ions can be conducted through the CDPK or CaM pathways to activate the transcription factors. The HSFs (red) and hormones responsive transcription factors (blue) bind to their respective cis-elements and promote the transcription of HSPs, antioxidant enzymes (like SOD, POD, CAT), miRNAs and other genes. HSPs inhibit thermal denaturation of cellular proteins through increased chaperone capacity (HSP70/60), prevention of aggregation (sHSP/HSP70) and increased transcription (HSP70/90). miRNAs negatively regulate their targets genes, some of which include HSPs, HSFs and chromatin modifiers to trigger positive or negative feedback loops for retention of high temperature memory. On exposure to stress, primed plants show enhanced thermo-tolerance due to preparedness of the molecular machinery. ROS, reactive oxygen species; HSP, heat shock protein; HSF, heat stress transcription factor; MPK, mitogen-activated protein kinases; CDPK, calcium-dependent protein kinase; CaM, Calmodulin; ABA, abscisic acid; Aux, auxin; SOD, superoxide dismutase; CAT, catalase; POD, Peroxidases; HSE, heat shock sequence element; ABRE, abscisic acid response elements.
There are now several reports from studies on thermo-priming and other stress priming events that point towards the underlying mechanisms. Analysis of transcriptome and proteome of heat primed plants showed that the translation of genes encoding cellular receptors, signal transducers, chaperones, enzymes associated with the process of photosynthesis and sucrose synthesis, transcription factors and HSPs were positively regulated whereas genes encoding enzymes involved in metabolism were negatively regulated (Xin et al., 2016; Zhang et al., 2016b; Xiao et al., 2017; Wang X. et al., 2020). The priming information was transmitted to the progenies in the form of cellular signals, HSPs and structural alterations in protein (Zhang et al., 2016b). Similarly, during excess light stress some transcripts were elevated within seconds and these were found to be essential for light stress acclimation (Suzuki et al., 2015). Priming with pathogens or biotic stress elicitors induced primary metabolism, pattern-recognition receptors, Mitogen-activated protein kinases (MAPKs) and chromatin modifications (Slaughter et al., 2012; Balmer et al., 2015; Conrath et al., 2015). Priming with mild drought or waterlogging in wheat seedlings induced proteins participating in oxidative stress, cell defense, carbon metabolism and photosynthesis (Xiao et al., 2017).
Synchronized phosphorylation and dephosphorylation of proteins play significant roles in priming plant tolerance to both abiotic and biotic stresses. The Receptor Like Kinases (RLKs) and MAPKs constitute two important signaling cascades for eliciting stress responses (Nakagami et al., 2005).
Receptor like kinases make-up one of the most extensive gene families found in plants and include a large subfamily of Leucine-Rich Repeats (LRR) that play essential roles in plant adaptation to diverse stresses (Monaghan and Zipfel, 2012). GbRLK, identified from Gossypium barbadense (cotton), plays a fundamental role in preventing wilt induced by Verticillium dahliae and tolerance to salinity and drought by regulation of stress-responsive genes (Zhao et al., 2013). Not much is, however, known about their role in HTS and thermo-priming responses as temperature-specific sensors or receptors are not yet known in plants (Liu J. et al., 2015). It is hypothesized that temperature-induced changes in membrane rigidity/fluidity might be involved in the perception of the temperature extremes (Guo et al., 2016).
Mitogen-activated protein kinases represent another large and conserved cascade of three sequentially functioning threonine and tyrosine (TXY) kinases, which mainly act downstream to the RLKs in the signaling events (Jonak et al., 2002; Sharma et al., 2020). They play a major role in amplification and transduction of intracellular signals. The MAPK (MPK) is the last enzyme in the cascade. It is phosphorylated and activated by its upstream MAPK kinase (MAPKK, MKK, or MEK) (Conrath, 2011). MAPKK activity is regulated by phosphorylation by the MAPKK kinase (MAPKKK or MEKK), which either directly or indirectly receives the stress signals from receptors or sensors (Takahashi et al., 2007). MAPKs can activate a variety of downstream effectors and TFs to control thousands of genes to ensure proper cellular functions. The involvement of MAPKs in HTS response has been experimentally validated (Sinha et al., 2011).
MPKs, MEKs, and MEKKs are considered as excellent candidates for transducing signals that mediate thermo-priming (Conrath, 2011). In wheat plants, genes encoding MAPK cascade, Ca2+ signaling kinases and other protein kinases were found to be up regulated in primed plants as compared to non-primed plants (Wang M. et al., 2016). In alfalfa, MKK1 and MKK4, which are intermediaries in wound-responsive MAP kinase signaling were also associated with priming for abiotic stress (Wassie et al., 2020). Transcriptome analysis also indicated a role for genes encoding Ca2+-dependent protein kinases and MAPKK in cold priming response in Arabidopsis (Byun et al., 2014). The accumulation of MPK3 and MPK6 was crucial for priming Arabidopsis plants and was related to increased pal1 and pr1 gene activation, ROS homeostasis, cold stress regulation and pathogen signaling (Beckers et al., 2009). Treatment with benzo (1,2,3) thiadiazole-7-carbothioic acid S-methyl ester (BTH), a synthetic equivalent of the SA resulted in the accumulation of transcript for mpk3 and mpk6, as well as the gradual accumulation of MPK3 and MPK6 proteins (Beckers et al., 2009).
Forgetter2 (FGT2), a type 2C protein phosphatase, also plays an important role in HTS memory by maintaining the dynamics of cellular membranes (Castellanos et al., 2020). FGT2 also interacts with Phospholipase D (PLDα2) to produce phosphatidic acid (PA) and trigger lipid signaling for the maintenance of HTS memory. Mutants of fgt2 and pldα2 were defective in thermo-memory when exposed to reoccurring HTS.
In Arabidopsis, suppression of Target of Rapamycin (TOR) kinase is also well known for providing tolerance to different stresses (Bakshi et al., 2017; Wang P. et al., 2018; Dong et al., 2019). TOR overexpression lines were more immune to abiotic stress (Bakshi et al., 2017) but showed higher susceptibility to bacterial and fungal pathogens (De Vleesschauwer et al., 2018). TOR closely interacted with SnRK1 (Sucrose non-fermenting [SNF] related kinase 1) to regulate plant development and growth (Wang P. et al., 2018). It was demonstrated that in unstressed plants, TOR phosphorylated the ABA receptor to repress the ABA signaling pathway and prevent activation of stress responses. Whereas, ABA-dependent repression of TOR and SnRK1 supported plant survival under extreme hypoxia, starvation and darkness (Baena-González and Sheen, 2008). The SnRK2 isoform, SnRK2.10, is implicated in osmotic stress response independent of ABA. SNRK2.10 mutant plants showed increased sensitivity to dehydration stress with respect to wild type plants. The target proteins of this kinase included dehydrins, ERD10 and ERD14, which are also induced during salinity stress (Maszkowska et al., 2019).
Ca2+-sensing protein kinases (CPKS) or kinases interacting with Ca2+ sensors (CIPKs) and Ca2+ dependent protein kinases (CDPKs) are also associated with conferring stress resistance (Drerup et al., 2013; Mittler and Blumwald, 2015; Kudla et al., 2018). It was shown that exposure of apple trees to salt stress induced MdCIPK13, which phosphorylated to activate a sugar transporter, MdSUD2.2. The resulting sucrose accumulation provided the plants with protection from desiccation by increasing the osmotic pressure (Ma et al., 2019). The CDPKs take up a “primed conformation” as a first response to priming stimulus but their complete activation is possible upon exposure to subsequent harsh stress (Hake and Romeis, 2019).
Superoxide (O2–), hydrogen peroxide (H2O2) and hydroxyl radicals (OH–) are the most prominent ROS in early reaction of plants to many types of stresses. The burst of ROS production under abiotic stresses is usually analogous with the depression of photosynthesis (Kurepin et al., 2015). Plants have both enzyme dependent and non-enzyme dependent pathways for scavenging and detoxifying ROS. The common ROS-scavenging enzymes are superoxide dismutase (SOD), ascorbate peroxidase (APX), catalase (CAT), glutathione peroxidase (GPX), peroxiredoxin (PrxR) and glutathione reductase (GR). The major non-enzymatic antioxidants are ascorbic acid (ASA), reduced glutathione (GSH), carotenoids and flavonoids (Mittler et al., 2004).
Thermo-primed wheat plants produced significantly less amount of O2– as compared to non-primed plants (Wang et al., 2014a). Low ROS rates are generally regarded as necessary secondary messengers for cellular metabolism. Accumulation of ROS likely has diverse functions in phytohormone biosynthesis, transport and signaling so it may affect the plant responses mediated by the hormones during priming (Xia et al., 2015). It also plays an important role in cross-tolerance to both abiotic and biotic stress by eliciting the respiratory burst oxidase homologs (RBOHs) and regulation of ABA signals (Xia et al., 2014). However, high ROS levels result in lipid peroxidation, degradation of pigments, DNA damage and carbohydrate oxidation eventually resulting in programmed cell death (Suzuki et al., 2012). Cold priming and drought priming were shown to individually increase SOD, APX and GR activities to shield photosynthetic machinery and protect the cellular membranes (Thomashow, 1999; Wang et al., 2014b).
H2O2 can act as a second messenger and play diverse roles in stress priming and cross-tolerance to multiple abiotic stressors (Suzuki et al., 2012; Hossain et al., 2015; Mittler and Blumwald, 2015). The ABA-dependent accumulation of H2O2 could stimulate the expression of cat1 gene (Guan et al., 2000). Earlier investigations have demonstrated that exogenous application of 10 mM H2O2 solution could prime maize leaves to confer tolerance to salt stress by modulating various cellular processes associated with high antioxidant enzyme activity and increasing content of soluble proteins and carbohydrates (Gondim et al., 2013). Priming with H2O2 improved photosynthesis in leaves exposed to HTS in cucumber and salt stress in maize by enhancing the activity of carbon fixation enzyme, protection of chloroplasts and modulation of metabolites (Khan et al., 2018; dos Santos Araújo et al., 2021). It was shown that plants treated with brassinosteroid (BR) exhibited increased NADPH oxidase activity and enhanced the levels of H2O2 in cucumber apoplasts. These plants also showed resistance to CMV and tolerance to photo-oxidation and low temperature stress (Xia et al., 2009).
The ROS pathway interacts with Methylglyoxal (MG), a potentially toxic compound for plant cells that is produced rapidly as a result of abiotic stress. MG is primarily a by-product of glycolysis but it can also be produced during photosynthesis and metabolism of protein and lipids (Hoque et al., 2016). Excessive cellular accumulation of MG leads to protein glycation resulting in their degradation, endoreduplication and increased DNA strand breaks, sister chromatic exchange and point mutations (Kaur et al., 2014; Hoque et al., 2016). It has been shown that the application of exogenous GSH reduces MG levels via the glyoxalase pathway (Yadav et al., 2005). At low concentrations, MG was found to function as a signal molecule in plants (Li, 2016) and it was associated with the regulation of stomatal movement. MG can cross-talk with ROS and Ca2+ to activate MAPK and regulate the expression of stress tolerance-associated genes, rd29b and rab18 in an ABA-dependent manner (Hoque et al., 2012, 2016). Exogenous application of MG could improve wheat seed germination and seedling establishment under salt and cadmium stresses (Li, 2016; Li X. D. et al., 2017; Li et al., 2017a,b). Recently, MG was reported to act as a HTS priming agent in maize seedlings by inducing AsA-GSH cycle and the ROS-scavenging system (Majláth et al., 2020).
Co-activation of the glyoxalase and ROS-antioxidant pathways is an important feature of abiotic stress and cross-stress tolerances in plants (Yadav et al., 2005; Upadhyaya et al., 2011). It was observed that a short spell of cold shock (6°C, 5.5 h) or heat shock (42°C, 5 h) promoted cross-tolerance to drought and salt stresses in mustard (Brassica campestris L.) seedlings, due to the induction of cellular detoxification of ROS and MG (Hossain et al., 2013a,b). The thermo-priming response involved both ROS and MG pathways, which were implicated in promoting cross-tolerance to HTS, cold stress, drought stress, salt stress and heavy metals in rice (Hsu and Kao, 2007), pea (Streb et al., 2008), maize (Gong et al., 2001), wheat (Lei et al., 2005), tomato (Zhang et al., 2013b), and cucumber (Kang and Saltveit, 2001).
The abiotic stresses also triggered the formation of nitric oxide (NO), which disturbed the redox potential of the cell by affecting the antioxidant and MG, detoxification systems. NO is also involved in the post-translational S-nitrosylation and nitration of target proteins. The exogenous application of NO and hydrogen sulfide (H2S) could induce a primed state which protected plants from the negative effects of salt stress (Antoniou et al., 2020) and low-temperature stress (Amooaghaie and Nikzad, 2013) but their role in thermo-priming has not been elucidated so far. The molecular oxygen and/or O2– anion interact with NO to yield reactive nitrogen species (RNS). These molecules act together to transduce signals during stressed conditions and maintain redox equilibrium inside the cell (Suzuki et al., 2012). The redox hub formed by interaction between ROS, RNS and reactive sulphur species (RSS) is referred to as RONSS (reactive oxygen, nitrogen and sulfur species) and is considered essential for priming strategies to enhance cross-tolerance (Terrile et al., 2020). The RONSS primed state induced translation of a pool of transcripts inclusive of those coding for antioxidant enzymes, osmo-protectant proteins and polyamine biosynthesis.
One of the earliest indicators of the HTS response is the formation of heat shock proteins (HSPs). The HSPs act as molecular chaperones and are important for maintaining the structure and function of proteins, removing potentially toxic polypeptides and restoring protein homeostasis (Kotak et al., 2007; Timperio et al., 2008). They prevent the aggregation of non-native proteins by facilitating their refolding into their native conformation thereby minimizing toxicity of unfolded or denatured proteins during stress.
The HSPs are also associated with the maintenance of acquired thermo-tolerance (Xue et al., 2014). They are classified according to their molecular weight and functions into five broad families, namely HSP100, HSP90, HSP70, HSP60 and small HSP (sHSPs). The transcription of HSPs is stringently controlled by different members of HTS transcription factors (HSFs). The HSP90 and HSP70 represent the major families of HSPs and their activities are modulated by stress-induced HOP (Hsp70-Hsp90 organizing protein) protein (Carrigan et al., 2006). HSPs and HOP proteins were highly expressed in drought or thermo-primed plants, which helped in improving grain filling during HTS in wheat (Wang et al., 2014b; Zhang et al., 2016b) and rice (Kushawaha et al., 2021).
Under normal conditions, the HSP90.1 bound with ROF1 (rotamase FKBP 1), a member of the FKBP family, to form a cytoplasmic protein complex. Upon exposure to HTS, HSFA2 binds to HSP90.1-ROF1 and the resulting complex translocates to the nucleus, where it putatively functions to enhance the transcriptional activity of HSFA2 and maintain the expression of hsp genes regulated by it during HTS recovery (Meiri and Breiman, 2009). Accordingly, Arabidopsis plants overexpressing rof1 displayed improved HTS memory while rof1 mutant plants had impaired memory response. It was recently demonstrated that during the recovery phase NBR1 (Next to BRCA1) interacts with HSP90.1-ROF1 and facilitates their breakdown by autophagy to repress the response to HTS. Thus, HSP90 has a vital function in controlling of recovery from HTS and resetting the cellular memory of HTS in Arabidopsis. Loss-of-function mutants of NBR1 showed a stronger HTS memory (Thirumalaikumar et al., 2021). The HSP90 also displays hormonal cross talk by inducing TFs like ARFs and reducing the accumulation of transcriptional repressors like Aux/IAAs concentration (Wang R. et al., 2016; Watanabe et al., 2016). Studies in Arabidopsis and rice have indicated that HSP101-HSA32 interaction provides another conserved positive feedback loop during thermo-memory. Continuous accumulation of HSP101 during thermo-memory phase results in high abundance of HSA32. The HSFA32 in turn increases stability of HSP101 by retarding its degradation.
Several small HSPs like sHSP17.6II, sHSP22, sHSP18.2 are also associated with HTS memory. The Arabidopsis Hikeshi-Like Protein1 (HLP1) interacts with HSP70 to provide thermo-tolerance in plants (Koizumi et al., 2014). Induction of Heat Stress Associated 32 (hsa32) gene by HSP101 is necessary for thermo-tolerance and HTS memory preservation (Wu et al., 2013). HSA32 works in tandem with Brushy1/Tonsoku/Mgoun3 (BRU1/TSK/MGO3) which is necessary for the sustained activation of HTS memory genes by inducing changes in chromatin structure (Suzuki et al., 2004; Takeda et al., 2004; Brzezinka et al., 2019).
Another interesting mode of biochemically reproducible memory is provided by sustainable changes in the protein conformation and function of prion domain proteins (PrD). These proteins are ubiquitously present in fungi, mammals and plants (Shorter and Lindquist, 2005; Chakrabortee et al., 2016). The PrDs were shown to switch between non-aggregated states and higher-order functional oligomers. The highly ordered aggregates acted as self-replicating entities that had the ability to propagate and could be transmitted to next generations. These characteristics enabled them to bestow stable alterations in biological states, which is important in molecular biology (Newby and Lindquist, 2013), but their significance in plant stress and memory remains largely unknown. A recent report has identified candidate PrDs in approximately 500 plant proteins using computational algorithms and predicted their diverse functional roles in stress and developmental responses including flowering time and thermo-sensory responsiveness (Garai et al., 2021). Transition from vegetative to reproductive stage also involves memorizing and integrating previously encountered environmental conditions. It was reported that Luminidependens (LD) proteins that are involved in regulating the timing of flowering in Arabidopsis behaved as prion-like conformational switch (Lee et al., 1994; Chakrabortee et al., 2016). The LD may be responsible for memorizing and assimilating the ambient signals required to control flower timing. Recently, an Arabidopsis PrD, ELF3, was found to be associated with thermo-sensory response (Jung et al., 2020).
Response to stress results in alterations in gene expression and the foremost amongst them involve activation of transcription, stabilization of mRNA and synthesis of new proteins. The genes can be grouped to differentiate those regulating the “non-memory” response from the group of genes that delineate the “transcriptional memory” (Ding et al., 2013). This became evident from whole-genome transcriptome studies in Arabidopsis plants exposed to dehydration stress. Single exposure to stress identified >6500 differentially expressed genes, while upon repeated exposure, 4500 genes responded to each stimulus in a same way while 1963 genes generated considerably varied levels of transcripts each time.
Analysis of transcriptional changes, in Arabidopsis thaliana and Zea mays plants, in response to multiple stimuli indicated that transcriptional memory was based on an evolutionarily conserved mechanism that could discriminate between single and repeated stresses. The memory genes could induce or alter mRNA synthesis resulting in cellular changes and/or interactions between overlapping signaling pathways (Ding et al., 2014). These genes produced enzymes, osmolytes, dehydrins and chaperones, which were involved in cellular detoxification, protection and damage-repair. In Arabidopsis, stimuli like touch, wind, wounding, rain or transition of plants from light into darkness caused the translation of tch (touch induced) genes (Chehab et al., 2012). One of the members of this gene family was identified as a calmodulin homolog that could be involved in Ca2+ signaling (Chehab et al., 2009).
Transcriptome studies on stress challenged plant tissues identified changes in the transcripts coding for different TFs. Several genes from the MYB, NAC, HSFs, WRKY ethylene response factors (ERFs or AP2), and zinc finger (ZNF) super families have been shown to provide cross-tolerance to abiotic and biotic stressors in plants.
The responses to HTS and thermo-priming are transduced through the MAP kinase pathway to induce the translation of HSFs, which are prime regulators of plant response (Zhang et al., 2016b). HSFs are a conserved family of TFs that bind directly to heat shock elements (HSEs) to control HTS-related gene expression (Ohama et al., 2017). The HSEs consist of tandem inverted repeats of the pentameric consensus sequence nGAAn (nTTCnnGAAnnTTCn) and the AGGGG motifs (Swindell et al., 2007; von Koskull-Döring et al., 2007). Variable number of HSFs are observed in plants, viz. rice (25 members), wheat (56 members), Arabidopsis (21 members), soybean (52 members), tomato (26 members), maize (30 members), carrot (35 members), pepper (25 members), cotton (40 members) etc. (Scharf et al., 2012; Xue et al., 2014; Guo et al., 2016; Yang X. et al., 2016; Li et al., 2019). HSFs are divided into three structural classes: A, B and C, with class A serving as the principal activator of thermo-tolerance genes (Guo et al., 2008; Scharf et al., 2012).
Among the Class A HSF, the HSFA1 subfamily participates as early response genes for activating the other HTS response factors, for instance HSFA2 and DREB2A (Guo et al., 2008; Liu et al., 2011a; Scharf et al., 2012). Recent investigations have shown that HSFA1a directly senses HTS and gets activated through modifications in its redox state (Liu et al., 2013). HSFA1a is involved in the expression of genes coding for HLP1, dehydration-responsive element-binding protein 2a (DREB2a), HSFA7a, HSFBs, HSFA2 and multi-protein binding factor 1C (MBF1C) by binding to HSEs present in their upstream regions. HSFA2 is highly stimulated during HTS and is crucial for growth as well as maintaining the thermo-tolerance response during recovery period. It is regulated by three other TFs, HSFA1b, HSFA1d and HSFA1e (Yeh et al., 2012; Li X. D. et al., 2017). Thermo-priming-induced HSFA2 transiently binds at the promoter region of HTS memory genes, thereby facilitating di- and tri-methylation of histone H3 (Lämke et al., 2016). This chromatin modification triggers hyper-activation of transcription at these loci under subsequent HTS. HSFA3 expression is regulated by DREB2a and DREB2c and is essential for thermo-tolerance (Yoshida et al., 2011; Sato et al., 2014). In tomato, HsfA3 shows strong phosphorylation by HTS activated MAP kinases (Sinha et al., 2011). Other HSFs like HSFA4a and HSFA8 are also involved in sensing the ROS and induction of antioxidant system in plants (Qu et al., 2013). HSFA9 is exclusively expressed in late seed development stages.
The Class B HSFs, such as HSFB1 and HSFB2b primarily act as transcriptional repressors to inhibit HTS-induced gene expression (Ikeda et al., 2011). They act as co-activator in combination with class-A HSFs (Ikeda et al., 2011; Fragkostefanakis et al., 2015). The role of Class C HSFs in HTS is not clear. TF-HSFC1a was found to be negatively controlled by early HTS treatment in rice (Mittal et al., 2009), while overexpression of HSFC2a-B in wheat increased the expression of heat associated genes, resulting in improved thermo-tolerance (Hu et al., 2018).
NAC binding sites are known to be present in the promoters of several HSFs e.g., HSFA1b, HSFA6b, HSFA7a, and HSFC1 (Guo et al., 2015). The NAC TF family is found in plants and has been linked to the regulation of development and a stress related response. Overexpression of OsNAC6 improved tolerance of rice plants to drought and salt by activation of many stress-responsive genes (Nakashima et al., 2007). Heat priming elevated the expression of ATAF1 but its level again decreased during thermo recovery phase (Alshareef et al., 2019). JUB1 and ANAC019, members of NAC-TF family, were reported to regulate HTS and heat memory. JUB1 was reported to show thermo-memory related expression, which was similar to that of HSFA2 and HSFA32 (Shahnejat-Bushehri et al., 2012).
The stress induced increase in ABA, signaled the activation of R2R3-MYB TF, TaPIMP1 in wheat. This increased resistance to the fungus, Bipolaris sorokiniana and tolerance to drought stress (Zhang et al., 2012). The MYB-TFs also conferred cross tolerance to abiotic stimuli such as salinity and drought in transgenic tobacco by increasing the activity of phenylalanine ammonia lyase (PAL) and SOD (Liu et al., 2011b).
The expressions of ERF/AP2-TFs and ZNF proteins were induced in cold-primed plants indicating their crucial roles in priming plant response to cold tolerance (Byun et al., 2014). In cereals, long-term exposure to cold lead to the stable induction of VRN1, a TF related to AP1 (Trevaskis et al., 2003; Yan et al., 2003). This process was accompanied by accumulation of H3K4me at the VRN1 locus (Huan et al., 2018) indicating a possibility of analogous pathway for regulating chromatin in long-term memory of cold in cereals.
Priming by dehydration induced ABA-dependent memory through recruitment of MYC2-TFs, mobilization of mediator complex and accumulation of Ser5P RNA Pol II (Liu and Avramova, 2016). The ABA-dependent expression of a stress inducible OsWRKY45 gene increased tolerance of Arabidopsis to salt and drought stress (Qiu and Yu, 2009). WRKY40 and Zat12, TFs showed a triphasic transient expression pattern during the response to stress. Their expression first peaked as a rapid response within a few seconds of stress (Suzuki et al., 2015), second time after a few minutes and third time after a few hours (Van Aken et al., 2016), indicating that TF pulses control the transcriptome in plants (Davletova et al., 2005).
Genome-wide transcriptome and small RNA analysis has linked the non-coding RNAs to stress response in plants. The stress responsive expressions of several conserved and novel miRNAs have been captured in a variety of plants like rice, tomato, populus, mustard, Arabidopsis, wheat, switch grass, cavendish banana and french beans (Chen L. et al., 2012; Yu et al., 2012; Kruszka et al., 2014; Raghuram et al., 2014; Jyothi and Rai, 2015; Liu W. et al., 2015; Hivrale et al., 2016; Pan et al., 2017). Different miRNAs such a miR156, miR159 and miR160 have been found to play essential roles in response to HTS (Stief et al., 2014). Many miRNAs induce response to HTS and thermo-memory by regulating key TFs and enzymes. For instance, miR159 acts on the GAMYB transcripts, miR396 regulates transcripts of WRKY TFs, miR528 regulates transcripts encoding F-box protein and miR5054 acts on cat transcripts (Giacomelli et al., 2012; Wang Y. et al., 2012). The miRNA168 acts in thermo-tolerance memory by regulating the function of AGO1 in thermo-memory, which generates a negative feedback loop and changes the expression of a larger number of miRNAs (Vaucheret et al., 2006).
It has been shown that miR156 was highly induced after HTS, to down regulate the Squamosa Promoter Binding Protein-Like (spl) TFs. Thermo-priming seems to generate HTS memory in Arabidopsis by repressing the transcription of spl2 and spl11 transcripts by inducing transcription of miR156 (Yu et al., 2012; Stief et al., 2014). During recovery from HTS, the spl levels were restored. The HTS also induced a positive feedback loop consisting of HSFA regulated miR398. The levels of miR398 were increased in response to HTS for down regulating the copper/zinc superoxide dismutase gene (Guan et al., 2013). This lead to increased levels of ROS, which in turn induced the HSFs and HSPs.
Another study showed that miR167 regulated DNA methylation process in response to HTS (Wu et al., 2010; Sanchez and Paszkowski, 2014; Naydenov et al., 2015), indicating a possible role for imprinting in HTS memory. The expression of osa-miR531 is regulated under different abiotic stress conditions including short duration HTS by signaling through MAPKs (Raghuram et al., 2014). HTS was shown to enhance the synthesis of vitamin E in chloroplasts, which up regulated miR398 biogenesis by retrograde signaling and provided protection to the Arabidopsis plants (Fang et al., 2019). A role for tRNA-derived siRNA fragments in trans-generational transmission of thermo-priming memory has recently been reported in durum wheat (Liu, 2020).
Our group has shown that miR169:NFY module may play a crucial role in integrating stress memory induced during HTS priming with light regulated development (Khan et al., 2017; Kushawaha et al., 2019). NGS analysis of rice transcriptome showed that levels of several miRNAs, HSPs, and HSFs were dis-regulated by thermo-priming (Kushawaha et al., 2021). Several heat responsive miRNAs like osa-miR531a, osa-miR5149, osa-miR168a-5p, osa-miR1846d-5p, osa-miR5077, osa-miR156b-3p, osa-miR167e-3p were identified that acted on respective HSF or HSP targets to differentially alter gene expression (Kushawaha et al., 2021). The other differentially expressed transcripts were mainly associated with redox pathway, protein phosphorylations and regulation of transcription.
Epigenetic regulation resulting in chromatin modification is a common mechanism used to regulate transcriptional activity in eukaryotes. Chromatin is composed of negatively charged DNA associated with positively charged histones that are arranged as nucleosomes. Each nucleosome consists of two molecules of each four histones (H2A, H2B, H3 and H4) forming an octamer, which is wrapped by DNA. The nucleosomes are connected to each other by a DNA strand linked with histone H1 (Kornberg, 1974; Thoma et al., 1979).
The DNA and histones are subjected to covalent modifications such as methylation, acetylation, ubiquitination and poly-ADP ribosylation during gene regulation. DNA methylation and histone modifications are two main mediators of epigenetic regulation. DNA methylation is a definitive chemical process in which a methyl group is added to DNA. It usually occurs when a cytosine (C) is linked to guanine (G) through the phosphate (p) linkage resulting in a CpG site. The methylation reaction is catalyzed by a large family of DNA-methyltransferase (DNMTs) and distinct enzymes perform de novo DNA methylation and maintenance DNA methylation (Kotkar and Giri, 2020).
The epigenetic modifications can be inherited via mitotic and meiotic cell divisions and act to synchronize the changes in gene expression, which form the basis of memory responses (Eichten et al., 2014; Kinoshita and Seki, 2014; Avramova, 2015; Crisp et al., 2016; Liu and Avramova, 2016). Initial indications for involvement of chromatin modification in priming response came from studies in which plants primed with benzothiadiazole showed increased levels of histone methylation (Jaskiewicz et al., 2011). The bi- and tri-methylation of lysine 4 in histone 3 (H3K4me2 and H3K4me3, respectively) is correlated with active transcription of genes (Ruthenburg et al., 2007; Jaskiewicz et al., 2011) and were recognized as potential abiotic stress memory markers.
Response to HTS comprises of two components, induction of stress memory-associated genes and differential expression of HTS regulated genes. Both components are related with increased levels of H3K4me3 and H3K4me2 and are dependent on functional TFs (Lämke et al., 2016; Liu Y. et al., 2019). An increase in H3K4me2 and H3K4me3 was observed after heat priming in Arabidopsis (Conrath et al., 2015). H3K4me2/3 accumulation was also seen in Arabidopsis plants that had been primed with JA (Liu and Avramova, 2016).
Stress related memory might particularly develop when the plant is recovering from stress. This has been best exemplified by studies on vernalization leading to development of memory for cold stress. During the cold phase, repressive chromatin marks were stored at the nucleation regions of FLC, but epigenetic modifications were triggered after reversing to warm conditions. During the recovery from cold signal, the Polycomb Repressive Complex 2 (PHD-PRC2) was deregulated across the entire FLC locus and H3K27me3 increased remarkably throughout the entire gene to achieve epigenetic silencing (De Lucia et al., 2008; Angel et al., 2011).
Modification of chromatin provided potential memory for stress during priming events by maintaining the basal transcriptional machinery at the promoters of these genes to facilitate their rapid or strong expression in response to recurring stress (Conrath et al., 2015). Increase in H3K4me3 was retained as a memory on the stress responsive genes during stress, while H3K4me3 accumulated at primed defense genes before their transcription (Conrath, 2011; Jaskiewicz et al., 2011). Repeated exposures of Arabidopsis plants to mild drought like conditions increased the levels of H3K4me3 and stalled RNA polymerase II at RD29B and RAB18 loci allowing their rapid activation during the stressed phase (Ding et al., 2012). Increased H3K4me3 levels also caused increase in the occupancy of TATA-binding protein (TBP), a key step in the formation of the pre-initiation complex (PIC), at the promoters of memory genes (Liu and Avramova, 2016). HTS memory was also maintained by H3K27me demethylases, Relative of Early Flowering 6 (REF6) and the Jumonji (JMJ30) proteins by controlling the level of histone modification of HSP22 and HSP17.6 genes (Yamaguchi and Ito, 2021).
Some stress-induced TFs were reported to function as prime components of transcriptional memory and are probably involved in recruiting specific chromatin-regulatory proteins to the target loci. For example, bZIP28/60 TFs were stimulated by the stress induced Unfolded Protein Response (UPR) protein (Liu and Howell, 2010) to recruit the COMPASS-like complex (Jiang et al., 2011) for initiating the accumulation of H3K4me during HTS (Song et al., 2015). It was shown that the same mechanism triggered the deposition of H3K4me3 at loci of dehydration memory genes. In yeast, both COMPASS and Mediator complexes were required for the H3K4me accumulation and transcriptional memory of the gene encoding inositol-1-phosphate synthase (Light et al., 2010, 2013; D’Urso et al., 2016). The composition of these protein complexes changed under memory and non-memory situations to allow differential regulation of transcription (D’Urso et al., 2016).
HSFA2 and HSFA1 are known to function in somatic HTS memory (Charng et al., 2007; Lämke et al., 2016; Liu Y. et al., 2019). Thermo-priming induced accumulation of H3K4me2 and H3K4me3 to generate HTS memory was dependent on HSFA2 (Lämke et al., 2016). Binding of HSFA2 occurred during first few hours of heat shock but the enhanced H3K4me3 and H3K4me2 levels persisted even after HSFA2 association with the gene had declined. The HSFA2 acts in trimeric complexes along with other HSFs like HSFA3 [reviewed in Scharf et al. (2012)], indicating that heat memory might be specific to few TF complexes and not individual TFs. HSEs were found to be the sites of active chromatin regions associated with histone (H3K9 and H3K14) acetylation and H3K4me3 (Guertin and Lis, 2010). Transcriptional activation of few HSF and HSP genes in Arabidopsis by HSF1A/B occurred through deposition of H3K56 acetylation under HTS (Weng et al., 2014).
The trans-generational HTS memory involves a heritable positive feedback loop consisting of REF6 and HSFA2 (Liu J. et al., 2019). The HSFA2 and REF6 promote the expression of each other to continuously maintain the active state of HSFA2. The HSFA2 in turn activates the expression of suppressor of gene silencing 3 (sgs3) interacting protein 1 (sgip1), an E3 ligase, to mediate SGS3 degradation. This represses biosynthesis of trans-acting siRNA (tasiRNA) and results in the release of its target, Heat-Induced Tas1 Target 5 (htt5) to promote early flowering in the progeny plants. Subsequent studies demonstrated the role of FGT3 and HSFA3 in inducing transcription of memory-related genes during recovery from HTS.
Nucleosome remodeling has also been related to HTS induced memory. Arabidopsis FGT1 protein maintains low nucleosome occupancy at thermo-memory related genes like hsa32, hsp22.0, hsp18.2, and hsp101. FGT1 acts by interacting with proteins belonging to the chromatin remodeling complexes such as ATP-dependent Switch/Sucrose Non Fermentable (SWI/SNF), Imitation Switch family (ISWI) or Brahma (BRM), Chromo-domain Helicase DNA-binding (CHD) and INO80 complex ATPase subunit (INO80) to provide thermo-memory response (Brzezinka et al., 2016; Liu H. et al., 2021).
The trans-generational priming responses include both inheritable (DNA and chromatin structure change) and non-inheritable (metabolites, proteins or mRNA in the seeds) components (Boyko and Kovalchuk, 2011). The enzyme lysine-specific histone demethylase 1, which is involved in histone demethylation and epigenetic modification, was up regulated in the progenies of the primed plants. This suggested that the epigenetic modification could be involved in the trans-generational stress memory resulting in enhanced tolerance to stress.
The study of mechanism behind maintenance of epigenetic modifications over a longer period of time led to the discovery of trans factors that mediated faithful copying of the chromatin conformation through replication. One such protein, BRU, is necessary for the sustained activation of HTS memory genes (Suzuki et al., 2004; Takeda et al., 2004; Brzezinka et al., 2019). It is also essential to sustain the transcription of HTS responsive genes hsa32, apx2, hsp22.0, and hsp21 (Stief et al., 2014). BRU1 has been implicated in the inheritance of chromatin states in transcriptional silencing and the DNA damage response across DNA replication and cell division.
Recently, a BRU1 orthologue from mammals was found to bind to single-stranded DNA and newly incorporated nucleosomes after replication (Huang et al., 2018). The histone chaperone, Chromatin Assembly Factor-1 (CAF-1) plays a key role in depositing histone H3H4 tetramers into newly replicated DNA. Mutants of caf-1 showed a constitutive priming response against pathogens and increased H3K4me3 at primed defense-response genes. This suggested that regulated deposition of histone tetramers may be essential for inheritance of priming responses (Mozgova et al., 2015).
DNA methylation is another mechanism of epigenetic modification and is shown to be a dynamic regulatory mechanism of defense genes and stress priming. Single base-resolution DNA methylome profiling of cold primed tissues revealed the global loss of DNA methylation with increase in some locally hypermethylated sites. The de novo methylation of cytosine residues (CG, CxG, Cxx) in plant DNA can be triggered by small interfering RNAs (siRNA). The onset of RNA-directed DNA methylation begins with the formation of siRNAs. The siRNAs may be produced through the Polymerase IV (Pol IV) pathway and loaded in Argonaute 4 (AGO4) complex. Alternatively, the siRNAs can be generated through Pol I-RNA Dependent RNA Polymerase 6 (RDR6) pathway and are loaded into AGO6 complex. The AGO4/6 complex, interact with Pol V to recruit a Nuclear RNA Polymerase E1 (NRPE1) complex, which subsequently establishes DNA methylation through Domains Rearranged Methyltransferase2 (DRM2). During cell divisions, the maintenance of known DNA methylation marks in CG and CxG are catalyzed by Methyltransferase1 (MET1) and Chromomethylase3 (CMT3), respectively (Ramirez-Prado et al., 2018; Zhang H. et al., 2018).
The classic growth promoting phytohormones viz. auxin, cytokinin (CK), strigolactones (SLs) and BR and the stress related hormones such as ABA, JA and SA, play an essential role in orchestrating protection in response high temperature and other abiotic stresses (Tang et al., 2008; Verma et al., 2016; Kumar et al., 2019; Li et al., 2021). Phytohormones act by positively regulating stress responsive gene expression, nutrient allocation, photosynthetic activity, osmolyte biosynthesis and antioxidant metabolism (Alam et al., 2013; Peng et al., 2014; Qiu et al., 2014; Shi et al., 2014; Jin and Pei, 2015; Cheng et al., 2016). Exogenous application of ABA, ethylene and SA or enhancing their endogenous levels helped to significantly overcome HTS induced damage and improved thermo-tolerance response (Table 2). Combined application of gibberellins and ABA in rice lead to increase in pollen germination and vigor. The phytohormones acted by improving anti-oxidant activity and membrane stability (Chhabra et al., 2009; Song et al., 2012). The role of ethylene in reducing HTS by reducing oxidative damage has been studied at seedling stage in Arabidopsis and rice (Larkindale and Knight, 2002; Wu and Yang, 2019).
The primary stress hormone, ABA, modulates plant responses to multifarious environmental stresses. The involvement of ABA in response to HTS and thermo-priming has emerged through many different studies (Larkindale and Knight, 2002; Zhou et al., 2014; Li et al., 2021). ABA-mediated ROS accumulation in guard cells caused closure of stomata (Kwak et al., 2003) and helped to decrease loss of water. This resulted in cross-tolerance to temperature (heat or cold) and drought stresses. The ABA signals activated the expression of several stress responsive genes such as dehydration-responsive 22 (rd22), pr1a.205, thaumatin-like protein 4 (tlp4), and myb in wheat resulting in increased drought tolerance and fungus resistance (Zhang et al., 2012). ABA enhanced tolerance to HTS by up regulation of HSFs and HSPs, increase in sugar metabolism and reduction in electrolyte leakage (Larkindale and Knight, 2002; Wind et al., 2010; Wang X. et al., 2017; Rezaul et al., 2019). Arabidopsis plants treated with inhibitor of ABA biosynthesis showed impaired response to HTS and reduction in ROS levels (Larkindale et al., 2005). HTS also evoked a rapid and transient expression of endogenous ABA that in turn increased the levels of ROS like H2O2 thereby augmenting thermo-tolerance (Larkindale et al., 2005).
Interaction of ABA and ROS is considered as one of the prime factors for the acclimation of plants exposed simultaneously to drought and salt stresses (Suzuki et al., 2016; Zandalinas et al., 2016). Mutants in ABA biosynthesis or signaling components displayed suppressed H2O2 accumulation and increased HTS sensitivity in plants (Larkindale et al., 2005). Loss-of-function mutation in the ABA induced NADPH oxidases, also known as RBOHs exhibited impaired HTS tolerance as measured by decrease in seed survival and germination capacities (Larkindale et al., 2005; Silva-Correia et al., 2014). Heat- or cold- priming were also found to increase the endogenous concentration of ABA, SA and H2O2 (Liu et al., 2006; Wan et al., 2009). Cold priming induced frost tolerance in winter and spring wheat (Triticum monococcum) was associated with greater accumulation of ABA and dehydrins (Vanková et al., 2014). Priming with drought induced cold stress tolerance was associated with ABA and ROS accumulations in the leaf tissues of wheat (Li X. et al., 2015).
The biotic stress-induced hormones, JA and SA, can prime transcription by stimulating the expression of defense genes after attack by the pests or pathogens (Beckers and Conrath, 2007; Chen R. et al., 2012). The basic helix–loop–helix (bHLH) TF, MYC2, is an important part of the JA signaling pathway and its activity is regulated by binding of repressor (JAZs) and co-repressors (TPL or related TRPs) proteins (Chini et al., 2007). The JA-induced proteolytic degradation of JAZ repressors allows MYC2 to bind with promoters. It then interacts with MED25 subunit to facilitate the assembly of Mediator complex (Elfving et al., 2011; Çevik et al., 2012), which in turn recruits the pre-initiation complex (PIC) to activate transcription. The cross-talk between JA- and ABA-mediated pathways, has been well established (Wasternack and Hause, 2013; de Ollas et al., 2015). Both JA and ABA mediate induction of drought-inducible genes in response to dehydration stress at the initial stage. However, in subsequent dehydration stress, ABA produced during the initial stress reduces the production of the MYC2 to inhibit biosynthesis of JA (Avramova, 2019).
The role of SA has been discussed in numerous HTS related studies in plants. Exogenous application of SA or its functional synthetic analogs like BTH or INA (2,6-dichloroisonicotinic acid) and the non-protein amino acid, BABA (b-amino butyric acid), prior to HTS were shown to enhance plant biomass, height and photosynthetic efficiency (Kauss et al., 1992; Oostendorp et al., 2001). Priming of plants with BABA also induced SA signals and activated the defense response (Ton et al., 2005). SA enhanced the activities of antioxidant enzymes like SOD, CAT and peroxidases to scavenge ROS and increased enzyme activities required for proline biosynthesis as an adaptive strategy to tolerate HTS. It also helped in reducing membrane damage caused by HTS and decreased the proline-metabolizing enzymes (Verbruggen and Hermans, 2008; Szabados and Savouré, 2010; Lv et al., 2011). Application of SA at seedling stage in plants such as maize, potato, wheat, cotton, pigeon pea, Arabidopsis, mung bean, grape and alfalfa lead to increase in antioxidant enzymes, photosynthetic activity and proline accumulation which reduced cellular damage. It also enhanced basal thermo-tolerance by up regulating HSP expression in some cases (Clarke et al., 2004; Saleh et al., 2007; Khan et al., 2013; Wang et al., 2014a; Hameed and Ali, 2016; Khanna et al., 2016; Makarova et al., 2018; Jahan et al., 2019; Kaur et al., 2019; Wassie et al., 2020).
The Set Domain Group 8 (SDG8) proteins which represent the major H3K36 di- and tri- methyltransferase in Arabidopsis have been implicated in the JA- and SA-mediated plant defense (Ding and Wang, 2015; Li Y. et al., 2015). SDG8 is essential for H3K36me3 for expression of the R-gene, lazarus 5 (LAZ5), in response to fungal infection (Berr et al., 2010; Palma et al., 2010). This memory can be transmitted to the progeny plants and the off-springs were primed for enhanced activation of the WRKY6, PR1 and WRKY53 genes that play roles in immunity (Slaughter et al., 2012).
Warm ambient temperature signals can promote a series of structural changes in plants like elongation, growth and flowering, which are collectively termed as thermo-morphogenesis (Quint et al., 2016) and auxin plays a major role in these processes. At normal growth temperatures, the exogenous application of auxin does not elicit hypocotyl elongation but at higher temperatures the auxin-responsive gene expression causes hypocotyl elongation and leaf hyponasty (Küpers et al., 2020). Auxin also act as thermo-protectant of anthers in barley and rice (Sakata et al., 2010; Oshino et al., 2011; Zhang C. et al., 2018).
The light responsive Phytochrome Interacting Factor 4 (PIF4) and PIF7 are involved in regulating hypocotyl elongation and leaf hyponasty. Recent studies showed that PIFs are inducible by HTS and they interact with at least one of the phytochromes thereby indicating their important role in transducing both light and temperature signals (Choi and Oh, 2016; Pham et al., 2018; Kim et al., 2020). The temperature-induced hypocotyl elongation is delayed by the suppression of PIF4 activity by blue light photoreceptor, Cryptochrome 1 (CRY1) (Ma et al., 2016). Loss-of-function mutants of pif4 and pif7 abrogate HTS mediated thermo-morphogenesis and show drastic reductions in auxin biosynthetic enzymes (aminotransferase, YUCCA and cytochrome P450s) (Sun et al., 2012; Fiorucci et al., 2020).
Several studies have suggested the role of CK in acquisition of thermo-tolerance by inducing higher antioxidant activity and metabolism. When a part of the plant or whole plants were exposed to HTS, a rapid increase in CK was observed which further activated the carbohydrate metabolism and photosynthetic genes (Dobrá et al., 2015). Agrobacterium mediated ectopic transcription of isopentenyltransferase (ipt), which encodes a key enzyme in CK biosynthesis system, resulted in plants that were better adapted to HTS (Skalák et al., 2016). An interesting pattern of expression of interconnected proteins was observed in the chloroplasts, in response to HTS and CK. The adverse effect of HTS on spikelet formation and panicle differentiation is mitigated by CK application (Wu et al., 2017). In Arabidopsis and in crops like maize, wheat and rice, CKs were shown to provide HTS tolerance by reduction in kernel abortion, increase in rate of grain filling, modulating spikelet injury and regulating ROS production (Cheikh and Jones, 1994; Yang D. et al., 2016; Wu et al., 2017; Prerostova et al., 2020).
Brassinosteroids are known to perform surveillance for HTS induced lipid peroxidation, ion leakage and survival rate (Mazorra et al., 2011). Treatment of plants with BR lead to a rise in basic thermo-tolerance due to higher translation of proteins like HSPs, aquaporins, etc. (Dhaubhadel et al., 2002; Sadura et al., 2020). The levels of ROS and expression of antioxidant enzymes also spiked up after the application of exogenous BR under HTS (Nie et al., 2013). However, in tomato seedlings both overproduction and deficiency of BR showed similar response to thermo-tolerance indicating that the process might be independent of BR homeostasis (Mazorra et al., 2011). Later it was shown that Brassinazole-resistant 1 (BZR1), the prime TF in BR signaling, activated PIF4 gene expression to integrate the plant response to different environmental stimuli (Ibañez et al., 2018). BRs also influenced the levels of other phytohormones like ABA, ethylene and SA to regulate oxidative stress under HTS and boost thermo-tolerance (Divi et al., 2010; Kothari and Lachowiec, 2021).
The role of phyto-melatonin is also implicated in governing the response and memory to plant stress (Arnao and Hernández-Ruiz, 2019). Melatonin, a predominantly animal hormone, is present in plants and performs a number of physiological activities including chlorophyll preservation, root and shoot growth, photosynthesis, reduction of oxidative damage and suppression of leaf senescence (Byeon et al., 2012; Tan et al., 2012; Zhang N. et al., 2014; Li X. et al., 2018). Exogenous application of melatonin to roots resulted in its absorption and mobilization in xylem and subsequent accumulation in the leaves (Yoon et al., 2019). Extracellular melatonin enhanced the level of TaSNAT transcripts, which encode a key enzyme in the melatonin biosynthetic pathway and increased the intracellular melatonin levels.
Various reports have also shown the protective effects of phyto-melatonin against biotic and abiotic stress (Li et al., 2012; Li X. et al., 2018; Bajwa et al., 2014; Meng et al., 2014; Shi et al., 2015b). Melatonin is an important master regulator of redox homeostasis in plants (Arnao and Hernández-Ruiz, 2019). It induced the DREB1/CBF TFs AtCBF1, AtCBF2, and AtCBF3 that caused increased tolerance to drought, cold, salt, and Pst-DC3000 (Shi et al., 2015a). Melatonin also up regulated polyamine levels by promoting the synthesis of polyamines from its precursor amino acids, arginine and methionine. In wheat seedlings, Melatonin attenuated the effect of salt stress by preventing polyamine degradation (Ke et al., 2018).
According to Intergovernmental Panel on Climate Change (IPCC, 2018), the unprecedented increase in global mean temperatures has caused an upsurge in the incidence of extreme HTS. Its adverse effects on plant growth, reproduction and yield are proportional to the intensity and duration of high temperatures (Prasad et al., 2017; Ali et al., 2020). The increase in global temperatures accompanied by fluctuations or changes in climate regimes has increased the occurrence of abiotic stresses such as HTS, salinity, drought and flooding as well as biotic stresses such as pathogen infections and pest invasions. These challenges have emerged as major factors limiting crop production yield (Pandey et al., 2017).
High temperature stress results in various impairments including early leaf senescence, accumulation of ROS, reduced germination, decreased rate of photosynthesis, inhibition of root growth, lower seed set, reduced vigor and elevated rates of lipid peroxidation, protein denaturation, spikelet sterility and anther indehiscence (Wahid et al., 2007; Hasanuzzaman et al., 2013; Rodríguez et al., 2015; Narayanan et al., 2016; Iqbal et al., 2017; Ohama et al., 2017; Ali et al., 2020). Sustainable agriculture necessitates the adaptation of strategies to boost plant responses to improve their tolerance to these stresses. Although lot of efforts like conventional breeding, application phytohormones, comparative genetics, etc., have been initiated in this direction but a detailed understanding of the underlying mechanisms is still needed.
Priming has the potential to be developed as a very promising technique for improving the long-term sustainability of agriculture and increasing crop yields in the present context of global warming and climate change. Plants can be thermo-primed by moderately high temperatures to sustain extreme temperatures that are otherwise fatal to an unadapted plant (Bäurle, 2016). In our lab, experiments on thermo-priming mature rice before and after flowering, showed that priming allows the crops to tolerate HTS and help in prevention of seed loss and increase seed production by sustaining grain filling (Kushawaha et al., 2021). The response may vary from crop to crop depending upon their life cycle, habitat and stress tolerance levels. Therefore comprehensive investigations are required using a broad range of plant species to determine the most efficient and effective stage and duration of priming. The analyses also need to be extended from study of individual stresses to include a mixture of stressors so that plants can be acclimated to tolerate a wide range of temperatures.
Epigenetic regulations, transcript modifications, changes in protein conformations and generation of hormonal or metabolic signals are only a few of the cellular processes that have been studied in response to stress priming in the last decade. Epigenetic alterations resulting in changes in structure and composition of chromatin may play a vital role in stress memory by regulating transcription and/or plant response. The mechanisms overlap with the stress response pathways and often involve activation of epigenetic imprints and feedback loops to initiate faster recruitment of the stress response.
Advance investigations at the chromatin level will be fundamental for understanding the nature of modifications involved in inheriting tolerance to different stresses in the same species. Few features like accumulation of H3K4me may be commonly related to more than one type of stresses, while memory of individual stress may involve specific molecular elements. More research is required to recognize these patterns of stress memory and determine the exact mechanisms by which they are generated. The importance of synchronized gene silencing by miRNAs and other regulatory non-coding RNAs in stress primed plants is a significant question which will require an in-depth analysis. Their role and pathways for integration of priming with stress memory in regulation of plant development and productivity will be an important question to investigate.
It is apparent that our understanding of effects of priming and induced memory of stress in plants is far from clear. The maintenance of memory and its inheritance across cell divisions needs to be investigated. It will be essential to investigate how long the memory phase is maintained and regulated. Further studies are also required to comprehend the precise mechanisms that elicit and reset stress memory. The advancements in various omic-technologies will help in providing better understanding of priming induced cross-talk during stress tolerance in plants. It is evident that increased knowledge of the priming pathways will aid in designing strategies for improving plant performance and seed production. This will not only help them to pre-adapt to changing environmental conditions but also enable them to habituate in new domiciles.
NS-M and SKS conceptualized the idea of the study. AK, VK, and KP prepared the draft. SKS and NS-M edited the manuscript. All authors read and approved the final manuscript.
This research was supported by grants from the SERB (SPF/2021/000198), Government of India. SKS was thankful to SERB, Government of India, for providing Distinguished Fellowship award.
The authors declare that the research was conducted in the absence of any commercial or financial relationships that could be construed as a potential conflict of interest.
All claims expressed in this article are solely those of the authors and do not necessarily represent those of their affiliated organizations, or those of the publisher, the editors and the reviewers. Any product that may be evaluated in this article, or claim that may be made by its manufacturer, is not guaranteed or endorsed by the publisher.
Abd El-Daim, I. A., Bejai, S., and Meijer, J. (2014). Improved heat stress tolerance of wheat seedlings by bacterial seed treatment. Plant Soil 379, 337–350. doi: 10.1007/s11104-014-2063-3
Alam, M. M., Hasanuzzaman, M., Nahar, K., and Fujita, M. (2013). Exogenous salicylic acid ameliorates short-term drought stress in mustard (Brassica juncea L.) seedlings by up-regulating the antioxidant defense and glyoxalase system. Australian J. Crop Sci. 7:1053.
Alexandrov, V. Y. (1956). Cytophysiological and cytoecological investigations of heat resistance of plant cells toward the action of high and low temperature. Q. Rev. Biol. 39, 35–77. doi: 10.1086/404089
Ali, S., Rizwan, M., Arif, M. S., Ahmad, R., Hasanuzzaman, M., Ali, B., et al. (2020). Approaches in enhancing thermotolerance in plants: an updated review. J. Plant Growth Regul. 39, 456–480. doi: 10.1007/s00344-019-09994-x
Alonso-Ramírez, A., Rodríguez, D., Reyes, D., Jiménez, J. A., Nicolás, G., López-Climent, M., et al. (2009). Evidence for a role of gibberellins in salicylic acid-modulated early plant responses to abiotic stress in Arabidopsis seeds. Plant Physiol. 150, 1335–1344. doi: 10.1104/pp.109.139352
Alshareef, N. O., Rey, E., Khoury, H., Tester, M., and Schmöckel, S. M. (2019). Genome wide identification of NAC transcription factors and their role in abiotic stress tolerance in Chenopodium quinoa. BioRxiv [Preprint] BioRxiv: 693093, doi: 10.1101/693093
Amooaghaie, R., and Nikzad, K. (2013). The role of nitric oxide in priming-induced low-temperature tolerance in two genotypes of tomato. Seed Sci. Res. 23, 123–131. doi: 10.1017/S0960258513000068
Anderson, M., and Habiger, J. (2012). Characterization and identification of productivity-associated rhizobacteria in wheat. Appl. Environ. Microbiol. 78, 4434–4446. doi: 10.1128/AEM.07466-11
Angel, A., Song, J., Dean, C., and Howard, M. (2011). A Polycomb-based switch underlying quantitative epigenetic memory. Nature 476, 105–108. doi: 10.1038/nature10241
Antoniou, C., Xenofontos, R., Chatzimichail, G., Christou, A., Kashfi, K., and Fotopoulos, V. (2020). Exploring the potential of nitric oxide and hydrogen sulfide (NOSH)-releasing synthetic compounds as novel priming agents against drought stress in Medicago sativa plants. Biomolecules 10:120. doi: 10.3390/biom10010120
Aranega-Bou, P., de la O Leyva, M., Finiti, I., García-Agustín, P., and González-Bosch, C. (2014). Priming of plant resistance by natural compounds. Hexanoic acid as a model. Front. Plant Sci. 5:488. doi: 10.3389/fpls.2014.00488
Arnao, M. B., and Hernández-Ruiz, J. (2019). Melatonin: a new plant hormone and/or a plant master regulator? Trends Plant Sci. 24, 38–48. doi: 10.1016/j.tplants.2018.10.010
Asthir, B., and Bhatia, S. (2014). In vivo studies on artificial induction of thermotolerance to detached panicles of wheat (Triticum aestivum L) cultivars under heat stress. J. Food Sci. Technol. 51, 118–123. doi: 10.1007/s13197-011-0458-1
Avramova, Z. (2015). Transcriptional ‘memory’ of a stress: transient chromatin and memory (epigenetic) marks at stress-response genes. Plant J. 83, 149–159. doi: 10.1111/tpj.12832
Avramova, Z. (2019). Defence-related priming and responses to recurring drought: two manifestations of plant transcriptional memory mediated by the ABA and JA signalling pathways. Plant Cell Environ. 42, 983–997. doi: 10.1111/pce.13458
Baena-González, E., and Sheen, J. (2008). Convergent energy and stress signaling. Trends Plant Sci. 13, 474–482. doi: 10.1016/j.tplants.2008.06.006
Bajwa, V. S., Shukla, M. R., Sherif, S. M., Murch, S. J., and Saxena, P. K. (2014). Role of melatonin in alleviating cold stress in Arabidopsis thaliana. J. Pineal Res. 56, 238–245. doi: 10.1111/jpi.12115
Bakshi, A., Moin, M., Kumar, M. U., Reddy, A. B. M., Ren, M., Datla, R., et al. (2017). Ectopic expression of Arabidopsis Target of Rapamycin (AtTOR) improves water-use efficiency and yield potential in rice. Sci. Rep. 7, 1–16. doi: 10.1038/srep42835
Balmer, A., Pastor, V., Gamir, J., Flors, V., and Mauch-Mani, B. (2015). The ‘prime-ome’: towards a holistic approach to priming. Trends Plant Sci. 20, 443–452. doi: 10.1016/j.tplants.2015.04.002
Banerjee, A., and Roychoudhury, A. (2020). “Seed priming as a method to generate heat-stress tolerance in plants: a mini review,” in Heat Stress Tolerance in Plants: Physiological, Molecular and Genetic Perspectives, eds S. H. Wani and V. Kumar (Hoboken, NJ: Wiley), 23–32. doi: 10.1002/9781119432401.ch2
Banti, V., Loreti, E., Novi, G., Santaniello, A., Alpi, A., and Perata, P. (2008). Heat acclimation and cross-tolerance against anoxia in Arabidopsis. Plant Cell Environ. 31, 1029–1037. doi: 10.1111/j.1365-3040.2008.01816.x
Bäurle, I. (2016). Plant heat adaptation: priming in response to heat stress. F1000Research 5:694. doi: 10.12688/f1000research.7526.1
Beckers, G. J., and Conrath, U. (2007). Priming for stress resistance: from the lab to the field. Curr. Opin. Plant Biol. 10, 425–431. doi: 10.1016/j.pbi.2007.06.002
Beckers, G. J., Jaskiewicz, M., Liu, Y., Underwood, W. R., He, S. Y., Zhang, S., et al. (2009). Mitogen-activated protein kinases 3 and 6 are required for full priming of stress responses in Arabidopsis thaliana. Plant Cell 21, 944–953. doi: 10.1105/tpc.108.062158
Begum, N., Qin, C., Ahanger, M. A., Raza, S., Khan, M. I., Ashraf, M., et al. (2019). Role of arbuscular mycorrhizal fungi in plant growth regulation: implications in abiotic stress tolerance. Front. Plant Sci. 10:1068. doi: 10.3389/fpls.2019.01068
Berr, A., McCallum, E. J., Alioua, A., Heintz, D., Heitz, T., and Shen, W. H. (2010). Arabidopsis histone methyltransferase SET DOMAIN GROUP8 mediates induction of the jasmonate/ethylene pathway genes in plant defense response to necrotrophic fungi. Plant Physiol. 154, 1403–1414. doi: 10.1104/pp.110.161497
Bilichak, A. I. (2015). The elucidation of stress memory inheritance in Brassica rapa plants. Front. Plant Sci. 6:5. doi: 10.3389/fpls.2015.00005
Böhm, J., Scherzer, S., Krol, E., Kreuzer, I., von Meyer, K., Lorey, C., et al. (2016). The Venus flytrap Dionaea muscipula counts prey-induced action potentials to induce sodium uptake. Curr. Biol. 26, 286–295. doi: 10.1016/j.cub.2015.11.057
Boyko, A., and Kovalchuk, I. (2011). Genome instability and epigenetic modification—heritable responses to environmental stress? Curr. Opin. Plant Biol. 14, 260–266. doi: 10.1016/j.pbi.2011.03.003
Bruce, T. J., Matthes, M. C., Napier, J. A., and Pickett, J. A. (2007). Stressful “memories” of plants: evidence and possible mechanisms. Plant Sci. 173, 603–608. doi: 10.1016/j.plantsci.2007.09.002
Brzezinka, K., Altmann, S., and Bäurle, I. (2019). BRUSHY1/TONSOKU/MGOUN3 is required for heat stress memory. Plant Cell Environ. 42, 771–781. doi: 10.1111/pce.13365
Brzezinka, K., Altmann, S., Czesnick, H., Nicolas, P., Gorka, M., Benke, E., et al. (2016). Arabidopsis FORGETTER1 mediates stress-induced chromatin memory through nucleosome remodeling. Elife 5:e17061. doi: 10.7554/eLife.17061.037
Byeon, B., Bilichak, A., and Kovalchuk, I. (2019). Transgenerational response to heat stress in the form of differential expression of noncoding RNA fragments in Brassica rapa plants. Plant Genome 12:180022. doi: 10.3835/plantgenome2018.04.0022
Byeon, Y., Park, S., Kim, Y. S., Park, D. H., Lee, S., and Back, K. (2012). Light-regulated melatonin biosynthesis in rice during the senescence process in detached leaves. J. Pineal Res. 53, 107–111. doi: 10.1111/j.1600-079X.2012.00976.x
Byun, Y. J., Koo, M. Y., Joo, H. J., Ha-Lee, Y. M., and Lee, D. H. (2014). Comparative analysis of gene expression under cold acclimation, deacclimation and reacclimation in Arabidopsis. Physiol. Plant. 152, 256–274. doi: 10.1111/ppl.12163
Carrigan, P. E., Sikkink, L. A., Smith, D. F., and Ramirez-Alvarado, M. (2006). Domain: domain interactions within Hop, the Hsp70/Hsp90 organizing protein, are required for protein stability and structure. Protein Sci. 15, 522–532. doi: 10.1110/ps.051810106
Carter, A. H., Chen, X., Garland-Campbell, K., and Kidwell, K. (2009). Identifying QTL for high-temperature adult-plant resistance to stripe rust (Puccinia striiformis f. sp. tritici) in the spring wheat (Triticum aestivum L.) cultivar ‘Louise’. Theor. Appl. Genet. 119, 1119–1128. doi: 10.1007/s00122-009-1114-2
Castellanos, M., Mothi, N., and Muñoz, V. (2020). Eukaryotic transcription factors can track and control their target genes using DNA antennas. Nat. Commun. 11:540. doi: 10.1038/s41467-019-14217-8
Çevik, V., Kidd, B. N., Zhang, P., Hill, C., Kiddle, S., Denby, K. J., et al. (2012). MEDIATOR25 acts as an integrative hub for the regulation of jasmonate-responsive gene expression in Arabidopsis. Plant Physiol. 160, 541–555. doi: 10.1104/pp.112.202697
Chakrabortee, S., Kayatekin, C., Newby, G. A., Mendillo, M. L., Lancaster, A., and Lindquist, S. (2016). Luminidependens (LD) is an Arabidopsis protein with prion behavior. Proc. Natl. Acad. Sci. U.S.A. 113, 6065–6070. doi: 10.1073/pnas.1604478113
Charng, Y. Y., Liu, H. C., Liu, N. Y., Chi, W. T., Wang, C. N., Chang, S. H., et al. (2007). A heat-inducible transcription factor, HsfA2, is required for extension of acquired thermotolerance in Arabidopsis. Plant Physiol. 143, 251–262. doi: 10.1104/pp.106.091322
Chehab, E. W., Eich, E., and Braam, J. (2009). Thigmomorphogenesis: a complex plant response to mechano-stimulation. J. Exp. Bot. 60, 43–56. doi: 10.1093/jxb/ern315
Chehab, E. W., Yao, C., Henderson, Z., Kim, S., and Braam, J. (2012). Arabidopsis touch-induced morphogenesis is jasmonate mediated and protects against pests. Curr. Biol. 22, 701–706. doi: 10.1016/j.cub.2012.02.061
Cheikh, N., and Jones, R. J. (1994). Disruption of maize kernel growth and development by heat stress (role of cytokinin/abscisic acid balance). Plant Physiol. 106, 45–51. doi: 10.1104/pp.106.1.45
Chen, L., Ren, Y., Zhang, Y., Xu, J., Sun, F., Zhang, Z., et al. (2012). Genome-wide identification and expression analysis of heat-responsive and novel microRNAs in Populus tomentosa. Gene 504, 160–165. doi: 10.1016/j.gene.2012.05.034
Chen, R., Jiang, H., Li, L., Zhai, Q., Qi, L., Zhou, W., et al. (2012). The Arabidopsis mediator subunit MED25 differentially regulates jasmonate and abscisic acid signaling through interacting with the MYC2 and ABI5 transcription factors. Plant Cell 24, 2898–2916. doi: 10.1105/tpc.112.098277
Cheng, Y., JalalAhammed, G., Yu, J., Yao, Z., Ruan, M., Ye, Q., et al. (2016). Putative WRKYs associated with regulation of fruit ripening revealed by detailed expression analysis of the WRKY gene family in pepper. Sci. Rep. 6, 1–11. doi: 10.1038/srep39000
Chhabra, M., Dhawan, A., Sangwan, N., Dhawan, K., and Singh, D. (2009). “Phytohormones induced amelioration of high temperature stress in Brassica juncea (L.) Czern and Coss,” in Proceedings of 16th Australian Research Assembly on Brassicas, Ballarat, VIC, 10–14.
Chini, A., Fonseca, S. G. D. C., Fernandez, G., Adie, B., Chico, J. M., Lorenzo, O., et al. (2007). The JAZ family of repressors is the missing link in jasmonate signalling. Nature 448, 666–671. doi: 10.1038/nature06006
Choi, C.-S., and Sano, H. (2007). Abiotic-stress induces demethylation and transcriptional activation of a gene encoding a glycerophosphodiesterase-like protein in tobacco plants. Mol. Genet. Genomics 277, 589–600. doi: 10.1007/s00438-007-0209-1
Choi, H., and Oh, E. (2016). PIF4 integrates multiple environmental and hormonal signals for Plant growth regulation in Arabidopsis. Mol. Cells 39:587. doi: 10.14348/molcells.2016.0126
Chou, T.-S., Chao, Y.-Y., and Kao, C. H. (2012). Involvement of hydrogen peroxide in heat shock-and cadmium-induced expression of ascorbate peroxidase and glutathione reductase in leaves of rice seedlings. J. Plant Physiol. 169, 478–486. doi: 10.1016/j.jplph.2011.11.012
Clarke, S. M., Cristescu, S. M., Miersch, O., Harren, F. J., Wasternack, C., and Mur, L. A. (2009). Jasmonates act with salicylic acid to confer basal thermotolerance in Arabidopsis thaliana. New Phytol. 182, 175–187. doi: 10.1111/j.1469-8137.2008.02735.x
Clarke, S. M., Mur, L. A., Wood, J. E., and Scott, I. M. (2004). Salicylic acid dependent signaling promotes basal thermotolerance but is not essential for acquired thermotolerance in Arabidopsis thaliana. Plant J. 38, 432–447. doi: 10.1111/j.1365-313X.2004.02054.x
Cohen, S. P., Liu, H., Argueso, C. T., Pereira, A., Vera Cruz, C., Verdier, V., et al. (2017). RNA-Seq analysis reveals insight into enhanced rice Xa7-mediated bacterial blight resistance at high temperature. PLoS One 12:e0187625. doi: 10.1371/journal.pone.0187625
Collins, G. G., Nie, X., and Saltveit, M. E. (1995). Heat shock proteins and chilling sensitivity of mung bean hypocotyls. J. Exp. Bot. 46, 795–802. doi: 10.1093/jxb/46.7.795
Conrath, U. (2011). Molecular aspects of defence priming. Trends Plant Sci. 16, 524–531. doi: 10.1016/j.tplants.2011.06.004
Conrath, U., Beckers, G. J., Langenbach, C. J., and Jaskiewicz, M. R. (2015). Priming for enhanced defense. Annu. Rev. Phytopathol. 53, 97–119. doi: 10.1146/annurev-phyto-080614-120132
Crisp, P. A., Ganguly, D., Eichten, S. R., Borevitz, J. O., and Pogson, B. J. (2016). Reconsidering plant memory: intersections between stress recovery, RNA turnover, and epigenetics. Sci. Adv. 2:e1501340. doi: 10.1126/sciadv.1501340
Darwin, C., and Darwin, F. (1880). The Power Of Movement In Plants. London: John Murray. doi: 10.5962/bhl.title.102319
Davletova, S., Schlauch, K., Coutu, J., and Mittler, R. (2005). The zinc-finger protein Zat12 plays a central role in reactive oxygen and abiotic stress signaling in Arabidopsis. Plant Physiol. 139, 847–856. doi: 10.1104/pp.105.068254
De Lucia, F., Crevillen, P., Jones, A. M., Greb, T., and Dean, C. (2008). A PHD-polycomb repressive complex 2 triggers the epigenetic silencing of FLC during vernalization. Proc. Natl. Acad. Sci. U.S.A. 105, 16831–16836. doi: 10.1073/pnas.0808687105
de Ollas, C., Arbona, V., and Gómez-Cadenas, A. (2015). Jasmonic acid interacts with abscisic acid to regulate plant responses to water stress conditions. Plant Signal. Behav. 10:e1078953. doi: 10.1080/15592324.2015.1078953
De Vleesschauwer, D., Filipe, O., Hoffman, G., Seifi, H. S., Haeck, A., Canlas, P., et al. (2018). Target of rapamycin signaling orchestrates growth–defense trade-offs in plants. New Phytol. 217, 305–319. doi: 10.1111/nph.14785
Dhaubhadel, S., Browning, K. S., Gallie, D. R., and Krishna, P. (2002). Brassinosteroid functions to protect the translational machinery and heat-shock protein synthesis following thermal stress. Plant J. 29, 681–691. doi: 10.1046/j.1365-313X.2002.01257.x
Ding, B., and Wang, G.-L. (2015). Chromatin versus pathogens: the function of epigenetics in plant immunity. Front. Plant Sci. 6:675. doi: 10.3389/fpls.2015.00675
Ding, Y., Fromm, M., and Avramova, Z. (2012). Multiple exposures to drought ‘train’ transcriptional responses in Arabidopsis. Nat. Commun. 3, 1–9. doi: 10.1038/ncomms1732
Ding, Y., Liu, N., Virlouvet, L., Riethoven, J.-J., Fromm, M., and Avramova, Z. (2013). Four distinct types of dehydration stress memory genes in Arabidopsis thaliana. BMC Plant Biol. 13:229. doi: 10.1186/1471-2229-13-229
Ding, Y., Virlouvet, L., Liu, N., Riethoven, J.-J., Fromm, M., and Avramova, Z. (2014). Dehydration stress memory genes of Zea mays; comparison with Arabidopsis thaliana. BMC Plant Biol. 14:141. doi: 10.1186/1471-2229-14-141
Divi, U. K., Rahman, T., and Krishna, P. (2010). Brassinosteroid-mediated stress tolerance in Arabidopsis shows interactions with abscisic acid, ethylene and salicylic acid pathways. BMC Plant Biol. 10:151. doi: 10.1186/1471-2229-10-151
Djanaguiraman, M., Prasad, P. V., and Seppanen, M. (2010). Selenium protects sorghum leaves from oxidative damage under high temperature stress by enhancing antioxidant defense system. Plant Physiol. Biochem. 48, 999–1007. doi: 10.1016/j.plaphy.2010.09.009
Dobrá, J., Černý, M., Štorchová, H., Dobrev, P., Skalák, J., Jedelský, P. L., et al. (2015). The impact of heat stress targeting on the hormonal and transcriptomic response in Arabidopsis. Plant Sci. 231, 52–61. doi: 10.1016/j.plantsci.2014.11.005
Dong, Y., Teleman, A., Jedmowski, C., Wirtz, M., and Hell, R. (2019). The Arabidopsis THADA homologue modulates TOR activity and cold acclimation. Plant Biol. 21, 77–83. doi: 10.1111/plb.12893
dos Santos Araújo, G., de Oliveira Paula-Marinho, S., de Paiva Pinheiro, S. K., de Castro Miguel, E., de Sousa Lopes, L., Marques, E. C., et al. (2021). H2O2 priming promotes salt tolerance in maize by protecting chloroplasts ultrastructure and primary metabolites modulation. Plant Sci. 303:110774. doi: 10.1016/j.plantsci.2020.110774
Drerup, M. M., Schlücking, K., Hashimoto, K., Manishankar, P., Steinhorst, L., Kuchitsu, K., et al. (2013). The calcineurin B-like calcium sensors CBL1 and CBL9 together with their interacting protein kinase CIPK26 regulate the Arabidopsis NADPH oxidase RBOHF. Mol. Plant 6, 559–569. doi: 10.1093/mp/sst009
D’Urso, A., Takahashi, Y. H., Xiong, B., Marone, J., Coukos, R., Randise-Hinchliff, C., et al. (2016). Set1/COMPASS and Mediator are repurposed to promote epigenetic transcriptional memory. Elife 5:e16691. doi: 10.7554/eLife.16691.020
Eichten, S. R., Schmitz, R. J., and Springer, N. M. (2014). Epigenetics: beyond chromatin modifications and complex genetic regulation. Plant Physiol. 165, 933–947. doi: 10.1104/pp.113.234211
Elfving, N., Davoine, C., Benlloch, R., Blomberg, J., Brännström, K., Müller, D., et al. (2011). The Arabidopsis thaliana Med25 mediator subunit integrates environmental cues to control plant development. Proc. Natl. Acad. Sci. U.S.A. 108, 8245–8250. doi: 10.1073/pnas.1002981108
Fan, Y., Ma, C., Huang, Z., Abid, M., Jiang, S., Dai, T., et al. (2018). Heat priming during early reproductive stages enhances thermo-tolerance to post-anthesis heat stress via improving photosynthesis and plant productivity in winter wheat (Triticum aestivum L.). Front. Plant Sci. 9:805. doi: 10.3389/fpls.2018.00805
Fang, X., Zhao, G., Zhang, S., Li, Y., Gu, H., Li, Y., et al. (2019). Chloroplast-to-nucleus signaling regulates microRNA biogenesis in Arabidopsis. Dev. Cell 48, 371–382. doi: 10.1016/j.devcel.2018.11.046
Faralli, M., Lektemur, C., Rosellini, D., and Gürel, F. (2015). Effects of heat shock and salinity on barley growth and stress-related gene transcription. Biol. Plant. 59, 537–546. doi: 10.1007/s10535-015-0518-x
Feng, B., Zhang, C., Chen, T., Zhang, X., Tao, L., and Fu, G. (2018). Salicylic acid reverses pollen abortion of rice caused by heat stress. BMC Plant Biol. 18:245. doi: 10.1186/s12870-018-1472-5
Fiorucci, A. S., Galvão, V. C., Ince, Y. Ç, Boccaccini, A., Goyal, A., Allenbach Petrolati, L., et al. (2020). Phytochrome interacting factor 7 is important for early responses to elevated temperature in Arabidopsis seedlings. New Phytol. 226, 50–58. doi: 10.1111/nph.16316
Fragkostefanakis, S., Röth, S., Schleiff, E., and Scharf, K. D. (2015). Prospects of engineering thermotolerance in crops through modulation of heat stress transcription factor and heat shock protein networks. Plant Cell Environ. 38, 1881–1895. doi: 10.1111/pce.12396
Fu, Y., Gu, Q., Dong, Q., Zhang, Z., Lin, C., Hu, W., et al. (2019). Spermidine enhances heat tolerance of rice seeds by modulating endogenous starch and polyamine metabolism. Molecules 24:1395. doi: 10.3390/molecules24071395
Gagliano, M., Abramson, C. I., and Depczynski, M. (2018). Plants learn and remember: lets get used to it. Oecologia 186, 29–31. doi: 10.1007/s00442-017-4029-7
Garai, S., Singla-Pareek, S. L., Sopory, S. K., Kaur, C., and Yadav, G. (2021). Complex networks of prion-like proteins reveal cross talk between stress and memory pathways in plants. Front. Plant Sci. 12:1513. doi: 10.3389/fpls.2021.707286
Giacomelli, J. I., Weigel, D., Chan, R. L., and Manavella, P. A. (2012). Role of recently evolved miRNA regulation of sunflower HaWRKY6 in response to temperature damage. New Phytol. 195, 766–773. doi: 10.1111/j.1469-8137.2012.04259.x
Gondim, F. A., Miranda, R. D. S., Gomes-Filho, E., and Prisco, J. T. (2013). Enhanced salt tolerance in maize plants induced by H2O2 leaf spraying is associated with improved gas exchange rather than with non-enzymatic antioxidant system. Theor. Exp. Plant Physiol. 25, 251–260. doi: 10.1590/S2197-00252013000400003
Gong, M., Chen, B., Li, Z.-G., and Guo, L.-H. (2001). Heat-shock-induced cross adaptation to heat, chilling, drought and salt stress in maize seedlings and involvement of H2O2. J. Plant Physiol. 158, 1125–1130. doi: 10.1078/0176-1617-00327
González-Cruz, J., and Pastenes, C. (2012). Water-stress-induced thermotolerance of photosynthesis in bean (Phaseolus vulgaris L.) plants: the possible involvement of lipid composition and xanthophyll cycle pigments. Environ. Exp. Bot. 77, 127–140. doi: 10.1016/j.envexpbot.2011.11.004
Guan, L. M., Zhao, J., and Scandalios, J. G. (2000). Cis-elements and trans-factors that regulate expression of the maize Cat1 antioxidant gene in response to ABA and osmotic stress: H2O2 is the likely intermediary signaling molecule for the response. Plant J. 22, 87–95. doi: 10.1046/j.1365-313x.2000.00723.x
Guan, Q., Lu, X., Zeng, H., Zhang, Y., and Zhu, J. (2013). Heat stress induction of mi R 398 triggers a regulatory loop that is critical for thermotolerance in Arabidopsis. Plant J. 74, 840–851. doi: 10.1111/tpj.12169
Guertin, M. J., and Lis, J. T. (2010). Chromatin landscape dictates HSF binding to target DNA elements. PLoS Genet. 6:e1001114. doi: 10.1371/journal.pgen.1001114
Guo, J., Wu, J., Ji, Q., Wang, C., Luo, L., Yuan, Y., et al. (2008). Genome-wide analysis of heat shock transcription factor families in rice and Arabidopsis. J. Genet. Genomics 35, 105–118. doi: 10.1016/S1673-8527(08)60016-8
Guo, M., Liu, J.-H., Ma, X., Luo, D.-X., Gong, Z.-H., and Lu, M.-H. (2016). The plant heat stress transcription factors (HSFs): structure, regulation, and function in response to abiotic stresses. Front. Plant Sci. 7:114. doi: 10.3389/fpls.2016.00114
Guo, M., Lu, J.-P., Zhai, Y.-F., Chai, W.-G., Gong, Z.-H., and Lu, M.-H. (2015). Genome-wide analysis, expression profile of heat shock factor gene family (CaHsfs) and characterisation of CaHsfA2 in pepper (Capsicum annuum L.). BMC Plant Biol. 15:1–20. doi: 10.1186/s12870-015-0512-7
Hake, K., and Romeis, T. (2019). Protein kinase-mediated signalling in priming: immune signal initiation, propagation, and establishment of long-term pathogen resistance in plants. Plant Cell Environ. 42, 904–917. doi: 10.1111/pce.13429
Hameed, S., and Ali, M. K. (2016). Exogenous application of salicylic acid: inducing thermotolerance in cotton (Gossypium Hirsutum L.) seedlings. Int. J. Agric. Food Res. 5, 9–18. doi: 10.24102/ijafr.v5i4.691
Harrington, H. M., and Alm, D. M. (1988). Interaction of heat and salt shock in cultured tobacco cells. Plant Physiol. 88, 618–625. doi: 10.1104/pp.88.3.618
Hasanuzzaman, M., and Fotopoulos, V. (2019). Priming And Pretreatment Of Seeds And Seedlings. Singapore: Springer. doi: 10.1007/978-981-13-8625-1
Hasanuzzaman, M., Nahar, K., Alam, M., Roychowdhury, R., and Fujita, M. (2013). Physiological, biochemical, and molecular mechanisms of heat stress tolerance in plants. Int. J. Mol. Sci. 14, 9643–9684. doi: 10.3390/ijms14059643
Herman, J. J., and Sultan, S. E. (2011). Adaptive transgenerational plasticity in plants: case studies, mechanisms, and implications for natural populations. Front. Plant Sci. 2:102. doi: 10.3389/fpls.2011.00102
Hilker, M., and Schmülling, T. (2019). Stress Priming, Memory, And Signalling In Plants. Hoboken, NJ: Wiley Online Library. doi: 10.1111/pce.13526
Hilker, M., Schwachtje, J., Baier, M., Balazadeh, S., Bäurle, I., Geiselhardt, S., et al. (2016). Priming and memory of stress responses in organisms lacking a nervous system. Biol. Rev. 91, 1118–1133. doi: 10.1111/brv.12215
Hivrale, V., Zheng, Y., Puli, C. O. R., Jagadeeswaran, G., Gowdu, K., Kakani, V. G., et al. (2016). Characterization of drought- and heat- responsive microRNAs in switchgrass. Plant Sci. 242, 214–223. doi: 10.1016/j.plantsci.2015.07.018
Hoque, T. S., Hossain, M. A., Mostofa, M. G., Burritt, D. J., Fujita, M., and Tran, L.-S. P. (2016). Methylglyoxal: an emerging signaling molecule in plant abiotic stress responses and tolerance. Front. Plant Sci. 7:1341. doi: 10.3389/fpls.2016.01341
Hoque, T., Uraji, M., Tuya, A., Nakamura, Y., and Murata, Y. (2012). Methylglyoxal inhibits seed germination and root elongation and up-regulates transcription of stress-responsive genes in ABA-dependent pathway in Arabidopsis. Plant Biol. 14, 854–858. doi: 10.1111/j.1438-8677.2012.00607.x
Hossain, M. A., Bhattacharjee, S., Armin, S. M., Qian, P., Xin, W., Li, H. Y., et al. (2015). Hydrogen peroxide priming modulates abiotic oxidative stress tolerance: insights from ROS detoxification and scavenging. Front. Plant Sci. 6:420. doi: 10.3389/fpls.2015.00420
Hossain, M. A., Liu, F., Burritt, D., Fujita, M., and Huang, B. (2020). Priming-Mediated Stress and Cross-Stress Tolerance in Crop Plants. Cambridge, MA: Academic Press.
Hossain, M. A., Mostofa, M. G., and Fujita, M. (2013a). Cross protection by cold-shock to salinity and drought stress-induced oxidative stress in mustard (Brassica campestris L.) seedlings. Mol. Plant Breed. 4, 50–70. doi: 10.5376/mpb.2013.04.0007
Hossain, M. A., Mostofa, M. G., and Fujita, M. (2013b). Heat-shock positively modulates oxidative protection of salt and drought-stressed mustard (Brassica campestris L.) seedlings. J. Plant Sci. Mol. Breed. 2:2. doi: 10.7243/2050-2389-2-2
Hsu, Y. T., and Kao, C. H. (2007). Heat shock-mediated H 2 O 2 accumulation and protection against Cd toxicity in rice seedlings. Plant Soil 300, 137–147. doi: 10.1007/s11104-007-9396-0
Hu, Q., Zhang, S., and Huang, B. (2019). Strigolactones promote leaf elongation in tall fescue through upregulation of cell cycle genes and downregulation of auxin transport genes in tall fescue under different temperature regimes. Int. J. Mol. Sci. 20:1836. doi: 10.3390/ijms20081836
Hu, X. J., Chen, D., Lynne Mclntyre, C., Fernanda Dreccer, M., Zhang, Z. B., Drenth, J., et al. (2018). Heat shock factor C2a serves as a proactive mechanism for heat protection in developing grains in wheat via an ABA-mediated regulatory pathway. Plant Cell Environ. 41, 79–98. doi: 10.1111/pce.12957
Huan, Q., Mao, Z., Chong, K., and Zhang, J. (2018). Global analysis of H3K4me3/H3K27me3 in Brachypodium distachyon reveals VRN 3 as critical epigenetic regulation point in vernalization and provides insights into epigenetic memory. New Phytol. 219, 1373–1387. doi: 10.1111/nph.15288
Huang, T.-H., Fowler, F., Chen, C.-C., Shen, Z.-J., Sleckman, B., and Tyler, J. K. (2018). The histone chaperones ASF1 and CAF-1 promote MMS22L-TONSL-mediated Rad51 loading onto ssDNA during homologous recombination in human cells. Mol. Cell 69, 879–892. E8. doi: 10.1016/j.molcel.2018.01.031
Hussain, M., Khan, T. A., Yusuf, M., and Fariduddin, Q. (2019). Silicon-mediated role of 24-epibrassinolide in wheat under high-temperature stress. Environ. Sci. Pollut. Res. 26, 17163–17172. doi: 10.1007/s11356-019-04938-0
Iba, K. (2002). Acclimative response to temperature stress in higher plants: approaches of gene engineering for temperature tolerance. Annu. Rev. Plant Biol. 53, 225–245. doi: 10.1146/annurev.arplant.53.100201.160729
Ibañez, C., Delker, C., Martinez, C., Bürstenbinder, K., Janitza, P., Lippmann, R., et al. (2018). Brassinosteroids dominate hormonal regulation of plant thermomorphogenesis via BZR1. Curr. Biol. 28, 303–310. doi: 10.1016/j.cub.2017.11.077
Ikeda, M., Mitsuda, N., and Ohme-Takagi, M. (2011). Arabidopsis HsfB1 and HsfB2b act as repressors of the expression of heat-inducible Hsfs but positively regulate the acquired thermotolerance. Plant Physiol. 157, 1243–1254. doi: 10.1104/pp.111.179036
IPCC (2018). “Summary for policymakers,” in Global Warming of 1.5°C. An IPCC Special Report on the Impacts of Global Warming of 1.5°C Above Pre-industrial Levels and Related Global Greenhouse gas Emission Pathways, in the Context of Strengthening the Global Response to the Threat of Climate Change, Sustainable Development, and Efforts to Eradicate Poverty, eds V. Masson-Delmotte, P. Zhai, H.-O. Pörtner, D. Roberts, J. Skea, P. R. Shukla, et al. (Geneva: World Meteorological Organization), 32.
Iqbal, M., Raja, N. I., Yasmeen, F., Hussain, M., Ejaz, M., and Shah, M. (2017). Impacts of heat stress on wheat: a critical review. Adv. Crop Sci. Technol. 5, 01–09. doi: 10.4172/2329-8863.1000251
Jagadish, S., Muthurajan, R., Oane, R., Wheeler, T. R., Heuer, S., Bennett, J., et al. (2010). Physiological and proteomic approaches to address heat tolerance during anthesis in rice (Oryza sativa L.). J. Exp. Bot. 61, 143–156. doi: 10.1093/jxb/erp289
Jahan, M. S., Wang, Y., Shu, S., Zhong, M., Chen, Z., Wu, J., et al. (2019). Exogenous salicylic acid increases the heat tolerance in Tomato (Solanum lycopersicum L) by enhancing photosynthesis efficiency and improving antioxidant defense system through scavenging of reactive oxygen species. Sci. Hortic. 247, 421–429. doi: 10.1016/j.scienta.2018.12.047
Janda, T., Lejmel, M. A., Molnár, A. B., Majláth, I., Pál, M., Nguyen, Q. T., et al. (2020). Interaction between elevated temperature and different types of Na-salicylate treatment in Brachypodium dystachion. PLoS One 15:e0227608. doi: 10.1371/journal.pone.0227608
Janeczko, A., Oklešt́ková, J., Pociecha, E., Kościelniak, J., and Mirek, M. (2011). Physiological effects and transport of 24-epibrassinolide in heat-stressed barley. Acta Physiol. Plant. 33, 1249–1259. doi: 10.1007/s11738-010-0655-y
Jaskiewicz, M., Conrath, U., and Peterhänsel, C. (2011). Chromatin modification acts as a memory for systemic acquired resistance in the plant stress response. EMBO Rep. 12, 50–55. doi: 10.1038/embor.2010.186
Jegadeesan, S., Beery, A., Altahan, L., Meir, S., Pressman, E., and Firon, N. (2018). Ethylene production and signaling in tomato (Solanum lycopersicum) pollen grains is responsive to heat stress conditions. Plant Reprod. 31, 367–383. doi: 10.1007/s00497-018-0339-0
Jennings, P., and Saltveit, M. E. (1994). Temperature and chemical shocks induce chilling tolerance in germinating Cucumis sativus (cv. Poinsett 76) seeds. Physiol. Plant. 91, 703–707. doi: 10.1111/j.1399-3054.1994.tb03008.x
Jiang, D., Kong, N. C., Gu, X., Li, Z., and He, Y. (2011). Arabidopsis COMPASS-like complexes mediate histone H3 lysine-4 trimethylation to control floral transition and plant development. PLoS Genet. 7:e1001330. doi: 10.1371/journal.pgen.1001330
Jin, Z., and Pei, Y. (2015). Physiological implications of hydrogen sulfide in plants: pleasant exploration behind its unpleasant odour. Oxid. Med. Cell. Longev. 2015:397502. doi: 10.1155/2015/397502
Jonak, C., Ökrész, L., Bögre, L., and Hirt, H. (2002). Complexity, cross talk and integration of plant MAP kinase signalling. Curr. Opin. Plant Biol. 5, 415–424. doi: 10.1016/S1369-5266(02)00285-6
Jung, J. H., Barbosa, A. D., Hutin, S., Kumita, J. R., Gao, M., Derwort, D., et al. (2020). A prion-like domain in ELF3 functions as a thermosensor in Arabidopsis. Nature 585, 256–260. doi: 10.1038/s41586-020-2644-7
Jyothi, M., and Rai, D. (2015). Identification and characterization of high temperature stress responsive novel miRNAs in French bean (Phaseolus vulgaris). Appl. Biochem. Biotechnol. 176, 835–849. doi: 10.1007/s12010-015-1614-2
Kang, H. M., and Saltveit, M. E. (2001). Activity of enzymatic antioxidant defense systems in chilled and heat shocked cucumber seedling radicles. Physiol. Plant. 113, 548–556. doi: 10.1034/j.1399-3054.2001.1130414.x
Kata, L. P., Bhaskaran, M., and Umarani, R. (2014). Influence of priming treatments on stress tolerance during seed germination of rice. Int. J. Agric. Environ. Biotechnol. 7, 225–232. doi: 10.5958/2230-732X.2014.00238.1
Kaur, C., Singla-Pareek, S. L., and Sopory, S. K. (2014). Glyoxalase and methylglyoxal as biomarkers for plant stress tolerance. Crit. Rev. Plant Sci. 33, 429–456. doi: 10.1080/07352689.2014.904147
Kaur, N., Kaur, J., Grewal, S. K., and Singh, I. (2019). Effect of heat stress on antioxidative defense system and its amelioration by heat acclimation and salicylic acid pre-treatments in three pigeonpea genotypes. Indian J. Agric. Biochem. 32, 106–110. doi: 10.5958/0974-4479.2019.00014.5
Kaur, P., Ghai, N., and Sangha, M. K. (2009). Induction of thermotolerance through heat acclimation and salicylic acid in Brassica species. African J. Biotechnol. 8, 619–625.
Kaushal, N., Gupta, K., Bhandhari, K., Kumar, S., Thakur, P., and Nayyar, H. (2011). Proline induces heat tolerance in chickpea (Cicer arietinum L.) plants by protecting vital enzymes of carbon and antioxidative metabolism. Physiol. Mol. Biol. Plants 17, 203–213. doi: 10.1007/s12298-011-0078-2
Kauss, H., Theisinger-Hinkel, E., Mindermann, R., and Conrath, U. (1992). Dichloroisonicotinic and salicylic acid, inducers of systemic acquired resistance, enhance fungal elicitor responses in parsley cells. Plant J. 2, 655–660. doi: 10.1111/j.1365-313X.1992.tb00134.x
Ke, Q., Ye, J., Wang, B., Ren, J., Yin, L., Deng, X., et al. (2018). Melatonin mitigates salt stress in wheat seedlings by modulating polyamine metabolism. Front. Plant Sci. 9:914. doi: 10.3389/fpls.2018.00914
Khan, A., Bilal, S., Khan, A. L., Imran, M., Shahzad, R., Al-Harrasi, A., et al. (2020). Silicon and gibberellins: synergistic function in harnessing ABA signaling and heat stress tolerance in date palm (Phoenix dactylifera L.). Plants 9:620. doi: 10.3390/plants9050620
Khan, A., Goswami, K., Sopory, S. K., and Sanan-Mishra, N. (2017). “Mirador” on the potential role of miRNAs in synergy of light and heat networks. Indian J. Plant Physiol. 22, 587–607. doi: 10.1007/s40502-017-0329-5
Khan, M. I. R., Iqbal, N., Masood, A., Per, T. S., and Khan, N. A. (2013). Salicylic acid alleviates adverse effects of heat stress on photosynthesis through changes in proline production and ethylene formation. Plant Signal. Behav. 8:e26374. doi: 10.4161/psb.26374
Khan, T., Yusuf, M., and Fariduddin, Q. (2018). Hydrogen peroxide in regulation of plant metabolism: signalling and its effect under abiotic stress. Photosynthetica 56, 1237–1248. doi: 10.1007/s11099-018-0830-8
Khanna, P., Kaur, K., and Gupta, A. K. (2016). Salicylic acid induces differential antioxidant response in spring maize under high temperature stress. Indian J. Exp. Biol. 54, 386–393.
Kim, S., Hwang, G., Kim, S., Thi, T. N., Kim, H., Jeong, J., et al. (2020). The epidermis coordinates thermoresponsive growth through the phyB-PIF4-auxin pathway. Nat. Commun. 11, 1–13. doi: 10.1038/s41467-020-14905-w
Kinoshita, T., and Seki, M. (2014). Epigenetic memory for stress response and adaptation in plants. Plant Cell Physiol. 55, 1859–1863. doi: 10.1093/pcp/pcu125
Kochhar, S., and Kochhar, V. K. (2005). Expression of antioxidant enzymes and heat shock proteins in relation to combined stress of cadmium and heat in Vigna mungo seedlings. Plant Sci. 168, 921–929. doi: 10.1016/j.plantsci.2004.11.013
Koizumi, S., Ohama, N., Mizoi, J., Shinozaki, K., and Yamaguchi-Shinozaki, K. (2014). Functional analysis of the Hikeshi-like protein and its interaction with HSP70 in Arabidopsis. Biochem. Biophys. Res. Commun. 450, 396–400. doi: 10.1016/j.bbrc.2014.05.128
Kollist, H., Zandalinas, S. I., Sengupta, S., Nuhkat, M., Kangasjärvi, J., and Mittler, R. (2019). Rapid responses to abiotic stress: priming the landscape for the signal transduction network. Trends Plant Sci. 24, 25–37. doi: 10.1016/j.tplants.2018.10.003
Kornberg, R. D. (1974). Chromatin structure: a repeating unit of histones and DNA. Science 184, 868–871. doi: 10.1126/science.184.4139.868
Kotak, S., Larkindale, J., Lee, U., von Koskull-Döring, P., Vierling, E., and Scharf, K.-D. (2007). Complexity of the heat stress response in plants. Curr. Opin. Plant Biol. 10, 310–316. doi: 10.1016/j.pbi.2007.04.011
Kothari, A., and Lachowiec, J. (2021). Roles of brassinosteroids in mitigating heat stress damage in cereal crops. Int. J. Mol. Sci. 22:2706. doi: 10.3390/ijms22052706
Kotkar, H., and Giri, A. (2020). “Plant epigenetics and the ‘intelligent’priming system to combat biotic stress,” in Epigenetics Of The Immune System, eds D. Kabelitz and J. Bhat (Amsterdam: Elsevier), 25–38. doi: 10.1016/B978-0-12-817964-2.00002-2
Kruszka, K., Pacak, A., Swida-Barteczka, A., Nuc, P., Alaba, S., Wroblewska, Z., et al. (2014). Transcriptionally and post-transcriptionally regulated microRNAs in heat stress response in barley. J. Exp. Bot. 65, 6123–6135. doi: 10.1093/jxb/eru353
Kudla, J., Becker, D., Grill, E., Hedrich, R., Hippler, M., Kummer, U., et al. (2018). Advances and current challenges in calcium signaling. New Phytol. 218, 414–431. doi: 10.1111/nph.14966
Kumar, M., Kesawat, M. S., Ali, A., Lee, S.-C., Gill, S. S., and Kim, H. U. (2019). Integration of abscisic acid signaling with other signaling pathways in plant stress responses and development. Plants 8:592. doi: 10.3390/plants8120592
Kumar, R. R., Sharma, S. K., Goswami, S., Verma, P., Singh, K., Dixit, N., et al. (2015). Salicylic acid alleviates the heat stress-induced oxidative damage of starch biosynthesis pathway by modulating the expression of heat-stable genes and proteins in wheat (Triticum aestivum). Acta Physiol. Plant. 37, 1–12. doi: 10.1007/s11738-015-1899-3
Kumar, S., Kaushal, N., Nayyar, H., and Gaur, P. (2012). Abscisic acid induces heat tolerance in chickpea (Cicer arietinum L.) seedlings by facilitated accumulation of osmoprotectants. Acta Physiol. Plant. 34, 1651–1658. doi: 10.1007/s11738-012-0959-1
Kumari, V. V., Banerjee, P., Nath, R., and Sengupta, K. (2019). Effect of foliar spray on phenology and yield of Lentil sown on different dates. J. Crop Weed 15, 54–58. doi: 10.22271/09746315.2019.v15.i3.1237
Kumari, V. V., Roy, A., Vijayan, R., Banerjee, P., Verma, V. C., Nalia, A., et al. (2021). Drought and heat stress in cool-season food legumes in sub-tropical regions: consequences, adaptation and mitigation strategies. Plants 10:1038. doi: 10.3390/plants10061038
Küpers, J. J., Oskam, L., and Pierik, R. (2020). Photoreceptors regulate plant developmental plasticity through auxin. Plants 9:940. doi: 10.3390/plants9080940
Kurepin, L. V., Ivanov, A. G., Zaman, M., Pharis, R. P., Allakhverdiev, S. I., Hurry, V., et al. (2015). Stress-related hormones and glycinebetaine interplay in protection of photosynthesis under abiotic stress conditions. Photosynthesis Res. 126, 221–235. doi: 10.1007/s11120-015-0125-x
Kurepin, L. V., Qaderi, M. M., Back, T. G., Reid, D. M., and Pharis, R. P. (2008). A rapid effect of applied brassinolide on abscisic acid concentrations in Brassica napus leaf tissue subjected to short-term heat stress. Plant Growth Regul. 55, 165–167. doi: 10.1007/s10725-008-9276-5
Kushawaha, A. K., Khan, A., Sopory, S. K., and Sanan-Mishra, N. (2019). Light regulated osa-miR169e is implicated during priming under high temperature stress in rice. Am. J. Plant Sci. 10:1662. doi: 10.4236/ajps.2019.109118
Kushawaha, A. K., Khan, A., Sopory, S. K., and Sanan-Mishra, N. (2021). Priming by High Temperature stress induces microRNA regulated heat shock modules indicating their involvement in thermopriming response in rice. Life 11:291. doi: 10.3390/life11040291
Kwak, J. M., Mori, I. C., Pei, Z. M., Leonhardt, N., Torres, M. A., Dangl, J. L., et al. (2003). NADPH oxidase AtrbohD and AtrbohF genes function in ROS-dependent ABA signaling in Arabidopsis. EMBO J. 22, 2623–2633. doi: 10.1093/emboj/cdg277
Lämke, J., Brzezinka, K., Altmann, S., and Bäurle, I. (2016). A hit-and-run heat shock factor governs sustained histone methylation and transcriptional stress memory. EMBO J. 35, 162–175. doi: 10.15252/embj.201592593
Larkindale, J., and Knight, M. R. (2002). Protection against heat stress-induced oxidative damage in Arabidopsis involves calcium, abscisic acid, ethylene, and salicylic acid. Plant Physiol. 128, 682–695. doi: 10.1104/pp.010320
Larkindale, J., Hall, J. D., Knight, M. R., and Vierling, E. (2005). Heat stress phenotypes of Arabidopsis mutants implicate multiple signaling pathways in the acquisition of thermotolerance. Plant Physiol. 138, 882–897. doi: 10.1104/pp.105.062257
Lee, I., Aukerman, M. J., Gore, S. L., Lohman, K. N., Michaels, S. D., Weaver, L. M., et al. (1994). Isolation of LUMINIDEPENDENS: a gene involved in the control of flowering time in Arabidopsis. Plant Cell 6, 75–83. doi: 10.1105/tpc.6.1.75
Lei, Y. B., Song, S. Q., and Fu, J. R. (2005). Possible involvement of anti-oxidant enzymes in the cross-tolerance of the germination/growth of wheat seeds to salinity and heat stress. J. Integr. Plant Biol. 47, 1211–1219. doi: 10.1111/j.1744-7909.2005.00152.x
Leopold, A. C. (2014). Smart plants: memory and communication without brains. Plant Signal. Behav. 9:e972268. doi: 10.4161/15592316.2014.972268
Li, C., Jiang, D., Wollenweber, B., Li, Y., Dai, T., and Cao, W. (2011). Waterlogging pretreatment during vegetative growth improves tolerance to waterlogging after anthesis in wheat. Plant Sci. 180, 672–678. doi: 10.1016/j.plantsci.2011.01.009
Li, C., Wang, P., Wei, Z., Liang, D., Liu, C., Yin, L., et al. (2012). The mitigation effects of exogenous melatonin on salinity-induced stress in Malus hupehensis. J. Pineal Res. 53, 298–306. doi: 10.1111/j.1600-079X.2012.00999.x
Li, G., Zhang, C., Zhang, G., Fu, W., Feng, B., Chen, T., et al. (2020). Abscisic acid negatively modulates heat tolerance in rolled leaf rice by increasing leaf temperature and regulating energy homeostasis. Rice 13, 1–16. doi: 10.1186/s12284-020-00379-3
Li, G., Zhang, Y., Zhang, H., Zhang, Y., Zhao, L., Liu, Z., et al. (2019). Characteristics and regulating role in thermotolerance of the heat shock transcription factor ZmHsf12 from Zea mays L. J. Plant Biol. 62, 329–341. doi: 10.1007/s12374-019-0067-5
Li, N., Euring, D., Cha, J. Y., Lin, Z., Lu, M., Huang, L. J., et al. (2021). Plant hormone-mediated regulation of heat tolerance in response to global climate change. Front. Plant Sci. 11:2318. doi: 10.3389/fpls.2020.627969
Li, S., Liu, J., Liu, Z., Li, X., Wu, F., and He, Y. (2014). Heat-induced TAS1 TARGET1 mediates thermotolerance via heat stress transcription factor A1a–directed pathways in Arabidopsis. Plant Cell 26, 1764–1780. doi: 10.1105/tpc.114.124883
Li, X., Brestic, M., Tan, D. X., Zivcak, M., Zhu, X., Liu, S., et al. (2018). Melatonin alleviates low PS I-limited carbon assimilation under elevated CO 2 and enhances the cold tolerance of offspring in chlorophyll b-deficient mutant wheat. J. Pineal Res. 64:e12453. doi: 10.1111/jpi.12453
Li, X., Cai, J., Liu, F., Dai, T., Cao, W., and Jiang, D. (2014). Cold priming drives the sub-cellular antioxidant systems to protect photosynthetic electron transport against subsequent low temperature stress in winter wheat. Plant Physiol. Biochem. 82, 34–43. doi: 10.1016/j.plaphy.2014.05.005
Li, X., Topbjerg, H. B., Jiang, D., and Liu, F. (2015). Drought priming at vegetative stage improves the antioxidant capacity and photosynthesis performance of wheat exposed to a short-term low temperature stress at jointing stage. Plant Soil 393, 307–318. doi: 10.1007/s11104-015-2499-0
Li, X.-D., Wang, X.-L., Cai, Y.-M., Wu, J.-H., Mo, B.-T., and Yu, E.-R. (2017). Arabidopsis heat stress transcription factors A2 (HSFA2) and A3 (HSFA3) function in the same heat regulation pathway. Acta Physiol. Plant. 39:67. doi: 10.1007/s11738-017-2351-7
Li, Y., Mukherjee, I., Thum, K. E., Tanurdzic, M., Katari, M. S., Obertello, M., et al. (2015). The histone methyltransferase SDG8 mediates the epigenetic modification of light and carbon responsive genes in plants. Genome Biol. 16, 1–15. doi: 10.1186/s13059-015-0640-2
Li, Z.-G. (2016). Methylglyoxal and glyoxalase system in plants: old players, new concepts. Bot. Rev. 82, 183–203. doi: 10.1007/s12229-016-9167-9
Li, Z.-G., and Zhu, L.-P. (2015). Hydrogen sulfide donor sodium hydrosulfide-induced accumulation of betaine is involved in the acquisition of heat tolerance in maize seedlings. Braz. J. Bot. 38, 31–38. doi: 10.1007/s40415-014-0106-x
Li, Z.-G., Duan, X.-Q., Min, X., and Zhou, Z.-H. (2017a). Methylglyoxal as a novel signal molecule induces the salt tolerance of wheat by regulating the glyoxalase system, the antioxidant system, and osmolytes. Protoplasma 254, 1995–2006. doi: 10.1007/s00709-017-1094-z
Li, Z.-G., Duan, X.-Q., Xia, Y.-M., Wang, Y., Zhou, Z.-H., and Min, X. (2017b). Methylglyoxal alleviates cadmium toxicity in wheat (Triticum aestivum L). Plant Cell Rep. 36, 367–370. doi: 10.1007/s00299-016-2070-3
Light, W. H., Brickner, D. G., Brand, V. R., and Brickner, J. H. (2010). Interaction of a DNA zip code with the nuclear pore complex promotes H2A. Z incorporation and INO1 transcriptional memory. Mol. Cell 40, 112–125. doi: 10.1016/j.molcel.2010.09.007
Light, W. H., Freaney, J., Sood, V., Thompson, A., D’Urso, A., Horvath, C. M., et al. (2013). A conserved role for human Nup98 in altering chromatin structure and promoting epigenetic transcriptional memory. PLoS Biol. 11:e1001524. doi: 10.1371/journal.pbio.1001524
Liu, B., Zhang, L., Rusalepp, L., Kaurilind, E., Sulaiman, H. Y., Püssa, T., et al. (2021). Heat priming improved heat tolerance of photosynthesis, enhanced terpenoid and benzenoid emission and phenolics accumulation in Achillea millefolium. Plant Cell Environ. 44, 2365–2385. doi: 10.1111/pce.13830
Liu, H. A. (2020). Transgenerational effects of water-deficit and heat stress on germination and seedling vigour—new insights from durum wheat microRNAs. Plants 9:89. doi: 10.3390/plants9020189
Liu, H. C., Liao, H. T., and Charng, Y. Y. (2011a). The role of class A1 heat shock factors (HSFA1s) in response to heat and other stresses in Arabidopsis. Plant Cell Environ. 34, 738–751. doi: 10.1111/j.1365-3040.2011.02278.x
Liu, H., Able, A. J., and Able, J. A. (2021). Priming crops for the future: rewiring stress memory. Trends Plant Sci. S1360–1385, 321–326. doi: 10.1016/j.tplants.2021.11.015
Liu, H., Zhou, X., Dong, N., Liu, X., Zhang, H., and Zhang, Z. (2011b). Expression of a wheat MYB gene in transgenic tobacco enhances resistance to Ralstonia solanacearum, and to drought and salt stresses. Funct. Integr. Genomics 11, 431–443. doi: 10.1007/s10142-011-0228-1
Liu, H.-T., Liu, Y.-Y., Pan, Q.-H., Yang, H.-R., Zhan, J.-C., and Huang, W.-D. (2006). Novel interrelationship between salicylic acid, abscisic acid, and PIP2-specific phospholipase C in heat acclimation-induced thermotolerance in pea leaves. J. Exp. Bot. 57, 3337–3347. doi: 10.1093/jxb/erl098
Liu, J., Feng, L., Gu, X., Deng, X., Qiu, Q., Li, Q., et al. (2019). An H3K27me3 demethylase-HSFA2 regulatory loop orchestrates transgenerational thermomemory in Arabidopsis. Cell Res. 29, 379–390.
Liu, J., Feng, L., Li, J., and He, Z. (2015). Genetic and epigenetic control of plant heat responses. Front. Plant Sci. 6:267. doi: 10.3389/fpls.2015.00267
Liu, J.-X., and Howell, S. H. (2010). bZIP28 and NF-Y transcription factors are activated by ER stress and assemble into a transcriptional complex to regulate stress response genes in Arabidopsis. Plant Cell 22, 782–796. doi: 10.1105/tpc.109.072173
Liu, N., and Avramova, Z. (2016). Molecular mechanism of the priming by jasmonic acid of specific dehydration stress response genes in Arabidopsis. Epigenet. Chromatin 9, 1–23. doi: 10.1186/s13072-016-0057-5
Liu, W., Xu, L., Wang, Y., Shen, H., Zhu, X., Zhang, K., et al. (2015). Transcriptome-wide analysis of chromium-stress responsive microRNAs to explore miRNA-mediated regulatory networks in radish (Raphanus sativus L.). Sci. Rep. 5, 1–17. doi: 10.1038/srep14024
Liu, Y., Liu, K., Yin, L., Yu, Y., Qi, J., Shen, W. H., et al. (2019). H3K4me2 functions as a repressive epigenetic mark in plants. Epigenet. Chromatin 12, 1–14. doi: 10.1186/s13072-019-0285-6
Liu, Y., Zhang, C., Chen, J., Guo, L., Li, X., Li, W., et al. (2013). Arabidopsis heat shock factor HsfA1a directly senses heat stress, pH changes, and hydrogen peroxide via the engagement of redox state. Plant Physiol. Biochem. 64, 92–98. doi: 10.1016/j.plaphy.2012.12.013
Luna, E., Bruce, T. J., Roberts, M. R., Flors, V., and Ton, J. (2012). Next-generation systemic acquired resistance. Plant Physiol. 158, 844–853. doi: 10.1104/pp.111.187468
Lurie, S., and Klein, J. D. (1991). Acquisition of low-temperature tolerance in tomatoes by exposure to high-temperature stress. J. Am. Soc. Hortic. Sci. 116, 1007–1012. doi: 10.21273/JASHS.116.6.1007
Lv, W.-T., Lin, B., Zhang, M., and Hua, X.-J. (2011). Proline accumulation is inhibitory to Arabidopsis seedlings during heat stress. Plant Physiol. 156, 1921–1933. doi: 10.1104/pp.111.175810
Ma, D., Li, X., Guo, Y., Chu, J., Fang, S., Yan, C., et al. (2016). Cryptochrome 1 interacts with PIF4 to regulate high temperature-mediated hypocotyl elongation in response to blue light. Proc. Natl. Acad. Sci. U.S.A. 113, 224–229. doi: 10.1073/pnas.1511437113
Ma, Q. J., Sun, M. H., Kang, H., Lu, J., You, C. X., and Hao, Y. J. (2019). A CIPK protein kinase targets sucrose transporter MdSUT2. 2 at Ser254 for phosphorylation to enhance salt tolerance. Plant Cell Environ. 42, 918–930. doi: 10.1111/pce.13349
Majláth, I., Éva, C., Tajti, J., Khalil, R., Elsayed, N., Darko, E., et al. (2020). Exogenous methylglyoxal enhances the reactive aldehyde detoxification capability and frost-hardiness of wheat. Plant Physiol. Biochem. 149, 75–85. doi: 10.1016/j.plaphy.2020.02.003
Makarova, S., Makhotenko, A., Spechenkova, N., Love, A. J., Kalinina, N. O., and Taliansky, M. (2018). Interactive responses of potato (Solanum tuberosum L.) plants to heat stress and infection with potato virus Y. Front. Microbiol. 9:2582. doi: 10.3389/fmicb.2018.02582
Martel, A. B., and Qaderi, M. M. (2016). Does salicylic acid mitigate the adverse effects of temperature and ultraviolet-B radiation on pea (Pisum sativum) plants? Environ. Exp. Bot. 122, 39–48. doi: 10.1016/j.envexpbot.2015.09.002
Martinez-Medina, A., Flors, V., Heil, M., Mauch-Mani, B., Pieterse, C. M., Pozo, M. J., et al. (2016). Recognizing plant defense priming. Trends Plant Sci. 21, 818–822. doi: 10.1016/j.tplants.2016.07.009
Maszkowska, J., Dêbski, J., Kulik, A., Kistowski, M., Bucholc, M., Lichocka, M., et al. (2019). Phosphoproteomic analysis reveals that dehydrins ERD10 and ERD14 are phosphorylated by SNF1-related protein kinase 2.10 in response to osmotic stress. Plant Cell Environ. 42, 931–946. doi: 10.1111/pce.13465
Maya, M. A., and Matsubara, Y.-I. (2013). Influence of arbuscular mycorrhiza on the growth and antioxidative activity in cyclamen under heat stress. Mycorrhiza 23, 381–390. doi: 10.1007/s00572-013-0477-z
Mazorra, L. M., Holton, N., Bishop, G. J., and Núñez, M. (2011). Heat shock response in tomato brassinosteroid mutants indicates that thermotolerance is independent of brassinosteroid homeostasis. Plant Physiol. Biochem. 49, 1420–1428. doi: 10.1016/j.plaphy.2011.09.005
McLellan, C. A., Turbyville, T. J., Wijeratne, E. K., Kerschen, A., Vierling, E., Queitsch, C., et al. (2007). A rhizosphere fungus enhances Arabidopsis thermotolerance through production of an HSP90 inhibitor. Plant Physiol. 145, 174–182. doi: 10.1104/pp.107.101808
Mei, Y. Q., and Song, S. Q. (2010). Response to temperature stress of reactive oxygen species scavenging enzymes in the cross-tolerance of barley seed germination. J. Zhejiang Univ. Sci. B 11, 965–972. doi: 10.1631/jzus.B1000147
Meiri, D., and Breiman, A. (2009). Arabidopsis ROF1 (FKBP62) modulates thermotolerance by interacting with HSP90. 1 and affecting the accumulation of HsfA2-regulated sHSPs. Plant J. 59, 387–399. doi: 10.1111/j.1365-313X.2009.03878.x
Mendanha, T., Rosenqvist, E., Hyldgaard, B., and Ottosen, C.-O. (2018). Heat priming effects on anthesis heat stress in wheat cultivars (Triticum aestivum L.) with contrasting tolerance to heat stress. Plant Physiol. Biochem. 132, 213–221. doi: 10.1016/j.plaphy.2018.09.002
Meng, J. F., Xu, T. F., Wang, Z. Z., Fang, Y. L., Xi, Z. M., and Zhang, Z. W. (2014). The ameliorative effects of exogenous melatonin on grape cuttings under water-deficient stress: antioxidant metabolites, leaf anatomy, and chloroplast morphology. J. Pineal Res. 57, 200–212. doi: 10.1111/jpi.12159
Migicovsky, Z., Yao, Y., and Kovalchuk, I. (2014). Transgenerational phenotypic and epigenetic changes in response to heat stress in Arabidopsis thaliana. Plant Signal. Behav. 9:e27971. doi: 10.4161/psb.27971
Mittal, D., Chakrabarti, S., Sarkar, A., Singh, A., and Grover, A. (2009). Heat shock factor gene family in rice: genomic organization and transcript expression profiling in response to high temperature, low temperature and oxidative stresses. Plant Physiol. Biochem. 47, 785–795. doi: 10.1016/j.plaphy.2009.05.003
Mittler, R., and Blumwald, E. (2015). The roles of ROS and ABA in systemic acquired acclimation. Plant Cell 27, 64–70. doi: 10.1105/tpc.114.133090
Mittler, R., Finka, A., and Goloubinoff, P. (2012). How do plants feel the heat? Trends Biochem. Sci. 37, 118–125. doi: 10.1016/j.tibs.2011.11.007
Mittler, R., Vanderauwera, S., Gollery, M., and Van Breusegem, F. (2004). Reactive oxygen gene network of plants. Trends Plant Sci. 9, 490–498. doi: 10.1016/j.tplants.2004.08.009
Monaghan, J., and Zipfel, C. (2012). Plant pattern recognition receptor complexes at the plasma membrane. Curr. Opin. Plant Biol. 15, 349–357. doi: 10.1016/j.pbi.2012.05.006
Mozgova, I., Wildhaber, T., Liu, Q., Abou-Mansour, E., L’Haridon, F., Metraux, J. P., et al. (2015). Chromatin assembly factor CAF-1 represses priming of plant defence response genes. Nat. Plants 1, 1–8. doi: 10.1038/nplants.2015.127
Munné-Bosch, S., and Alegre, L. (2013). Cross-stress tolerance and stress “memory” in plants. Environ. Exp. Bot. 94, 1–88. doi: 10.1016/j.envexpbot.2013.02.002
Nahar, K., Hasanuzzaman, M., Alam, M. M., and Fujita, M. (2015). Exogenous glutathione confers high temperature stress tolerance in mung bean (Vigna radiata L.) by modulating antioxidant defense and methylglyoxal detoxification system. Environ. Exp. Bot. 112, 44–54. doi: 10.1016/j.envexpbot.2014.12.001
Nakagami, H., Pitzschke, A., and Hirt, H. (2005). Emerging MAP kinase pathways in plant stress signalling. Trends Plant Sci. 10, 339–346. doi: 10.1016/j.tplants.2005.05.009
Nakashima, K., Tran, L. S. P., Van Nguyen, D., Fujita, M., Maruyama, K., Todaka, D., et al. (2007). Functional analysis of a NAC-type transcription factor OsNAC6 involved in abiotic and biotic stress-responsive gene expression in rice. Plant J. 51, 617–630. doi: 10.1111/j.1365-313X.2007.03168.x
Narayanan, S., Tamura, P. J., Roth, M. R., Prasad, P. V., and Welti, R. (2016). Wheat leaf lipids during heat stress: I. High day and night temperatures result in major lipid alterations. Plant Cell Environ. 39, 787–803. doi: 10.1111/pce.12649
Naydenov, M., Baev, V., Apostolova, E., Gospodinova, N., Sablok, G., Gozmanova, M., et al. (2015). High-temperature effect on genes engaged in DNA methylation and affected by DNA methylation in Arabidopsis. Plant Physiol. Biochem. 87, 102–108. doi: 10.1016/j.plaphy.2014.12.022
Newby, G. A., and Lindquist, S. (2013). Blessings in disguise: biological benefits of prion-like mechanisms. Trends Cell Biol. 23, 251–259. doi: 10.1016/j.tcb.2013.01.007
Nie, W. F., Wang, M. M., Xia, X. J., Zhou, Y. H., Shi, K., Chen, Z., et al. (2013). Silencing of tomato RBOH1 and MPK2 abolishes brassinosteroid-induced H2O2 generation and stress tolerance. Plant Cell Environ. 36, 789–803. doi: 10.1111/pce.12014
Niu, J. H., Ahmad Anjum, S., Wang, R., Li, J. H., Liu, M. R., Song, J. X., et al. (2016). Exogenous application of brassinolide can alter morphological and physiological traits of Leymus chinensis (Trin.) Tzvelev under room and high temperatures. Chilean J. Agric. Res. 76, 27–33. doi: 10.4067/S0718-58392016000100004
Nožková, V., Mieslerova, B., Luhova, L., Piterkova, J., Novák, O., Špundová, M., et al. (2018). Effect of heat-shock pre-treatment on tomato plants infected by powdery mildew fungus. Plant Prot. Sci. 55, 31–42. doi: 10.17221/24/2018-PPS
Ohama, N., Sato, H., Shinozaki, K., and Yamaguchi-Shinozaki, K. (2017). Transcriptional regulatory network of plant heat stress response. Trends Plant Sci. 22, 53–65. doi: 10.1016/j.tplants.2016.08.015
Omoarelojie, L. O., Kulkarni, M. G., Finnie, J. F., Pospíšil, T., Strnad, M., and Van Staden, J. (2020). Synthetic strigolactone (rac-GR24) alleviates the adverse effects of heat stress on seed germination and photosystem II function in lupine seedlings. Plant Physiol. Biochem. 155, 965–979. doi: 10.1016/j.plaphy.2020.07.043
Oostendorp, M., Kunz, W., Dietrich, B., and Staub, T. (2001). Induced disease resistance in plants by chemicals. Eur. J. Plant Pathol. 107, 19–28. doi: 10.1023/A:1008760518772
Orzech, K., and Burke, J. (1988). Heat shock and the protection against metal toxicity in wheat leaves 1. Plant Cell Environ. 11, 711–714. doi: 10.1111/j.1365-3040.1988.tb01154.x
Oshino, T., Miura, S., Kikuchi, S., Hamada, K., Yano, K., Watanabe, M., et al. (2011). Auxin depletion in barley plants under high-temperature conditions represses DNA proliferation in organelles and nuclei via transcriptional alterations. Plant Cell Environ. 34, 284–290. doi: 10.1111/j.1365-3040.2010.02242.x
Palma, K., Thorgrimsen, S., Malinovsky, F. G., Fiil, B. K., Nielsen, H. B., Brodersen, P., et al. (2010). Autoimmunity in Arabidopsis acd11 is mediated by epigenetic regulation of an immune receptor. PLoS Pathog. 6:e1001137. doi: 10.1371/journal.ppat.1001137
Pan, C., Ye, L., Zheng, Y., Wang, Y., Yang, D., Liu, X., et al. (2017). Identification and expression profiling of microRNAs involved in the stigma exsertion under high-temperature stress in tomato. BMC Genomics 18:843. doi: 10.1186/s12864-017-4238-9
Pandey, P., Irulappan, V., Bagavathiannan, M. V., and Senthil-Kumar, M. (2017). Impact of combined abiotic and biotic stresses on plant growth and avenues for crop improvement by exploiting physio-morphological traits. Front. Plant Sci. 8:537. doi: 10.3389/fpls.2017.00537
Peng, J., Li, Z., Wen, X., Li, W., Shi, H., Yang, L., et al. (2014). Salt-induced stabilization of EIN3/EIL1 confers salinity tolerance by deterring ROS accumulation in Arabidopsis. PLoS Genet. 10:e1004664. doi: 10.1371/journal.pgen.1004664
Pérez-Salamó, I., Papdi, C., Rigó, G., Zsigmond, L., Vilela, B., Lumbreras, V., et al. (2014). The heat shock factor A4A confers salt tolerance and is regulated by oxidative stress and the mitogen-activated protein kinases MPK3 and MPK6. Plant Physiol. 165, 319–334. doi: 10.1104/pp.114.237891
Pham, V. N., Kathare, P. K., and Huq, E. (2018). Phytochromes and phytochrome interacting factors. Plant Physiol. 176, 1025–1038. doi: 10.1104/pp.17.01384
Pieterse, C. M., Zamioudis, C., Berendsen, R. L., Weller, D. M., Van Wees, S. C., and Bakker, P. A. (2014). Induced systemic resistance by beneficial microbes. Annu. Rev. Phytopathol. 52, 347–375. doi: 10.1146/annurev-phyto-082712-102340
Prasad, P. V., Bheemanahalli, R., and Jagadish, S. K. (2017). Field crops and the fear of heat stress—opportunities, challenges and future directions. Field Crops Res. 200, 114–121. doi: 10.1016/j.fcr.2016.09.024
Prerostova, S., Dobrev, P. I., Kramna, B., Gaudinova, A., Knirsch, V., Spichal, L., et al. (2020). Heat acclimation and inhibition of cytokinin degradation positively affect heat stress tolerance of Arabidopsis. Front. Plant Sci. 11:87. doi: 10.3389/fpls.2020.00087
Qiu, Y., and Yu, D. (2009). Over-expression of the stress-induced OsWRKY45 enhances disease resistance and drought tolerance in Arabidopsis. Environ. Exp. Bot. 65, 35–47. doi: 10.1016/j.envexpbot.2008.07.002
Qiu, Z., Guo, J., Zhu, A., Zhang, L., and Zhang, M. (2014). Exogenous jasmonic acid can enhance tolerance of wheat seedlings to salt stress. Ecotoxicol. Environ. Saf. 104, 202–208. doi: 10.1016/j.ecoenv.2014.03.014
Qu, A.-L., Ding, Y.-F., Jiang, Q., and Zhu, C. (2013). Molecular mechanisms of the plant heat stress response. Biochem. Biophys. Res. Commun. 432, 203–207. doi: 10.1016/j.bbrc.2013.01.104
Quint, M., Delker, C., Franklin, K. A., Wigge, P. A., Halliday, K. J., and Van Zanten, M. (2016). Molecular and genetic control of plant thermomorphogenesis. Nat. Plants 2, 1–9. doi: 10.1038/nplants.2015.190
Raghuram, B., Sheikh, A. H., and Sinha, A. K. (2014). Regulation of MAP kinase signaling cascade by microRNAs in Oryza sativa. Plant Signal. Behav. 9:e972130. doi: 10.4161/psb.29804
Ramirez-Prado, J. S., Abulfaraj, A. A., Rayapuram, N., Benhamed, M., and Hirt, H. (2018). Plant immunity: from signaling to epigenetic control of defense. Trends Plant Sci. 23, 833–844. doi: 10.1016/j.tplants.2018.06.004
Rasheed, R., Wahid, A., Farooq, M., Hussain, I., and Basra, S. M. (2011). Role of proline and glycinebetaine pretreatments in improving heat tolerance of sprouting sugarcane (Saccharum sp.) buds. Plant Growth Regul. 65, 35–45. doi: 10.1007/s10725-011-9572-3
Redman, R. S., Sheehan, K. B., Stout, R. G., Rodriguez, R. J., and Henson, J. M. (2002). Thermotolerance generated by plant/fungal symbiosis. Science 298, 1581–1581. doi: 10.1126/science.1078055
Rezaul, I. M., Baohua, F., Tingting, C., Weimeng, F., Caixia, Z., Longxing, T., et al. (2019). Abscisic acid prevents pollen abortion under high-temperature stress by mediating sugar metabolism in rice spikelets. Physiol. Plant. 165, 644–663. doi: 10.1111/ppl.12759
Rodríguez, V. M., Soengas, P., Alonso-Villaverde, V., Sotelo, T., Cartea, M. E., and Velasco, P. (2015). Effect of temperature stress on the early vegetative development of Brassica oleracea L. BMC Plant Biol. 15:145. doi: 10.1186/s12870-015-0535-0
Rozenzvieg, D., Elmaci, C., Samach, A., Lurie, S., and Porat, R. (2004). Isolation of four heat shock protein cDNAs from grapefruit peel tissue and characterization of their expression in response to heat and chilling temperature stresses. Physiol. Plant. 121, 421–428. doi: 10.1111/j.1399-3054.2004.00334.x
Ruthenburg, A. J., Allis, C. D., and Wysocka, J. (2007). Methylation of lysine 4 on histone H3: intricacy of writing and reading a single epigenetic mark. Mol. Cell 25, 15–30. doi: 10.1016/j.molcel.2006.12.014
Sabehat, A., Weiss, D., and Lurie, S. (1996). The correlation between heat-shock protein accumulation and persistence and chilling tolerance in tomato fruit. Plant Physiol. 110, 531–537. doi: 10.1104/pp.110.2.531
Sadura, I., Libik-Konieczny, M., Jurczyk, B., Gruszka, D., and Janeczko, A. (2020). Plasma membrane ATPase and the aquaporin HvPIP1 in barley brassinosteroid mutants acclimated to high and low temperature. J. Plant Physiol. 244:153090. doi: 10.1016/j.jplph.2019.153090
Sah, S. K., Reddy, K. R., and Li, J. (2016). Abscisic acid and abiotic stress tolerance in crop plants. Front. Plant Sci. 7:571. doi: 10.3389/fpls.2016.00571
Sakata, T., Oshino, T., Miura, S., Tomabechi, M., Tsunaga, Y., Higashitani, N., et al. (2010). Auxins reverse plant male sterility caused by high temperatures. Proc. Natl. Acad. Sci. U.S.A. 107, 8569–8574. doi: 10.1073/pnas.1000869107
Saleh, A. A., Abdel-Kader, D. Z., and El Elish, A. M. (2007). Role of heat shock and salicylic acid in antioxidant homeostasis in mungbean (Vigna radiata L.) plant subjected to heat stress. Am. J. Plant Physiol. 2, 344–355. doi: 10.3923/ajpp.2007.344.355
Sanchez, D. H., and Paszkowski, J. (2014). Heat-induced release of epigenetic silencing reveals the concealed role of an imprinted plant gene. PLoS Genet. 10:e1004806. doi: 10.1371/journal.pgen.1004806
Sarwar, M., Saleem, M. F., Ullah, N., Rizwan, M., Ali, S., Shahid, M. R., et al. (2018). Exogenously applied growth regulators protect the cotton crop from heat-induced injury by modulating plant defense mechanism. Sci. Rep. 8, 1–15. doi: 10.1038/s41598-018-35420-5
Sato, H., Mizoi, J., Tanaka, H., Maruyama, K., Qin, F., Osakabe, Y., et al. (2014). Arabidopsis DPB3-1, a DREB2A interactor, specifically enhances heat stress-induced gene expression by forming a heat stress-specific transcriptional complex with NF-Y subunits. Plant Cell 26, 4954–4973. doi: 10.1105/tpc.114.132928
Sato, Y., Murakami, T., Funatsuki, H., Matsuba, S., Saruyama, H., and Tanida, M. (2001). Heat shock-mediated APX gene expression and protection against chilling injury in rice seedlings. J. Exp. Bot. 52, 145–151. doi: 10.1093/jexbot/52.354.145
Scharf, K.-D., Berberich, T., Ebersberger, I., and Nover, L. (2012). The plant heat stress transcription factor (Hsf) family: structure, function and evolution. Biochim. Biophys. Acta BBA Gene Regul. Mechanisms 1819, 104–119. doi: 10.1016/j.bbagrm.2011.10.002
Scherzer, S., Shabala, L., Hedrich, B., Fromm, J., Bauer, H., Munz, E., et al. (2017). Insect haptoelectrical stimulation of Venus flytrap triggers exocytosis in gland cells. Proc. Natl. Acad. Sci. U.S.A. 114, 4822–4827. doi: 10.1073/pnas.1701860114
Sedaghatmehr, M., Mueller-Roeber, B., and Balazadeh, S. (2016). The plastid metalloprotease FtsH6 and small heat shock protein HSP21 jointly regulate thermomemory in Arabidopsis. Nat. Commun. 7, 1–14. doi: 10.1038/ncomms12439
Serrano, N., Ling, Y., Bahieldin, A., and Mahfouz, M. M. (2019). Thermopriming reprograms metabolic homeostasis to confer heat tolerance. Sci. Rep. 9, 1–14. doi: 10.1038/s41598-018-36484-z
Shahnejat-Bushehri, S., Mueller-Roeber, B., and Balazadeh, S. (2012). Arabidopsis NAC transcription factor JUNGBRUNNEN1 affects thermomemory-associated genes and enhances heat stress tolerance in primed and unprimed conditions. Plant Signal. Behav. 7, 1518–1521. doi: 10.4161/psb.22092
Sharma, D., Verma, N., Pandey, C., Verma, D., Bhagat, P. K., Noryang, S., et al. (2020). “MAP kinase as regulators for stress responses in plants: an overview,” in Protein Kinases And Stress Signaling In Plants, ed. G. K. Pandey (Hoboken, NJ: Wiley), 369–392. doi: 10.1002/9781119541578.ch15
Shekhawat, K., Saad, M. M., Sheikh, A., Mariappan, K., Al-Mahmoudi, H., Abdulhakim, F., et al. (2021). Root endophyte induced plant thermotolerance by constitutive chromatin modification at heat stress memory gene loci. EMBO Rep. 22:e51049. doi: 10.15252/embr.202051049
Shi, H., Tan, D. X., Reiter, R. J., Ye, T., Yang, F., and Chan, Z. (2015b). Melatonin induces class A1 heat-shock factors (HSFA 1s) and their possible involvement of thermotolerance in Arabidopsis. J. Pineal Res. 58, 335–342. doi: 10.1111/jpi.12219
Shi, H., Qian, Y., Tan, D. X., Reiter, R. J., and He, C. (2015a). Melatonin induces the transcripts of CBF/DREB1s and their involvement in both abiotic and biotic stresses in Arabidopsis. J. Pineal Res. 59, 334–342. doi: 10.1111/jpi.12262
Shi, H., Wang, X., Ye, T., Chen, F., Deng, J., Yang, P., et al. (2014). The Cysteine2/Histidine2-type transcription factor zinc finger of Arabidopsis thaliana 6 modulates biotic and abiotic stress responses by activating salicylic acid-related genes and C-repeat-binding factor genes in Arabidopsis. Plant Physiol. 165, 1367–1379. doi: 10.1104/pp.114.242404
Shi, W., Lawas, L., Raju, B., and Jagadish, S. (2016). Acquired thermo-tolerance and trans-generational heat stress response at flowering in rice. J. Agron. Crop Sci. 202, 309–319. doi: 10.1111/jac.12157
Shorter, J., and Lindquist, S. (2005). Prions as adaptive conduits of memory and inheritance. Nat. Rev. Genet. 6, 435–450. doi: 10.1038/nrg1616
Silva-Correia, J., Freitas, S., Tavares, R. M., Lino-Neto, T., and Azevedo, H. (2014). Phenotypic analysis of the Arabidopsis heat stress response during germination and early seedling development. Plant Methods 10, 1–11. doi: 10.1186/1746-4811-10-7
Singh, A. K., Dhanapal, S., Finkelshtein, A., and Chamovitz, D. A. (2021). CSN5A subunit of COP9 signalosome is required for resetting transcriptional stress memory after recurrent heat stress in Arabidopsis. Biomolecules 11:668. doi: 10.3390/biom11050668
Sinha, A. K., Jaggi, M., Raghuram, B., and Tuteja, N. (2011). Mitogen-activated protein kinase signaling in plants under abiotic stress. Plant Signal. Behav. 6, 196–203. doi: 10.4161/psb.6.2.14701
Skalák, J., Černý, M., Jedelský, P., Dobrá, J., Ge, E., Novák, J., et al. (2016). Stimulation of ipt overexpression as a tool to elucidate the role of cytokinins in high temperature responses of Arabidopsis thaliana. J. Exp. Bot. 67, 2861–2873. doi: 10.1093/jxb/erw129
Slaughter, A., Daniel, X., Flors, V., Luna, E., Hohn, B., and Mauch-Mani, B. (2012). Descendants of primed Arabidopsis plants exhibit resistance to biotic stress. Plant Physiol. 158, 835–843. doi: 10.1104/pp.111.191593
Song, L., Jiang, Y., Zhao, H., and Hou, M. (2012). Acquired thermotolerance in plants. Plant Cell Tissue Organ Cult. 111, 265–276. doi: 10.1007/s11240-012-0198-6
Song, Z.-T., Sun, L., Lu, S.-J., Tian, Y., Ding, Y., and Liu, J.-X. (2015). Transcription factor interaction with COMPASS-like complex regulates histone H3K4 trimethylation for specific gene expression in plants. Proc. Natl. Acad. Sci. U.S.A. 112, 2900–2905. doi: 10.1073/pnas.1419703112
Stief, A., Altmann, S., Hoffmann, K., Pant, B. D., Scheible, W.-R., and Bäurle, I. (2014). Arabidopsis miR156 regulates tolerance to recurring environmental stress through SPL transcription factors. Plant Cell 26, 1792–1807. doi: 10.1105/tpc.114.123851
Streb, P., Aubert, S., Gout, E., Feierabend, J., and Bligny, R. (2008). Cross tolerance to heavy-metal and cold-induced photoinhibiton in leaves of Pisum sativum acclimated to low temperature. Physiol. Mol. Biol. Plants 14, 185–193. doi: 10.1007/s12298-008-0018-y
Su, Y., Huang, Y., Dong, X., Wang, R., Tang, M., Cai, J., et al. (2021). Exogenous methyl jasmonate improves heat tolerance of perennial ryegrass through alteration of osmotic adjustment, antioxidant defense, and expression of jasmonic acid-responsive genes. Front. Plant Sci. 12:664519. doi: 10.3389/fpls.2021.664519
Sun, J., Qi, L., Li, Y., Chu, J., and Li, C. (2012). PIF4–mediated activation of YUCCA8 expression integrates temperature into the auxin pathway in regulating Arabidopsis hypocotyl growth. PLoS Genet. 8:e1002594. doi: 10.1371/journal.pgen.1002594
Suter, L., and Widmer, A. (2013). Environmental heat and salt stress induce transgenerational phenotypic changes in Arabidopsis thaliana. PLoS One 8:e60364. doi: 10.1371/journal.pone.0060364
Suzuki, N., Bassil, E., Hamilton, J. S., Inupakutika, M. A., Zandalinas, S. I., Tripathy, D., et al. (2016). ABA is required for plant acclimation to a combination of salt and heat stress. PLoS One 11:e0147625. doi: 10.1371/journal.pone.0147625
Suzuki, N., Devireddy, A. R., Inupakutika, M. A., Baxter, A., Miller, G., Song, L., et al. (2015). Ultra-fast alterations in mRNA levels uncover multiple players in light stress acclimation in plants. Plant J. 84, 760–772. doi: 10.1111/tpj.13039
Suzuki, N., Koussevitzky, S., Mittler, R., and Miller, G. (2012). ROS and redox signalling in the response of plants to abiotic stress. Plant Cell Environ. 35, 259–270. doi: 10.1111/j.1365-3040.2011.02336.x
Suzuki, T., Inagaki, S., Nakajima, S., Akashi, T., Ohto, M. A., Kobayashi, M., et al. (2004). A novel Arabidopsis gene TONSOKU is required for proper cell arrangement in root and shoot apical meristems. Plant J. 38, 673–684. doi: 10.1111/j.1365-313X.2004.02074.x
Swindell, W. R., Huebner, M., and Weber, A. P. (2007). Transcriptional profiling of Arabidopsis heat shock proteins and transcription factors reveals extensive overlap between heat and non-heat stress response pathways. BMC Genomics 8:125. doi: 10.1186/1471-2164-8-125
Szabados, L., and Savouré, A. (2010). Proline: a multifunctional amino acid. Trends Plant Sci. 15, 89–97. doi: 10.1016/j.tplants.2009.11.009
Takahashi, F., Yoshida, R., Ichimura, K., Mizoguchi, T., Seo, S., Yonezawa, M., et al. (2007). The mitogen-activated protein kinase cascade MKK3–MPK6 is an important part of the jasmonate signal transduction pathway in Arabidopsis. Plant Cell 19, 805–818. doi: 10.1105/tpc.106.046581
Takeda, S., Tadele, Z., Hofmann, I., Probst, A. V., Angelis, K. J., Kaya, H., et al. (2004). BRU1, a novel link between responses to DNA damage and epigenetic gene silencing in Arabidopsis. Genes Dev. 18, 782–793. doi: 10.1101/gad.295404
Tan, D. X., Hardeland, R., Manchester, L. C., Korkmaz, A., Ma, S., Rosales-Corral, S., et al. (2012). Functional roles of melatonin in plants, and perspectives in nutritional and agricultural science. J. Exp. Bot. 63, 577–597. doi: 10.1093/jxb/err256
Tang, R.-S., Zheng, J.-C., Jin, Z.-Q., Zhang, D.-D., Huang, Y.-H., and Chen, L.-G. (2008). Possible correlation between high temperature-induced floret sterility and endogenous levels of IAA, GAs and ABA in rice (Oryza sativa L.). Plant Growth Regul. 54, 37–43. doi: 10.1007/s10725-007-9225-8
Terrile, M., Iglesias, M., Casalongué, C., and París, R. (2020). “Reactive nitrogen species mediated cross-stress tolerance in plants,” in Priming-Mediated Stress and Cross-Stress Tolerance in Crop Plants, eds B. Huang, D. J. Burritt, F. Liu, M. Fujita, and M. A. Hossain (Amsterdam: Elsevier), 133–148. doi: 10.1016/B978-0-12-817892-8.00008-8
Thirumalaikumar, V. P., Gorka, M., Schulz, K., Masclaux-Daubresse, C., Sampathkumar, A., Skirycz, A., et al. (2021). Selective autophagy regulates heat stress memory in Arabidopsis by NBR1-mediated targeting of HSP90. 1 and ROF1. Autophagy 17, 2184–2199. doi: 10.1080/15548627.2020.1820778
Thoma, F., Koller, T., and Klug, A. (1979). Involvement of histone H1 in the organization of the nucleosome and of the salt-dependent superstructures of chromatin. J. Cell Biol. 83, 403–427. doi: 10.1083/jcb.83.2.403
Thomashow, M. F. (1999). Plant cold acclimation: freezing tolerance genes and regulatory mechanisms. Annu. Rev. Plant Biol. 50, 571–599. doi: 10.1146/annurev.arplant.50.1.571
Timperio, A. M., Egidi, M. G., and Zolla, L. (2008). Proteomics applied on plant abiotic stresses: role of heat shock proteins (HSP). J. Proteomics 71, 391–411. doi: 10.1016/j.jprot.2008.07.005
Ton, J., Jakab, G., Toquin, V., Flors, V., Iavicoli, A., Maeder, M. N., et al. (2005). Dissecting the β-aminobutyric acid–induced priming phenomenon in Arabidopsis. Plant Cell 17, 987–999. doi: 10.1105/tpc.104.029728
Trevaskis, B., Bagnall, D. J., Ellis, M. H., Peacock, W. J., and Dennis, E. S. (2003). MADS box genes control vernalization-induced flowering in cereals. Proc. Natl. Acad. Sci. U.S.A. 100, 13099–13104. doi: 10.1073/pnas.1635053100
Upadhyaya, C. P., Venkatesh, J., Gururani, M. A., Asnin, L., Sharma, K., Ajappala, H., et al. (2011). Transgenic potato overproducing L-ascorbic acid resisted an increase in methylglyoxal under salinity stress via maintaining higher reduced glutathione level and glyoxalase enzyme activity. Biotechnol. Lett. 33, 2297–2307. doi: 10.1007/s10529-011-0684-7
Van Aken, O., De Clercq, I., Ivanova, A., Law, S. R., Van Breusegem, F., Millar, A. H., et al. (2016). Mitochondrial and chloroplast stress responses are modulated in distinct touch and chemical inhibition phases. Plant Physiol. 171, 2150–2165. doi: 10.1104/pp.16.00273
Vanková, R., Kosová, K., Dobrev, P., Vítámvás, P., Trávníčková, A., Cvikrová, M., et al. (2014). Dynamics of cold acclimation and complex phytohormone responses in Triticum monococcum lines G3116 and DV92 differing in vernalization and frost tolerance level. Environ. Exp. Bot. 101, 12–25. doi: 10.1016/j.envexpbot.2014.01.002
Vaucheret, H., Mallory, A. C., and Bartel, D. P. (2006). AGO1 homeostasis entails coexpression of MIR168 and AGO1 and preferential stabilization of miR168 by AGO1. Mol. Cell 22, 129–136. doi: 10.1016/j.molcel.2006.03.011
Verbruggen, N., and Hermans, C. (2008). Proline accumulation in plants: a review. Amino Acids 35, 753–759. doi: 10.1007/s00726-008-0061-6
Verma, V., Ravindran, P., and Kumar, P. P. (2016). Plant hormone-mediated regulation of stress responses. BMC Plant Biol. 16:86. doi: 10.1186/s12870-016-0771-y
von Koskull-Döring, P., Scharf, K.-D., and Nover, L. (2007). The diversity of plant heat stress transcription factors. Trends Plant Sci. 12, 452–457. doi: 10.1016/j.tplants.2007.08.014
Vu, W. T., Chang, P. L., Moriuchi, K. S., and Friesen, M. L. (2015). Genetic variation of transgenerational plasticity of offspring germination in response to salinity stress and the seed transcriptome of Medicago truncatula. BMC Evol. Biol. 15:59. doi: 10.1186/s12862-015-0322-4
Wahid, A., Gelani, S., Ashraf, M., and Foolad, M. R. (2007). Heat tolerance in plants: an overview. Environ. Exp. Bot. 61, 199–223. doi: 10.1016/j.envexpbot.2007.05.011
Walter, J., Nagy, L., Hein, R., Rascher, U., Beierkuhnlein, C., Willner, E., et al. (2011). Do plants remember drought? Hints towards a drought-memory in grasses. Environ. Exp. Bot. 71, 34–40. doi: 10.1016/j.envexpbot.2010.10.020
Wan, S. B., Tian, L., Tian, R. R., Pan, Q. H., Zhan, J. C., Wen, P. F., et al. (2009). Involvement of phospholipase D in the low temperature acclimation-induced thermotolerance in grape berry. Plant Physiol. Biochem. 47, 504–510. doi: 10.1016/j.plaphy.2008.12.010
Wang, K., Zhang, X., and Ervin, E. (2013). Effects of nitrate and cytokinin on creeping bentgrass under supraoptimal temperatures. J. Plant Nutr. 36, 1549–1564. doi: 10.1080/01904167.2013.799184
Wang, M., Yue, H., Feng, K., Deng, P., Song, W., and Nie, X. (2016). Genome-wide identification, phylogeny and expressional profiles of mitogen activated protein kinase kinase kinase (MAPKKK) gene family in bread wheat (Triticum aestivum L.). BMC Genomics 17:668. doi: 10.1186/s12864-016-2993-7
Wang, P., Zhao, Y., Li, Z., Hsu, C. C., Liu, X., Fu, L., et al. (2018). Reciprocal regulation of the TOR kinase and ABA receptor balances plant growth and stress response. Mol. Cell 69, 100–112. doi: 10.1016/j.molcel.2017.12.002
Wang, R., Zhang, Y., Kieffer, M., Yu, H., Kepinski, S., and Estelle, M. (2016). HSP90 regulates temperature-dependent seedling growth in Arabidopsis by stabilizing the auxin co-receptor F-box protein TIR1. Nat. Commun. 7, 1–11. doi: 10.1038/ncomms10269
Wang, X., Cai, J., Liu, F., Dai, T., Cao, W., Wollenweber, B., et al. (2014a). Multiple heat priming enhances thermo-tolerance to a later high temperature stress via improving subcellular antioxidant activities in wheat seedlings. Plant Physiol. Biochem. 74, 185–192. doi: 10.1016/j.plaphy.2013.11.014
Wang, X., Vignjevic, M., Jiang, D., Jacobsen, S., and Wollenweber, B. (2014b). Improved tolerance to drought stress after anthesis due to priming before anthesis in wheat (Triticum aestivum L.) var. Vinjett. J. Exp. Bot. 65, 6441–6456. doi: 10.1093/jxb/eru362
Wang, X., Cai, J., Liu, F., Jin, M., Yu, H., Jiang, D., et al. (2012). Pre-anthesis high temperature acclimation alleviates the negative effects of post-anthesis heat stress on stem stored carbohydrates remobilization and grain starch accumulation in wheat. J. Cereal Sci. 55, 331–336. doi: 10.1016/j.jcs.2012.01.004
Wang, X., Li, Z., Liu, B., Zhou, H., Elmongy, M. S., and Xia, Y. (2020). Combined proteome and transcriptome analysis of heat-primed azalea reveals new insights into plant heat acclimation memory. Front. Plant Sci. 11:1278. doi: 10.3389/fpls.2020.01278
Wang, X., Vignjevic, M., Liu, F., Jacobsen, S., Jiang, D., and Wollenweber, B. (2015). Drought priming at vegetative growth stages improves tolerance to drought and heat stresses occurring during grain filling in spring wheat. Plant Growth Regul. 75, 677–687. doi: 10.1007/s10725-014-9969-x
Wang, X., Xin, C., Cai, J., Zhou, Q., Dai, T., Cao, W., et al. (2016). Heat priming induces trans-generational tolerance to high temperature stress in wheat. Front. Plant Sci. 7:501. doi: 10.3389/fpls.2016.00501
Wang, X., Zhang, X., Chen, J., Wang, X., Cai, J., Zhou, Q., et al. (2018). Parental drought-priming enhances tolerance to post-anthesis drought in offspring of wheat. Front. Plant Sci. 9:261. doi: 10.3389/fpls.2018.00261
Wang, X., Zhuang, L., Shi, Y., and Huang, B. (2017). Up-regulation of HSFA2c and HSPs by ABA contributing to improved heat tolerance in tall fescue and Arabidopsis. Int. J. Mol. Sci. 18:1981. doi: 10.3390/ijms18091981
Wang, Y., Sun, F., Cao, H., Peng, H., Ni, Z., Sun, Q., et al. (2012). TamiR159 directed wheat TaGAMYB cleavage and its involvement in anther development and heat response. PLoS One 7:e48445. doi: 10.1371/journal.pone.0048445
Waqas, M., Khan, A. L., Shahzad, R., Ullah, I., Khan, A. R., and Lee, I.-J. (2015). Mutualistic fungal endophytes produce phytohormones and organic acids that promote japonica rice plant growth under prolonged heat stress. J. Zhejiang Univ. Sci. B 16, 1011–1018. doi: 10.1631/jzus.B1500081
Wassie, M., Zhang, W., Zhang, Q., Ji, K., Cao, L., and Chen, L. (2020). Exogenous salicylic acid ameliorates heat stress-induced damages and improves growth and photosynthetic efficiency in alfalfa (Medicago sativa L.). Ecotoxicol. Environ. Saf. 191:110206. doi: 10.1016/j.ecoenv.2020.110206
Wasternack, C., and Hause, B. (2013). Jasmonates: biosynthesis, perception, signal transduction and action in plant stress response, growth and development. An update. Ann. Bot. 111, 1021–1058. doi: 10.1093/aob/mct067
Watanabe, C. K., Yamori, W., Takahashi, S., Terashima, I., and Noguchi, K. (2016). Mitochondrial alternative pathway-associated photoprotection of photosystem II is related to the photorespiratory pathway. Plant Cell Physiol. 57, 1426–1431. doi: 10.1093/pcp/pcw036
Weng, M., Yang, Y. U. E., Feng, H., Pan, Z., Shen, W. H., Zhu, Y. A. N., et al. (2014). Histone chaperone ASF1 is involved in gene transcription activation in response to heat stress in Arabidopsis thaliana. Plant Cell Environ. 37, 2128–2138. doi: 10.1111/pce.12299
Wind, J., Smeekens, S., and Hanson, J. (2010). Sucrose: metabolite and signaling molecule. Phytochemistry 71, 1610–1614. doi: 10.1016/j.phytochem.2010.07.007
Wu, C., Cui, K., Wang, W., Li, Q., Fahad, S., Hu, Q., et al. (2017). Heat-induced cytokinin transportation and degradation are associated with reduced panicle cytokinin expression and fewer spikelets per panicle in rice. Front. Plant Sci. 8:371. doi: 10.3389/fpls.2017.00371
Wu, L., Zhou, H., Zhang, Q., Zhang, J., Ni, F., Liu, C., et al. (2010). DNA methylation mediated by a microRNA pathway. Mol. Cell 38, 465–475. doi: 10.1016/j.molcel.2010.03.008
Wu, T. Y., Juan, Y. T., Hsu, Y. H., Wu, S. H., Liao, H. T., Fung, R. W., et al. (2013). Interplay between heat shock proteins HSP101 and HSA32 prolongs heat acclimation memory posttranscriptionally in Arabidopsis. Plant Physiol. 161, 2075–2084. doi: 10.1104/pp.112.212589
Wu, Y.-S., and Yang, C.-Y. (2019). Ethylene-mediated signaling confers thermotolerance and regulates transcript levels of heat shock factors in rice seedlings under heat stress. Bot. Stud. 60, 1–12. doi: 10.1186/s40529-019-0272-z
Xia, X. J., Gao, C. J., Song, L. X., Zhou, Y. H., Shi, K., and Yu, J. Q. (2014). Role of H2O2 dynamics in brassinosteroid-induced stomatal closure and opening in Solanum lycopersicum. Plant Cell Environ. 37, 2036–2050. doi: 10.1111/pce.12275
Xia, X. J., Wang, Y. J., Zhou, Y. H., Tao, Y., Mao, W. H., Shi, K., et al. (2009). Reactive oxygen species are involved in brassinosteroid-induced stress tolerance in cucumber. Plant Physiol. 150, 801–814. doi: 10.1104/pp.109.138230
Xia, X.-J., Zhou, Y.-H., Shi, K., Zhou, J., Foyer, C. H., and Yu, J.-Q. (2015). Interplay between reactive oxygen species and hormones in the control of plant development and stress tolerance. J. Exp. Bot. 66, 2839–2856. doi: 10.1093/jxb/erv089
Xiao, W., Liu, F.-l, and Jiang, D. (2017). Priming: a promising strategy for crop production in response to future climate. J. Integr. Agric. 16, 2709–2716. doi: 10.1016/S2095-3119(17)61786-6
Xin, C., Wang, X., Cai, J., Zhou, Q., Liu, F., Dai, T., et al. (2016). Changes of transcriptome and proteome are associated with the enhanced post-anthesis high temperature tolerance induced by pre-anthesis heat priming in wheat. Plant Growth Regul. 79, 135–145. doi: 10.1007/s10725-015-0119-x
Xue, G.-P., Sadat, S., Drenth, J., and McIntyre, C. L. (2014). The heat shock factor family from Triticum aestivum in response to heat and other major abiotic stresses and their role in regulation of heat shock protein genes. J. Exp. Bot. 65, 539–557. doi: 10.1093/jxb/ert399
Yadav, S. K., Singla-Pareek, S. L., Ray, M., Reddy, M., and Sopory, S. (2005). Methylglyoxal levels in plants under salinity stress are dependent on glyoxalase I and glutathione. Biochem. Biophys. Res. Commun. 337, 61–67. doi: 10.1016/j.bbrc.2005.08.263
Yamaguchi, N., and Ito, T. (2021). Expression profiling of H3K27me3 demethylase genes during plant development and in response to environmental stress in Arabidopsis. Plant Signal. Behav. 16:1950445. doi: 10.1080/15592324.2021.1950445
Yan, L., Loukoianov, A., Tranquilli, G., Helguera, M., Fahima, T., and Dubcovsky, J. (2003). Positional cloning of the wheat vernalization gene VRN1. Proc. Natl. Acad. Sci. U.S.A. 100, 6263–6268. doi: 10.1073/pnas.0937399100
Yang, D., Li, Y., Shi, Y., Cui, Z., Luo, Y., Zheng, M., et al. (2016). Exogenous cytokinins increase grain yield of winter wheat cultivars by improving stay-green characteristics under heat stress. PLoS One 11:e0155437. doi: 10.1371/journal.pone.0155437
Yang, J., Fei, K., Chen, J., Wang, Z., Zhang, W., and Zhang, J. (2020). Jasmonates alleviate spikelet-opening impairment caused by high temperature stress during anthesis of photo-thermo-sensitive genic male sterile rice lines. Food Energy Security 9:e233. doi: 10.1002/fes3.233
Yang, J., Kloepper, J. W., and Ryu, C.-M. (2009). Rhizosphere bacteria help plants tolerate abiotic stress. Trends Plant Sci. 14, 1–4. doi: 10.1016/j.tplants.2008.10.004
Yang, X., Zhu, W., Zhang, H., Liu, N., and Tian, S. (2016). Heat shock factors in tomatoes: genome-wide identification, phylogenetic analysis and expression profiling under development and heat stress. PeerJ 4:e1961. doi: 10.7717/peerj.1961
Yeh, C.-H., Kaplinsky, N. J., Hu, C., and Charng, Y.-Y. (2012). Some like it hot, some like it warm: phenotyping to explore thermotolerance diversity. Plant Sci. 195, 10–23. doi: 10.1016/j.plantsci.2012.06.004
Yoon, Y. H., Kim, M., and Park, W. J. (2019). Foliar accumulation of melatonin applied to the roots of maize (Zea mays) seedlings. Biomolecules 9:26. doi: 10.3390/biom9010026
Yoshida, T., Ohama, N., Nakajima, J., Kidokoro, S., Mizoi, J., Nakashima, K., et al. (2011). Arabidopsis HsfA1 transcription factors function as the main positive regulators in heat shock-responsive gene expression. Mol. Genet. Genomics 286, 321–332. doi: 10.1007/s00438-011-0647-7
Yu, X., Wang, H., Lu, Y., de Ruiter, M., Cariaso, M., Prins, M., et al. (2012). Identification of conserved and novel microRNAs that are responsive to heat stress in Brassica rapa. J. Exp. Bot. 63, 1025–1038. doi: 10.1093/jxb/err337
Yucel, M., Burke, J. J., and Nguyen, H. T. (1992). Inhibition and recovery of photosystem II following exposure of wheat to heat shock. Environ. Exp. Bot. 32, 133–135. doi: 10.1016/0098-8472(92)90037-3
Zandalinas, S. I., Balfagón, D., Arbona, V., Gómez-Cadenas, A., Inupakutika, M. A., and Mittler, R. (2016). ABA is required for the accumulation of APX1 and MBF1c during a combination of water deficit and heat stress. J. Exp. Bot. 67, 5381–5390. doi: 10.1093/jxb/erw299
Zhang, C., Li, G., Chen, T., Feng, B., Fu, W., Yan, J., et al. (2018). Heat stress induces spikelet sterility in rice at anthesis through inhibition of pollen tube elongation interfering with auxin homeostasis in pollinated pistils. Rice 11, 1–12. doi: 10.1186/s12284-018-0206-5
Zhang, H., Lang, Z., and Zhu, J.-K. (2018). Dynamics and function of DNA methylation in plants. Nat. Rev. Mol. Cell Biol. 19, 489–506. doi: 10.1038/s41580-018-0016-z
Zhang, J. H., Huang, W. D., Liu, Y. P., and Pan, Q. H. (2005). Effects of temperature acclimation pretreatment on the ultrastructure of mesophyll cells in young grape plants (Vitis vinifera L. cv. Jingxiu) under cross-temperature stresses. J. Integr. Plant Biol. 47, 959–970. doi: 10.1111/j.1744-7909.2005.00109.x
Zhang, N., Zhang, H. J., Zhao, B., Sun, Q. Q., Cao, Y. Y., Li, R., et al. (2014). The RNA-seq approach to discriminate gene expression profiles in response to melatonin on cucumber lateral root formation. J. Pineal Res. 56, 39–50. doi: 10.1111/jpi.12095
Zhang, X., Cai, J., Wollenweber, B., Liu, F., Dai, T., Cao, W., et al. (2013a). Multiple heat and drought events affect grain yield and accumulations of high molecular weight glutenin subunits and glutenin macropolymers in wheat. J. Cereal Sci. 57, 134–140. doi: 10.1016/j.jcs.2012.10.010
Zhang, X., Shen, L., Li, F., Meng, D., and Sheng, J. (2013b). Arginase induction by heat treatment contributes to amelioration of chilling injury and activation of antioxidant enzymes in tomato fruit. Postharvest Biol. Technol. 79, 1–8. doi: 10.1016/j.postharvbio.2012.12.019
Zhang, X., Zhou, Q., Wang, X., Cai, J., Dai, T., Cao, W., et al. (2016b). Physiological and transcriptional analyses of induced post-anthesis thermo-tolerance by heat-shock pretreatment on germinating seeds of winter wheat. Environ. Exp. Bot. 131, 181–189. doi: 10.1016/j.envexpbot.2016.08.002
Zhang, X., Wang, X., Zhong, J., Zhou, Q., Wang, X., Cai, J., et al. (2016a). Drought priming induces thermo-tolerance to post-anthesis high-temperature in offspring of winter wheat. Environ. Exp. Bot. 127, 26–36. doi: 10.1016/j.envexpbot.2016.03.004
Zhang, X., Xu, Y., and Huang, B. (2019b). Lipidomic reprogramming associated with drought stress priming-enhanced heat tolerance in tall fescue (Festuca arundinacea). Plant Cell Environ. 42, 947–958. doi: 10.1111/pce.13405
Zhang, X., Wang, X., Zhuang, L., Gao, Y., and Huang, B. (2019a). Abscisic acid mediation of drought priming-enhanced heat tolerance in tall fescue (Festuca arundinacea) and Arabidopsis. Physiol. Plant. 167, 488–501. doi: 10.1111/ppl.12975
Zhang, Y., He, J., Yang, S., and Chen, Y. (2014). Exogenous 24-epibrassinolide ameliorates high temperature-induced inhibition of growth and photosynthesis in Cucumis melo. Biol. Plant. 58, 311–318. doi: 10.1007/s10535-014-0395-8
Zhang, Z., Liu, X., Wang, X., Zhou, M., Zhou, X., Ye, X., et al. (2012). An R2R3 MYB transcription factor in wheat, Ta PIMP 1, mediates host resistance to Bipolaris sorokiniana and drought stresses through regulation of defense-and stress-related genes. New Phytol. 196, 1155–1170. doi: 10.1111/j.1469-8137.2012.04353.x
Zhao, D.-Q., Li, T.-T., Hao, Z.-J., Cheng, M.-L., and Tao, J. (2019). Exogenous trehalose confers high temperature stress tolerance to herbaceous peony by enhancing antioxidant systems, activating photosynthesis, and protecting cell structure. Cell Stress Chaperones 24, 247–257.
Zhao, J., Gao, Y., Zhang, Z., Chen, T., Guo, W., and Zhang, T. (2013). A receptor-like kinase gene (GbRLK) from Gossypium barbadense enhances salinity and drought-stress tolerance in Arabidopsis. BMC Plant Biol. 13:110. doi: 10.1186/1471-2229-13-110
Zhou, J., Wang, J., Li, X., Xia, X. J., Zhou, Y. H., Shi, K., et al. (2014). H2O2 mediates the crosstalk of brassinosteroid and abscisic acid in tomato responses to heat and oxidative stresses. J. Exp. Bot. 65, 4371–4383. doi: 10.1093/jxb/eru217
Zhou, R., Yu, X., Li, X., Dos Santos, T. M., Rosenqvist, E., and Ottosen, C. O. (2020). Combined high light and heat stress induced complex response in tomato with better leaf cooling after heat priming. Plant Physiol. Biochem. 151, 1–9. doi: 10.1016/j.plaphy.2020.03.011
Zhu, J. K. (2016). Abiotic stress signaling and responses in plants. Cell 167, 313–324. doi: 10.1016/j.cell.2016.08.029
Keywords: priming, high temperature stress, phytohormones, chromatin modification, molecular mechanisms
Citation: Khan A, Khan V, Pandey K, Sopory SK and Sanan-Mishra N (2022) Thermo-Priming Mediated Cellular Networks for Abiotic Stress Management in Plants. Front. Plant Sci. 13:866409. doi: 10.3389/fpls.2022.866409
Received: 31 January 2022; Accepted: 25 February 2022;
Published: 13 May 2022.
Edited by:
Girdhar Kumar Pandey, University of Delhi, IndiaReviewed by:
Suprasanna Penna, Bhabha Atomic Research Centre, IndiaCopyright © 2022 Khan, Khan, Pandey, Sopory and Sanan-Mishra. This is an open-access article distributed under the terms of the Creative Commons Attribution License (CC BY). The use, distribution or reproduction in other forums is permitted, provided the original author(s) and the copyright owner(s) are credited and that the original publication in this journal is cited, in accordance with accepted academic practice. No use, distribution or reproduction is permitted which does not comply with these terms.
*Correspondence: Neeti Sanan-Mishra, bmVldGlAaWNnZWIucmVzLmlu
Disclaimer: All claims expressed in this article are solely those of the authors and do not necessarily represent those of their affiliated organizations, or those of the publisher, the editors and the reviewers. Any product that may be evaluated in this article or claim that may be made by its manufacturer is not guaranteed or endorsed by the publisher.
Research integrity at Frontiers
Learn more about the work of our research integrity team to safeguard the quality of each article we publish.