- 1Department of Plant Physiology, University of Granada, Granada, Spain
- 2Institute of Biotechnology, University of Granada, Granada, Spain
Enhanced crop growth and yield are the recurring concerns in agricultural field, considering the soaring world population and climate change. Abiotic stresses are one of the major limiting factors for constraining crop production, for several economically important horticultural crops, and contribute to almost 70% of yield gap. Salt stress is one of these unsought abiotic stresses that has become a consistent problem in agriculture over the past few years. Salinity further induces ionic, osmotic, and oxidative stress that result in various metabolic perturbations (including the generation of reactive oxygen, carbonyl, and nitrogen species), reduction in water potential (ψw), distorted membrane potential, membrane injury, altered rates of photosynthesis, leaf senescence, and reduced nitrogen assimilation, among others); thereby provoking a drastic reduction in crop growth and yield. One of the strategies to mitigate salt stress is the use of natural plant extracts (PEs) instead of chemical fertilizers, thus limiting water, soil, and environmental pollution. PEs mainly consist of seeds, roots, shoots, fruits, flowers, and leaves concentrates employed either individually or in mixtures. Since PEs are usually rich in bioactive compounds (e.g., carotenoids, flavonoids, phenolics, etc.), therefore they are effective in regulating redox metabolism, thereby promoting plant growth and yield. However, various factors like plant growth stage, doses applied, application method, soil, and environmental conditions may greatly influence their impact on plants. PEs have been reported to enhance salt tolerance in plants primarily through modulation of signaling signatures and pathways (e.g., Na+, ANNA4, GIPC, SOS3, and SCaBP8 Ca2+ sensors, etc.), and regulation of redox machinery [e.g., superoxide dismutase (SOD), catalase (CAT), ascorbate peroxidase (APX), non-specific peroxidase (POX), glutathione peroxidase (GPX), peroxiredoxin (Prx), ascorbic acid (AsA), glutathione (GSH), α-tocopherol, etc.]. The current study highlights the role of PEs in terms of their sources, methods of preparation, and mode of action with subsequent physiological changes induced in plants against salinity. However, an explicit mode of action of PEs remains nebulous, which might be explicated utilizing transcriptomics, proteomics, metabolomics, and bioinformatics approaches. Being ecological and economical, PEs might pave the way for ensuring the food security in this challenging era of climate change.
Introduction
The most defining concern of the present and future agriculture, and of humanity, is climate change. Human dependence on wild grains began in Halocene—a geological epoch, which further laid the basis of agriculture, as it was characterized by stable and warm temperatures. However, a rapid surge in the atmospheric carbon dioxide levels from 100 ppm to over 400 ppm with an average temperature increase by 1°C, in the past 70 years, could be a steppingstone for unstable environmental temperatures. A further escalation of temperature by 3°C by the year 2100, and even by 8°C or more is also predicted. Such drastic escalations have been identified as antagonists of human civilization (Gowdy, 2020). Similarly, agricultural activities, particularly, the use of chemical fertilizers not only incites diabetes and cancer like chronic diseases in humans, but also deteriorates the environment; thereby aggravating the climate change (Ahmad et al., 2021b). In this regard, European Union (EU) has implemented a “European Green Deal” program that proposes a 20% reduction in the use of fertilizers and a 55% reduction in greenhouse gases by 2030 as compared to 1990 levels (European-Union, 2020). Apart from this, soaring human population that is expected to reach 9.7 billion by 2050, is amplifying the pressure on agriculture to meet the growing food demands globally. Substantially lower crop yield ha–1 in comparison to increasing world population is already reported by World Food Program (WFP). Likewise, by the year 2100 a decrease in the yield of maize, wheat, and rice, by 20–45, 5–50, and 5–50%, respectively, has been predicted by Food and Agriculture Organization (FAO) if the climate change remains incessant (Arora, 2019). This ever-increasing food demand has provoked intensive agriculture systems with the unprecedented use of chemical fertilizers, pesticides, herbicides, fungicides, along with the exploitation of land and water resources; thereby further aggravating the climate change. If such trends persist, they will not only compromise the global food safety and security but may also provoke a civilization collapse.
Climate change provokes a plethora of abiotic stresses (flooding, drought, salinity, etc.) in plants (Arora, 2019; Gowdy, 2020). These abiotic stresses are one of the major limiting factors for constraining crop production (Ramadoss et al., 2013), for several economically important horticultural crops, and contribute to almost 70% of yield gap (Rouphael and Colla, 2020). Salinity is one such abiotic stress that hampers plant physiological functioning in a number of ways: resulting in lower crop production. It is attributed as a measure of salt amount in soil or water content (Arif et al., 2020), and is classified as primary salinity: caused as a result of natural processes i.e., rain, weathering, wind, etc., and secondary salinity: caused as a result of anthropogenic activity, i.e., excessive irrigation, deforestation, clearing land (Munns and Tester, 2008; Arif et al., 2020). The climate change is directly responsible for primary salinity considering the excessive rains only, for example 100 mm of rainfall year–1 would deposit 10 Kg ha–1 of sodium chloride if it contains 10 mg Kg–1 of salt (Munns and Tester, 2008), whereas indirectly to secondary salinity. Subsequently, intensive agricultural systems and poor agronomic practices, like over-fertilization, desertification, excessive irrigation, etc., have increased the levels of alkalinity and salinity of cultivated soils (Arora, 2019; Li et al., 2021). In 2013, the salinity affected cultivated soils were estimated to be over 800 million ha (Ramadoss et al., 2013) globally that culminated to around 900 million ha in 2020 (Velmurugan et al., 2020). Such a rapid conversion of fertile soils into saline ones can be regarded as a major threat to food security and agricultural sustainability.
Salinity is expressed as the electric conductivity (EC) of the soil solution, and the soil is generally denominated as saline if its EC is 4 dS m–1 or more, it approximately equals to 40 mM NaCl, producing a 0.2 MPa approximate osmotic pressure (Munns and Tester, 2008). Sodium ions (Na+) are considered as major contributors to salinity, whereas Cl–, Mg2+, SO42–, or HCO3– are also responsible for soil salinity but to a lesser extent (Munns and Tester, 2008; Zörb et al., 2019). Higher concentrations of these salts trigger ionic and osmotic stress resulting in the generation of reactive oxygen species (ROS), reduced cell/leaf expansion, leaf abscission, reduction in ψw, distorted membrane potential, membrane injury, altered rates of photosynthesis, stomatal closure, protein destabilization, altered carbon portioning, cavitation, reduced nitrogen assimilation, ion cytotoxicity, and cell death among others; thereby provoking a drastic reduction in crop growth and yield (Ashraf and Harris, 2004; Munns and Tester, 2008; Acosta-Motos et al., 2017; Farooq et al., 2017; Arif et al., 2020). Therefore, it is indispensable to devise novel strategies for combating salinity.
Use of natural plant extracts (PEs) (or “botanicals”) could be one of the salinity mitigation ecofriendly strategies. PEs are potential alternatives to chemical fertilizers. PEs fall under the umbrella of plant biostimulants, and are used to enhance plant growth (Calvo et al., 2014; Drobek et al., 2019). PEs are the concentrates of plants and could be prepared using any part of the plant, i.e., seeds, roots, stems, leaves, bark, flowers, etc. (Semida and Rady, 2014; Lorenzo et al., 2019; Nessim and Kasim, 2019; Rady et al., 2019b; Zulfiqar et al., 2020b). The application of PEs could be either in liquid form as foliar spray and/or root treatment, or as soil preparations like granules, concentrates, solutions added to soil, or powders (Drobek et al., 2019). PEs can be associated with the amelioration of salinity as they are the sources of prominent phytochemicals like vitamins, carotenoids, amino acids, phytohormones, mineral nutrients, phenolics, and antioxidants (Latef et al., 2017). There are several reports where these compounds, used either individually or in mixtures, were found to be effective against salinity (Calvo et al., 2014; Iqbal et al., 2014; Bulgari et al., 2015; Drobek et al., 2019; Shukla et al., 2019; Zulfiqar et al., 2020b). However, the effect of PEs is often concentration dependent. Similarly, plant part and age of plant used as an extract also influences the PEs overall proficiency.
Currently, there are no extensive studies reported on the particular use of PEs and their subsequent mechanism of action against salinity. Previously reported studies mostly discuss the use of biostimulants that is rather a broader term and even includes microbial and non-microbial formulations, protein hydrolyzates, PEs, amino acid, seaweed extracts, etc. (Du Jardin, 2015). Furthermore, previous studies have discussed the use of biostimulants on abiotic stresses in general (Calvo et al., 2014; Drobek et al., 2019; Shukla et al., 2019; Rouphael and Colla, 2020; Zulfiqar et al., 2020b), whereas no specific study on the use of PEs against salinity is reported. Therefore, the aim of the study was to elaborate the potential of ecofriendly and natural PEs as salinity alleviators, and to underline their possible mode of action with the subsequent physiological changes thus induced. Since an explicit mode of action of PEs remains nebulous, hence this subject has been estimated considering the up or down regulation of signaling signatures, altered photosynthetic rates, and redox metabolism in general.
This review is divided into three sections. Impact of plant based biostimulants under normal conditions is discussed first followed by salinity induced physiological, biochemical, and genetic changes in plants. Subsequently, the use of PEs, including their sources and methods of preparation, as salinity mitigation strategy is discussed. Finally, all this is concluded by identifying the limitations and future perspectives of the use of PEs against salinity.
Plant Based Biostimulants
Use of plant based biostimulants is rapidly gaining popularity in agriculture. Plant based biostimulants, apart from inducing stress tolerance, are also effective in regulating a number of plants physiological processes; thereby improving plant growth and yield (Brown and Saa, 2015). They may comprise of protein hydrolyzates and amino acids, hormone-, amino acids-, or nutrients containing products, vegetable oils, etc. of plant origin (Ikrina and Kolbin, 2004; Kauffman et al., 2007; La Torre et al., 2016; Yakhin et al., 2017). Their mechanism of action might involve phosphorus (P) release from soils, activation of nitrogen (N) metabolism, stimulation of root growth, nutritional and hormonal regulation, or generic stimulation of soil microbial activity. Previous reports have documented that the application of biostimulants has enhanced various physiological processes including plant nutrient uptake and utilization, photosynthesis, water use efficiency, synthesis and concentration of growth hormones (auxins, gibberellins, and cytokinins), germination, and senescence reduction (Parađiković et al., 2011; Bulgari et al., 2015; Nasir et al., 2016; Merwad, 2017; Ur Rehman et al., 2017; Milić et al., 2018; Younis et al., 2018), which in return increase plant production, yield, post-harvest quality, and shelf life of agricultural products.
Among various plant based biostimulants, PEs are economical and easy to prepare. Several studies have reported their beneficial effects on plants growth and yield. For instances, an increase in the growth and hormonal profile was observed in eggplant and snap bean when aqueous garlic extracts were applied (Elzaawely et al., 2018; Ali et al., 2019). Similar results were reported in case of moringa leaf extracts being applied on sword lily, where they improved plant growth and vase life by regulating various physiological processes (Zulfiqar et al., 2020c). Likewise, borage extracts were reported to supplement the primary metabolism, by enhancing leaf pigments and photosynthetic activity, and reduced chlorophyl a fluorescence, by incrementing the number of active reaction centers per cross section, in lettuce plants (Bulgari et al., 2017). Likewise, vine-shoot and oak extracts were found to significantly improve wine yield and quality by triggering amino acids and volatile compounds production (Sánchez-Gómez et al., 2016). In addition, PEs are also responsible for increasing the shelf life and postharvest quality of agricultural products. For example, moringa leaf extracts were found to significantly improve avocado and citrus fruit shelf life and quality by lowering the respiration rate and water transfer from the fruits (Adetunji et al., 2012; Tesfay and Magwaza, 2017). All of these studies indicate the potential of PEs in positively regulating several physiological processes. Therefore, extrapolating the incredible potential of PEs to combat abiotic stresses, especially salinity, would be a promising approach for a sustainable agriculture.
Salinity Perception and Signaling in Plants
Stress perception and signaling hold an imperative role for subsequent plant behavior. Apoplastic and symplastic pathways are the recognized routes of ions entry into plant that result in salinity (Lamers et al., 2020) (Figure 1). Stress signals are perceived by plant cell surface-based receptors that stimulate the production of secondary messengers like Ca2+, ROS, and inositol phosphates, among others (Rao et al., 2016). The most common surface based receptors involved in the cation sensing (Na+ and Ca2+) are membrane bound proteins or ion channels including glycosyl inositol phosphorylceramide (GIPC) sphingolipids synthesized by MONOCATION-INDUCED [Ca2+]cyt INCREASES1 (MOCA1), Arabidopsis ANNEXIN4 (ANN4), and Arabidopsis HIGH-AFFINITY K+ TRANSPORTER1 (HKT1) (Chen et al., 2021). Similarly, ethylene receptors like Nicotiana tabacum histidine kinase 1 (NTHK1) are also reported to modulate stress signaling (Cao et al., 2008). While Ca2+ signaling is an important mechanism for salt-sensing and is modulated through SALT OVERLY SENSITIVE (SOS) pathway. SOS pathway consists of SOS1 Na+/H+ antiporter, SOS2 and SOS2-LIKE PROTEIN KINASE5 (PKB5) protein kinases, and SOS3 and SCaBP8 Ca2+ sensors (Lamers et al., 2020). The intercellular Ca2+ perturbations trigger Ca2+ sensors, e.g., SOS3 and SCaBP8, bringing about conformational changes in them in a calcium-dependent manner. These sensors further interact with their respective partners, e.g., activate SOS2, whereby a phosphorylation cascade is initiated (Quan et al., 2007; Rao et al., 2016). Subsequently, SOS1 is activated carrying out the efflux of Na+ ions (Figure 2). It is reported that within 20 s of stress (sodium) application a change in SOS1 exchanger activity can be detected (Lamers et al., 2020). Ultimately these alterations result in the genomic regulations (activation of transcription factors and stress responsive genes) by biosynthesizing metabolites and other compounds needed to combat salinity. There are two categories of stress- responsive genes, i.e., (a) early induced genes: immediate activation on receiving the stress signal with short-term persistence (including transcription factors, interfering RNAs, etc.), and (b) late induced genes: late activation with longer persistence periods (including membrane stabilizing, osmolytes, antioxidants, etc.) (Rao et al., 2016).
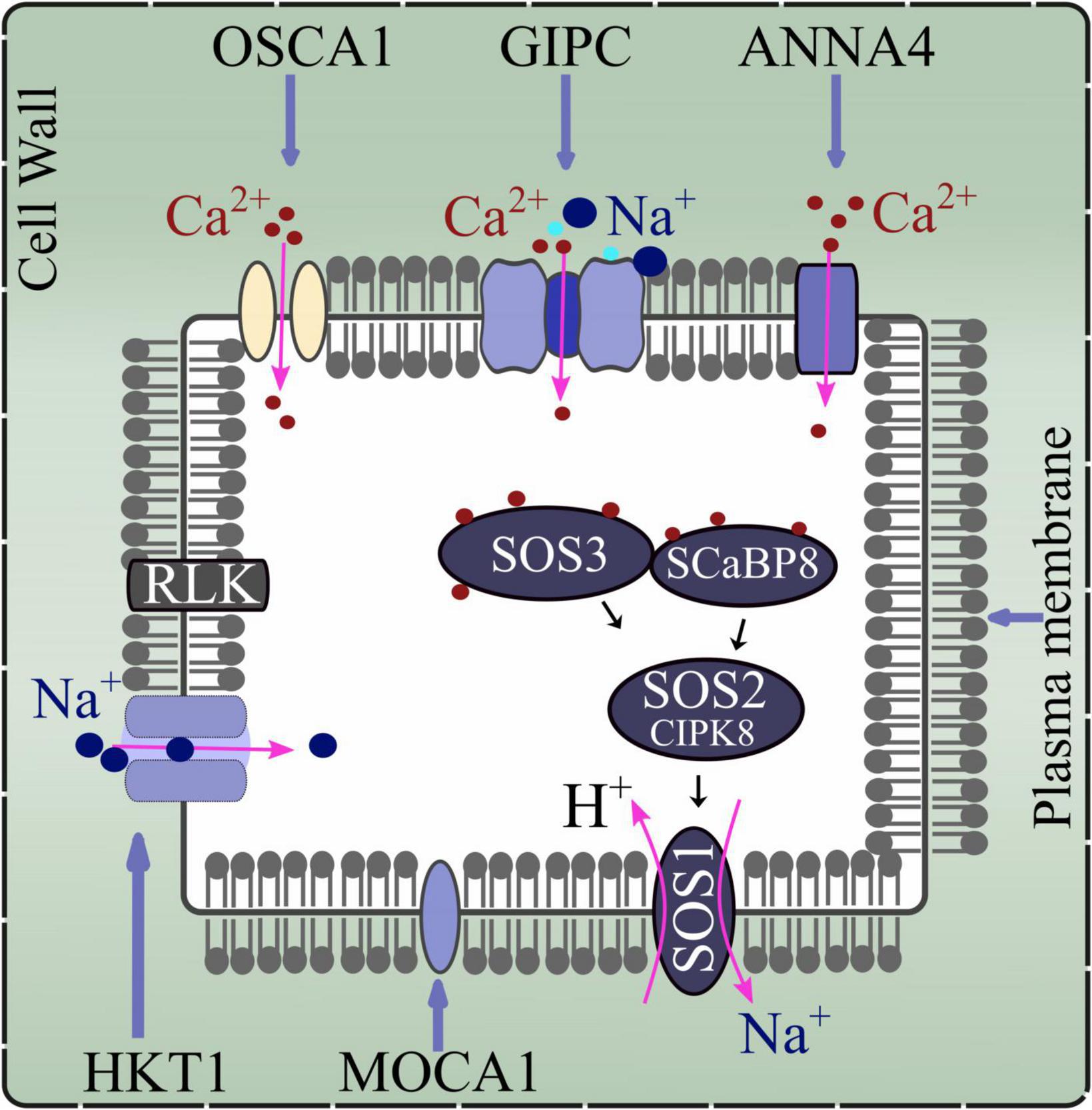
Figure 2. Representation of membrane-based proteins, and ion channels that regulate ions movement in plants (under saline environment).
Since plants undergo an osmotic stress under saline environment, therefore it is proposed that receptors involved in osmotic, or drought sensing can also be implicated for salinity signal transduction. It is reported that a plasma membrane located channel encoded by OSCA1 regulates the Ca2+ influx under plasma membrane tension or extracellular osmotic pressure. Similarly, the receptor-like kinases (RLKs) present on plasma membrane have also been reported to play certain role (Lamers et al., 2020) (Figure 2).
Plant Responses to Salinity
Plants generally vary in their response to salinity. Their response could be cellular- and tissue level, morphological, or physiological. Such response depends on various factors including duration and severity of stress, plant age and its developmental stage, and plant species (Peleg et al., 2011). Therefore, some plants are found to be more tolerant (less sensitive) to salinity than others. For example, barley (Hordeum vulgare) is more tolerant to salinity than rice (Oryza sativa) (Munns and Tester, 2008). Plants are generally categorized as halophytes or euhalophytes based on their genetic adaptability to salinity, whereas they are termed as glycophytes if they are less-tolerant or not adapted to salt stress (Acosta-Motos et al., 2017). The general effects of salt stress in glycophytes occur in the following two forms (Munns and Tester, 2008; Acosta-Motos et al., 2017);
(i) Immediate response: onset of osmotic stress on surpassing the threshold limit of salts in the root zone resulting in the reduction of shoot biomass.
(ii) Slower response: onset of ionic stress on accumulation of salt ions (Na+ and Cl–) in older leaves resulting in the impairment of photosynthetic machinery and leaf senescence.
The rapid osmotic phase response of plants begins at the root-soil periphery. The toxic concentrations of salt ions build up osmotic pressure that negatively regulates the rate of leaf expansion, emergence of new leaves, lateral buds- and shoots formation. The second phase is characterized by the higher accumulation rate (i.e., toxic concentrations) of Na+ ions in the older leaves that cannot dilute these salts due to lack of expansion, ultimately resulting in their death. Although, some plant species are sensitive to higher Cl– concentrations. This results in the reduced photosynthetic rates of plants that causes a reduction in their growth rate. Since ionic stress is time taking due to the accumulation of ions, therefore plant growth is affected much later with lesser impact as compared to osmotic stress.
Mechanisms of Salinity Tolerance in Plants
Salinity tolerance in plants is achieved through a series of complex signaling and biosynthetic responses. However, the commonly known mechanisms of salinity tolerance include morphological (roots and aerial parts), metabolic (osmotic regulation, ionic and molecular homeostasis, and hormonal homeostasis), and genetic responses. Comprehensive reports of salinity tolerance mechanisms have been reported previously (Munns and Tester, 2008; Gupta and Huang, 2014; Tang et al., 2015; Acosta-Motos et al., 2017; Farooq et al., 2017; Arif et al., 2020). Here, a description of these mechanisms is presented to elaborate the use of PEs, as they reinforce these salinity tolerance mechanisms.
Morphological Adjustments
Plants can adapt their morphological features to sustain the normal functioning and cellular homeostasis in case of any unfavorable stimulus. This is characterized as phenotypic plasticity. This also occurs in case of salinity. Although, it varies among salt tolerant and salt sensitive species. Generally, the productivity or yield of an agricultural crop can be assessed by analyzing its above-and below-ground parts. Therefore, few of the growth indices, as per Beadle (1993), taken into consideration for salt stress studies in plants are listed below:
Where W represents the total dry weight, WL is the total dry weight of leaves, A is the total leaf area, t is the time, 1 and 2 represents the start and end of a period, respectively. Considering these growth indices as standard, various studies have demonstrated relative effects of salinity on plant morphology as a decrease in RGR in five ornamental plants (Cassaniti et al., 2012), a decrease in NAR in Hibiscus cannabinus and Argyranthemum coronopifolium (Curtis and Lauchli, 1986; Morales et al., 1998), a decrease in LAR in A. coronopifolium (Morales et al., 1998), and an increase in LWR in Asteriscus maritimus (Rodrıguez et al., 2005). Similarly, other morphological changes observed in plants (salt sensitive) under salinity include decrease in leaf thickness, succulence values, surface to volume ratio of cells and tissue density, spongy parenchyma, and number of mitochondrial cristae, whereas an increase in the lower area/volume ratio of mesophyll cells, mesophyll thickness, leaf water balance, leaf size, palisade cell size, succulence values, intercellular space, and palisade parenchyma has been reported (Longstreth and Nobel, 1979; Romero-Aranda et al., 1998; Franco-Navarro et al., 2016; Acosta-Motos et al., 2017). Most of these changes suggest that plants under salt stress intend to increase the CO2 diffusion so that the energy production should not be disrupted along with an increased water use efficiency through higher photosynthetic performance. Similarly, leaf senescence and leaf color change are also salinity mitigation mechanisms, in which chlorophyll is gradually degraded resulting in the accumulation of carotenoids and anthocyanins that provide protection against oxidative stress (Hörtensteiner, 2006; Garriga et al., 2014).
Plant roots experience some morphological changes in their size, diameter, and number so as to maximize the nutrients and water uptake. Increased root to shoot ratio helps plants in compartmentalization and ions retention. Likewise, root proliferation helps plant to curb toxic ions accumulations. Also, root density and electrical conductivity increases under saline environment (Acosta-Motos et al., 2017; Arif et al., 2020). The other anatomical and ultra-structural change under salinity is the development of casparian strip and suberin lamella serving as apoplastic barrier. Similarly, plants’ chloroplast number and stable size structure increases under salinity (Arif et al., 2020) to maintain the effective photosynthetic rates.
Metabolic Adjustments
Salinity creates an ionic imbalance in plants that may result in denaturation or damage to subcellular organelles like chloroplasts and mitochondria. Therefore, plants compartmentalize the excessive ions into their vacuoles, and usually put into play their inclusion and exclusion mechanisms. This is generally regulated by (a) sequestration of excessive salt into vacuole with the help of various pumps (e.g., Na+/H+ antiporters); (b) ionic equilibrium: modulation of Na+, Cl–, K+, and Ca2+ in the plant cell via SOS and non-selective cationic channels (NSCCs) (Gupta and Huang, 2014; Arif et al., 2020). Osmotic potential (ψs) of plant cell is critical for its growth, development, and yield. That is why its proper regulation is important. Plants generally produce osmoprotectants to keep in check their ψs. These compounds are also described as compatible solutes. Unlike ions they neither paralyze the metabolic functions of enzymes, nor they destabilize the cellular membranes. In comparison to inorganic compounds, higher concentrations of these compounds are non-toxic to cellular metabolism (Nahar et al., 2016). Additionally, osmoprotectants play a diverse role in plant physiology under harsh conditions. Some of their prominent roles regarding metabolic adjustments in plants under stress include stabilization of proteins structures, regulation of protein folding, detoxification of ROS, stabilization of thylakoid membranes, protection of antioxidant enzymes, regulation of redox balance, and activation of stress responsive genes that result in redox homeostasis, stress signaling, upregulation of photosynthesis, and scavenging of toxic radicals (Zulfiqar et al., 2020a). These compatible solutes include betaines [proline betaine, hydroxyproline betaine, glycine betaine (GB), and pipecolate betaine], proline, sugars (fructose, glucose, sucrose, and fructans), and sugar alcohols (mannitol, sorbitol, and inositol). Of these compatible solutes, GB, a quaternary ammonium compound, usually ameliorates the toxic effects by accumulating in the cell and by distinguishing Na+ to K+ ratio. It is also reported to safeguard PSII under salt stress. Also, proline acts as an osmoprotectant as well as a molecular chaperone sustaining the structural integrity of macromolecules. Similarly, higher amounts of reduced sugars, i.e., fructose, glucose, sucrose, and fructans, stabilize the membrane integrity and prevent them from denaturation. Likewise, mannitol, sorbitol, and inositol facilitate in maintaining turgor, Na+ sequestration into the vacuole, and quenching ROS (Tang et al., 2015; Acosta-Motos et al., 2017; Arif et al., 2020).
One of the most common abiotic stress indicators in plants is the induction of oxidative stress. Salt stress also results in oxidative stress that comprises of ROS, reactive carbonyl species (RCS), and reactive nitrogen species (RNS) (Mano, 2012; Corpas, 2016; Fancy et al., 2017). However, these indicators of stress are also found in plant cells under normal conditions and a proper regulation of their intrinsic cellular concentration exists because they are also involved in plant growth and development, and signaling at subcellular and intercellular level (Corpas, 2016; Fancy et al., 2017; Zulfiqar and Ashraf, 2021a). Under any unfavorable condition, homeostatic balance of these reactive species disrupts resulting in altered cellular redox potential that results in the denaturation of various vital compounds including nucleic acids, proteins, lipids, etc. and disruption of cellular structures (Mano, 2012; Hasanuzzaman et al., 2020; Zulfiqar et al., 2020a). Nitric oxide (NO) and derived molecules altogether constitute RNS, whereas methylglyoxal (MG) and other α,β-unsaturated carbonyl compounds constitute RCS that are more stable than ROS (Mano et al., 2019; Nareshkumar et al., 2020). Mitigation of these radicals protect plant organelles in a number of ways. For example, the photoproduction and removal of ROS not only protects the chloroplast from the damaging effects of ROS but also acts as an escape valve for excess photons (Hasanuzzaman et al., 2020). Similarly, MG detoxification may result in improved cell proliferation, miotic index, seed germination, photosynthesis, stress-related gene expression, etc. (Mostofa et al., 2018). Preferred sites of ROS generation have been identified as chloroplast, mitochondria, and peroxisomes, whereas for NO as peroxisomes—although this remains a subject of further research (Hernandez et al., 1995; Hernández et al., 2001; Corpas, 2016). Similarly, RCS are generated as a by-product in various metabolic pathways, i.e., sugar metabolism, oxidative degradation of glucose and glycated proteins, glycolysis, lipid peroxidation, photosynthesis, etc., (Kaur et al., 2016; Mostofa et al., 2018), and therefore can be associated to be present in chloroplast, mitochondria, peroxisomes, cell membranes, nucleus, endoplasmic reticulum, and cytosol.
Ascorbate–glutathione (AsA–GSH) is a key metabolic pathway that keeps the oxidative stress of plants in check through enzymatic [catalase (CAT) EC 1.11.1.6, ascorbate peroxidase (APX) EC 1.11.1.11, dehydroascorbate reductase (DHAR) EC 1.8.5.1, monodehydroascorbate reductase (MDHAR) EC 1.6.5.4, glutathione-S-transferase (GST) EC 2.5.1.18, glutathione reductase (GR) EC 1.6.4.2, guaiacol peroxidase (GPX) EC 1.11.1.7, glutathione peroxidase (GPX) EC 1.11.1.9) and non-enzymatic (ascorbic acid (AsA), glutathione (GSH)] antioxidant players (Acosta-Motos et al., 2017; Hasanuzzaman et al., 2020). Similarly, RCS scavenging system also comprises of enzymatic and non-enzymatic compounds (Mano et al., 2019). A rise in MG levels is usually observed under salt stress that triggers the synthesis of glyoxalase: enzyme responsible for the detoxification of MG (Kaur et al., 2016). Under salt stress, mitochondria and chloroplast are specifically found to be affected. Consequently, electron transport chain is disrupted due to stomatal closure. Accordingly, the final electron acceptor in PSI (NADP+) of the electron chain suffers a halt in its regeneration that triggers Mehler Reaction (transfer of electron from ferredoxin to oxygen to form O2–) (Gururani et al., 2015; Acosta-Motos et al., 2017). This O2– is further converted to hydrogen peroxide (H2O2) and superoxide (O2–) by superoxide dismutase (SOD). Similarly, O2– generation in peroxisomes is modulated by APX and CAT activities. Protein denaturation and other structural damages have been reported previously in salt stressed cells, affecting particularly chloroplast and mitochondria due to the accumulation of H2O2 and O2– radicals. Therefore, in order to protect the photosynthetic machinery from ROS, plants regulate ‘xanthophyll cycle’ in which violaxanthin de-epoxidase converts carotenoid violaxanthin to zeaxanthin. This cycle helps in excessive energy dissipation in the form of heat, constituting the main mechanism of excessive energy dissipation, from PSII through non-photochemical quenching (NPQ). Zeaxanthin serves as an antioxidant for photoinhibition and photo-oxidation by scavenging ROS in thylakoid membranes. Similarly, salinity also results in the decrease in chlorophyl content that causes an increase in the anthocyanin and carotenoid accumulation, which help in toxic radicals scavenging and chloroplasts protection from photoinhibition and photooxidation. In the same way, another adapted mechanism for salinity tolerance is photorespiration that constantly recycles carbon dioxide from the decarboxylation of glycine in the mitochondria, so that the Calvin cycle is kept operational. Consequently, it diminishes ROS generation in electron transport chain. In addition, plants also use the water-water cycle to scavenge the ROS and dissipate excessive energy. In this cycle, water generated electrons in PSII are used to; (a) photo-reduce the dioxygen to superoxide in PSI, and (b) recycle ascorbate; thereby sustaining a linear electron flow for ATP generation. Furthermore, NO is involved in the glutathione metabolism by regulating GSH-dependent enzymes, i.e., GST, GR, and GSH. Also, it is reported that NO is a multifunctional molecule that regulates salt stress through genetic and molecular level regulations. Besides the aforementioned mechanisms, plants can also mitigate the oxidative stress through selective up-regulation of antioxidant enzymes, as found in Lycopersicon pennellii (Hernandez et al., 1995; Asada, 1999; Hernández et al., 2001; Mittova et al., 2003; Hörtensteiner, 2006; Garriga et al., 2014; Corpas, 2016; Acosta-Motos et al., 2017; Mano et al., 2019).
Phytohormonal Adjustments
Phytohormones modulate salinity by participating in signaling pathways and gene regulation. Abscisic acid (ABA) is known to regulate genes responsible for stomatal closure and osmoprotectant biosynthesis. It also helps in plant acclimation and inhibition of lateral root growth (Arif et al., 2020). Indole-3-acetic acid (IAA) promotes ion homeostasis by upregulating the expression of various genes including auxin/indoleacetic acid (Aux/IAA), small auxin-up RNA (SAUR) and GH3 (Sun et al., 2018), apart from regulating plant growth and development. Likewise, brassinosteroids (BRs) help plant to cope up with salinity by playing their role in the pollen tube growth, reproduction, proton pump activation, vascular differentiation, photosynthesis, and by improving antioxidant and osmoprotectant contents (Nolan et al., 2020). Also, cytokinins (CKs) are involved in salinity mitigation by increasing shoot to root ratio and antioxidants gene expression (Arif et al., 2020). Furthermore, ethylene is involved in salinity signaling perception and upregulating the expression of osmoprotectant genes, e.g., GB (Fahad et al., 2015). As well, gibberellins (GAs) are increased under salinity and modulate it by improving redox metabolism, sugar signaling, and osmolyte production (Fahad et al., 2015; Rao et al., 2016). Additionally, jasmonic acid (JA) reinforces the expression of arginine decarboxylase, invertase, and Rubisco genes to mitigate salinity. It is also involved in the metabolism of fatty acid along with methyl jasmonate (MeJA). The upregulation of arginine decarboxylase genes results in the modulation of polyamines biosynthesis that serve as osmolytes. Furthermore, it facilitates protein synthesis and CO2 fixation under saline conditions (Arif et al., 2020). Additionally, higher amounts of polyamines (PAs), nitrogen-containing aliphatic compounds, are accumulated in salt stressed cells to modulate signaling, cell proliferation, genetic expression, cell turgidity, and senescence (Ismail and Horie, 2017). In addition, salicylic acid (SA) modulates plant salinity to a great deal by participating in signaling pathways and regulating various genes expression. It also modulates ion homeostasis, i.e., limits Na+ influx in roots, Na+ regulates sequestration and exclusion, facilitates root H+-ATPase activity, and augments K+ concentration in aerial parts. As well, it upregulates genes of various ion channels to avoid K+ leakage (Arif et al., 2020).
Genomic Adjustments
Plants affected by salinity undertake various genomic adjustments in which various genes are up- and down-regulated. Currently, several advanced genomic techniques have made it possible to assess the molecular changes going-on in a plant under salt stress. Although, this is a set of complex mechanisms that range from transcription to post-translational modifications. Such genetic variation of expression results in the higher production of RNAs and proteins necessary to mitigate salinity. For instance, an upregulation of genes responsible for osmoprotectants biosynthesis is certainly a desired behavior for combating salinity (Amirbakhtiar et al., 2019). Nevertheless, an upregulation of genes is not always the case, rather the genomic behavior of plants may result in down-regulation, moderate expression, or even no expression. Similarly, gene expression can also be altered by the involvement of transcription factors or interfering RNAs. It has been discovered that the endogenous small interfering RNAs (siRNAs) and microRNAs (miRNAs) e.g., miR530a, miR1445, miR1446a-e, miR1447, miR396, miR394, miR393, miR319, miR171 miR169, miR168, etc., also play important roles in stress mitigation (Mangrauthia et al., 2013; Rao et al., 2016).
Following four functional categories of stress-responsive genes of plants have been established by Rao et al. (2016):
(a) Molecular chaperones (e.g., HSP genes).
(b) Ion transport or homeostasis (e.g., SOS genes, AtNHX1, and H+-ATPase).
(c) Dehydration-related transcription factors (e.g., DREB).
(d) Senescence-associated genes (e.g., SAG).
Some representative and differentially expressed genes (DEGs) are presented in Table 1, where genes or their families are grouped based on their function and involvement in signaling transduction, stress (ionic, osmotic, and oxidative), and metabolites biosynthesis. Generally, all these sets of genes are upregulated under saline environment with few exceptions (Arif et al., 2020). In a recent study, around 5128 DEGs for Triticum aestivum, treated with 150 mM NaCl, have been reported (Amirbakhtiar et al., 2019). This huge number of transcripts indicate the level of complexity involved in the regulation of salt stress, although it varies from species to species. Moreover, this study also underlined the upregulation of a set of genes involved particularly in signaling pathways, ion transporters, and oxidative stress.
Furthermore, it has been reported that genes coding for calcium sensing molecules (SOS3/CBL4) are up regulated in saline conditions. Their activation leads to the formation of a protein complex resulting in the transcription of Na+ antiporter gene (SOS1). Where Ca2+ causes Na+/H+ EXCHANGER 1 (NHX1) antiporter assisted Na+ sequestration into the vacuole, SOS1 gene induces Na+ efflux from cytosol (Arif et al., 2020). Likewise, genes encoding for proteins of photosynthetic machinery, ROS scavenging activity, SOD, cytochrome production, and isoflavone reductase production have also been found to be upregulated (Fahad et al., 2015; Rao et al., 2016; Amirbakhtiar et al., 2019; Khan et al., 2019). Briefly, all of the enzymes, proteins, osmoprotectants, co-factors, transporters, metabolites, etc. involved in ionic, oxidative, and osmotic stress (as described above: in morphological adjustments and metabolic adjustments) get their respective genes upregulated under the salt stress. For example, calcium pathways and SOS signaling genes have been reported to play their role in cell homeostasis and salt acclimation (Fahad et al., 2015). Although, several transcription factors play their intermediate role in these processes and post-translational modifications, but a comprehensive elucidation of their role remains ambiguous.
Salinity Mitigation Through Conventional Methods
Salts accumulation around the plants root cause salinity in plants. In order to remove these toxic concentrations of salts so as to gain maximum plant yield, commonly implied strategies include flushing, scraping, and leaching. Of these leaching is most widely used strategy, in which irrigation is sustained over the evapotranspiration rates. The excessive amount of water retains the concentrations of salts below their critical limits. For instance, in a study 20–30% extra irrigated water was found to leach 70% of salts from the maize roots (Plaut et al., 2013; Rao et al., 2016). Various irrigation models (e.g., SALTMED, CWPF, Enviro-Gro, WATSUIT, TETRANS, UNSATCHEM, MOPECO-Salt, etc.) based on the leaching principle have also been suggested as salinity mitigation approach and to improve crop yield (Plaut et al., 2013). But these models either considered the salt concentration as constant for any given time or were limited in their performance under various environmental factors; thereby constraining crop yield.
Soil mulching is another conventional approach for salinity mitigation. For this, soil surface is covered with mulch or plastic sheet to enhance water availability by limiting water evaporation. Previous studies conducted on cotton plants have demonstrated positive impact of mulching against salinity in terms of reduced activity of MDA, decreased accumulation of Na+ in leaves and roots, inhibition of lipid oxidation, improved photosynthesis, and higher biomass (Dong et al., 2008, 2009). However, this approach has short term effects and is only efficient to affect the upper soil layer. Irrigation water treatment through aeration and/or magnetic processing is another salinity mitigation technique (Plaut et al., 2013). Nevertheless, this is not widely adopted technique due to associated costs and intricacy of process. Another conventional approach is the cultivation of halophytes in saline soils for eliminating or reducing the accumulated salts to the threshold levels for glycophytes. Some halophytes are reported to have salt glands for this purpose that possess the ability to exclude salts, whereas others are reported to have salt hairs that serve to accumulate salts. Additionally, better agronomic and farm management practices can also improve salinity. For instance, with the drip irrigation a controlled amount of water can be applied to the soil, whereby limiting the soil salination. Also, surface and sprinkler irrigations might prove effective to leach down the excessive salts from root zone. Similarly, crop rotation with perennial crops can be practiced particularly in rain-fed areas. Deep roots of perennial crops might help restore the salt-water equilibrium in the soil (Plaut et al., 2013; Rao et al., 2016).
Selection, conventional breeding, and/or genetic engineering for salinity tolerant crops are also reported as salinity mitigation techniques (Athar and Ashraf, 2009; Plaut et al., 2013). In this regard, halophytes or salinity tolerant genotypes could be bred with desired salinity susceptible crop plants to get salt tolerant progeny. Similarly, salinity susceptible plants can be genetically transformed with salt tolerant genes or can be engineered for having salt glands/hairs. Equally, elicitation of plant bioregulators, osmolytes, antioxidants, or other metabolites biosynthesis has also been regarded as a valuable approach (Ashraf and Akram, 2009; Zulfiqar and Ashraf, 2021a). However, despite of the remarkable potential, these strategies are rather limited due to huge amounts of time required and the associated costs. Equally, salinity tolerance is a complex process that is regulated by a large number of genes that obscures the crop breeding and genetic transformation processes. Therefore, attaining a salt resistant transgenic line with its subsequent adoption in field conditions still remains a challenge.
In the same way, use of microbial inoculants, chemical and organic soil amendments, and electro remediation are other promising salinity mitigation strategies that are gaining increased scientific attention lately (Sahab et al., 2020). As chemical soil amendments pose threats to soil microbiota and indirectly to human life, therefore this cannot be regarded as a sustainable approach. An alternative method of salinity mitigation is the exogenous application of nutrients and metabolites that relieves plants from Na+ and Cl– injury (Munns, 2002; Plaut et al., 2013). Use of natural PEs, in this regard, can be associated with this strategy of salinity mitigation, although PEs do not only have phytonutrients but also other stress relieving metabolites, e.g., GB, proline, melatonin, etc.
Use of Plant Extracts Against Salinity
Use of PEs to mitigate salinity can be regarded as an environment friendly and sustainable way of fighting abiotic stress, as it contains no synthetic chemicals. Depending upon the parts of plants used to prepare PE, it may contain various amounts of bioactive compounds (flavonols, phenolics, betaines, amino-polysaccharides, sterols, glucosinolates, terpenoids, furostanol glycosides, etc.), phytohormones, mineral elements, photosynthetic pigments, amino acids, nucleotides or nucleosides, lipids, etc. Due to the associated detoxifying and ROS quenching capabilities of these compounds, PEs are frequently used in pharmacological industry for providing protection against neurodegeneration, diabetes, muscular dystrophy, and cancer like chronic diseases (Pehlivan, 2018). Since these natural compounds are also effective in preventing macromolecules like lipids, proteins, and DNA from damage in animal cells (Pehlivan, 2018), therefore it can be deduced that they might also be effective in plants against salinity as it disrupts redox balance in plants. This has been demonstrated in previous studies that PEs contribute to a better growth, development, yield, disease-, and stress-resistance in plants given the presence of aforementioned compounds (Howladar, 2014; Drobek et al., 2019; Desoky et al., 2020; Zulfiqar et al., 2020b). Nevertheless, further scientific evidences are yet to be excavated to ensure that such a wide variety of molecules in PEs is functional or not. Similarly, viability and quality of PEs is also an aspiring research area.
Sources of PEs, methods of application, and their implications against salinity are discussed below.
Sources, Preparation, and Application Methods
Plant extracts or botanicals are prepared from natural resources like higher plants. They can be prepared either from a whole plant or from any specific part of the plant, i.e., fruits, flowers, bark, roots, leaves, stems, seeds, pollens, grains, etc. (Semida and Rady, 2014; Lorenzo et al., 2019; Nessim and Kasim, 2019; Rady et al., 2019a; Zulfiqar et al., 2020b; Suryaman et al., 2021). Whereas plant derived products like protein hydrolyzates, polyamines, polyols, amides, etc. fall under the category of plant derived biostimulants (PDBs), as PEs are multicomponent mixtures. However, the extraction of a particular compound or a mixture of compounds can be reinforced by selecting an appropriate method of extract preparation.
Conventionally PEs are prepared by maceration. The extraction is done in some solvent either hydrous or organic. For aqueous extraction, desired plant part is macerated or processed mechanically in deionized H2O, followed by its purification and centrifugation. The resultant analyte is diluted as per requirement and applied to plant (Rady and Mohamed, 2015; Abd El-Mageed et al., 2017; Yakhin et al., 2017; Ali et al., 2018; Zulfiqar et al., 2020b). In organic solvent extraction, the desired plant part is homogenized in an organic solvent, e.g., ethanol, followed by fractionated extraction with hexane, ethyl acetate, and/or butanol like solvents. Further, the resultant extractants are purified by removing organic solvents through evaporation (Salama et al., 2013; Lim et al., 2014; Brockman and Brennan, 2017; Yakhin et al., 2017; Pehlivan, 2018). Aqueous extraction is considered relatively easier, faster, and economical as compared to the organic solvent-based extraction. Furthermore, several other methods for homogenates preparation can be implied such as bead impact methods, rotor–stator homogenizer, high pressure batch/flow, low-pressure droplet method, ultrasonic processors, etc. (Goldberg, 2008). Besides, these basic methods can be further modified based on the desired extractant, i.e., lipophilic, or hydrophilic. However, an appropriate method of PE preparation is important as it affects the stability characteristics of the formulation (Lötze and Hoffman, 2016). In addition, the extractions carried out using organic solvent, like ethanol, may vary in triggering the physiological response as compared to the extracts prepared through aqueous extraction. The possible reason of such difference could be the variation in physiochemical properties, i.e., pH, temperature, electric charge, surface tension, solubility, etc., of the aqueous and organic extracts. For instance, a subsequent increase in the extract viscosity was observed when pH and temperature were increased (Briceño-Domínguez et al., 2014). Similarly, penetration and assimilation of applied extract may vary depending upon its hydrophilic nature, mode of application, environmental conditions (light, temperature, relative humidity, etc.), ontogenesis, and permeability of plant surface (Fernández and Brown, 2013; Fernández et al., 2017). A turgid cell might not absorb more water resulting in no absorption of aqueous extract. Likewise, a PE prepared through organic solvent-based extraction might also not get absorbed due to the hydrophilic nature of plant cuticle. All these variable factors greatly influence plant physiological response to PEs. A stepwise illustration of the preparation of PEs is presented in Figure 3.
Usually, PEs are applied to plants through following three methods;
(a) Foliar spray (Habib et al., 2012; Lorenzo et al., 2019).
(b) Soil based application (Pehlivan, 2018; Hassanein et al., 2019; Brazales-Cevallos et al., 2022).
(c) Biopriming (seed priming) (Panuccio et al., 2018; ElSayed et al., 2022).
For a good penetration and assimilation of ingredients, PEs should be water soluble (or in any other suitable solvent). To overcome the lipophilicity and molecular size like uptake problems might be solved by mixing PEs with surfactants or other additives (Yakhin et al., 2017). Similarly, the absorbability of PEs also depends upon the molecular structure of cuticle of the plant under study, environmental conditions, and other extrinsic factors (Bulgari et al., 2015).
Implications of Plant Extracts Against Salinity
The basic aim of the use of PEs is reinforcing the plant responses to salinity so as to sustain the cellular homeostasis. Exogenous application of PEs is found to take part in the signaling, primary and secondary metabolic pathways, and other physiological processes of plant (Table 2). Similarly, morphological, and anatomical adjustments are important mechanisms for stress regulation in plants. Improvement in growth traits including plant height, root and shoot length, fresh and dry weight of shoots, fresh and dry weight of roots, root/shoot ratio, number of leaves, leaf area, leaf thickness leaf relative water content (RWC), number of pods, pods weight, number of seeds, seed weight, grain yield, biological yield, and harvest index results on the use of PEs on salt stressed plants (Habib et al., 2012; Rady et al., 2015; Latif and Mohamed, 2016; Merwad, 2020; Suryaman et al., 2021). These altered characteristics might help glycophytes in better acclimation and tolerance, presumably, by enhanced robustness, a higher accumulation of reserves, photosynthetic pigments, gaseous exchange, and ionic compartmentalization. Nevertheless, nutrients are the fundamental players for such alterations providing energy and substrates. Nutrient uptake is greatly challenged under salinity conditions (Munns and Tester, 2008; Zörb et al., 2019) that can be assuaged by the exogenous application of PEs. Several studies have reported an improvement in nutrient (particularly NPK, Fe, Zn, and Mn) uptake and assimilation in salt stressed plant upon the application of PEs (Bulgari et al., 2015; Rady et al., 2019a; Merwad, 2020).
The PEs rich in antioxidants can be associated to facilitate the stress mitigation processes through enzymatic and non-enzymatic processes. For instance, salinity caused osmotic stress results in lower ψs, photosynthetic performance (Fv/Fm, intracellular CO2 concentration, CO2 assimilation rate, net photosynthetic rate, transpiration rate, and stomatal conductance), altered ions concentrations (K+/Na+, Ca2+/Na+, K+ + Ca2+/Na+), and disrupted hormonal content. These perturbations could be mitigated by the enhanced content of GSH and AsA that improve the tolerance by decreasing electrolyte leakage and stabilizing membrane integrity. Enhanced content of osmoprotectants/osmolytes (GB, proline, pipecolate betaine mannitol, sorbitol, etc.) could be attributed to enhanced ionic, hormonal, and osmotic adjustments that result in improved acclimation, photosynthetic efficiency, growth, and yield (Bulgari et al., 2015; ur Rehman et al., 2021). Overall, lower concentrations of PEs have been found to induce these positive results as higher concentrations might produce harmful results.
Similarly, PEs are efficient sources of ROS and RNS scavengers that are needed to mitigate toxic radicals and stabilize cell homeostasis. They can restore the K+/Na+ ratios of both root and leaf (Abbas et al., 2010). Desoky et al. (2020) reported a decreased in the contents of these reactive species on the application of seed extracts on Vigna unguiculata irrigated with seawater (EC: 3.5 and 7 dS m–1). Among others, they attributed the improved K+/Na+ ratio and membrane integrity to antioxidants and polyphenols rich seed extracts. Analogous results have been reported in case of Phaseolus vulgaris, Vicia faba, Triticum aestivum, Lupinus termis, and Zea mays plants subjected to varying degree of salt concentrations (Rady and Mohamed, 2015; Latif and Mohamed, 2016; Latef et al., 2019; Nessim and Kasim, 2019; Aboualhamed and Loutfy, 2020; ur Rehman et al., 2021). In another study, presence of phenols, flavonoids, and AsA in Rosmarinus officinalis L. extracts was associated to salinity alleviation in apple seedlings (Mahmoudand and Dahab, 2018). Each plant responds differently as per PEs used and therefore various oxidative stress mitigation strategies can be observed. Likewise, the negligible or no activity of some antioxidants might be attributed to various fluctuations in the activation of corresponding transcription factor/genes. A demonstration of negative effects of salinity on plant and its mitigation by PEs is illustrated in Figure 4.
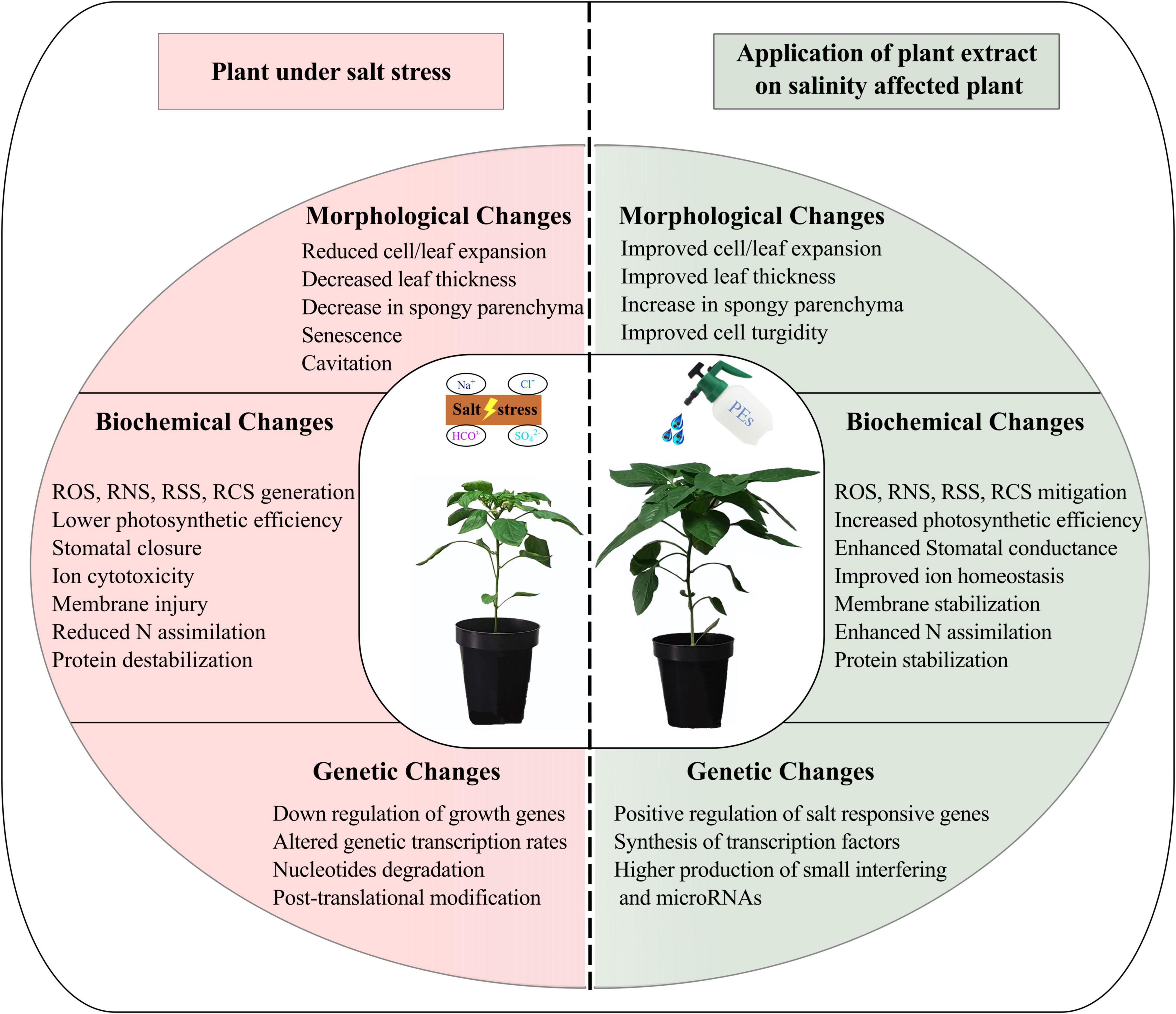
Figure 4. Illustration of the impact of salinity, in terms of morphological, biochemical, and genetic changes, on plants (Left) in comparison with the plants supplied with PEs (Right).
In the same way, PEs carried phytohormones, particularly ABA, JA, ethylene, and SA, stimulate the signaling pathway; whereby triggering various transcription factors and stress related genes. Equally, phytohormones might also be associated to bolster the photosynthetic machinery and overall ionic balance in the cell. In a recent study, Cupressus macrocarpa foliar extract primed seed were found to have upregulated various stress related genes (CuZnSOD2, CAT1, DHAR, APX, PrxQ, and GR) in Cucurbita pepo (ElSayed et al., 2022). Upregulation of PrxQ-, APX-, SOD-, DHAR-, GR-, and CAT-encoding genes have also reported in Pisum sativum when its seeds were primed with Glycyrrhiza glabra root extracts and subjected to 150 mM NaCl stress (Desoky et al., 2019). Another evidence of the possible role of PEs in signaling was backed in a study on Arabidopsis, where moringa leaf extracts facilitated salinity mitigation by transcriptionally activating ABA-, SA-, AUX-, and ET-related signaling pathways (Brazales-Cevallos et al., 2022). ABA is also reported to regulate the transcription factor ABI5 (ABSCISIC ACID INSENSIVE 1) that is required by plants to activate ABI5 expression and salt acclimation (Zörb et al., 2019). However, comprehensive studies to establish the molecular basis of PEs phytohormones in signaling are either in dearth or non-existent.
Limitations and Future Perspective
Plant extracts being amalgams of various biological compounds make it difficult to map out their exact mode of action. Studies undertaken to compare the individual effects of various osmolytes (e.g., GB) or phytohormones (e.g., SA) along with PEs, concluded the supremacy of PEs as being more efficient (Abbas et al., 2010; Rady et al., 2015). Similarly, the role of PEs in signal transduction remains ambiguous as exogenous application of relevant compounds (i.e., ethylene, AsA, etc.) can also elicit the plant response. Either PEs work as elicitors of natural compounds or PEs carried molecule assimilation results in the desired results, needs further investigation. Similarly, the involvement of protein kinases has already been documented in saline environments (Zörb et al., 2019; Arif et al., 2020), but no such studies have been undertaken upon the use of PEs. Likewise, elucidation of the potential role of reactive sulfur species (RSS) (Corpas and Barroso, 2015) and RCS with respect to salinity and its subsequent mitigation through PEs might open new avenues of research. In addition, application of nanoparticles (NPs) coated PEs might improve their efficiency exponentially as various NPs have been documented effective against salinity (Zulfiqar and Ashraf, 2021b). Nevertheless, intensive research is needed for the application of PEs coated with NPs because of the reported toxicities of NPs based on their physiochemical properties and plant species (Ahmad et al., 2021a).
The role of PEs in morphological and anatomical traits like shape and size of palisade and mesophyll cells, integrity of grana and thylakoids, integrity of cristae, the number and size of plastoglobuli, number and diameter of xylem and phloem tissues, width of cortex, suberin and casparian strips development, root-, shoot-apex, endodermis, and exodermis etc. needs more scientific attention, as these factors play crucial role in gaseous exchanges, ions permeability, ψw, ψs, and energy generation processes (Acosta-Motos et al., 2017). Analogously, comprehensive studies using omics approaches (genomics, transcriptomics, proteomics, metabolomics, and bioinformatics) can further shed lights on positive or negative regulators of morphological-, metabolic-, and genomic-adjustments, target molecules, and the potential receptors activated by the use of PEs. The already identified signaling signatures, genes, and other key metabolites can be used to investigate such processes on the use of PEs. The influence of PEs on interference RNA mechanism to combat salinity also remains an enticing research area.
Use of PEs to combat salinity is a green, ecofriendly, and sustainable approach. This also opens further doors of investigation on the use of invasive plant species to be used as salinity moderator. Another important aspect is that plant response owing to PEs varies greatly from species to species and even within the same species. This might be answered by undertaking further comparative studies. Furthermore, use of salinity to trigger the production of various osmolytes and antioxidants can be utilized as an elicitation approach. Plants subjected to salinity can be used to prepare PEs that might prove more promising against salinity than conventional ones.
Conclusion
Salt stress has become a consistent problem in agriculture over the past few years, and was reported to culminate around 900 million ha in 2020. Plants perceive salinity by sensors, e.g., cell surface-based receptors, protein kinases, etc., resulting in a cascade of phosphorylation that regulates subsequent genetic expression. Salinity results in ionic, osmotic, and oxidative stress, which further disrupts various physiological and metabolic processes in plants. Use of PEs to combat salinity is an efficient, economical, and sustainable approach. Whole plants or parts of plants, i.e., roots, leaves, flowers, bark, seeds, pollens, etc. can be used to prepare PEs through aqueous or organic-solvent extraction techniques. PEs are multicomponent organic mixtures, containing vitamins, carotenoids, amino acids, phytohormones, mineral nutrients, phenolics, and antioxidants, etc., which facilitate stress signaling, genes regulation, redox metabolism, and synthesis of various proteins and metabolites. The degree of impact of PEs depends on various factors like plant species, age of plant, application method, etc. Molecular characterization of the PEs produced effects can pave the way for elucidating their comprehensive mechanism of action.
Author Contributions
AA, BB, and VM: conceptualization. AA: study design, data collection and analysis, draft writing and editing, and Illustrations. VM and BB: critical analysis, revision, and supervision. All authors have read and agreed to the published version of the manuscript.
Funding
This work was supported by the projects: “VIRTUOUS” funded from the European Union’s Horizon 2020 Project H2020-MSCA-RISE-2019. Ref. 872181, “SUSTAINABLE” funded from the European Union’s Horizon 2020 Project H2020-MSCA-RISE-2020. Ref. 101007702, and the “Project of Excellence” from FEDER (Fondo Europeo de Desarrollo Regional)- Junta de Andalucia 2018. Ref. P18-H0-4700. The funding organizations had no role in study design, data collection and analysis, decision to publish, or preparation of the manuscript.
Conflict of Interest
The authors declare that the research was conducted in the absence of any commercial or financial relationships that could be construed as a potential conflict of interest.
Publisher’s Note
All claims expressed in this article are solely those of the authors and do not necessarily represent those of their affiliated organizations, or those of the publisher, the editors and the reviewers. Any product that may be evaluated in this article, or claim that may be made by its manufacturer, is not guaranteed or endorsed by the publisher.
References
Abbas, W., Ashraf, M., and Akram, N. A. (2010). Alleviation of salt-induced adverse effects in eggplant (Solanum melongena L.) by glycinebetaine and sugarbeet extracts. Sci. Hortic. 125, 188–195. doi: 10.1016/j.scienta.2010.04.008
Abd El-Mageed, T. A., Semida, W. M., and Rady, M. M. (2017). Moringa leaf extract as biostimulant improves water use efficiency, physio-biochemical attributes of squash plants under deficit irrigation. Agric. Water Manag. 193, 46–54. doi: 10.1016/j.agwat.2017.08.004
Aboualhamed, M. F., and Loutfy, N. (2020). Ocimum basilicum Leaf Extract Induces Salinity Stress Tolerance in Faba Bean Plants. Egypt. J. Bot. 60, 681–690.
Acosta-Motos, J. R., Ortuño, M. F., Bernal-Vicente, A., Diaz-Vivancos, P., Sanchez-Blanco, M. J., and Hernandez, J. A. (2017). Plant responses to salt stress: adaptive mechanisms. Agronomy 7:18. doi: 10.3390/agronomy7010018
Adetunji, C., Fawole, O., Arowora, K., Nwaubani, S., Ajayi, E., Oloke, J., et al. (2012). Quality and safety of Citrus Sinensis coated with Hydroxypropylmethylcellulose edible coatings containing Moringa oleifera extract stored at ambient temperature. Global J. Sci. Front. Res. Biol. Technol. Genet. 12, 29–33.
Ahmad, A., Ordoñez, J., Cartujo, P., and Martos, V. (2021b). Remotely Piloted Aircraft (RPA) in Agriculture: a Pursuit of Sustainability. Agronomy 11:7. doi: 10.3390/agronomy11010007
Ahmad, A., Hashmi, S. S., Palma, J. M., and Corpas, F. J. (2021a). Influence of metallic, metallic oxide, and organic nanoparticles on plant physiology. Chemosphere 290:133329. doi: 10.1016/j.chemosphere.2021.133329
Albaladejo, I., Egea, I., Morales, B., Flores, F. B., Capel, C., Lozano, R., et al. (2018). Identification of key genes involved in the phenotypic alterations of res (restored cell structure by salinity) tomato mutant and its recovery induced by salt stress through transcriptomic analysis. BMC Plant Biol. 18:213. doi: 10.1186/s12870-018-1436-9
Ali, E., Hassan, F., and Elgimabi, M. (2018). Improving the growth, yield and volatile oil content of Pelargonium graveolens L. Herit by foliar application with moringa leaf extract through motivating physiological and biochemical parameters. S. Afr. J. Bot. 119, 383–389. doi: 10.1016/j.sajb.2018.10.003
Ali, M., Cheng, Z.-H., Hayat, S., Ahmad, H., Ghani, M. I., and Tao, L. (2019). Foliar spraying of aqueous garlic bulb extract stimulates growth and antioxidant enzyme activity in eggplant (Solanum melongena L.). J. Integr. Agric. 18, 1001–1013. doi: 10.1016/s2095-3119(18)62129-x
Amirbakhtiar, N., Ismaili, A., Ghaffari, M. R., Nazarian Firouzabadi, F., and Shobbar, Z.-S. (2019). Transcriptome response of roots to salt stress in a salinity-tolerant bread wheat cultivar. PLoS One 14:e0213305. doi: 10.1371/journal.pone.0213305
Arif, Y., Singh, P., Siddiqui, H., Bajguz, A., and Hayat, S. (2020). Salinity induced physiological and biochemical changes in plants: an omic approach towards salt stress tolerance. Plant Physiol. Biochem. 156, 64–77. doi: 10.1016/j.plaphy.2020.08.042
Arora, N. K. (2019). Impact of climate change on agriculture production and its sustainable solutions. Environ. Sustain. 2, 95–96. doi: 10.1007/s42398-019-00078-w
Asada, K. (1999). The water-water cycle in chloroplasts: scavenging of active oxygens and dissipation of excess photons. Ann. Rev. Plant Biol. 50, 601–639. doi: 10.1146/annurev.arplant.50.1.601
Ashraf, M., and Akram, N. A. (2009). Improving salinity tolerance of plants through conventional breeding and genetic engineering: an analytical comparison. Biotechnol. Adv. 27, 744–752. doi: 10.1016/j.biotechadv.2009.05.026
Ashraf, M., and Harris, P. (2004). Potential biochemical indicators of salinity tolerance in plants. Plant Sci. 166, 3–16. doi: 10.1016/j.plantsci.2003.10.024
Athar, H. R., and Ashraf, M. (2009). “Strategies for crop improvement against salinity and drought stress: An overview,” in Salinity and water stress, eds M. Ashraf, M. Ozturk, and H. Athar (Dordrecht: Springer), 1–16. doi: 10.3390/ijms22116119
Bajwa, A. A., Farooq, M., and Nawaz, A. (2018). Seed priming with sorghum extracts and benzyl aminopurine improves the tolerance against salt stress in wheat (Triticum aestivum L.). Physiol. Mol. Biol. Plants 24, 239–249. doi: 10.1007/s12298-018-0512-9
Beadle, C. (1993). “Growth analysis,” in Photosynthesis and Production in a Changing Environment, ed. D. O. Hall (Berlin: Springer), 36–46.
Brazales-Cevallos, D. K., Romero-Contreras, Y. J., Vences-Guzmán, M. Á, Torres, M., Aviles-Baltazar, N. Y., Sohlenkamp, C., et al. (2022). Transcriptional characterization of the biostimulant effect of Moringa oleifera leaf extracts using Arabidopsis thaliana as a model. S. Afr. J. Bot. 144, 250–256. doi: 10.1016/j.sajb.2021.09.011
Briceño-Domínguez, D., Hernández-Carmona, G., Moyo, M., Stirk, W., and van Staden, J. (2014). Plant growth promoting activity of seaweed liquid extracts produced from Macrocystis pyrifera under different pH and temperature conditions. J. Appl. Phycol. 26, 2203–2210. doi: 10.1007/s10811-014-0237-2
Brockman, H. G., and Brennan, R. F. (2017). The effect of foliar application of Moringa leaf extract on biomass, grain yield of wheat and applied nutrient efficiency. J. Plant Nutr. 40, 2728–2736. doi: 10.1080/01904167.2017.1381723
Brown, P., and Saa, S. (2015). Biostimulants in agriculture. Front. Plant Sci. 6:671. doi: 10.3389/fpls.2015.00671
Bulgari, R., Cocetta, G., Trivellini, A., Vernieri, P., and Ferrante, A. (2015). Biostimulants and crop responses: a review. Biol. Agric. Hortic. 31, 1–17. doi: 10.1080/01448765.2014.964649
Bulgari, R., Morgutti, S., Cocetta, G., Negrini, N., Farris, S., Calcante, A., et al. (2017). Evaluation of borage extracts as potential biostimulant using a phenomic, agronomic, physiological, and biochemical approach. Front. Plant Sci. 8:935. doi: 10.3389/fpls.2017.00935
Calvo, P., Nelson, L., and Kloepper, J. W. (2014). Agricultural uses of plant biostimulants. Plant Soil 383, 3–41. doi: 10.1007/s11104-014-2131-8
Cao, Y.-R., Chen, S.-Y., and Zhang, J.-S. (2008). Ethylene signaling regulates salt stress response: an overview. Plant Signal. Behav. 3, 761–763. doi: 10.4161/psb.3.10.5934
Cassaniti, C., Romano, D., and Timothy, J. F. (2012). “The Response of Ornamental Plants to Saline Irrigation Water,” in Irrigation-Water Management, Pollution and Alternative Strategies, eds I. García-Garizábal and R. Abrahao (London, UK: IntechOpen Limited), 130–158.
Chakraborty, K., Sairam, R. K., and Bhattacharya, R. (2012). Differential expression of salt overly sensitive pathway genes determines salinity stress tolerance in Brassica genotypes. Plant Physiol. Biochem. 51, 90–101. doi: 10.1016/j.plaphy.2011.10.001
Chen, X., Ding, Y., Yang, Y., Song, C., Wang, B., Yang, S., et al. (2021). Protein kinases in plant responses to drought, salt, and cold stress. J. Integr. Plant Biol. 63, 53–78. doi: 10.1111/jipb.13061
Corpas, F. J. (2016). Reactive nitrogen species (RNS) in plants under physiological and adverse environmental conditions: current view. Prog. Bot. 78, 97–119. doi: 10.1007/124_2016_3
Corpas, F. J., and Barroso, J. B. (2015). Reactive sulfur species (RSS): possible new players in the oxidative metabolism of plant peroxisomes. Front. Plant Sci. 6:116. doi: 10.3389/fpls.2015.00116
Curtis, P. S., and Lauchli, A. (1986). The role of leaf area development and photosynthetic capacity in determining growth of kenaf under moderate salt stress. Funct. Plant Biol. 13, 553–565. doi: 10.1071/pp9860553
Deinlein, U., Stephan, A. B., Horie, T., Luo, W., Xu, G., and Schroeder, J. I. (2014). Plant salt-tolerance mechanisms. Trends Plant Sci. 19, 371–379.
Desoky, E.-S. M., El-maghraby, L. M., Awad, A. E., Abdo, A. I., Rady, M. M., and Semida, W. M. (2020). Fennel and ammi seed extracts modulate antioxidant defence system and alleviate salinity stress in cowpea (Vigna unguiculata). Sci. Hortic. 272:109576. doi: 10.1016/j.scienta.2020.109576
Desoky, E.-S. M., ElSayed, A. I., Merwad, A.-R. M., and Rady, M. M. (2019). Stimulating antioxidant defenses, antioxidant gene expression, and salt tolerance in Pisum sativum seedling by pretreatment using licorice root extract (LRE) as an organic biostimulant. Plant Physiol. Biochem. 142, 292–302. doi: 10.1016/j.plaphy.2019.07.020
Dhanaraj, B., Papanna, M. K., Adinarayanan, S., Vedachalam, C., Sundaram, V., Shanmugam, S., et al. (2015). Prevalence and risk factors for adult pulmonary tuberculosis in a metropolitan city of South India. PLoS One 10:e0124260. doi: 10.1371/journal.pone.0124260
Dong, H., Li, W., Tang, W., and Zhang, D. (2008). Furrow seeding with plastic mulching increases stand establishment and lint yield of cotton in a saline field. Agron. J. 100, 1640–1646. doi: 10.2134/agronj2008.0074
Dong, H., Li, W., Tang, W., and Zhang, D. (2009). Early plastic mulching increases stand establishment and lint yield of cotton in saline fields. Field Crops Res. 111, 269–275. doi: 10.1016/j.fcr.2009.01.001
Drobek, M., Frąc, M., and Cybulska, J. (2019). Plant biostimulants: importance of the quality and yield of horticultural crops and the improvement of plant tolerance to abiotic stress—A review. Agronomy 9:335. doi: 10.3390/agronomy9060335
Du Jardin, P. (2015). Plant biostimulants: definition, concept, main categories and regulation. Sci. Hortic. 196, 3–14. doi: 10.1016/j.scienta.2015.09.021
ElSayed, A. I., Rafudeen, M. S., Ganie, S. A., Hossain, M. S., and Gomaa, A. M. (2022). Seed priming with cypress leaf extract enhances photosynthesis and antioxidative defense in zucchini seedlings under salt stress. Sci. Hortic. 293:110707. doi: 10.1016/j.scienta.2021.110707
Elzaawely, A. A., Ahmed, M. E., Maswada, H. F., Al-Araby, A. A., and Xuan, T. D. (2018). Growth traits, physiological parameters and hormonal status of snap bean (Phaseolus vulgaris L.) sprayed with garlic cloves extract. Arch. Agron. Soil Sci. 64, 1068–1082. doi: 10.1080/03650340.2017.1410543
European-Union (2020). European Green Deal [Online]. Available: https://ec.europa.eu/clima/eu-action/european-green-deal_en (accessed Oct 1, 2022).
Fahad, S., Hussain, S., Matloob, A., Khan, F. A., Khaliq, A., Saud, S., et al. (2015). Phytohormones and plant responses to salinity stress: a review. Plant Growth Reg. 75, 391–404. doi: 10.1007/s10725-014-0013-y
Fancy, N. N., Bahlmann, A. K., and Loake, G. J. (2017). Nitric oxide function in plant abiotic stress. Plant Cell Environ. 40, 462–472. doi: 10.1111/pce.12707
Farooq, M., Gogoi, N., Hussain, M., Barthakur, S., Paul, S., Bharadwaj, N., et al. (2017). Effects, tolerance mechanisms and management of salt stress in grain legumes. Plant Physiol. Biochem. 118, 199–217. doi: 10.1016/j.plaphy.2017.06.020
Fernández, V., and Brown, P. H. (2013). From plant surface to plant metabolism: the uncertain fate of foliar-applied nutrients. Front. Plant Sci. 4:289. doi: 10.3389/fpls.2013.00289
Fernández, V., Bahamonde, H. A., Javier Peguero-Pina, J., Gil-Pelegrín, E., Sancho-Knapik, D., Gil, L., et al. (2017). Physico-chemical properties of plant cuticles and their functional and ecological significance. J. Exp. Bot. 68, 5293–5306. doi: 10.1093/jxb/erx302
Franco-Navarro, J. D., Brumós, J., Rosales, M. A., Cubero-Font, P., Talón, M., and Colmenero-Flores, J. M. (2016). Chloride regulates leaf cell size and water relations in tobacco plants. J. Exp. Bot. 67, 873–891. doi: 10.1093/jxb/erv502
Garriga, M., Retamales, J. B., Romero-Bravo, S., Caligari, P. D., and Lobos, G. A. (2014). Chlorophyll, anthocyanin, and gas exchange changes assessed by spectroradiometry in Fragaria chiloensis under salt stress. J. Integr. Plant Biol. 56, 505–515. doi: 10.1111/jipb.12193
Ghaffari, M. R., Ghabooli, M., Khatabi, B., Hajirezaei, M. R., Schweizer, P., and Salekdeh, G. H. (2016). Metabolic and transcriptional response of central metabolism affected by root endophytic fungus Piriformospora indica under salinity in barley. Plant Mol. Biol. 90, 699–717. doi: 10.1007/s11103-016-0461-z
Ghezal, N., Rinez, I., Sbai, H., Saad, I., Farooq, M., Rinez, A., et al. (2016). Improvement of Pisum sativum salt stress tolerance by bio-priming their seeds using Typha angustifolia leaves aqueous extract. S. Afr. J. Bot. 105, 240–250. doi: 10.1016/j.sajb.2016.04.006
Goldberg, S. (2008). Mechanical/physical methods of cell disruption and tissue homogenization. Methods Mol. Biol. 424, 3–22. doi: 10.1007/978-1-60327-064-9_1
Gowdy, J. (2020). Our hunter-gatherer future: climate change, agriculture and uncivilization. Futures 115:102488. doi: 10.1016/j.futures.2019.102488
Guo, S.-M., Tan, Y., Chu, H.-J., Sun, M.-X., and Xing, J.-C. (2019). Transcriptome sequencing revealed molecular mechanisms underlying tolerance of Suaeda salsa to saline stress. PLoS One 14:e0219979. doi: 10.1371/journal.pone.0219979
Gupta, B., and Huang, B. (2014). Mechanism of salinity tolerance in plants: physiological, biochemical, and molecular characterization. Int. J. Genomics 2014:701596. doi: 10.1155/2014/701596
Gururani, M. A., Venkatesh, J., and Tran, L. S. P. (2015). Regulation of photosynthesis during abiotic stress-induced photoinhibition. Mol. Plant 8, 1304–1320. doi: 10.1016/j.molp.2015.05.005
Habib, N., Ashraf, M., Ali, Q., and Perveen, R. (2012). Response of salt stressed okra (Abelmoschus esculentus Moench) plants to foliar-applied glycine betaine and glycine betaine containing sugarbeet extract. S. Afr. J. Bot. 83, 151–158. doi: 10.1016/j.sajb.2012.08.005
Hasanuzzaman, M., Bhuyan, M., Zulfiqar, F., Raza, A., Mohsin, S. M., Mahmud, J. A., et al. (2020). Reactive oxygen species and antioxidant defense in plants under abiotic stress: revisiting the crucial role of a universal defense regulator. Antioxidants 9:681. doi: 10.3390/antiox9080681
Hassan, F., Al-Yasi, H., Ali, E., Alamer, K., Hessini, K., Attia, H., et al. (2020). Mitigation of salt-stress effects by moringa leaf extract or salicylic acid through motivating antioxidant machinery in damask rose. Can. J. Plant Sci. 101, 157–165. doi: 10.1016/j.plaphy.2021.05.042
Hassanein, R. A., Abdelkader, A. F., and Faramawy, H. M. (2019). Moringa leaf extracts as biostimulants-inducing salinity tolerance in the sweet basil plant. Egypt. J. Bot. 59, 303–318.
Hernández, J. A., Ferrer, M. A., Jiménez, A., Barceló, A. R., and Sevilla, F. (2001). Antioxidant systems and O2.-/H2O2 production in the apoplast of pea leaves. Its relation with salt-induced necrotic lesions in minor veins. Plant Physiol. 127, 817–831. doi: 10.1104/pp.010188
Hernandez, J., Olmos, E., Corpas, F., Sevilla, F., and Del Rio, L. (1995). Salt-induced oxidative stress in chloroplasts of pea plants. Plant Sci. 105, 151–167. doi: 10.1016/0168-9452(94)04047-8
Hörtensteiner, S. (2006). Chlorophyll degradation during senescence. Annu. Rev. Plant Biol. 57, 55–77. doi: 10.1146/annurev.arplant.57.032905.105212
Howladar, S. M. (2014). A novel Moringa oleifera leaf extract can mitigate the stress effects of salinity and cadmium in bean (Phaseolus vulgaris L.) plants. Ecotoxicol. Environ. Saf. 100, 69–75. doi: 10.1016/j.ecoenv.2013.11.022
Huang, P., He, L., Abbas, A., Hussain, S., Hussain, S., Du, D., et al. (2021). Seed priming with sorghum water extract improves the performance of camelina (camelina sativa (L.) crantz.) under salt stress. Plants 10:749. doi: 10.3390/plants10040749
Ikrina, M. A., and Kolbin, A. M. (2004). Peptides as universal biological regulators. Her. Russ. Acad. Sci. 80, 419–429. doi: 10.1134/S1019331610050011
Iqbal, N., Umar, S., Khan, N. A., and Khan, M. I. R. (2014). A new perspective of phytohormones in salinity tolerance: regulation of proline metabolism. Environ. Exp. Bot. 100, 34–42. doi: 10.1016/j.envexpbot.2013.12.006
Ismail, A. M., and Horie, T. (2017). Genomics, physiology, and molecular breeding approaches for improving salt tolerance. Ann. Rev. Plant Biol. 68, 405–434. doi: 10.1146/annurev-arplant-042916-040936
Jithesh, M., Shukla, P. S., Kant, P., Joshi, J., Critchley, A. T., and Prithiviraj, B. (2019). Physiological and transcriptomics analyses reveal that Ascophyllum nodosum extracts induce salinity tolerance in Arabidopsis by regulating the expression of stress responsive genes. J. Plant Growth Reg. 38, 463–478. doi: 10.1007/s00344-018-9861-4
Kauffman, G. L., Kneivel, D. P., and Watschke, T. L. (2007). Effects of a biostimulant on the heat tolerance associated with photosynthetic capacity, membrane thermostability, and polyphenol production of perennial ryegrass. Crop Sci. 47, 261–267. doi: 10.2135/cropsci2006.03.0171
Kaur, C., Sharma, S., Singla-Pareek, S. L., and Sopory, S. K. (2016). Methylglyoxal detoxification in plants: role of glyoxalase pathway. Ind. J. Plant Physiol. 21, 377–390. doi: 10.1007/s40502-016-0260-1
Khan, T. A., Yusuf, M., Ahmad, A., Bashir, Z., Saeed, T., Fariduddin, Q., et al. (2019). Proteomic and physiological assessment of stress sensitive and tolerant variety of tomato treated with brassinosteroids and hydrogen peroxide under low-temperature stress. Food Chem. 289, 500–511. doi: 10.1016/j.foodchem.2019.03.029
La Torre, A., Battaglia, V., and Caradonia, F. (2016). An overview of the current plant biostimulant legislations in different European Member States. J. Sci. Food Agric. 96, 727–734. doi: 10.1002/jsfa.7358
Lamers, J., Van Der Meer, T., and Testerink, C. (2020). How plants sense and respond to stressful environments. Plant Physiol. 182, 1624–1635. doi: 10.1104/pp.19.01464
Latef, A. A. H. A., Mostofa, M. G., Rahman, M. M., Abdel-Farid, I. B., and Tran, L.-S. P. (2019). Extracts from yeast and carrot roots enhance maize performance under seawater-induced salt stress by altering physio-biochemical characteristics of stressed plants. J. Plant Growth Reg. 38, 966–979. doi: 10.1007/s00344-018-9906-8
Latef, A. A., Alhmad, M. F. A., and Hammad, S. A. (2017). Foliar application of fresh moringa leaf extract overcomes salt stress in fenugreek (Trigonellafoenum-graecum) plants. Egypt. J. Bot. 57, 157–179.
Latif, H., and Mohamed, H. (2016). Exogenous applications of moringa leaf extract effect on retrotransposon, ultrastructural and biochemical contents of common bean plants under environmental stresses. S. Afr. J. Bot. 106, 221–231. doi: 10.1016/j.sajb.2016.07.010
Li, H., Yue, H., Li, L., Liu, Y., Zhang, H., Wang, J., et al. (2021). Seed biostimulant Bacillus sp. MGW9 improves the salt tolerance of maize during seed germination. AMB Express 11, 1–15. doi: 10.1186/s13568-021-01237-1
Lim, S. M., Goh, Y. M., Kuan, W. B., and Loh, S. P. (2014). Effect of germinated brown rice extracts on pancreatic lipase, adipogenesis and lipolysis in 3T3-L1 adipocytes. Lipids Health Dis. 13:169. doi: 10.1186/1476-511X-13-169
Longstreth, D. J., and Nobel, P. S. (1979). Salinity effects on leaf anatomy: consequences for photosynthesis. Plant Physiol. 63, 700–703. doi: 10.1104/pp.63.4.700
Lorenzo, P., Souza-Alonso, P., Guisande-Collazo, A., and Freitas, H. (2019). Influence of Acacia dealbata Link bark extracts on the growth of Allium cepa L. plants under high salinity conditions. J. Sci. Food Agric. 99, 4072–4081. doi: 10.1002/jsfa.9637
Lötze, E., and Hoffman, E. W. (2016). Nutrient composition and content of various biological active compounds of three South African-based commercial seaweed biostimulants. J. Appl. Phycol. 28, 1379–1386. doi: 10.1007/s10811-015-0644-z
Mahmoudand, R. A., and Dahab, A. A. (2018). Response of Apple Seedlings Grown Under Saline Conditions to Natural Plant Extracts. Biosci. Res. 15, 589–601.
Mangrauthia, S. K., Agarwal, S., Sailaja, B., Madhav, M. S., and Voleti, S. (2013). “MicroRNAs and their role in salt stress response in plants,” in in Salt stress in plants, eds P. Ahmad, M. M. Azooz, and M. N. V. Prasad (New York, NY: Springer), 15–46. doi: 10.1007/978-1-4614-6108-1_2
Mano, J. I, Biswas, M., and Sugimoto, K. (2019). Reactive carbonyl species: a missing link in ROS signaling. Plants 8:391. doi: 10.3390/plants8100391
Mano, J. I. (2012). Reactive carbonyl species: their production from lipid peroxides, action in environmental stress, and the detoxification mechanism. Plant Physiol. Biochem. 59, 90–97. doi: 10.1016/j.plaphy.2012.03.010
Merwad, A.-R. M. (2017). Effect of humic and fulvic substances and Moringa leaf extract on Sudan grass plants grown under saline conditions. Can. J. Soil Sci. 97, 703–716.
Merwad, A.-R. M. (2020). Mitigation of salinity stress effects on growth, yield and nutrient uptake of wheat by application of organic extracts. Commun. Soil Sci. Plant Anal. 51, 1150–1160. doi: 10.1080/00103624.2020.1751188
Michler, C. (2014). Overexpression of AtSTO1 leads to improved salt tolerance in Populus tremula× P. alba. Transgenic Res. 23, 817–826. doi: 10.1007/s11248-014-9808-x
Milić, B., Tarlanović, J., Keserović, Z., Magazin, N., Miodragović, M., and Popara, G. (2018). Bioregulators can improve fruit size, yield and plant growth of northern highbush blueberry (Vaccinium corymbosum L.). Sci. Hortic. 235, 214–220. doi: 10.1016/j.scienta.2018.03.004
Mittova, V., Tal, M., Volokita, M., and Guy, M. (2003). Up-regulation of the leaf mitochondrial and peroxisomal antioxidative systems in response to salt-induced oxidative stress in the wild salt-tolerant tomato species Lycopersicon pennellii. Plant Cell Environ. 26, 845–856. doi: 10.1046/j.1365-3040.2003.01016.x
Mochida, K., and Shinozaki, K. (2011). Advances in omics and bioinformatics tools for systems analyses of plant functions. Plant Cell Physiol. 52, 2017–2038. doi: 10.1093/pcp/pcr153
Morales, M., Sánchez-Blanco, M., Olmos, E., Torrecillas, A., and Alarcon, J. (1998). Changes in the growth, leaf water relations and cell ultrastructure in Argyranthemum coronopifolium plants under saline conditions. J. Plant Physiol. 153, 174–180. doi: 10.1016/s0176-1617(98)80062-x
Mostofa, M. G., Ghosh, A., Li, Z.-G., Siddiqui, M. N., Fujita, M., and Tran, L.-S. P. (2018). Methylglyoxal–a signaling molecule in plant abiotic stress responses. Free Radic. Biol. Med. 122, 96–109. doi: 10.1016/j.freeradbiomed.2018.03.009
Munns, R. (2002). Comparative physiology of salt and water stress. Plant Cell Environ. 25, 239–250. doi: 10.1046/j.0016-8025.2001.00808.x
Munns, R., and Tester, M. (2008). Mechanisms of salinity tolerance. Annu. Rev. Plant Biol. 59, 651–681.
Mutlu-Durak, H., and Yildiz Kutman, B. (2021). Seed Treatment with Biostimulants Extracted from Weeping Willow (Salix babylonica) Enhances Early Maize Growth. Plants 10:1449. doi: 10.3390/plants10071449
Nahar, K., Hasanuzzaman, M., and Fujita, M. (2016). “Roles of osmolytes in plant adaptation to drought and salinity, Iqbal,” in Osmolytes and plants acclimation to changing environment: Emerging omics technologies, eds N. Nazar and R. A. Khan (Berlin: Springer), 37–68. doi: 10.1007/978-81-322-2616-1_4
Nareshkumar, A., Subbarao, S., Vennapusa, A. R., Ashwin, V., Banarjee, R., Kulkarni, M. J., et al. (2020). Enzymatic and non-enzymatic detoxification of reactive carbonyl compounds improves the oxidative stress tolerance in cucumber, tobacco and rice seedlings. J. Plant Growth Reg. 39, 1359–1372. doi: 10.1007/s00344-020-10072-w
Nasir, M., Khan, A. S., Basra, S. A., and Malik, A. U. (2016). Foliar application of moringa leaf extract, potassium and zinc influence yield and fruit quality of ‘Kinnow’mandarin. Sci. Hortic. 210, 227–235. doi: 10.1016/j.scienta.2016.07.032
Nessim, A., and Kasim, W. (2019). Physiological impact of seed priming with CaCl2 or Carrot root extract on Lupinus termis plants fully grown under salinity stress. Egypt. J. Bot. 59, 763–777.
Nolan, T. M., Vukašinović, N., Liu, D., Russinova, E., and Yin, Y. (2020). Brassinosteroids: multidimensional regulators of plant growth, development, and stress responses. Plant Cell 32, 295–318. doi: 10.1105/tpc.19.00335
Panuccio, M., Chaabani, S., Roula, R., and Muscolo, A. (2018). Bio-priming mitigates detrimental effects of salinity on maize improving antioxidant defense and preserving photosynthetic efficiency. Plant Physiol. Biochem. 132, 465–474. doi: 10.1016/j.plaphy.2018.09.033
Parađiković, N., Vinković, T., Vrček, I. V., Žuntar, I., Bojić, M., and Medić-Šarić, M. (2011). Effect of natural biostimulants on yield and nutritional quality: an example of sweet yellow pepper (Capsicum annuum L.) plants. J. Sci. Food Agric. 91, 2146–2152. doi: 10.1002/jsfa.4431
Pehlivan, N. (2018). Salt stress relief potency of whortleberry extract biopriming in maize. 3 Biotech 8, 1–10. doi: 10.1007/s13205-018-1113-6
Peleg, Z., Apse, M. P., and Blumwald, E. (2011). Engineering salinity and water-stress tolerance in crop plants: getting closer to the field. Adv. Bot. Res. 57, 405–443. doi: 10.1016/b978-0-12-387692-8.00012-6
Plaut, Z., Edelstein, M., and Ben-Hur, M. (2013). Overcoming salinity barriers to crop production using traditional methods. Crit. Rev. Plant Sci. 32, 250–291. doi: 10.1080/07352689.2012.752236
Quan, R., Lin, H., Mendoza, I., Zhang, Y., Cao, W., Yang, Y., et al. (2007). SCABP8/CBL10, a putative calcium sensor, interacts with the protein kinase SOS2 to protect Arabidopsis shoots from salt stress. Plant Cell 19, 1415–1431. doi: 10.1105/tpc.106.042291
Rady, M. M., and Mohamed, G. F. (2015). Modulation of salt stress effects on the growth, physio-chemical attributes and yields of Phaseolus vulgaris L. plants by the combined application of salicylic acid and Moringa oleifera leaf extract. Sci. Hortic. 193, 105–113. doi: 10.1016/j.scienta.2015.07.003
Rady, M. M., Mohamed, G. F., Abdalla, A., and Ahmed, Y. H. (2015). Integrated application of salicylic acid and Moringa oleifera leaf extract alleviates the salt-induced adverse effects in common bean plants. Int. J. Agric. Technol. 11, 1595–1614.
Rady, M. M., Talaat, N. B., Abdelhamid, M. T., Shawky, B. T., and Desoky, E.-S. M. (2019b). Maize (Zea mays L.) grains extract mitigates the deleterious effects of salt stress on common bean (Phaseolus vulgaris L.) growth and physiology. J. Hortic. Sci. Biotechnol. 94, 777–789. doi: 10.1080/14620316.2019.1626773
Rady, M. M, Desoky, E.-S., Elrys, A., and Boghdady, M. (2019a). Can licorice root extract be used as an effective natural biostimulant for salt-stressed common bean plants? S. Afr. J. Bot. 121, 294–305. doi: 10.1016/j.sajb.2018.11.019
Rady, M. M., Varma, B., and Howladar, S. M. (2013). Common bean (Phaseolus vulgaris L.) seedlings overcome NaCl stress as a result of presoaking in Moringa oleifera leaf extract. Sci. Hortic. 162, 63–70. doi: 10.1016/j.scienta.2013.07.046
Ramadoss, D., Lakkineni, V. K., Bose, P., Ali, S., and Annapurna, K. (2013). Mitigation of salt stress in wheat seedlings by halotolerant bacteria isolated from saline habitats. SpringerPlus 2, 1–7. doi: 10.1186/2193-1801-2-6
Rao, A. Q., Ud, S., Akhtar, S., Sarwar, M. B., Ahmed, M., Rashid, B., et al. (2016). Genomics of salinity tolerance in plants. Plant Genomics 2016, 273–299. doi: 10.1007/978-3-7643-8554-5_25
Rodrıguez, P., Torrecillas, A., Morales, M., Ortuno, M., and Sánchez-Blanco, M. (2005). Effects of NaCl salinity and water stress on growth and leaf water relations of Asteriscus maritimus plants. Environ. Exp. Bot. 53, 113–123. doi: 10.1016/j.envexpbot.2004.03.005
Romero-Aranda, R., Moya, J., Tadeo, F., Legaz, F., and Primo-Millo, E. (1998). 623 Talon M (1998) Physiological and anatomical disturbances 624 induced by chloride salts in sensitive and tolerant citrus: 625 beneficial and detrimental effects of cations. Plant Cell Environ. 626, 1243–1253. doi: 10.1046/j.1365-3040.1998.00349.x
Rouphael, Y., and Colla, G. (2020). Biostimulants in agriculture. Front. Plant Sci. 11:40. doi: 10.3389/fpls.2020.00040
Sahab, S., Suhani, I., Srivastava, V., Chauhan, P. S., Singh, R. P., and Prasad, V. (2020). Potential risk assessment of soil salinity to agroecosystem sustainability: current status and management strategies. Sci. Total Environ. 764:144164. doi: 10.1016/j.scitotenv.2020.144164
Salama, Z. A., El Fouly, M. M., and Gaafar, A. A. (2013). Mitigation of the adverse effect of salinity through stimulation some secondary metabolites and antioxidant enzymes of methanolic extract of maize cultivars by exogenous ascorbic acid. J. Food Agric. Environ. 11, 1328–1335.
Sánchez-Gómez, R., Garde-Cerdán, T., Zalacain, A., Garcia, R., Cabrita, M., and Salinas, M. (2016). Vine-shoot waste aqueous extract applied as foliar fertilizer to grapevines: effect on amino acids and fermentative volatile content. Food Chem. 197, 132–140. doi: 10.1016/j.foodchem.2015.10.034
Semida, W. M., and Rady, M. M. (2014). Presoaking application of propolis and maize grain extracts alleviates salinity stress in common bean (Phaseolus vulgaris L.). Sci. Hortic. 168, 210–217. doi: 10.1016/j.scienta.2014.01.042
Shukla, P. S., Mantin, E. G., Adil, M., Bajpai, S., Critchley, A. T., and Prithiviraj, B. (2019). Ascophyllum nodosum-based biostimulants: sustainable applications in agriculture for the stimulation of plant growth, stress tolerance, and disease management. Front. Plant Sci. 10:655. doi: 10.3389/fpls.2019.00655
Sottosanto, J. B., Saranga, Y., and Blumwald, E. (2007). Impact of AtNHX1, a vacuolar Na+/H+ antiporter, upon gene expression during short-and long-term salt stress in Arabidopsis thaliana. BMC Plant Biol. 7:18. doi: 10.1186/1471-2229-7-18
Sun, L.-J., Zhou, J.-J., Pan, J.-L., Liang, Y.-Y., Fang, Z.-J., Xie, Y., et al. (2018). Electrochemical mapping of indole-3-acetic acid and salicylic acid in whole pea seedlings under normal conditions and salinity. Sens. Actuators B Chem. 276, 545–551. doi: 10.1016/j.snb.2018.08.152
Suryaman, M., Sunarya, Y., Istarimila, I., and Fudholi, A. (2021). Effect of salinity stress on the growth and yield of mungbean (Vigna radiata (L.) R. Wilczek) treated with mangosteen pericarp extract. Biocatal. Agric. Biotechnol. 36:102132. doi: 10.1016/j.bcab.2021.102132
Taha, R. (2016). Improving salt tolerance of Helianthus annuus (L.) plants by Moringa oleifera leaf extract. Egypt. J. Agron. 38, 117–140. doi: 10.21608/agro.2016.301
Tang, X., Mu, X., Shao, H., Wang, H., and Brestic, M. (2015). Global plant-responding mechanisms to salt stress: physiological and molecular levels and implications in biotechnology. Crit. Rev. Biotechnol. 35, 425–437. doi: 10.3109/07388551.2014.889080
Tesfay, S. Z., and Magwaza, L. S. (2017). Evaluating the efficacy of moringa leaf extract, chitosan and carboxymethyl cellulose as edible coatings for enhancing quality and extending postharvest life of avocado (Persea americana Mill.) fruit. Food Packag. Shelf Life 11, 40–48. doi: 10.1016/j.fpsl.2016.12.001
Ur Rehman, H. U., Basra, S., Rady, M. M., Ghoneim, A. M., and Wang, Q. (2017). Moringa leaf extract improves wheat growth and productivity by affecting senescence and source-sink relationship. Int. J. Agric. Biol. 19, 479–484. doi: 10.17957/ijab/15.0316
ur Rehman, H., Alharby, H. F., Bamagoos, A. A., Abdelhamid, M. T., and Rady, M. M. (2021). Sequenced application of glutathione as an antioxidant with an organic biostimulant improves physiological and metabolic adaptation to salinity in wheat. Plant Physiol. Biochem. 158, 43–52. doi: 10.1016/j.plaphy.2020.11.041
Velmurugan, A., Swarnam, P., Subramani, T., Meena, B., and Kaledhonkar, M. (2020). “Water demand and salinity,” in Desalination - Challenges and Opportunities. eds M. H. D. A. Farahani, V. Vatanpour, and A. H. Taheri (London: IntechOpen). doi: 10.5772/intechopen.88095
Yakhin, O. I., Lubyanov, A. A., Yakhin, I. A., and Brown, P. H. (2017). Biostimulants in plant science: a global perspective. Front. Plant Sci. 7:2049. doi: 10.3389/fpls.2016.02049
Younis, A., Akhtar, M. S., Riaz, A., Zulfiqar, F., Qasim, M., Farooq, A., et al. (2018). Improved cut flower and corm production by exogenous moringa leaf extract application on gladiolus cultivars. Acta Sci. Pol. Hortorum Cultus 17, 25–38. doi: 10.24326/asphc.2018.4.3
Yousefirad, S., Soltanloo, H., Ramezanpour, S. S., Zaynali Nezhad, K., and Shariati, V. (2020). The RNA-seq transcriptomic analysis reveals genes mediating salt tolerance through rapid triggering of ion transporters in a mutant barley. PLoS One 15:e0229513. doi: 10.1371/journal.pone.0229513
Zörb, C., Geilfus, C. M., and Dietz, K. J. (2019). Salinity and crop yield. Plant Biol. 21, 31–38. doi: 10.1111/plb.12884
Zou, J., Liu, A., Chen, X., Zhou, X., Gao, G., Wang, W., et al. (2009). Expression analysis of nine rice heat shock protein genes under abiotic stresses and ABA treatment. J. Plant Physiol. 166, 851–861. doi: 10.1016/j.jplph.2008.11.007
Zulfiqar, F., Casadesús, A., Brockman, H., and Munné-Bosch, S. (2020b). An overview of plant-based natural biostimulants for sustainable horticulture with a particular focus on moringa leaf extracts. Plant Sci. 295:110194. doi: 10.1016/j.plantsci.2019.110194
Zulfiqar, F., Younis, A., Finnegan, P. M., and Ferrante, A. (2020c). Comparison of soaking corms with moringa leaf extract alone or in combination with synthetic plant growth regulators on the growth, physiology and vase life of sword lily. Plants 9:1590. doi: 10.3390/plants9111590
Zulfiqar, F., Akram, N. A., and Ashraf, M. (2020a). Osmoprotection in plants under abiotic stresses: new insights into a classical phenomenon. Planta 251:3. doi: 10.1007/s00425-019-03293-1
Zulfiqar, F., and Ashraf, M. (2021a). Bioregulators: unlocking their potential role in regulation of the plant oxidative defense system. Plant Mol. Biol. 105, 11–41. doi: 10.1007/s11103-020-01077-w
Keywords: salt stress, stress perception, signaling signatures, NaCl, bioactive compounds, climate change, antioxidants, osmoprotectants
Citation: Ahmad A, Blasco B and Martos V (2022) Combating Salinity Through Natural Plant Extracts Based Biostimulants: A Review. Front. Plant Sci. 13:862034. doi: 10.3389/fpls.2022.862034
Received: 25 January 2022; Accepted: 02 May 2022;
Published: 20 May 2022.
Edited by:
Rogelio Santiago, University of Vigo, SpainReviewed by:
Mohammad Anwar Hossain, Bangladesh Agricultural University, BangladeshFaisal Zulfiqar, Islamia University of Bahawalpur, Pakistan
Copyright © 2022 Ahmad, Blasco and Martos. This is an open-access article distributed under the terms of the Creative Commons Attribution License (CC BY). The use, distribution or reproduction in other forums is permitted, provided the original author(s) and the copyright owner(s) are credited and that the original publication in this journal is cited, in accordance with accepted academic practice. No use, distribution or reproduction is permitted which does not comply with these terms.
*Correspondence: Vanessa Martos, dmFuZUB1Z3IuZXM=
†These authors have contributed equally to this work and share last authorship