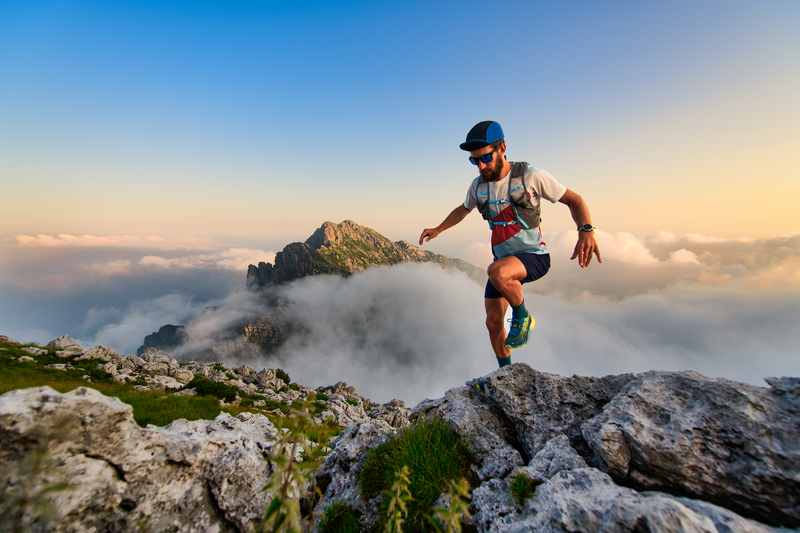
95% of researchers rate our articles as excellent or good
Learn more about the work of our research integrity team to safeguard the quality of each article we publish.
Find out more
REVIEW article
Front. Plant Sci. , 05 April 2022
Sec. Plant Physiology
Volume 13 - 2022 | https://doi.org/10.3389/fpls.2022.851631
This article is part of the Research Topic Signaling and Crosstalk of Melatonin, Nitric Oxide and Hydrogen Sulfide in Plants View all 6 articles
In plants senescence is the final stage of plant growth and development that ultimately leads to death. Plants experience age-related as well as stress-induced developmental ageing. Senescence involves significant changes at the transcriptional, post-translational and metabolomic levels. Furthermore, phytohormones also play a critical role in the programmed senescence of plants. Nitric oxide (NO) is a gaseous signalling molecule that regulates a plethora of physiological processes in plants. Its role in the control of ageing and senescence has just started to be elucidated. Here, we review the role of NO in the regulation of programmed cell death, seed ageing, fruit ripening and senescence. We also discuss the role of NO in the modulation of phytohormones during senescence and the significance of NO-ROS cross-talk during programmed cell death and senescence.
In plants, senescence is a ubiquitous and unavoidable process of ageing that ultimately leads to death, just like all other living organisms. Plants experience both age-related and stress-induced developmental ageing. As Schippers et al. (2015), describe it as “living to die and dying to live,” the process of senescence is extremely important for the survival of plants. Technically, senescence is the programmed, coordinated breakdown of leaf cells under strict genetic control, as part of the overall development of plants, to remobilize essential nutrients, ensure reproductive success and provide the necessary phenotypic plasticity for adapting to the changing climate (Schippers et al., 2015). This is also why senescence is thought to be a form of programmed cell death (PCD). Senescence is associated with a gradual loss of the green colour as chloroplasts are the first cellular organelles to be broken down (Dodge, 1970). Selective chloroplast breakdown helps salvage a major portion of essential macromolecules such as proteins and lipids for recycling during the final stages (Ischebeck et al., 2006) and provides up to 80% of the final nitrogen (N) content of grains (Girondé et al., 2015). However, it is important to mention that a certain fraction of plastids may be turned into chloroplasts by degrading the photosynthetic machinery.
Nitric oxide (NO) is a gaseous signalling molecule with key roles in several physiological processes involved in plant growth and development. NO has established roles in seed germination (Sirova et al., 2011; Deng and Song, 2012; Piterkova et al., 2012; Arc et al., 2013a,b; Albertos et al., 2015), plant growth (Kopyra and Gwozdz, 2004), phytohormone perception and homeostasis (Lopez-Molina et al., 2002; Huang et al., 2004; Terrile et al., 2012; Mur et al., 2013; Lang and Zuo, 2015), flower formation, reproduction and seed setting (Kwon et al., 2012), fruit ripening (Manjunatha et al., 2012; Corpas and Palma, 2018), plant defence against infection (Kawakita et al., 2004, 2010; Malik et al., 2011; Kawakita, 2014; Yun et al., 2016; Khan et al., 2019) and several abiotic stresses (Mun et al., 2017; Khan et al., 2019; Nabi et al., 2019). The free radical NO reacts with various extracellular/intracellular macromolecules forming a series of other molecules including peroxynitrite (ONOO−), nitrosonium ions (NO+), NO radicals (NO), higher nitrogen oxides (NOx), iron dinitrosyl complexes, and S-nitrosothiols (SNOs) among others. These molecules are collectively called reactive nitrogen species (RNS; Di Stasi et al., 2002). The process of S-nitrosylation, the covalent binding of an NO moiety to a reactive cysteine thiol to form an SNO, is the post-translational modification (PTM) of proteins that has emerged as a key redox-based PTM of proteins in plants. An armada of proteins involved in key physiological processes has been shown to be targets of S-nitrosylation in plants and animals. The direct role of NO in plant senescence has also been briefly described in a few studies (Corpas and Palma, 2018; Bruand and Meilhoc, 2019) such as leaf (De Michele et al., 2009), cotyledon (Du et al., 2013) and stress-induces senescence (Terrón-Camero et al., 2019). In plants, NO is produced through several different reductive and oxidative, enzymatic and non-enzymatic pathways (Hussain et al., 2022). Several studies hint towards NO production in the chloroplasts and mitochondria (Galatro et al., 2013; Vanlerberghe, 2013) which are the first cellular organelles to be dismantled during senescence (reviewed by León and Costa-Broseta, 2020). In animal systems, NO has a well-established regulatory role in apoptosis and cell death (Kim et al., 1999, 2001; Brüne, 2003; Snyder et al., 2009), a process analogous to senescence in plants. Interestingly, NO acts as a signalling molecule or a toxic molecule depending upon the situation.
In plants, programmed cell death is an important process regulating several aspects of plant growth and development. Besides, it is a natural mechanism in place to execute infected cells during plant responses to biotic and abiotic stresses. PCD is mediated by NO and reactive oxygen species (ROS; Wang et al., 2013b; Figure 1A). Plants rapidly-produce NO and various ROS in response to biotic and/or abiotic stresses leading to the intrinsic execution of the target cells. Interestingly, these small redox-active molecules can trigger PCD either synergistically or independently. In plants, a well-known form of PCD is the hypersensitive response (HR) characterized by the rapid execution of the infected and surrounding cells. Some HR characteristics in plants are similar to apoptosis in animal cells such as DNA cleavage, chromatin condensation, cytoplasmic vacuolization and membrane dysfunction (Greenberg and Yao, 2004; Choi et al., 2013; Iakimova et al., 2013), yet there are key differences between the two processes. For example, plant HR is usually accompanied by necrosis of infected and surrounding plant cells, a key mechanism for containing the pathogen within the necrotic cells and restricting its further spread to the healthy tissues. Furthermore, recent literature indicates that certain types of plant HR appears similar to the iron dependent cell death known as ferroptosis; which is also characterized by the accumulation of lipid peroxides (Yang and Stockwell, 2016). Recent experiments by (Dangol et al., 2019) indicate that iron- and ROS-dependent signalling cascades converge to regulate ferroptosis and HR in rice after avirulent Magnaporthe oryzae infection suggesting that HR and ferroptosis may share the same molecular machinery under certain conditions. In animal system, the oxidoreductase (cytochrome P450 oxidoreductase) contributes to phospholipid peroxidation during ferroptosis (Zou et al., 2021). Yan et al. (2021) showed that coupling of cytochrome P450 oxidoreductase and cytochrome B5 reductase 1 (CYB5R1) mediates the production of H2O2, which subsequently drives lipid peroxidation and ferroptosis through the iron-catalysed Fenton reaction. Although significant cross-talk exists between NO and ROS, both are implicated in HR. However, it is clear that a balance between intracellular levels of NO and ROS is a critical determinant for HR (Delledonne et al., 2001). Pathogen attack is followed by a rapid burst of NO and a “biphasic” production of ROS at the site of infection (Romero-Puertas et al., 2004) indicating the NO and ROS act synergistically or at least in combination to promote HR. However, NO and ROS can independently release cytochrome c from mitochondria, affecting the caspase-like signalling pathway that leads to HR (Mur et al., 2006). The mitogen-activated protein kinases (MAPKs) and phosphatases are key components of plant defence that are affected by NO and ROS subsequently affecting the establishment of HR. These are examples of cases where NO and ROS appear to converge promoting HR in response to infection. As described above, a major route for NO bioactivity is via the canonical PTM of proteins known as S-nitrosylation, several key proteins involved in HR are known to be S-nitrosated by NO or affected by the NO-ROS cross talk. For example, ROS signalling in plants is mediated by GAPDH is a direct target of NO-mediated S-nitrosylation that blunts its activity (Lindermayr et al., 2005). Furthermore, Peroxiredoxin-2E (PrxIIE) and Methionine adenosyl transferase (MAT) are other examples of key proteins involved in HR that are affected by ROS and NO-mediated S-nitrosylation (Dietz, 2003; Horling et al., 2003; Romero-Puertas et al., 2007a,b). However, NO is also known to scavenge H2O2 under certain circumstances thereby protecting plant cells from ROS-mediated damage (Beligni et al., 2002; Guo and Crawford, 2005). In plants, the enzyme S-nitrosoglutathione reductase 1 (GSNOR 1) regulates the global levels of S-nitrosated proteins (Hussain et al., 2019). NO S-Nitrosylates NADPH oxidase Respiratory burst oxidase homolog D (AtRBOHD) at Cys890 which blunts its function in ROS synthesis (Yun et al., 2011; Figure 1). Several studies involving loss of function gsnor mutant plants indicate that GNSOR1 regulates SA signalling, thermotolerance and acts downstream of superoxide to regulate cell death (Feechan et al., 2005; Lee et al., 2008; Chen et al., 2009; Wang et al., 2010).
Figure 1. Role of nitric oxide in fruit ripening, programmed cell death and leaf senescence. (A) NO and ROS-mediated control of programmed cell death. Plants rapidly-produce NO and various ROS in response to biotic and/or abiotic stresses leading to intrinsic execution of the target cells. NO and ROS can trigger PCD either synergistically or independently. Both NO and ROS are implicated in the hypersensitive response (HR), characterized by the execution of the infected and surrounding cells, is a well-known form of PCD in plants. However, significant cross-talk exists between NO and ROS during the establishment of HR. The establishment of HR warrants a critical balance between intracellular levels of NO and ROS. Several key proteins such as non-expressor of pathogensi-related genes 1 (NPR1), TGA1 (transcriptional activators that specifically bind to 5′-TGACG-3′), glyceraldehyde-3-phosphate dehydrorgenase (GAPDH), respiratory burst oxidase homolog D (RBOHD) are involved in HR are known to be S-nitrosated by NO or affected by the NO-ROS cross talk. NO is also known to scavenge H2O2 under certain circumstances thereby protecting plant cells from ROS-mediated damage. (B) NO-mediated regulation of senescence, seed dormancy and fruit ripening. NO affects leaf senescence by modulating phytohormones such as ABA, ET, and JA. Though the role of NO in seed ageing is not well-defined, NO is known to break seed dormancy via stimulation of ET production. NO donors and arginine (Arg) abolishes seed dormancy and stimulates germination whereas, the application of NO scavengers (such as cPTIO, L-Name, and CAN) promotes seed dormancy. NO-mediated removal of seed dormancy and promotion of germination is associated with alternations of nitrated and biotinylated proteins, ABA, JA and protein nitration. NO has also been implicated in fruit ripening. As the fruit ripens, ROS and RNS anabolic vs. catabolic rates change significantly altering the overall nitro-oxidative environment at the cellular level ultimately affecting the NO-dependent S-Nitorsation and Tyrosine Nitration events in fruit. Furthermore, NO mediated binding and chelation, S-nitrosylation of methionine adenosyl transferase (MAT), stoichiometric reduction of ACC and transcriptional regulation of several genes involved in the ethylene pathway plays an important role in fruit ripening.
In plants, the Class II (CGFS-type) glutaredoxins (GRXs) act as FeS cluster transferases. On the other hand, the Class I (CxxC/S-type) GRXs act as oxidoreductases and participate in oxidative signalling. GRx and FeS binding involves active site cysteines and glutathione (GSH; Couturier et al., 2015; Trnka et al., 2020). In eukaryotic cells, the CGFS-type GRXs function in iron metabolism, i.e., the biogenesis of plastidal, mitochondrial, or bacterial FeS clusters (Mühlenhoff et al., 2003; Moseler et al., 2015) and iron trafficking to the cytosol. Since these FeS-transfering GRXs are redox-active, they are also affected by NO and ROS. An important hub through which plants regulate PCD is through the involvement of ROS and NO in iron homeostasis. Iron is an essential micronutrient for plants. Iron in the form of heme and Fe-sulfur (FeS) clusters is required for primary metabolic processes, such as respiration, cell proliferation and differentiation, DNA biosynthesis and repair, photosynthesis and biosynthesis of chlorophyll and hormones (Camprubi et al., 2017). Despite its importance as an essential micronutrient, iron overload can prove to be toxic due to its potent electro-chemistry. Iron reacts with ROS such as H2O2 via the Fenton reaction; a catalytic reaction through which iron converts H2O2 into biologically more toxic ROS including the hydroxide radical (HO; Winterbourn, 1995). These potent ROS result in oxidative damage of nucleic acids, lipids and proteins. In severe cases the damage manifests in the form of PCD. The production of hydroxyl radical has been reported via the Fenton reaction in submitochondrial particles under oxidative stress (Thomas et al., 2009). On the other hand, NO appears to up-regulated Fe uptake by the plants and increase its bioavailability for metabolic functions. The formation of NO-Fe complexes is considered as one of the strategies for storing and stabilizing free NO and Fe within the cells (Hsiao et al., 2019). NO also plays a key role in the modulation of ferritin; the cellular Fe store house. Ferritin can store about 2,000–4,500 Fe atoms. Loss of function ferritin triple mutant plants fer1,3,4 exhibit massive Fe accumulation (Roschzttardtz et al., 2013). As described above, physiological excess of Fe results in oxidative damage due to the formation of hydorxyl radical via the Fenton reaction. NO is required for Fe-induced ferritin synthesis. NO acts downstream of Fe in the induction of ferritin transcripts and is a key signalling molecule for the regulation of Fe homeostasis in plants (Murgia et al., 2002; Tewari et al., 2020).
As described above, senescence is thought to be just another form of plant PCD. It is the final stage of plant development that occurs under strict genetic control but is also triggered and affected by environmental conditions. Furthermore, phytohormones such as abscisic acid (ABA), Auxin (IAA), cytokinin (CK), ethylene (ET), jasmonic acid (JA) and salicylic acid (SA) also affect the process of senescence (Lim et al., 2007). In plants, senescence is characterized by a dramatic increase in the H2O2 content in leaf tissues which results in the oxidation of macromolecules, proteins and lipids and triggers the expression of genes involved in senescence (Cui et al., 2013) indicating a dual role of ROS during senescence. Balazadeh et al. in 2010 and 2011 reported rapid and strong induction of senescence-associated NAC genes (sen-NACs) by H2O2 in roots and leaves (Balazadeh et al., 2010, 2011). Similarly, the WRKY253 and WRKY53 genes have been described to regulate plant senescence in a redox-dependent manner (Kim et al., 2009, 2017; Doll et al., 2020). ROS and NO induce senescence of rudimentary leaves and the expression profiles of the related genes in Litchi (Litchi chinensis) plants (Yang et al., 2018). However, significant cross-talk exists between various RNS and ROS during senescence and PCD (Figure 1A). On the other hand, both pro-and anti- senescence roles have been described for NO, depending upon the situation such as its subcellular location and concentration. NO is known to scavenge H2O2 and alleviate ROS toxicity thereby delaying senescence (Sang et al., 2008). Loss of function mutant plants nos1/noa1 which are NO-deficient, exhibit early senescence (Niu and Guo, 2012). Furthermore, ectopic expression of the NO-degrading dioxygenase (NOD) enzyme in plants results in early senescence (Mishina et al., 2007). Plants lacking the plasma membrane-localized cyclic nucleotide-gated ion channel 2 (CNGC2) exhibit an early senescence phenotype. For example, the Arabidopsis thaliana CNGC2 loss of function dnd1 plants exhibits loss of chlorophyll, H2O2 production, tissue necrosis and lipid peroxidation with significantly lower levels of NO as compared to wild type (WT) plants (Ma et al., 2010) indicating a contrasting behaviour of ROS and NO during senescence. This protective role of NO against ROS-mediated senescence can be associated with the activity of the antioxidant machinery that negatively regulates chlorophyll break down and promotes the stability of the thylakoid membranes during senescence (Liu and Guo, 2013).
Case studies where NO plays a pro-senescence role have also been reported. The rice (Oryza sativa) nitric oxide excess 1 (NOE1) gene encodes a rice catalase enzyme (OsCATC). The rice NO-accrual mutant noe1 accumulates higher amounts of H2O2 in the leaves that consequently promotes NO production via the activation of the nitrate reductase (NR) enzyme. Removal of excess NO resulted in reduced cell death, indicating the NO acts as a mediator of H2O2-induced cell death in this case. In addition, further reduction of cellular SNO levels by the over-expression of rice GSNOR reduced cell death in noe1 rice plants. In another study, exogenous application of NO led to rapid production of H2O2 and induction of cell death in maize (Zea mays) leaves (Kong et al., 2013). These results indicate that NO and NO-mediated S-nitrosylation of proteins are important mediators of H2O2 mediated cell death and senescence (Lin et al., 2011; Wang et al., 2013a).
Phytohormones are chemical messengers that coordinate various physiological activities in plants. The various types of phytohormones include IAA, gibberellins (GAs), CK, ET, ABA, SA, JA, and brassinosteriods (BR). For plants, life is not possible without these messenger molecules as they support key physiological events throughout plant life. Significant progress has been made in understanding the underlying mechanisms of phytohormone perception, biosynthesis, transportation, signal transduction, and downstream effects. An interesting feature is that the signalling pathways of some phytohormones work in parallel (synergistic relationship) whereas, those of others intersect each other (antagonistic relationship). Normal plant physiology requires basal levels of all these phytohormones. However, under stress conditions, drastic changes in the phytohormonal pathways are a key feature of plant responses to these stresses.
NO-mediated control of phytohormones is well-known. NO affects leaf senescence by modulating the activity of phytohormones such as ABA, ET, and JA (Figure 1B). NO has been shown to frequently interact with phytohormones leading to remarkably complex signalling cascades. Accumulating evidence in the literature indicates the interaction of NO with virtually all major phytohormones affecting their biosynthesis, perception, metabolism, transport, and downstream effects (Freschi, 2013). Furthermore, the type and magnitude of the interaction may vary under different conditions. So far key roles for NO have been described in the regulation of ABA, ET, IAA, GAs, JA, SA and BR in several plant species both at the transcriptome (Hussain et al., 2016) as well as the proteome level. NO-mediated regulation of senescence has been reported in several studies. The exogenous application of NO donors extends the post-harvest life of fruits and vegetables and slows down flower maturation (Leshem and Haramaty, 1996). NO has also been reported to counteract leaf senescence caused by ABA and methyl jasmonate (MeJA) in rice (Hung and Kao, 2004). NO concentration increases to delay MeJA-induced leaf senescence in plants after treatment with a low concentration of SA (Ji et al., 2016). The role of CK in fruit maturation and senescence is extremely important. Application of exogenous CK simultaneously stimulates the expression of the nitrate reductase (NR) enzyme for the production of NO and delays senescence (Magalhaes et al., 2000; Tun et al., 2001; Planchet et al., 2006; Kolbert et al., 2019). The CK signalling pathway is mediated by a phosphorelay system that sequentially transfers phosphoryl groups from the CK receptors to histidine phosphotransfer proteins (AHPs) and response regulators (ARRs). Feng et al. (2013) described that the S-nitrosylation of AHP1 at Cys 115 represses its phosphorylation and the subsequent transfer of the phosphoryl group to ARR1. They also showed that a non-nitrosatable mutant AHP1 is insensitive to NO in repressing its phosphorylation relieving the inhibitory effect of NO. These findings illustrate NO-mediated control of CK signalling during plant growth and development. CK also interacts with ET which is another important phytohormone required for senescence. Wilhelmova et al. (2006) reported that tobacco plants with high or low levels of CK show perturbed levels of ET and NO during senescence as compared to plants with normal levels of CK. They also reported that NO and ET production was under the control of genes other than those regulating senescence. Although their results did not indicate a direct link between ET production and CK levels, they recorded low NO levels. Furthermore, they also recorded that low CK level was associated with increased NO production. From the above studies, the interaction of NO and CK appears to be dose-dependent. Application of NO donors, [N-tert-butyl-α-phenylnitrone (PBN), sodium nitroprusside (SNP), 3-morpholinosydonimine, and AsA + NaNO2] has been reported to inhibit the increase of H2O2 levels during ABA- and JA-induced senescence in rice (Hung and Kao, 2004). The NO deficient mutant nos1/noa1 exhibits early senescence. On the other hand, the ethylene insensitive 2 (EIN2) positively regulates ET-induced senescence. Niu and Guo (2012) characterized the ein2-1nos1/noa1 double mutant plants to determine the relationship of NO and ET during senescence. Their results indicated that the dark-induced early senescence phenotype of nos1/noa1 was suppressed by the EIN2 mutation via a reduction in chlorophyll degradation, improving thylakoid membrane stability and significant inhibition in the up-regulation of senescence-associated genes, suggesting that EIN2 is involved in NO signalling during senescence. On the other hand, NO deficiency accelerates chlorophyll degradation and stability of the thylakoid membrane during dark-induced leaf senescence (Liu and Guo, 2013).
Ageing can simply be defined as growing old. Ageing means going through all phases of growth and development from germination to growth, senescence and seed production. Plants go through both stress-induced and age-related developmental ageing. Seed performance is a major determinant of crop yield and a base for plant growth and development. The longevity of seeds varies among the different plant species. However, seed quality and vigour deteriorates with age and is affected by internal and external factors (Sano et al., 2015).
Seed germination performance is a major determinant of crop yield. Deterioration of seed quality with age is associated with the accumulation of DNA damage. Ageing in seeds is characterized by a loss of membrane stability at the cellular level, nucleic acid degradation, an increase in oxidative damage due to ROS accumulation and a concomitant reduction in the antioxidant potential (Bailly et al., 2008; El-Maarouf-Bouteau et al., 2011). Though the role of NO in seed ageing is not well-defined, NO is known to break seed dormancy via stimulation of ET production (Gniazdowska et al., 2007, 2010). Furthermore, the application of NO donors and arginine (Arg) to apple (Malus domestica) seeds abolishes the dormancy of the embryo and stimulates germination (Krasuska et al., 2016). On the other hand, the application of NO scavengers (cPTIO, L-Name, CAN) promotes seed dormancy. They also reported that NO-mediated removal of seed dormancy and promotion of germination is associated with alternations of nitrated and biotinylated proteins (Krasuska et al., 2016). This NO-mediated removal of apple seed dormancy has been linked to differential expression of genes associated with ABA, JA and RNA nitration (Andryka-Dudek et al., 2019). Several other studies have described the importance of NO for the transition of seeds from dormancy to germination (Bykova et al., 2015; Signorelli and Considine, 2018; Figure 1B). NO treatment is regularly used for seed stratification, accelerating germination and improvement of seed vigour. At the molecular level, NO is involved in S-nitrosylation and tyrosine nitration of different proteins as well as nitration of nucleic acids and fatty acids (Mata-Pérez et al., 2017; Arasimowicz-Jelonek and Floryszak-Wieczorek, 2019). NO enhances the desiccation tolerance of recalcitrant Antiaris toxicaria seeds via S-nitrosylation (Bai et al., 2011) and reduces the accumulation of H2O2 (Bai et al., 2011). NO has been reported to act as an H2O2 scavenger in germinating wheat (Triticum aestivum) seeds (Cakmak et al., 1993). NO delays ageing of soybean (Glycine max) cotyledons via chlorophyll stabilization (Jasid et al., 2006).
As described, deterioration in seed vigour and low germination are the characteristics of seed ageing. Controlled deterioration treatments (CDT) are often used by researchers under laboratory conditions to investigate the effects of ageing on seeds. CDTs often involve gradual changes in storage temperature and humidity conditions. In 2018, He et al. (2018) investigated the effects of CDT-induced ageing on elm seeds and reported a significant reduction in seed vigour with a concomitant reduction in cellular NO levels. However, treatment of the seeds with the NO donor SNP before CDT treatment promoted the overall vigour of the seeds with a significant increase in their germination. Using the biotin switch method, they identified 82 putative S-nitrosated proteins involved in carbohydrate metabolism. In addition, they also reported 163 metabolites that responded to both NO and the CDT. Similarly, Mao et al. (2018) reported NO-mediated regulation of seedling growth and mitochondrial responses in aged oat seeds. They reported a significant increase in H2O2 accumulation and loss of vigour in artificially aged oat seeds. However, SNP treatment of aged seeds protected seeds from H2O2-meidated damage and improved seed vigour via enhancement of ROS scavenging in the mitochondria via upregulation of catalase (CAT), dehydroascorbate reductase (DHAR), glutathione reductase (GR), monodehydroascorbate reductase (MDHAR), activity.
The development of different plant parts such as fruits can be broadly divided into three major stages in sequence; growth, maturation, and senescence. The growth period can be characterized by cell division and enlargement, which accounts for the increase in the overall biomass or size of the fruits. The maturation stage is reached just before the end of growth and involves the development of colour, odour and taste or flavour. Senescence starts when the overall magnitude of degradative processes is more than the biosynthetic processes. Fruit ripening and senescence involve highly coordinated complex biochemical changes at the cellular level involving transcriptional changes in ripening-associated genes (RAGs), transcription promoters and repressors, enzymes and metabolic rewiring (Fuentes et al., 2019). In general, climacteric fruits ripen after harvest whereas, non-climacteric fruits do not ripen after harvest. Bananas (Musa spp.) left on a kitchen table will ripen because they are climacteric and continue to produce ET even after being harvested. However, strawberries (Fragaria ananassa) do not ripen after harvest as they do not produce ET after being harvested.
Though ET plays a key role in fruit ripening, NO has also been implicated in fruit ripening and its role in regulating the ripening associated genetic and metabolic networks has just started to be unravelled. NO has emerged as a promising molecule for extending the post-harvest life of fruits without affecting the quality traits such as colour, texture, aroma and taste. In this regard, the initial works of Leshem’s group (Leshem et al., 1998, 2001; Leshem and Pinchasov, 2000) are of particular importance. Since then, NO has emerged as an important RNS involved in the maintenance of agronomic traits in fruits and the regulation of physiological processes at the molecular level. As the fruit ripens, ROS and RNS anabolic vs. catabolic rates change significantly altering the overall nitro-oxidative environment at the cellular level ultimately affecting the NO-dependent S-nitrosylation and tyrosine nitration events in fruit. At the transcriptional level NO regulates the expression of RAGs and those involved in fruit antioxidant defence, whereas at the post-translational level, antioxidant enzymes have been identified as targets of both protein nitration and S-nitrosylation in fruits (Chaki et al., 2015; Corpas and Palma, 2018; Gramegna et al., 2019). In addition, NO is also involved in metabolic rewiring to regulate fruit ripening (Gramegna et al., 2019; Zuccarelli et al., 2020).
Several studies have reported the negative effects of NO on ET biosynthesis in fruit thereby regulating fruit ripening (Manjunatha et al., 2012) in both climacteric fruits such as banana (Cheng et al., 2009), tomato (Solanum lycopersicum; Eum et al., 2009), apple (Rudell and Mattheis, 2006) and pepper (Capsicum annum; Chaki et al., 2015) as well as non-climacteric fruits such as strawberries (Zhu and Zhou, 2007). NO affects ET-induced ripening via transcriptional regulation of 1-carboxylic acid oxidase (ACO) genes ACO1, ACOH2 and ACO4; S-nitrosylation of methionine adenosyl transferase (MAT) and stoichiometric reduction of ACC (a precursor of ethylene) into 1-malonyl aminocyclopropane-1-carboxylic acid (MACC). NO also inhibits ET-induced fruit ripening via binding and chelation to form an ACC-ACC oxidase–NO (Figure 1B). Furthermore, there are several unidentified and proposed models for NO-mediated suppression of ET-induced fruit ripening.
Functional genomic studies of the tomato shr mutant plants overproducing NO indicated that higher concentrations of NO shift metabolic profiles and suppresses fruit growth and ripening (Bodanapu et al., 2016). Zuccarelli et al. (2020) showed that pre-climacteric exogenous application of NO in tomato delays ripening. Through further transcriptomic analysis, they showed that approximately one-third of the fruit transcriptome was changed as a result of NO exposure including the down-regulation of RAGs thereby restricting the production of ET and the ET sensitivity of the fruit tissues. In addition, they also recorded NO-mediated suppression of H2O2 scavenging enzymes resulting in nitro-oxidative stress and S-nitrosylation throughout the ripening process. Metabolomic analysis indicated alterations in carotenoid, tocopherol, flavonoid and ascorbate levels in the fruits following exposure to NO that ultimately affected lycopene production. However, NO had little effect on food quality parameters such as taste and aroma indicating the importance of NO from an agronomic point of view.
NO plays a key role in plant biology. It regulates various physiological, biochemical and molecular aspects of senescence in plants. In fruits, NO has long been described as an anti-senescence molecule as its application delays ripening and senescence and extends the post-harvest shelf life of fruits and vegetables. NO deficient mutant plants show early or enhanced senescence phenotypes. NO counteracts leaf senescence by suppressing chlorophyll degradation and promoting thylakoid membrane stability. However, NO acts as a Pro-senescence as well as an anti-senescence agent under different circumstances. Furthermore, the production of different RNS and ROS is a routine requirement for the maintenance of normal plant physiology. During incompatible plant pathogen interactions, the plant leucin-rich repeat (LRR) receptors recognize pathogen effector molecules which subsequently induces rapid HR and cell death. Plant NO is a well-recognized player in such plant pathogen interactions. A wealth of knowledge exists indicating significant crosstalk exists between RNS and ROS although this relationship remains elusive during programmed cell death. NO and/or ROS dependent and glutathione-dependent antioxidant defence and the iron-dependent (ferroptosis) cell death are hallmarks of the plant immune system. NO appears to play critical role in regulating these processes though the exact molecular mechanisms are still being investigated. ROS burst, increase of intracellular iron and α-glutamylcysteine synthetase are considered as key markers for ferroptotic cell death in plants (Hiruma et al., 2013). NO interacts with various ROS, haem-containing proteins and glutathione within the cell. These interactions represent different avenues for NO-mediated control of cell death in plants. The role of NO in regulating programmed cell death, seed ageing, fruit ripening and overall plant senescence has just begun to be unravelled and a complete list of signalling pathways regulated by NO is still being developed and several key questions remain to be answered. What are the molecular mechanisms responsible for deciding Pro- or anti-senescence roles of NO? How NO and ROS cross talk regulates senescence and PCD? What is the role of NO in regulating PCD during incompatible plant pathogen interactions? What are the effects of NO on various phytohormones during senescence? Thus, further work is required to understand how this key molecule triggers the onset of senescence in plants.
AH: compiled the data, wrote the manuscript and made the figure. FS and FA: collected data and research papers. B-WY: wrote and reviewed the manuscript. All authors contributed to the article and approved the submitted version.
This work was supported by Basic Science Research Program through the National Research Foundation of Korea (NRF) funded by the Ministry of Education (Grant number 2020R1I1A3073247), Republic of Korea, and a project to train professional personnel in biological materials by the Ministry of Environment.
The authors declare that the research was conducted in the absence of any commercial or financial relationships that could be construed as a potential conflict of interest.
All claims expressed in this article are solely those of the authors and do not necessarily represent those of their affiliated organizations, or those of the publisher, the editors and the reviewers. Any product that may be evaluated in this article, or claim that may be made by its manufacturer, is not guaranteed or endorsed by the publisher.
Albertos, P., Romero-Puertas, M. C., Tatematsu, K., Mateos, I., Sanchez-Vicente, I., Nambara, E., et al. (2015). S-nitrosylation triggers ABI5 degradation to promote seed germination and seedling growth. Nat. Commun. 6, 1–10. doi: 10.1038/ncomms9669
Andryka-Dudek, P., Ciacka, K., Wiśniewska, A., Bogatek, R., and Gniazdowska, A. (2019). Nitric oxide-induced dormancy removal of apple embryos is linked to alterations in expression of genes encoding ABA and JA biosynthetic or transduction pathways and RNA nitration. Int. J. Mol. Sci. 20:1007. doi: 10.3390/ijms20051007
Arasimowicz-Jelonek, M., and Floryszak-Wieczorek, J. (2019). A physiological perspective on targets of nitration in NO-based signaling networks in plants. J. Exp. Bot. 70, 4379–4389. doi: 10.1093/jxb/erz300
Arc, E., Galland, M., Godin, B., Cueff, G., and Rajjou, L. (2013a). Nitric oxide implication in the control of seed dormancy and germination. Front. Plant Sci. 4:346. doi: 10.3389/fpls.2013.00346
Arc, E., Sechet, J., Corbineau, F., Rajjou, L., and Marion-Poll, A. (2013b). ABA crosstalk with ethylene and nitric oxide in seed dormancy and germination. Front. Plant Sci. 4:63. doi: 10.3389/fpls.2013.00063
Bai, X. G., Yang, L. M., Tian, M. H., Chen, J. H., Shi, J. S., Yang, Y. P., et al. (2011). Nitric oxide enhances desiccation tolerance of recalcitrant Antiaris toxicaria seeds via protein S-nitrosylation and carbonylation. PLoS One 6:e20714. doi: 10.1371/journal.pone.0020714
Bailly, C., El-Maarouf-Bouteau, H., and Corbineau, F. (2008). From intracellular signaling networks to cell death: the dual role of reactive oxygen species in seed physiology. C. R. Biol. 331, 806–814. doi: 10.1016/j.crvi.2008.07.022
Balazadeh, S., Kwasniewski, M., Caldana, C., Mehrnia, M., Zanor, M. I., Xue, G.-P., et al. (2011). ORS1, an H₂O₂-responsive NAC transcription factor, controls senescence in Arabidopsis thaliana. Mol. Plant 4, 346–360. doi: 10.1093/mp/ssq080
Balazadeh, S., Siddiqui, H., Allu, A. D., Matallana-Ramirez, L. P., Caldana, C., Mehrnia, M., et al. (2010). A gene regulatory network controlled by the NAC transcription factor ANAC092/AtNAC2/ORE1 during salt-promoted senescence. Plant J. 62, 250–264. doi: 10.1111/j.1365-313X.2010.04151.x
Beligni, M. V., Fath, A., Bethke, P. C., Lamattina, L., and Jones, R. L. (2002). Nitric oxide acts as an antioxidant and delays programmed cell death in barley aleurone layers. Plant Physiol. 129, 1642–1650. doi: 10.1104/pp.002337
Bodanapu, R., Gupta, S. K., Basha, P. O., Sakthivel, K., Sadhana, Sreelakshmi, Y., et al. (2016). Nitric oxide overproduction in tomato SHR mutant shifts metabolic profiles and suppresses fruit growth and ripening. Front. Plant Sci. 7:1714. doi: 10.3389/fpls.2016.01714
Bruand, C., and Meilhoc, E. (2019). Nitric oxide in plants: pro- or anti-senescence. J. Exp. Bot. 70, 4419–4427. doi: 10.1093/jxb/erz117
Brüne, B. (2003). Nitric oxide: NO apoptosis or turning it ON? Cell Death Differ. 10, 864–869. doi: 10.1038/sj.cdd.4401261
Bykova, N. V., Hu, J., Ma, Z., and Igamberdiev, A. U. (2015). “The role of reactive oxygen and nitrogen species in bioenergetics, metabolism, and signaling during seed germination,” in Reactive Oxygen and Nitrogen Species Signaling and Communication in Plants. eds. K. J. Gupta and A. U. Igamberdiev (Cham: Springer International Publishing), 177–195.
Cakmak, I., Strbac, D., and Marschner, H. (1993). Activities of hydrogen peroxide-scavenging enzymes in germinating wheat seeds. J. Exp. Bot. 44, 127–132. doi: 10.1093/jxb/44.1.127
Camprubi, E., Jordan, S. F., Vasiliadou, R., and Lane, N. (2017). Iron catalysis at the origin of life. IUBMB Life 69, 373–381. doi: 10.1002/iub.1632
Chaki, M., Álvarez De Morales, P., Ruiz, C., Begara-Morales, J. C., Barroso, J. B., Corpas, F. J., et al. (2015). Ripening of pepper (Capsicum annuum) fruit is characterized by an enhancement of protein tyrosine nitration. Ann. Bot. 116, 637–647. doi: 10.1093/aob/mcv016
Chen, R. Q., Sun, S. L., Wang, C., Li, Y. S., Liang, Y., An, F. Y., et al. (2009). The Arabidopsis PARAQUAT RESISTANT2 gene encodes an S-nitrosoglutathione reductase that is a key regulator of cell death. Cell Res. 19, 1377–1387. doi: 10.1038/cr.2009.117
Cheng, G., Yang, E., Lu, W., Jia, Y., Jiang, Y., and Duan, X. (2009). Effect of nitric oxide on ethylene synthesis and softening of banana fruit slice during ripening. J. Agric. Food Chem. 57, 5799–5804. doi: 10.1021/jf901173n
Choi, H. W., Kim, N. H., Lee, Y. K., and Hwang, B. K. (2013). The pepper extracellular xyloglucan-specific endo-β-1,4-glucanase inhibitor protein gene, CaXEGIP1, is required for plant cell death and defense responses. Plant Physiol. 161, 384–396. doi: 10.1104/pp.112.203828
Corpas, F. J., and Palma, J. M. (2018). Nitric oxide on/off in fruit ripening. Plant Biol. 20, 805–807. doi: 10.1111/plb.12852
Couturier, J., Przybyla-Toscano, J., Roret, T., Didierjean, C., and Rouhier, N. (2015). The roles of glutaredoxins ligating Fe–S clusters: sensing, transfer or repair functions? Biochim. Biophys. Acta, Mol. Cell Res. 1853, 1513–1527. doi: 10.1016/j.bbamcr.2014.09.018
Cui, M. H., Ok, S. H., Yoo, K. S., Jung, K. W., Yoo, S. D., and Shin, J. S. (2013). An Arabidopsis cell growth defect factor-related protein, CRS, promotes plant senescence by increasing the production of hydrogen peroxide. Plant Cell Physiol. 54, 155–167. doi: 10.1093/pcp/pcs161
Dangol, S., Chen, Y., Hwang, B. K., and Jwa, N.-S. (2019). Iron- and reactive oxygen species-dependent ferroptotic cell death in Rice-Magnaporthe oryzae interactions. Plant Cell 31, 189–209. doi: 10.1105/tpc.18.00535
De Michele, R., Formentin, E., Todesco, M., Toppo, S., Carimi, F., Zottini, M., et al. (2009). Transcriptome analysis of Medicago truncatula leaf senescence: similarities and differences in metabolic and transcriptional regulations as compared with Arabidopsis, nodule senescence and nitric oxide signalling. New Phytol. 181, 563–575. doi: 10.1111/j.1469-8137.2008.02684.x
Delledonne, M., Zeier, J., Marocco, A., and Lamb, C. (2001). Signal interactions between nitric oxide and reactive oxygen intermediates in the plant hypersensitive disease resistance response. Proc. Natl. Acad. Sci. U. S. A. 98, 13454–13459. doi: 10.1073/pnas.231178298
Deng, Z., and Song, S. (2012). Sodium nitroprusside, ferricyanide, nitrite and nitrate decrease the thermo-dormancy of lettuce seed germination in a nitric oxide-dependent manner in light. S. Afr. J. Bot. 78, 139–146. doi: 10.1016/j.sajb.2011.06.009
Di Stasi, A. M., Mallozzi, C., Macchia, G., Maura, G., Petrucci, T. C., and Minetti, M. (2002). Peroxynitrite affects exocytosis and SNARE complex formation and induces tyrosine nitration of synaptic proteins. J. Neurochem. 82, 420–429. doi: 10.1046/j.1471-4159.2002.00980.x
Dietz, K. J. (2003). Plant peroxiredoxins. Annu. Rev. Plant Biol. 54, 93–107. doi: 10.1146/annurev.arplant.54.031902.134934
Dodge, J. D. (1970). Changes in chloroplast fine structure during the autumnal senescence of betula leaves. Ann. Bot. 34, 817–824. doi: 10.1093/oxfordjournals.aob.a084412
Doll, J., Muth, M., Riester, L., Nebel, S., Bresson, J., Lee, H.-C., et al. (2020). Arabidopsis thaliana WRKY25 transcription factor mediates oxidative stress tolerance and regulates senescence in a redox-dependent manner. Front. Plant Sci. 10:734. doi: 10.3389/fpls.2019.01734
Du, J., Li, M., Kong, D., Wang, L., Lv, Q., Wang, J., et al. (2013). Nitric oxide induces cotyledon senescence involving co-operation of the NES1/MAD1 and EIN2-associated ORE1 signalling pathways in Arabidopsis. J. Exp. Bot. 65, 4051–4063. doi: 10.1093/jxb/ert429
El-Maarouf-Bouteau, H., Mazuy, C., Corbineau, F., and Bailly, C. (2011). DNA alteration and programmed cell death during ageing of sunflower seed. J. Exp. Bot. 62, 5003–5011. doi: 10.1093/jxb/err198
Eum, H. L., Kim, H. B., Choi, S. B., and Lee, S. K. (2009). Regulation of ethylene biosynthesis by nitric oxide in tomato (Solanum lycopersicum L.) fruit harvested at different ripening stages. Eur. Food Res. Technol. 228, 331–338. doi: 10.1007/s00217-008-0938-3
Feechan, A., Kwon, E., Yun, B. W., Wang, Y. Q., Pallas, J. A., and Loake, G. J. (2005). A central role for S-nitrosothiols in plant disease resistance. Proc. Natl. Acad. Sci. U. S. A. 102, 8054–8059. doi: 10.1073/pnas.0501456102
Feng, J., Wang, C., Chen, Q., Chen, H., Ren, B., Li, X., et al. (2013). S-nitrosylation of phosphotransfer proteins represses cytokinin signaling. Nat. Commun. 4:1529. doi: 10.1038/ncomms2541
Freschi, L. (2013). Nitric oxide and phytohormone interactions: current status and perspectives. Front. Plant Sci. 4:398. doi: 10.3389/fpls.2013.00398
Fuentes, L., Figueroa, C. R., and Valdenegro, M. (2019). Recent advances in hormonal regulation and cross-talk during non-climacteric fruit development and ripening. Horticulture 5:45. doi: 10.3390/horticulturae5020045
Galatro, A., Puntarulo, S., Guiamet, J. J., and Simontacchi, M. (2013). Chloroplast functionality has a positive effect on nitric oxide level in soybean cotyledons. Plant Physiol. Biochem. 66, 26–33. doi: 10.1016/j.plaphy.2013.01.019
Girondé, A., Etienne, P., Trouverie, J., Bouchereau, A., Le Cahérec, F., Leport, L., et al. (2015). The contrasting N management of two oilseed rape genotypes reveals the mechanisms of proteolysis associated with leaf N remobilization and the respective contributions of leaves and stems to N storage and remobilization during seed filling. BMC Plant Biol. 15:59. doi: 10.1186/s12870-015-0437-1
Gniazdowska, A., Dobrzyńska, U., Babańczyk, T., and Bogatek, R. (2007). Breaking the apple embryo dormancy by nitric oxide involves the stimulation of ethylene production. Planta 225, 1051–1057. doi: 10.1007/s00425-006-0384-z
Gniazdowska, A., Krasuska, U., Czajkowska, K., and Bogatek, R. (2010). Nitric oxide, hydrogen cyanide and ethylene are required in the control of germination and undisturbed development of young apple seedlings. Plant Growth Regul. 61, 75–84. doi: 10.1007/s10725-010-9452-2
Gramegna, G., Rosado, D., Sánchez Carranza, A. P., Cruz, A. B., Simon-Moya, M., Llorente, B., et al. (2019). Phytochrome-interacting factor 3 mediates light-dependent induction of tocopherol biosynthesis during tomato fruit ripening. Plant Cell Environ. 42, 1328–1339. doi: 10.1111/pce.13467
Greenberg, J. T., and Yao, N. (2004). The role and regulation of programmed cell death in plant-pathogen interactions. Cell. Microbiol. 6, 201–211. doi: 10.1111/j.1462-5822.2004.00361.x
Guo, F. Q., and Crawford, N. M. (2005). Arabidopsis nitric oxide synthase1 is targeted to mitochondria and protects against oxidative damage and dark-induced senescence. Plant Cell 17, 3436–3450. doi: 10.1105/tpc.105.037770
He, Y., Xue, H., Li, Y., and Wang, X. (2018). Nitric oxide alleviates cell death through protein S-nitrosylation and transcriptional regulation during the ageing of elm seeds. J. Exp. Bot. 69, 5141–5155. doi: 10.1093/jxb/ery270
Hiruma, K., Fukunaga, S., Bednarek, P., Pislewska-Bednarek, M., Watanabe, S., Narusaka, Y., et al. (2013). Glutathione and tryptophan metabolism are required for Arabidopsis immunity during the hypersensitive response to hemibiotrophs. Proc. Natl. Acad. Sci. U. S. A. 110, 9589–9594. doi: 10.1073/pnas.1305745110
Horling, F., Lamkemeyer, P., König, J., Finkemeier, I., Kandlbinder, A., Baier, M., et al. (2003). Divergent light-, ascorbate-, and oxidative stress-dependent regulation of expression of the peroxiredoxin gene family in Arabidopsis. Plant Physiol. 131, 317–325. doi: 10.1104/pp.010017
Hsiao, H.-Y., Chung, C.-W., Santos, J. H., Villaflores, O. B., and Lu, T.-T. (2019). Fe in biosynthesis, translocation, and signal transduction of NO: toward bioinorganic engineering of dinitrosyl iron complexes into NO-delivery scaffolds for tissue engineering. Dalton Trans. 48, 9431–9453. doi: 10.1039/C9DT00777F
Huang, X., Stettmaier, K., Michel, C., Hutzler, P., Mueller, M. J., and Durner, J. (2004). Nitric oxide is induced by wounding and influences jasmonic acid signaling in Arabidopsis thaliana. Planta 218, 938–946. doi: 10.1007/s00425-003-1178-1
Hung, K. T., and Kao, C. H. (2004). Nitric oxide acts as an antioxidant and delays methyl jasmonate-induced senescence of rice leaves. J. Plant Physiol. 161, 43–52. doi: 10.1078/0176-1617-01178
Hussain, A., Imran, Q. M., Shahid, M., and Yun, B.-W. (2022). “Nitric oxide synthase in the plant kingdom,” in Nitric Oxide in Plant Biology. eds. V. Pratap Singh, S. Singh, D. K. Tripathi, M. C. Romero-Puertas, and L. M. Sandalio (Academic Press), 43–52.
Hussain, A., Mun, B.-G., Imran, Q. M., Lee, S.-U., Adamu, T. A., Shahid, M., et al. (2016). Nitric oxide mediated transcriptome profiling reveals activation of multiple regulatory pathways in Arabidopsis thaliana. Front. Plant Sci. 7:975. doi: 10.3389/fpls.2016.00975
Hussain, A., Yun, B.-W., Kim, J. H., Gupta, K. J., Hyung, N.-I., and Loake, G. J. (2019). Novel and conserved functions of S-nitrosoglutathione reductase in tomato. J. Exp. Bot. 70, 4877–4886. doi: 10.1093/jxb/erz234
Iakimova, E. T., Sobiczewski, P., Michalczuk, L., Węgrzynowicz-Lesiak, E., Mikiciński, A., and Woltering, E. J. (2013). Morphological and biochemical characterization of Erwinia amylovora-induced hypersensitive cell death in apple leaves. Plant Physiol. Biochem. 63, 292–305. doi: 10.1016/j.plaphy.2012.12.006
Ischebeck, T., Zbierzak, A. M., Kanwischer, M., and DöRmann, P. (2006). A salvage pathway for phytol metabolism in Arabidopsis. J. Biol. Chem. 281, 2470–2477. doi: 10.1074/jbc.M509222200
Jasid, S., Simontacchi, M., Bartoli, C. G., and Puntarulo, S. (2006). Chloroplasts as a nitric oxide cellular source. Effect of reactive nitrogen species on chloroplastic lipids and proteins. Plant Physiol. 142, 1246–1255. doi: 10.1104/pp.106.086918
Ji, Y., Liu, J., and Xing, D. (2016). Low concentrations of salicylic acid delay methyl jasmonate-induced leaf senescence by up-regulating nitric oxide synthase activity. J. Exp. Bot. 67, 5233–5245. doi: 10.1093/jxb/erw280
Kawakita, K. (2014). Studies on NO functions in plant defense responses. J. Gen. Plant Pathol. 80, 510–513. doi: 10.1007/s10327-014-0552-0
Kawakita, K., Shahjahan, M. M., and Takemoto, D. (2010). “Plant defense-related activities of NO producing elicitor candidates on potato and Nicotiana benthamiana,” in Nitric Oxide-Biology and Chemistry. Vol. 22. USA: Academic Press Inc. Elsevier Science, S77–S77.
Kawakita, K., Yamamoto, A., Katou, S., Yoshioka, H., and Doke, N. (2004). “Involvement of NO in plant defense responses and role of nitrate reductase in NO production,” in Nitric Oxide-Biology and Chemistry. Vol. 11. USA: Academic Press Inc. Elsevier Science, 37–37.
Khan, M., Imran, Q. M., Shahid, M., Mun, B. G., Lee, S. U., Khan, M. A., et al. (2019). Nitric oxide- induced AtAO3 differentially regulates plant defense and drought tolerance in Arabidopsis thaliana. BMC Plant Biol. 19:602. doi: 10.1186/s12870-019-2210-3
Kim, Y.-M., Bombeck, C. A., and Billiar, T. R. (1999). Nitric oxide as a bifunctional regulator of apoptosis. Circ. Res. 84, 253–256. doi: 10.1161/01.RES.84.3.253
Kim, J., Kim, J. H., Lyu, J. I., Woo, H. R., and Lim, P. O. (2017). New insights into the regulation of leaf senescence in Arabidopsis. J. Exp. Bot. 69, 787–799. doi: 10.1093/jxb/erx287
Kim, J. H., Woo, H. R., Kim, J., Lim, P. O., Lee, I. C., Choi, S. H., et al. (2009). Trifurcate feed-forward regulation of age-dependent cell death involving miR164 in Arabidopsis. Science 323, 1053–1057. doi: 10.1126/science.1166386
Kim, P. K., Zamora, R., Petrosko, P., and Billiar, T. R. (2001). The regulatory role of nitric oxide in apoptosis. Int. Immunopharmacol. 1, 1421–1441. doi: 10.1016/S1567-5769(01)00088-1
Kolbert, Z., Feigl, G., Freschi, L., and Poór, P. (2019). Gasotransmitters in action: nitric oxide-ethylene crosstalk during plant growth and abiotic stress responses. Antioxidants 8:167. doi: 10.3390/antiox8060167
Kong, X., Zhang, D., Pan, J., Zhou, Y., and Li, D. (2013). Hydrogen peroxide is involved in nitric oxide-induced cell death in maize leaves. Plant Biol. 15, 53–59. doi: 10.1111/j.1438-8677.2012.00598.x
Kopyra, M., and Gwozdz, E. A. (2004). The role of nitric oxide in plant growth regulation and responses to abiotic stresses. Acta Physiol. Plant. 26, 459–473. doi: 10.1007/s11738-004-0037-4
Krasuska, U., Ciacka, K., Orzechowski, S., Fettke, J., Bogatek, R., and Gniazdowska, A. (2016). Modification of the endogenous NO level influences apple embryos dormancy by alterations of nitrated and biotinylated protein patterns. Planta 244, 877–891. doi: 10.1007/s00425-016-2553-z
Kwon, E., Feechan, A., Yun, B. W., Hwang, B. H., Pallas, J. A., Kang, J. G., et al. (2012). AtGSNOR1 function is required for multiple developmental programs in Arabidopsis. Planta 236, 887–900. doi: 10.1007/s00425-012-1697-8
Lang, Z. B., and Zuo, J. R. (2015). Say “NO” to ABA signaling in guard cells by S-nitrosylation of OST1. Sci. China Life Sci. 58, 313–314. doi: 10.1007/s11427-015-4823-6
Lee, U., Wie, C., Fernandez, B. O., Feelisch, M., and Vierling, E. (2008). Modulation of nitrosative stress by S-nitrosoglutathione reductase is critical for thermotolerance and plant growth in Arabidopsis. Plant Cell 20, 786–802. doi: 10.1105/tpc.107.052647
León, J., and Costa-Broseta, Á. (2020). Present knowledge and controversies, deficiencies, and misconceptions on nitric oxide synthesis, sensing, and signaling in plants. Plant Cell Environ. 43, 1–15. doi: 10.1111/pce.13617
Leshem, Y. A. Y., and Haramaty, E. (1996). The characterization and contrasting effects of the nitric oxide free radical in vegetative stress and senescence of Pisum sativum Linn. Foliage. J. Plant Physiol. 148, 258–263. doi: 10.1016/S0176-1617(96)80251-3
Leshem, Y. A. Y., and Pinchasov, Y. (2000). Non-invasive photoacoustic spectroscopic determination of relative endogenous nitric oxide and ethylene content stoichiometry during the ripening of strawberries Fragaria anannasa (Duch.) and avocados Persea americana (Mill.). J. Exp. Bot. 51, 1471–1473. doi: 10.1093/jexbot/51.349.1471
Leshem, Y. A. Y., Wills, R. B. H., and Ku, V. V.-V. (1998). Evidence for the function of the free radical gas — nitric oxide (NO•) — as an endogenous maturation and senescence regulating factor in higher plants. Plant Physiol. Biochem. 36, 825–833. doi: 10.1016/S0981-9428(99)80020-5
Leshem, Y., Wills, R., and Ku, V. (2001). Applications of nitric oxide (NO) for postharvest control. Acta Hortic. 553, 571–576. doi: 10.17660/ActaHortic.2001.553.135
Lim, P. O., Kim, H. J., and Nam, H. G. (2007). Leaf senescence. Annu. Rev. Plant Biol. 58, 115–136. doi: 10.1146/annurev.arplant.57.032905.105316
Lin, A., Wang, Y., Tang, J., Xue, P., Li, C., Liu, L., et al. (2011). Nitric oxide and protein S-nitrosylation are integral to hydrogen peroxide-induced leaf cell death in rice. Plant Physiol. 158, 451–464. doi: 10.1104/pp.111.184531
Lindermayr, C., Saalbach, G., and Durner, J. (2005). Proteomic identification of S-nitrosylated proteins in Arabidopsis. Plant Physiol. 137, 921–930. doi: 10.1104/pp.104.058719
Liu, F., and Guo, F. Q. (2013). Nitric oxide deficiency accelerates chlorophyll breakdown and stability loss of thylakoid membranes during dark-induced leaf senescence in Arabidopsis. PLoS One 8:e56345. doi: 10.1371/journal.pone.0056345
Lopez-Molina, L., Mongrand, B., Mclachlin, D. T., Chait, B. T., and Chua, N. H. (2002). ABI5 acts downstream of ABI3 to execute an ABA-dependent growth arrest during germination. Plant J. 32, 317–328. doi: 10.1046/j.1365-313X.2002.01430.x
Ma, W., Smigel, A., Walker, R. K., Moeder, W., Yoshioka, K., and Berkowitz, G. A. (2010). Leaf senescence signaling: the Ca2+−conducting Arabidopsis cyclic nucleotide gated channel2 acts through nitric oxide to repress senescence programming. Plant Physiol. 154, 733–743. doi: 10.1104/pp.110.161356
Magalhaes, J., Monte, D., and Durzan, D. J. (2000). Nitric oxide and ethylene emission in Arabidopsis thaliana. Physiol. Mol. Biol. Plants 6, 117–127.
Malik, S. I., Hussain, A., Yun, B. W., Spoel, S. H., and Loake, G. J. (2011). GSNOR-mediated de-nitrosylation in the plant defence response. Plant Sci. 181, 540–544. doi: 10.1016/j.plantsci.2011.04.004
Manjunatha, G., Gupta, K. J., Lokesh, V., Mur, L. A. J., and Neelwarne, B. (2012). Nitric oxide counters ethylene effects on ripening fruits. Plant Signal. Behav. 7, 476–483. doi: 10.4161/psb.19523
Mao, C., Zhu, Y., Cheng, H., Yan, H., Zhao, L., Tang, J., et al. (2018). Nitric oxide regulates seedling growth and mitochondrial responses in aged oat seeds. Int. J. Mol. Sci. 19:1052. doi: 10.3390/ijms19041052
Mata-Pérez, C., Sánchez-Calvo, B., Padilla, M. N., Begara-Morales, J. C., Valderrama, R., Corpas, F. J., et al. (2017). Nitro-fatty acids in plant signaling: new key mediators of nitric oxide metabolism. Redox Biol. 11, 554–561. doi: 10.1016/j.redox.2017.01.002
Mishina, T. E., Lamb, C., and Zeier, J. (2007). Expression of a nitric oxide degrading enzyme induces a senescence programme in Arabidopsis. Plant Cell Environ. 30, 39–52. doi: 10.1111/j.1365-3040.2006.01604.x
Moseler, A., Aller, I., Wagner, S., Nietzel, T., Przybyla-Toscano, J., Mühlenhoff, U., et al. (2015). The mitochondrial monothiol glutaredoxin S15 is essential for iron-sulfur protein maturation in Arabidopsis thaliana. Proc. Natl. Acad. Sci. U. S. A. 112, 13735–13740. doi: 10.1073/pnas.1510835112
Mühlenhoff, U., Gerber, J., Richhardt, N., and Lill, R. (2003). Components involved in assembly and dislocation of iron-sulfur clusters on the scaffold protein Isu1p. EMBO J. 22, 4815–4825. doi: 10.1093/emboj/cdg446
Mun, B. G., Lee, S. U., Park, E. J., Kim, H. H., Hussain, A., Imran, Q. M., et al. (2017). Analysis of transcription factors among differentially expressed genes induced by drought stress in Populus davidiana. 3 Biotech 7:209. doi: 10.1007/s13205-017-0858-7
Mur, L. A., Carver, T. L., and Prats, E. (2006). NO way to live; the various roles of nitric oxide in plant-pathogen interactions. J. Exp. Bot. 57, 489–505. doi: 10.1093/jxb/erj052
Mur, L. A. J., Prats, E., Pierre, S., Hall, M. A., and Hebelstrup, K. H. (2013). Integrating nitric oxide into salicylic acid and jasmonic acid/ethylene plant defense pathways. Front. Plant Sci. 4:215. doi: 10.3389/fpls.2013.00215
Murgia, I., Delledonne, M., and Soave, C. (2002). Nitric oxide mediates iron-induced ferritin accumulation in Arabidopsis. Plant J. 30, 521–528. doi: 10.1046/j.1365-313X.2002.01312.x
Nabi, R. B. S., Tayade, R., Hussain, A., Kulkarni, K. P., Imran, Q. M., Mun, B.-G., et al. (2019). Nitric oxide regulates plant responses to drought, salinity, and heavy metal stress. Environ. Exp. Bot. 161, 120–133. doi: 10.1016/j.envexpbot.2019.02.003
Niu, Y. H., and Guo, F. Q. (2012). Nitric oxide regulates dark-induced leaf senescence through EIN2 in Arabidopsis. J. Integr. Plant Biol. 54, 516–525. doi: 10.1111/j.1744-7909.2012.01140.x
Piterkova, J., Luhova, L., Hofman, J., Tureckova, V., Novak, O., Petrivalsky, M., et al. (2012). Nitric oxide is involved in light-specific responses of tomato during germination under normal and osmotic stress conditions. Ann. Bot. 110, 767–776. doi: 10.1093/aob/mcs141
Planchet, E., Sonoda, M., Zeier, J., and Kaiser, W. M. (2006). Nitric oxide (NO) as an intermediate in the cryptogein-induced hypersensitive response-a critical re-evaluation. Plant Cell Environ. 29, 59–69. doi: 10.1111/j.1365-3040.2005.01400.x
Romero-Puertas, M. C., Corpas, F. J., Rodríguez-Serrano, M., Gómez, M., Del Río, L. A., and Sandalio, L. M. (2007a). Differential expression and regulation of antioxidative enzymes by cadmium in pea plants. J. Plant Physiol. 164, 1346–1357. doi: 10.1016/j.jplph.2006.06.018
Romero-Puertas, M. C., Laxa, M., Mattè, A., Zaninotto, F., Finkemeier, I., Jones, A. M., et al. (2007b). S-nitrosylation of peroxiredoxin II E promotes peroxynitrite-mediated tyrosine nitration. Plant Cell 19, 4120–4130. doi: 10.1105/tpc.107.055061
Romero-Puertas, M. C., Perazzolli, M., Zago, E. D., and Delledonne, M. (2004). Nitric oxide signalling functions in plant-pathogen interactions. Cell. Microbiol. 6, 795–803. doi: 10.1111/j.1462-5822.2004.00428.x
Roschzttardtz, H., Conéjéro, G., Divol, F., Alcon, C., Verdeil, J.-L., Curie, C., et al. (2013). New insights into Fe localization in plant tissues. Front. Plant Sci. 4:350. doi: 10.3389/fpls.2013.00350
Rudell, D. R., and Mattheis, J. P. (2006). Nitric oxide and nitrite treatments reduce ethylene evolution from apple fruit disks. HortScience 41, 1462–1465. doi: 10.21273/HORTSCI.41.6.1462
Sang, J., Jiang, M., Lin, F., Xu, S., Zhang, A., and Tan, M. (2008). Nitric oxide reduces hydrogen peroxide accumulation involved in water stress-induced subcellular anti-oxidant defense in maize plants. J. Integr. Plant Biol. 50, 231–243. doi: 10.1111/j.1744-7909.2007.00594.x
Sano, N., Rajjou, L., North, H. M., Debeaujon, I., Marion-Poll, A., and Seo, M. (2015). Staying alive: molecular aspects of seed longevity. Plant Cell Physiol. 57, 660–674. doi: 10.1093/pcp/pcv186
Schippers, J. H. M., Schmidt, R., Wagstaff, C., and Jing, H.-C. (2015). Living to die and dying to live: the survival strategy behind leaf senescence. Plant Physiol. 169, 914–930. doi: 10.1104/pp.15.00498
Signorelli, S., and Considine, M. J. (2018). Nitric oxide enables germination by a four-pronged attack on ABA-induced seed dormancy. Front. Plant Sci. 9:296. doi: 10.3389/fpls.2018.00296
Sirova, J., Sedlarova, M., Piterkova, J., Luhova, L., and Petrivalsky, M. (2011). The role of nitric oxide in the germination of plant seeds and pollen. Plant Sci. 181, 560–572. doi: 10.1016/j.plantsci.2011.03.014
Snyder, C. M., Shroff, E. H., Liu, J., and Chandel, N. S. (2009). Nitric oxide induces cell death by regulating anti-apoptotic BCL-2 family members. PLoS One 4:e7059. doi: 10.1371/journal.pone.0007059
Terrile, M. C., París, R., Calderón-Villalobos, L. I., Iglesias, M. J., Lamattina, L., Estelle, M., et al. (2012). Nitric oxide influences auxin signaling through S-nitrosylation of the Arabidopsis transport inhibitor response 1 auxin receptor. Plant J. 70, 492–500. doi: 10.1111/j.1365-313X.2011.04885.x
Terrón-Camero, L. C., Peláez-Vico, M. Á., Del-Val, C., Sandalio, L. M., and Romero-Puertas, M. C. (2019). Role of nitric oxide in plant responses to heavy metal stress: exogenous application versus endogenous production. J. Exp. Bot. 70, 4477–4488. doi: 10.1093/jxb/erz184
Tewari, R. K., Horemans, N., and Watanabe, M. (2020). Evidence for a role of nitric oxide in iron homeostasis in plants. J. Exp. Bot. 72, 990–1006. doi: 10.1093/jxb/eraa484
Thomas, C., Mackey, M. M., Diaz, A. A., and Cox, D. P. (2009). Hydroxyl radical is produced via the Fenton reaction in submitochondrial particles under oxidative stress: implications for diseases associated with iron accumulation. Redox Rep. 14, 102–108. doi: 10.1179/135100009X392566
Trnka, D., Engelke, A. D., Gellert, M., Moseler, A., Hossain, M. F., Lindenberg, T. T., et al. (2020). Molecular basis for the distinct functions of redox-active and FeS-transfering glutaredoxins. Nat. Commun. 11:3445. doi: 10.1038/s41467-020-17323-0
Tun, N. N., Holk, A., and Scherer, G. F. (2001). Rapid increase of NO release in plant cell cultures induced by cytokinin. FEBS Lett. 509, 174–176. doi: 10.1016/S0014-5793(01)03164-7
Vanlerberghe, G. C. (2013). Alternative oxidase: a mitochondrial respiratory pathway to maintain metabolic and signaling homeostasis during abiotic and biotic stress in plants. Int. J. Mol. Sci. 14, 6805–6847. doi: 10.3390/ijms14046805
Wang, Y. Q., Chen, C., Loake, G. J., and Chu, C. C. (2010). Nitric oxide: promoter or suppressor of programmed cell death? Protein Cell 1, 133–142. doi: 10.1007/s13238-010-0018-x
Wang, Y., Lin, A., Loake, G. J., and Chu, C. (2013a). H2O2-induced leaf cell death and the crosstalk of reactive nitric/oxygen species. J. Integr. Plant Biol. 55, 202–208. doi: 10.1111/jipb.12032
Wang, Y., Loake, G., and Chu, C. (2013b). Cross-talk of nitric oxide and reactive oxygen species in plant programed cell death. Front. Plant Sci. 4:314. doi: 10.3389/fpls.2013.00314
Wilhelmova, N., Fuksova, H., Srbova, M., Mikova, D., Mytinova, Z., Prochazkova, D., et al. (2006). The effect of plant cytokinin hormones on the production of ethylene, nitric oxide, and protein nitrotyrosine in ageing tobacco leaves. Biofactors 27, 203–211. doi: 10.1002/biof.5520270118
Winterbourn, C. C. (1995). Toxicity of iron and hydrogen peroxide: the Fenton reaction. Toxicol. Lett. 82–83, 969–974. doi: 10.1016/0378-4274(95)03532-X
Yan, B., Ai, Y., Sun, Q., Ma, Y., Cao, Y., Wang, J., et al. (2021). Membrane damage during Ferroptosis is caused by oxidation of phospholipids catalyzed by the oxidoreductases POR and CYB5R1. Mol. Cell 81, 355.e10–369.e10. doi: 10.1016/j.molcel.2020.11.024
Yang, H., Kim, H.-J., Chen, H., Lu, Y., Lu, X., Wang, C., et al. (2018). Reactive oxygen species and nitric oxide induce senescence of rudimentary leaves and the expression profiles of the related genes in Litchi chinensis. Horticult. Res. 5:23. doi: 10.1038/s41438-018-0029-y
Yang, W. S., and Stockwell, B. R. (2016). Ferroptosis: death by lipid peroxidation. Trends Cell Biol. 26, 165–176. doi: 10.1016/j.tcb.2015.10.014
Yun, B. W., Feechan, A., Yin, M., Saidi, N. B., Le Bihan, T., Yu, M., et al. (2011). S-nitrosylation of NADPH oxidase regulates cell death in plant immunity. Nature 478, 264–268. doi: 10.1038/nature10427
Yun, B. W., Skelly, M. J., Yin, M., Yu, M., Mun, B. G., Lee, S. U., et al. (2016). Nitric oxide and S-nitrosoglutathione function additively during plant immunity. New Phytol. 211, 516–526. doi: 10.1111/nph.13903
Zhu, S.-H., and Zhou, J. (2007). Effect of nitric oxide on ethylene production in strawberry fruit during storage. Food Chem. 100, 1517–1522. doi: 10.1016/j.foodchem.2005.12.022
Zou, Y., Li, H., Graham, E. T., Deik, A. A., Eaton, J. K., Wang, W., et al. (2021). Author correction: cytochrome P450 oxidoreductase contributes to phospholipid peroxidation in ferroptosis. Nat. Chem. Biol. 17:501. doi: 10.1038/s41589-021-00767-w
Zuccarelli, R., Rodríguez-Ruiz, M., Lopes-Oliveira, P. J., Pascoal, G. B., Andrade, S. C. S., Furlan, C. M., et al. (2020). Multifaceted roles of nitric oxide in tomato fruit ripening: NO-induced metabolic rewiring and consequences for fruit quality traits. J. Exp. Bot. 72, 941–958. doi: 10.1093/jxb/eraa526
Keywords: nitric oxide, senescence, programmed cell death, ageing, ROS
Citation: Hussain A, Shah F, Ali F and Yun B-W (2022) Role of Nitric Oxide in Plant Senescence. Front. Plant Sci. 13:851631. doi: 10.3389/fpls.2022.851631
Received: 10 January 2022; Accepted: 15 March 2022;
Published: 05 April 2022.
Edited by:
Magda Pál, Centre for Agricultural Research, HungaryReviewed by:
Adam Solti, Eötvös Loránd University, HungaryCopyright © 2022 Hussain, Shah, Ali and Yun. This is an open-access article distributed under the terms of the Creative Commons Attribution License (CC BY). The use, distribution or reproduction in other forums is permitted, provided the original author(s) and the copyright owner(s) are credited and that the original publication in this journal is cited, in accordance with accepted academic practice. No use, distribution or reproduction is permitted which does not comply with these terms.
*Correspondence: Adil Hussain, YWRpbGh1c3NhaW5AYXdrdW0uZWR1LnBr; Byung-Wook Yun, Ynd5dW5Aa251LmFjLmty
Disclaimer: All claims expressed in this article are solely those of the authors and do not necessarily represent those of their affiliated organizations, or those of the publisher, the editors and the reviewers. Any product that may be evaluated in this article or claim that may be made by its manufacturer is not guaranteed or endorsed by the publisher.
Research integrity at Frontiers
Learn more about the work of our research integrity team to safeguard the quality of each article we publish.