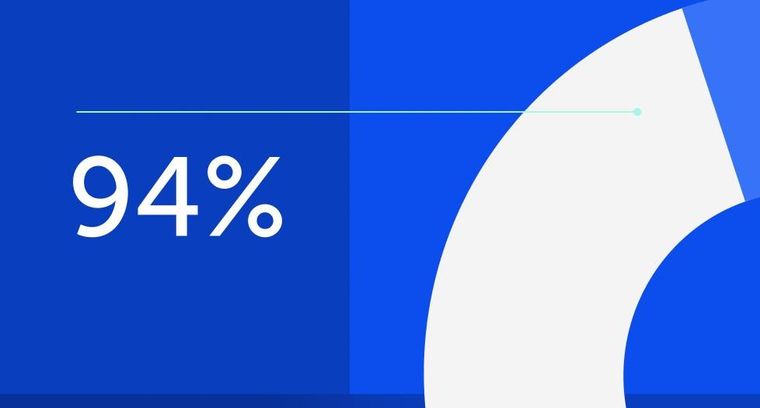
94% of researchers rate our articles as excellent or good
Learn more about the work of our research integrity team to safeguard the quality of each article we publish.
Find out more
ORIGINAL RESEARCH article
Front. Plant Sci., 24 March 2022
Sec. Plant Abiotic Stress
Volume 13 - 2022 | https://doi.org/10.3389/fpls.2022.848464
This article is part of the Research TopicChlorophyll Fluorescence Analysis in Biotic and Abiotic Stress, Volume IIView all 5 articles
Background and Aims: Climate warming has become an indisputable fact, and wheat is among the most heat-sensitive cereal crops. Heat stress during grain filling threatens global wheat production and food security. Here, we analyzed the physiological and proteomic changes by delayed sowing on the photosynthetic capacity of winter wheat leaves under heat stress. Our aim is to provide a new cultivation way for the heat stress resistance in wheat.
Methods: Through 2 years field experiment and an open warming simulation system, we compared the changes in wheat grain weight, yield, photosynthetic rate, and chlorophyll fluorescence parameters under heat stress at late grain–filling stage during normal sowing and delayed sowing. At the same time, based on the iTRAQ proteomics, we compared the changes of differentially expressed proteins (DEPs) during the two sowing periods under high temperature stress.
Key Results: In our study, compared with normal sowing, delayed sowing resulted in a significantly higher photosynthetic rate during the grain-filling stage under heat stress, as well as significantly increased grain weight and yield at maturity. The chlorophyll a fluorescence transient (OJIP) analysis showed that delayed sowing significantly reduced the J-step and I-step. Moreover, OJIP parameters, including RC/CSm, TRo/CSm, ETo/CSm, DIo/CSm and ΦPo, ψo, ΦEo, were significantly increased; DIo/CSm and ΦDo, were significantly reduced. GO biological process and KEGG pathway enrichment analyses showed that, among DEPs, proteins involved in photosynthetic electron transport were significantly increased and among photosynthetic metabolic pathways, we have observed upregulated proteins, such as PsbH, PsbR, and PetB.
Conclusion: Physiological and proteomic analyses indicate delaying the sowing date of winter wheat reduced heat dissipation by enhancing the scavenging capacity of reactive oxygen species (ROS) in flag leaves, and ensuring energy transmission along the photosynthetic electron transport chain; this increased the distribution ratio of available energy in photochemical reactions and maintained a high photosynthetic system assimilation capacity, which supported a high photosynthetic rate. Hence, delayed sowing may represent a new cultivation strategy for promoting heat stress tolerance in winter wheat.
The global temperature is projected to increase by 1.5–4°C by the end of this century, and climate warming has become an indisputable fact (Rasul et al., 2012; Field et al., 2014; Abbas et al., 2017; IPCC, 2018). Rising temperatures have severely affected agricultural production in many regions worldwide, including Russia (Giannakaki and Calanca, 2019), United States (Tack et al., 2015; Bergkamp et al., 2018), South Africa (Shew et al., 2020), South America (Rivelli et al., 2021), and China (Ren et al., 2011; Chen et al., 2018). Every 1°C increase from mean crop temperature causes global wheat yield losses of up to 6% (Lipec et al., 2013). Moreover, the area, frequency, and duration of heat stress during the wheat grain-filling stage has increased, threatening global wheat production and food security. Hence, enhancing the heat tolerance of winter wheat will have an important role in ensuring food security.
Photosynthesis is the process by which plants use light energy to convert absorbed carbon dioxide into organic compounds that provide energy for plant growth and development, and is an important way for green plants to accumulate dry matter (Pan et al., 2012). Heat stress affects many photosynthetic processes, including damage to photosystem II (PSII; Li et al., 2009; Mathur et al., 2014), damage to the thylakoid membrane (Prasad et al., 2008; Narayanan et al., 2015), alteration of antioxidant enzyme activity (Wang et al., 2011, 2015; Djanaguiraman et al., 2018), and reduction of chlorophyll content (Ristic et al., 2007; Prasad et al., 2008). Very high temperatures may also damage the leaf photosynthetic apparatus, leading to premature induction of leaf senescence or forced maturity at the grain-filling stage, resulting in decreased grain weight and yield (Zhao et al., 2007; Bergkamp et al., 2018; Mirosavljevi et al., 2021; Sarkar et al., 2021). At present, most studies promote crop resistance to heat stress through the application of exogenous hormones. Ethylene regulates photosynthesis through carbohydrate metabolism and antioxidant system, thus affecting the high temperature tolerance of rice (Gautam et al., 2022). Methyl jasmonate is a potential tool to protect PS II and D1 proteins in wheat plants under heat stress conditions, accelerating the recovery of photosynthetic capacity (Fatma et al., 2021). Melatonin and H2S protect against heat stress-induced photosynthetic inhibition by regulating carbohydrate metabolism (Iqbal et al., 2021).
In the North China Plain, global warming increased the average temperature from sowing to overwintering, and also prolongs the growth period before overwintering owing to the reduction of the period with frosts, as a result of which prompted farmers to delay the sowing time of winter wheat (Sun et al., 2007; Xiao et al., 2013, 2015; Liu et al., 2018). Delayed sowing shortens wheat growing period before overwintering, which reduced the number of main stem leaves and tillers, which reduces the number of leaves (of the main stem) and reduces the number of tillers, optimizes the nitrogen distribution in leaves (Yin et al., 2018). In addition, in short crop cycles there is less temporal dispersion between the oldest (basal) leaves and the newest (apical) ones, improves proportion of young leaves. All these changes result in higher photosynthetic rates at the individual leaf level and canopy photosynthetic nitrogen use efficiency, which resulted in the maintained grain yield (Yin et al., 2019). However, it is unclear whether delayed sowing of wheat can maintain a high photosynthetic capacity under heat stress during the grain-filling stage.
Isobaric tags for relative and absolute quantitation (iTRAQ) analysis has been used widely in proteomics quantitative research due to its unique advantages. Because proteins are the primary drivers of cellular events, proteomic analysis is becoming a powerful tool for analyzing plant reactions to environmental stresses (Budak et al., 2013) and uncovering the major processes triggered by such stresses, such as heat stress adaptation. Therefore, we conducted a comparative physiological and proteomic study of winter wheat leaves under normal and delayed sowing to reveal the mechanistic effects of delayed sowing on photosynthesis and their physiological function under heat stress, providing a theoretical basis and technical support for the cultivation of heat-tolerant winter wheat.
The field experiment was conducted during the 2017–2018 and 2018–2019 growing seasons at the experimental station of Dongwu Village (35°97′N, 117°03′E), in Tai’an City, Shandong Province, China. The previous crop grown in the field was summer maize. The top 20 cm of the soil contained 20.61 g kg–1 organic matter, 1.41 g kg–1 total nitrogen, 29.80 mg kg–1 available phosphorus, and 78.89 mg kg–1 available potassium.
According to studies on the high-yield, high nitrogen use efficiency cultivation potential of delayed sowing in winter wheat (Dai et al., 2013; Yin et al., 2018; Zhu et al., 2019), the large ears and low tillering capacity cultivar Tainong 18 and a planting density of 405 plants m–2 were selected for this experiment. Wheat was sown on two dates, 8 and 22 October (normal and delayed sowing, respectively), both sowing dates were treated with heat stress from 19 days after anthesis and lasted for 3 days. There was 1 day difference in flowering time between the two sowing dates, the growth process of wheat under each treatment was shown in Supplementary Table 1. The rainfall and average temperature during the 2017–2018 and 2018–2019 growing seasons are shown in Supplementary Figure 1. The cumulative temperature above 0°C for the whole growing season, for the normal and delayed sowing dates, was 2,351.7 and 2,083.2°C d in 2017–2018 and 2,408.3 and 2,179.5°C d in 2018–2019, respectively. The cumulative temperature above 0°C prior to wintering, for the normal and late-sown treatments, was 632.8 and 401.2°C d in 2017–2018 and 623.1 and 394.4°C d in 2018–2019, respectively.
The experiment adopts a completely random arrangement design, each sowing date × thermal treatment combination repeated three times, a total of six plots, and the test area of each plot is 12 m2. Data from subsequent physiological experiments were analyzed based on three biological replicates in single year. The base application of pure nitrogen 120 kg.hm–2, P2O5 120 kg.hm–2, and K2O 120 kg.hm–2 before sowing, and the topdressing of pure nitrogen 120 kg.hm–2 at the jointing stage. Other management measures were the same as for high-yield fields. To ensure consistency in the subsequent experiments, we selected 80 healthy and uniform plants during the flowering period of each test plot to mark them for the determination and analysis of various indicators.
Heat stress was induced with an open field warming system using industrial high-power heaters (20 KW) to generate hot air delivered via a fan to a general air conveying belt and then to branched air belts placed between wheat rows. The hot air was blown through tubers and distributed through the field to uniformly increase the temperature. The heat treatment was carried out from 19 to 21 days after anthesis (DAA), from 10:00 to 16:00 every day. A temperature and humidity recorder (RC-4HA/C; Elitech, Puteaux, France) was fixed at the flag leaf layer, and data were recorded every 1 min. During heat stress (10:00 to 16:00), the temperature of the normal and delayed sowing were 4.75 and 5.19°C higher than the ambient temperature at the time, respectively, while the humidity of the normal and delayed sowing were maintained at about 40.07 and 40.81%, respectively (Figure 1 and Supplementary Figure 2). We found that the leaves temperature was about 1.41°C lower than the air temperature when measuring photosynthetic parameters, but there was no significant difference between normal and delayed sowing (Supplementary Figure 3). During heat stress (19–22 DAA), the average daily temperatures of the normal and delayed sowing were 22.65 and 22.95°C in 2017–2018, respectively, while the average daily temperatures of the normal and delayed sowing were 21.42 and 22.07°C in 2018–2019, respectively (Figure 1). Additionally, during the whole grain-filling period, the average daily temperatures of the normal and delayed sowing were 22.90 and 23.10°C in 2017–2018, respectively, while the average daily temperatures of the normal and delayed sowing were 20.55 and 20.64°C in 2018–2019, respectively (Supplementary Figure 1).
Figure 1. The average temperature of the air at the flag leaf layer between delayed sowing and normal sowing during heat stress (19–21 DAA). (A,B) Are delayed sowing and normal sowing in the 2017–2018 growing seasons, respectively. (C,D) Are delayed sowing and normal sowing in the 2018–2019 growing seasons, respectively. NT, Natural temperature; HT, Temperature under heat stress.
Healthy and uniform flag leaves of wheat plants selected to measure the net photosynthetic rate every 5 days from 15 DAA to maturity; 15 DAA represented the time before heat treatment, 20 DAA represented the time during heat treatment, and 25, 30, and 35 DAA represented the times after heat treatment. We measured 10 plants in the same area. The photosynthesis measurements were taken at 09:00–10:00 on 20 DAA, and the other measurements were taken at 09:00–11:30 when it was sunny using a CIRAS-3 portable photosynthesis measurement system (PP Systems, Amesbury, MA, United States).
In the 2018–2019, Healthy and uniform ten flag leaves of wheat plants were selected every 5 days from 15 DAA to maturity to maturity and stored at −80°C. Superoxide dismutase (SOD) activity was determined by NBT photochemical reduction method. Peroxidase (POD) activity was determined by guaiacol method. Catalase (CAT) activity was determined by hydrogen peroxide method. Malondialdehyde (MDA) content was determined by referring to Zhao et al. (1994).
Chlorophyll fluorescence parameters were measured on the third day before and after heat stress. Before the measurement, the wheat flag leaf leaves were dark-adapted for 30 min, and then irradiated with saturated pulsed light (PFD = 3000 μmol m–2 s–1) for 1 s. We measured the OJIP curve and related parameters with an M-PEA continuous excitation fluorometer (Hansatech Instruments Ltd., Pentney, United Kingdom) (Strasser, 1997). We measured 10 plants in the same area. According to the method of Öz et al. (2014), the OJIP curve was standardized and analyzed using the JIP-test.
We selected 300 healthy and uniform plants during the flowering period of each test plot to mark them in the 2018–2019. Thirty panicles were sampled from 5 DAA and then every 5 days until wheat matured. The grain filling process was fitted by Logistic equation, as follows:
where y was grain weight, t was post-flowering time (d), and z, w, and m were fitting parameters of the equation.
In each plot, plants were harvested from an area of 2.0 m by six rows (3 m2) to determine the grain yield and its components. The standard moisture content was 13%. And at the same time, spike number per unit area, grain number per spike and grain weight were recorded.
Ten flag leaves were stored on the fourth day after heat treatment (25 DAA) in the same area. They were ground in liquid nitrogen and transferred to centrifuge tubes, and then sonicated on ice in lysis buffer (1 M sucrose, 0.5 M Tris 8.0, 0.1 M KCl, 50 mM ascorbic acid, 1% NP40, 1% sodium deoxycholate, 10 mM EDTA, 10 mM dithiothreitol, 1% protease inhibitor cocktail, and 1% phosphatase inhibitor cocktail). After centrifugation (16,000 × g, 4°C, 10 min), debris was removed and the protein was precipitated with cold 0.1 M ammonium acetate/methanol for 12 h at −20°C. After centrifugation (16,000 × g, 4°C, 10 min), the remaining precipitate was washed three times with cold acetone. The protein was redissolved in buffer (8 M urea, 50 mM Tris 8.0, 1% NP40, 1% sodium deoxycholate, 10 mM EDTA, 5 mM dithiothreitol, 1% protease inhibitor cocktail, and 1% phosphatase inhibitor cocktail) and the protein concentration was determined with a 2D Quant Kit (GE Healthcare, United States) following the manufacturer’s protocol.
We transferred 500 μg of protein from each sample to a new test tube. The test tubes were each adjusted to an equal volume with lysis buffer, and then incubated at 30°C for 30 min. After cooling to room temperature, 30 mM iodoacetamide was added, and the mixture was incubated in the dark at room temperature for 45 min. Then, five volumes of cold acetone were added to the mixture and incubated overnight at 20°C. After incubation, the mixture was centrifuged at 2,000 × g at 4°C for 10 min. The supernatant was removed, and the pellet was resuspended in cold acetone and washed three times. The precipitate was collected, and 300 μL of 0.1 M tetraethylammonium bromide (TEAB) was added to the protein after air-drying, after which ultrasonication on ice was performed to promote the dissolution of the protein precipitate. Then, 20 μg of trypsin was added and incubated overnight at 37°C. After incubation, 1% trifluoroacetic acid was added to terminate the reaction. After trypsinization, the samples were collected via centrifugation for 12 min and vacuum freeze-drying. Peptides were reconstituted in 50 μL of 0.5 M TEAB solution according to the manufacturer’s protocol for 8-plex iTRAQ reagent (Applied Biosystems, United States). The peptide mixtures were then incubated for 2 h at 25°C and then pooled, desalted, and dried using vacuum centrifugation. We used the 117 and 119 tags for the control and delayed sowing treatments, respectively.
The peptide mixtures of each sample were fractionated by high-pH reverse-phase high-performance liquid chromatography using an XBridge Shield C18RP column (4.6 mm × 250 mm, 3.5 μm; Waters, United States). Then, according to the peak range displayed under an ultraviolet lamp, ∼60 components of the eluted peptide were combined into 15 components, and the vacuum concentrator was drained. Next, the dried peptide fractions were dissolved in solvent A and then analyzed by nano liquid chromatography tandem mass spectrometry (MS/MS) using an UltiMate 3000 RSLCnano system (Dionex, United States) coupled to an Orbitrap Fusion mass spectrometer (Thermo Scientific, United States). The MS scan range was set to m/z = 375–1800, and intact peptides were detected at a resolution of 120,000; peptides were selected for MS/MS using 30% normalized collision energy, and the MS/MS scan was set to m/z = 100–1800 with a resolution of 30,000. The automatic gain controls were set as 3E6 and 1E5 for MS/MS and MS, respectively. These scans targeted the 20 most abundant ions in each survey with a dynamic exclusion time of 30 s. The data-dependent acquisition procedure was applied.
The specific proteomic analysis method was provided by Micrometer Biotech Company (Hangzhou, China). Data were analyzed statistically using one-way analysis of variance and Duncan’s multiple range test with SPSS 25 (IBM Corp., United States), and the experimental years were analyzed separately. Differences were considered significant at P < 0.05.
There was no significant difference in grain weight of delayed and normal sowing during 0–20 DAA. But the grain weight of delayed sowing was significantly higher than that of normal sowing during 25–40 DAA (after heat stress), the fitting grain weight increased by 4.11, 11.43, 15.95, and 18.19%, respectively (Supplementary Figure 4).
Under heat stress, sowing date significantly affected the grain weight and yield, but there was no difference in grain number per unit area. Compared with normal sowing, the grain weight increased by 9.51 and 11.16% under delayed sowing and grain yield increased by 10.31 and 10.10% in the 2017–2018 and 2018–2019 growing seasons, respectively (Figure 2).
Figure 2. Effect of delayed sowing on grain number, grain weight, and grain yield of wheat under heat stress at late grain–filling stage. Values are means of three replicates per treatment. Vertical bars indicate standard error. Different letters denote statistical differences by LSD test (P < 0.05) between treatments for each parameter in the same year.
With wheat growth and development after anthesis, the photosynthetic rate showed a downward trend; however, the photosynthetic rate of the delayed sowing treatment was significantly higher than that of the normal sowing treatment (Figure 3). In 2017–2018, compared with normal sowing, the net photosynthetic rate in flag leaves after anthesis (15–35 days) increased by 3.39, 9.18, 16.19, 40.51, and 84.08% under delayed sowing. Similarly, in 2018–2019, the net photosynthetic rate in flag leaves after anthesis (15–35 days) increased by 5.90, 8.57, 18.30, 29.67, and 115.38%.
Figure 3. Effect of delayed sowing on photosynthetic rate of wheat flag leaves under heat stress at late grain–filling stage. With a gray area from 19 to 21 DAA. Values are means of three replicates per treatment. Vertical bars indicate standard error.
Under heat stress, in 2018–2019, compared with normal sowing, the SOD activity in flag leaves after anthesis (15–35 days) increased by 2.01, 5.33, 22.74, 24.23, and 28.72% under delayed sowing. Similarly, POD activity increased by 6.14, 3.99, 11.20, 22.85, and 19.04%, CAT activity increased by 6.72, 5.61, 13.61, 17.07, and 51.52% and the MDA content decreased by 14.65, 15.09, 14.80, 19.72, and 28.62%, respectively, under delayed sowing (Figure 4).
Figure 4. Effect of delayed sowing on antioxidant enzymes activity and MDA content of wheat flag leaves under heat stress at late grain-filling stage.
According to the OJIP curve of flag leaves at the grain-filling stage, the JIP-test curve before heat stress was not affected by delayed sowing, indicating that sowing date had little effect on the PSII donor and acceptor sides (Figures 5A,C). However, after heat stress, the JIP-test curve of the delayed sowing treatment was significantly lower than that of the normal sowing treatment at the J-step and I-step, indicating that the primary (QA)-to-secondary (QB) acceptor plastoquinone electron transfer process in the leaves of the normal sowing treatment was severely inhibited under heat stress (Figures 5B,D). Moreover, sowing date significantly affected the amount of active PSII reaction centers per cross section (RC/CSm), trapped energy flux per CSm (TRo/CSm), electron transport flux per CSm (ETo/CSm), and dissipated energy flux per RC (DIo/CSm) in winter wheat flag leaves. In 2017–2018 under heat stress, RC/CSm, TRo/CSm, and ETo/CSm increased by 3.95–43.93%, 2.73–10.65%, and 3.22–38.79%, respectively, while DIo/CSm decreased by 1.76–6.87% under delayed sowing compared to normal sowing; similarly, in 2018–2019, RC/CSm, TRo/CSm, and ETo/CSm increased by 4.27–47.54%, 2.94–9.30%, and 4.23–37.39%, while DIo/CSm decreased by 2.73–7.74% (Table 1).
Figure 5. JIP-test curve of relative variable fluorescence intensity before heat stress. (A,C) Are JIP-test curve of relatively variable fluorescence on the third day before heat stress in the 2017–2018 and 2018–2019 growing seasons, respectively. (B,D) Are JIP-test curve of relatively variable fluorescence on the third day after heat stress in the 2017–2018 and 2018–2019 growing seasons, respectively.
Table 1. Effect of delayed sowing on the energy fluxes per excited cross section of wheat flag leaves under heat stress at late grain–filling stage.
Based on the absorbed light energy distribution ratio, sowing date significantly affected the maximal photochemical efficiency of PSII (ΦPo), excitation efficiency of electron transport beyond QA (ψo), quantum yield for electron transfer (ΦEo), and quantum ratio for heat dissipation (ΦDo) (Table 2). In 2017–2018 under heat stress, ΦPo, ψo, and ΦEo increased by 1.34–6.25%, 1.05–32.51%, and 2.61–39.41%, respectively, while ΦDo decreased by 6.44–13.37% under delayed sowing compared to normal sowing; similarly, in 2018–2019, ΦPo, ψo, and ΦEo increased by 1.65–6.14%, 1.26–30.46%, and 2.50–37.14%, while ΦDo decreased by 6.53–14.65%. Thus, under heat stress, a higher proportion of energy in the flag leaves enters electron transfer under delayed sowing, which increases the energy available in photochemical reactions, whereas a higher proportion of energy in the flag leaves is used for heat dissipation under normal sowing.
Table 2. Effect of delayed sowing on the energy distribution proportion of wheat flag leaves under heat stress at late grain–filling stage.
To explore the impact of sowing date under heat stress at the late grain-filling stage in winter wheat, we analyzed normal and delayed sowing treatment groups (three independent biological replicates each) using iTRAQ labeling proteomics. We identified 5,896 proteins, of which 4,411 were quantified (Supplementary Figure 5A). The groups were compared according to a fold-change >1.500 (significant increase) or <0.667 (significant reduction), as well as Student’s t-test using P < 0.05 to indicate significance. In the delayed sowing group, we identified 56 upregulated proteins and 83 downregulated proteins (Supplementary Figure 5B); we subsequently screened these 139 differentially expressed proteins (DEPs; Supplementary Figures 5A,B).
According to UniProt1, Gene Ontology (GO)2, and the biological processes related to the proteins, DEPs were divided into seven categories: protein activity (36, 25.90%), metabolism (29, 20.86%), photosynthesis (20, 14.39%), stress response (15, 10.79%), cell structure (12, 8.63%), transportation (13, 9.35%), and other categories (Supplementary Figure 5C and Supplementary Table 2). Thus, sowing date impacts multiple biological processes in wheat leaves under heat stress.
According to functional classification, upregulated proteins tended to be related to photosynthesis and stress response, whereas downregulated proteins tended to be related to cell structure, protein activity, metabolism, and transportation. Among the proteins related to stress response, 1 protein related to heat stress was significantly upregulated (A0A3B6PNM7), and 5 proteins related to oxidation-reduction process were significantly upregulated, such as glutathione reductase (GR) (A0A3B6JM67). Since photosynthesis showed a significantly increased trend with physiological indicators, we focused on the analysis of DEPs related to photosynthesis (Supplementary Table 2).
We identified 20 DEPs related to photosynthesis, of which 12 were significantly upregulated and eight were significantly downregulated (Table 3). Thus, delayed sowing may have a mitigating effect on heat stress at the late grain-filling stage. Furthermore, seven DEPs were related to the synthesis of chlorophyll precursors, of which four were significantly downregulated and three were significantly upregulated (A0A3B6LW30, A0A3B6U5D2, and A0A3B5Z1L6). Ten DEPs were related to photosynthetic electron transport, of which six were significantly upregulated (S4Z3A1, A0A3B6NU25, A0A3B6QKC9, A0A3B6EN87, A0A3B6NW49, and P60162). Finally, three DEPs were related to the Calvin cycle, all of which were significantly upregulated (A0A3B6MXQ2, A0A3B6JP0, and ZA0A2).
Table 3. Photosynthesis-related proteins differentially expressed under heat stress at late wheat grain–filling stage.
To further explain the response of wheat flag leaves under heat stress to the two sowing date treatments, we conducted GO biological process and KEGG pathway enrichment analyses. Among biological processes, cellular component assembly, nucleosome assembly, and other proteins related to cell structure decreased significantly, whereas proteins related to photosynthesis increased significantly, mainly those related to photosynthetic electron transport (e.g., GO: 00159796) (Figure 6A). Thus, delaying sowing of wheat can increase leaf photosynthesis and reduce cell structure activity under heat stress by increasing photosynthetic electron transport. Moreover, KEGG pathways such as ribosomal protein, phenylpropanoid biosynthesis, and arginine and proline metabolism were significantly downregulated, whereas pathways related to photosynthesis were significantly upregulated (Figure 6B). Among photosynthetic metabolic pathways, we only observed upregulated proteins (PsbH, PsbR, and PetB); among them, PsbH and PsbR are related to PSII and PetB is related to the cytochrome b6f complex (Figure 7). These results further confirm that delaying sowing of wheat is beneficial to leaf photosynthesis under heat stress.
Figure 6. Enrichment analysis of differential protein. (A) GO biological process analysis. (B) KEGG pathway analysis. The bars represent –log10(P) where P represents the Fisher’ exact test P-values.
Figure 7. DEPs involvement photosynthetic were mapped in the metabolic pathway by KEGG analysis (red represents upregulated protein).
As less than 50% of tillers in winter wheat produces grain-bearing ears (Whaley et al., 2000), tiller mortality wastes a large amount of wheat yielding resources (Berry et al., 2003). Delayed sowing significantly reduced the tiller number before winter and the maximum tiller number before jointing, reducing the number of leaves (main stem) and improving the tiller survival percentage (Yin et al., 2018). Meanwhile, in a shorter crop cycle, the time dispersion between the oldest (base) leaf and the newest (top) leaf was less. Therefore, under the same cultural environmental conditions, delayed sowing optimizes the nitrogen content in leaves to increase photosynthetic rate (Yin et al., 2019). This is consistent with our study, compared with normal sowing, delayed sowing resulted in a significantly higher photosynthetic rate and grain weight during the grain-filling stage under heat stress, thus ensuring a significant increase in grain weight and yield under heat stress.
Cereal crop growth after flowering depend on carbon remobilization from stems and photosynthesis from flag leaf and spike (Simpson et al., 1983; Blum, 1985; Elizabete et al., 2017; Townsend et al., 2018). Photosynthesis is sensitive to heat stress, and its performance under adverse conditions can reflect the stress tolerance of plants (Chaves et al., 2003; Lu et al., 2017; Mahrookashani et al., 2017). Therefore, it suggests that such delayed sowing wheat can maintain a higher photosynthetic capacity under heat stress, indicating the physiological basis for the heat tolerance of wheat imparted by delayed sowing.
Light energy captured by the photosynthetic apparatus is mainly used for photosynthetic electron transfer, but a small portion is dissipated in the form of fluorescence and heat energy; these three components compete and restrict one another (Li et al., 2005). The J-step and I-step of the OJIP standardized curve are related to the redox states of QA and plastoquinone, respectively (Strasser, 1997). In this study, the J-step and I-step in the fast chlorophyll fluorescence kinetic curve were higher under normal sowing than delayed sowing, indicating that the QA–QB process of photosynthetic electron transfer in flag leaves was inhibited under heat stress with normal sowing. Previous studies also showed that heat stress influences electron transport, which damages the function of PS II (Chen et al., 2014; Bi et al., 2016; Fatma et al., 2021). And our research shows that RC/CSm, TRo/CSm, ETo/CSm, and DIo/CSm were lower under normal sowing than delayed sowing. The excess excitation energy of PSII increases, resulting in the production of a large number of reactive oxygen species (ROS), which damages the photosynthetic apparatus (Pavel, 2011; Takahashi and Badger, 2011). Our study shows that compared with the normal sowing, SOD, POD, CAT enzyme activity of winter wheat flag leaf to maintain at a high level, and reduce the MDA content under delayed sowing, that late sown with winter wheat flag leaf ROS removal ability is stronger, so as to keep the dynamic balance of its generation and removal, and reduce the level of membrane lipid peroxidation. Therefore, compared with normal sowing, delayed sowing ensured the energy transmission through enhancing the scavenging capacity of ROS in flag leaves, therefore increased the distribution of available energy in photochemistry and improved the assimilation capacity of photosynthetic system.
Studies have shown that, high temperature inhibits most proteins involved in chlorophyll synthesis, photosynthetic electron transport, and the Calvin cycle, thereby reducing photosynthesis in wheat leaves (Lu et al., 2017). Some researchers have speculated that heat adaptation before anthesis can effectively alleviate the photosynthetic and oxidative damage to wheat flag leaves from heat stress after anthesis (Wang et al., 2011), but a proteomics-based mechanism of action has not been reported. In our study, delayed sowing significantly upregulated the expression of GR protein under heat stress, while normal sowing significantly downregulated the expression of GR protein and D2 protein (PsbR). D1, D2 and oxygen-associated complex (OEC) proteins are sensitive sites in photosynthetic apparatus to environmental stress, most biological and abiotic stresses can inhibit their protein synthesis, resulting in protein degradation and ROS increase, leading to the intensification of photoinhibition (Besford et al., 1993; Takahashi and Murata, 2008; Shu et al., 2015). Therefore, we think that D1 and OEC proteins are also affected by ROS, leading to leaf senescence under normal sowing. Meanwhile, comparison of DEPs revealed that under heat stress, the sowing date affects many proteins related to photosynthesis. Compared with normal sowing, three proteins related to chlorophyll precursor synthesis, six proteins related to photosynthetic electron transfer, and three proteins related to the Calvin cycle were significantly increased in winter wheat leaves under delayed sowing. GO biological process and KEGG pathway enrichment analyses further revealed that proteins related to photosynthesis in winter wheat leaves increased significantly under delayed sowing. Therefore, delayed sowing upregulated the expression of psbR in D2 synthesis protein of wheat flag leaf, and protected D2 protein from heat stress by enhancing antioxidant capacity and stability of flag leaf membrane protein, thus delaying the senescence of wheat leaves and improving photosynthetic capacity under heat stress.
We mapped the DEPs in the metabolic pathway involved in photosynthesis in winter wheat leaves under delayed sowing using KEGG analysis. The protein contents of PsbH, PsbR, and PetB increased significantly. PsbR is an important part of the eukaryotic PSII, and is mainly involved in the oxidation reaction and electron transfer of PSII (Liu et al., 2009; Allahverdiyeva et al., 2013). PsbR stabilizes the PSII complex, affecting the properties of both the acceptor- and donor-side electron transfer reactions (Allahverdiyeva et al., 2007). Meanwhile, PsbH is a single transmembrane helix subunit necessary for the proper function of PSII and its stable assembly (D’Haene et al., 2015). A PsbH deletion mutant showed retardation of QA–QB electron transfer (Mayes et al., 1993), and the absence of PsbH has also been shown to decrease the efficiency of the PSII repair cycle and cause oxidative damage to PSII proteins (Komenda et al., 2002; Bergantino et al., 2003). At present, studies have shown that Roles of Potassium silicate (K2SiO3, 1.5 mM) and silicon dioxide nanoparticles (SiO2NPs, 1.66 mM) in improving thermotolerance of wheat photosynthetic machinery via upregulation of PsbH, PsbB, and PsbD Genes encoding PSII core Proteins (Hassan et al., 2021). From a proteomics perspective, delayed sowing significantly increases the proteins related to photosynthetic electron transport (PsbH, PsbR, and PetB) under heat stress, thereby affecting the process of photosynthetic electron transport.
Under heat stress during grain filling, delayed sowing date of winter wheat reduced heat dissipation by enhancing the scavenging capacity of ROS in flag leaves, and ensuring energy transmission along the photosynthetic electron transport chain; this increased the distribution ratio of available energy in photochemical reactions and maintained a high photosynthetic system assimilation capacity, which supported a high photosynthetic rate. Proteomic analysis revealed that differential accumulation of photosynthesis-related proteins, such as PsbH and PsbR, under delayed sowing in response to heat stress was coupled with physiological traits, confirming that delayed sowing can mediate tolerance to heat stress via improved photosynthetic electron transport to enhance the photosynthetic capacity. These findings provide a theoretical basis for the cultivation of heat tolerant winter wheat.
The datasets presented in this study can be found in online repositories. The names of the repository/repositories and accession number(s) can be found below: ProteomeXchange; PXD031871.
XD and MH designed the experiments and managed the projects. LF, JC, XZ, and SD performed the experiments. LF performed the data analysis and wrote the manuscript. All authors listed have approved the manuscript that is enclosed.
This work was supported by the National Key Research and Development Program of China (2016YFD0300403 and 2017YFD0201705), National Natural Science Foundation of China (31801298), and Funds of Shandong “Double Top” Program (SYL2017YSTD05).
The authors declare that the research was conducted in the absence of any commercial or financial relationships that could be construed as a potential conflict of interest.
All claims expressed in this article are solely those of the authors and do not necessarily represent those of their affiliated organizations, or those of the publisher, the editors and the reviewers. Any product that may be evaluated in this article, or claim that may be made by its manufacturer, is not guaranteed or endorsed by the publisher.
The Supplementary Material for this article can be found online at: https://www.frontiersin.org/articles/10.3389/fpls.2022.848464/full#supplementary-material
DEPs, differentially expressed proteins; OJIP, the chlorophyll a fluorescence transient; DAA, days after anthesis; RC/CSm, the amount of active PSII reaction centers per cross section; TRo/CSm, trapped energy flux per CSm; ETo/CSm, electron transport flux per CSm; DIo/CSm, dissipated energy flux per RC; Φ Po, the maximal photochemical efficiency of PSII; ψ o, excitation efficiency of electron transport beyond QA; Φ Eo, quantum yield for electron transfer; Φ Do, quantum ratio for heat dissipation; ROS, reactive oxygen species; SOD, superoxide dismutase; POD, peroxidase; CAT, catalase; MDA, malondialdehyde; GO, Gene Ontology; KEGG, Kyoto Encyclopedia of Genes and Genomes.
Abbas, G., Ahmad, S., Ahmad, A., Nasim, W., Fatima, Z., Hussain, S., et al. (2017). Quantification the impacts of climate change and crop management on phenology of maize–based cropping system in Punjab, Pakistan. Agric. Forest Meteorol. 47, 42–55. doi: 10.1016/j.agrformet.2017.07.012
Allahverdiyeva, Y., Mamedov, F., Suorsa, M., Styring, S., Vass, I., and Aro, E. M. (2007). Insights into the function of PsbR protein in Arabidopsis thaliana. Biochim. Biophys. Acta 1767, 677–685. doi: 10.1016/j.bbabio.2007.01.011
Allahverdiyeva, Y., Suorsa, M., Rossi, F., Pavesi, A., Kater, M. M., and Antonacci, A. (2013). Arabidopsis plants lacking PsbQ and PsbR subunits of the oxygen-evolving complex show altered PSII super-complex organization and short-term adaptive mechanisms. Plant J. 75, 671–684. doi: 10.1111/tpj.12230
Bergantino, E., Brunetta, A., Touloupakis, E., Segalla, A., Szabò, I., and Giacometti, G. M. (2003). Role of the PSII-H subunit in photoprotection-novel aspects of D1 turnover in Synechocystis 6803. J. Biol. Chem. 278, 41820–41829. doi: 10.1074/jbc.M303096200
Bergkamp, B., Impa, S. M., Asebedo, A. R., Fritz, A. K., and Jagadish, S. V. K. (2018). Prominent winter wheat varieties response to post-flowering heat stress under controlled chambers and field based heat tents. Field Crop Res. 222, 143–152. doi: 10.1016/j.fcr.2018.03.009
Berry, P. M., Spink, J. H., Foulkes, M. J., and Wade, A. (2003). Quantifying the contributions and losses of dry matter from non-surviving shoots in four cultivars of winter wheat. Field Crop. Res. 80, 111–121. doi: 10.1016/S0378-4290(02)00174-0
Besford, R. T., Richardson, C. M., Campos, J. L., and Tiburcio, A. F. (1993). Effect of polyamines on stabilization of molecular complexes in thylakoid membranes of osmotically stressed oat leaves. Planta 189, 201–206. doi: 10.1007/BF00195077
Bi, A., Fan, J., Hu, Z., Wang, G., Amombo, E., Fu, J., et al. (2016). Differential acclimation of enzymatic antioxidant metabolism and photosystem II photochemistry in tall fescue under drought and heat and the combined stresses. Front. Plant Sci. 7:453. doi: 10.3389/fpls.2016.00453
Blum, A. (1985). Photosynthesis and transpiration in leaves and ears of wheat and barley varieties. J. Exp. Bot. 36, 432–440. doi: 10.1093/jxb/36.3.432
Budak, H., Akpinar, B. A., Unver, T., and Turktas, M. (2013). Proteome changes in wild and modern wheat leaves upon drought stress by two-dimensional electrophoresis and nanoLC-ESI-MS/MS. Plant Mol. Biol. 83, 89–103. doi: 10.1007/s11103-013-0024-5
Chaves, M. M., Maroco, J. P., and Pereira, J. S. (2003). Understanding plant responses to drought from genes to the whole plant. Funct. Plant Biol. 30, 239–264. doi: 10.1071/fp02076
Chen, K., Sun, X., Amombo, E., Zhu, Q., Zhao, Z., Chen, L., et al. (2014). High correlation between thermotolerance and photosystem II activity in tall fescue. Photosynth. Res. 122, 305–314. doi: 10.1007/s11120-014-0035-3
Chen, Y., Zhang, Z., Tao, F., Palosuo, T., and Rötter, R. P. (2018). Impacts of heat stress on leaf area index and growth duration of winter wheat in the North China Plain. Field Crop Res. 222, 230–237. doi: 10.1016/j.fcr.2017.06.007
Dai, X. L., Zhou, X. H., Jia, D. Y., Xiao, L. L., Kong, H. B., and He, M. R. (2013). Managing the seeding rate to improve nitrogen-use efficiency of winter wheat. Field Crop Res. 154, 100–109. doi: 10.1016/j.fcr.2013.07.024
D’Haene, S. E., Sobotka, R., Bučinská, L., Dekker, J. P., and Komenda, J. (2015). Interaction of the PsbH subunit with a chlorophyll bound to histidine 114 of CP47 is responsible for the red 77K fluorescence of Photosystem II. Biochim. Biophys. Acta 10, 1327–1334. doi: 10.1016/j.bbabio.2015.07.003
Djanaguiraman, M., Boyle, D. L., Welti, R., Jagadish, S. V. K., and Prasad, P. V. V. (2018). Decreased photosynthetic rate under high temperature in wheat is due to lipid desaturation, oxidation, acylation, and damage of organelles. BMC Plant Biol. 18:55. doi: 10.1186/s12870-018-1263-z
Elizabete, C. S., John, A. P., Scales, J. C., Joanna, C. S., Steven, M. D., Andrew, M., et al. (2017). Phenotyping of field-grown wheat in the UK highlights contribution of light response of photosynthesis and flag leaf longevity to grain yield. J. Exp. Bot. 68, 3473–3486. doi: 10.1093/jxb/erx169
Fatma, M., Iqbal, N., Sehar, Z., Alyemeni, M. N., Kaushik, P., Khan, N. A., et al. (2021). Methyl Jasmonate protects the PS II system by maintaining the stability of chloroplast D1 protein and accelerating enzymatic antioxidants in heat-stressed wheat plants. Antioxidants 10:1216. doi: 10.3390/antiox10081216
Field, C., Barros, V., Dokken, D., and Mach, K. (2014). IPCC: Climate Change 2014: Impacts, Adaptation, and Vulnerability. Cambridge: Cambridge University Press, doi: 10.2134/jeq2008.0015br
Gautam, H., Fatma, M., Sehar, Z., Iqbal, N., Albaqami, M., and Khan, N. A. (2022). Exogenously-sourced ethylene positively modulates photosynthesis, carbohydrate metabolism, and antioxidant defense to enhance heat tolerance in rice. Int. J. Mol. Sci. 23:1031. doi: 10.3390/ijms23031031
Giannakaki, P., and Calanca, P. (2019). Russian winter and spring wheat productivity, heat stress and drought conditions at flowering, and the role of atmospheric blocking. Clim. Res. 78, 135–147. doi: 10.3354/cr01563
Hassan, H., Alatawi, A., Abdulmajeed, A., Emam, M., and Khattab, H. (2021). Roles of Si and SiNPs in improving thermotolerance of wheat photosynthetic machinery via upregulation of PsbH, PsbB and PsbD genes encoding PSII core proteins. Horticulturae 7:16. doi: 10.3390/horticulturae7020016
IPCC (2018). Global warming of 1.5°C. special report, intergovernmental panel on climate change James Retal (2017) characterizing half-a-degree difference: a review of methods for identifying regional climate responses to global warming. WIREs Clim. Change 8:e45.
Iqbal, N., Fatma, M., Gautam, H., Umar, S., Sofo, A., Dippolito, I., et al. (2021). The crosstalk of melatonin and hydrogen sulfide determines photosynthetic performance by regulation of carbohydrate metabolism in wheat under heat stress. Plants 10:1778. doi: 10.3390/plants10091778
Komenda, J., Lupínková, L., and Kopecký, J. (2002). Absence of the PsbH gene product destabilizes photosystem II complex and bicarbonate binding on its acceptor side in Synechocystis PCC 6803. Eur. J. Biochem. 269, 610–619. doi: 10.1046/j.0014-2956.2001.02693.x
Li, P. M., Gao, H. Y., and Strasser, R. J. (2005). Application of the fast chlorophyll fluorescence induction dynamics analysis in photosynthesis study. J. Plant Physiol. Mol. Biol. 31, 559–566. (In Chinese with English Abstract), doi: 10.1360/aps040074
Li, P., Cheng, L., Gao, H., Jiang, C., and Peng, T. (2009). Heterogeneous behavior of PSII in soybean (Glycine max) leaves with identical PSII photochemistry efficiency under different high temperature treatments. J. Plant Physiol. 166, 1607–1615. doi: 10.1016/j.jplph.2009.04.013
Lipec, J., Doussan, C., Nosalewicz, A., and Kondracka, K. (2013). Effect of drought and heat stresses on plant growth and yield: a review. Int. Agrophys. 27, 463–477. doi: 10.2478/intag-2013-0017
Liu, H., Frankel, L. K., and Bricker, T. M. (2009). Characterization and complementation of a PsbR mutant in Arabidopsis thaliana. Arch. Biochem. Biophys. 489, 34–40. doi: 10.1016/j.abb.2009.07.014
Liu, Y., Chen, Q., Ge, Q., Dai, J., Qin, Y., and Dai, L. (2018). Modelling the impacts of climate change and crop management on phenological trends of spring and winter wheat in China. Agr. Forest. Meteorol. 248, 518–526. doi: 10.1016/j.agrformet.2017.09.008
Lu, Y. Z., Li, R. Q., Wang, R. C., Wang, X. M., Zheng, W. J., Sun, Q. X., et al. (2017). Comparative proteomic analysis of flag leaves reveals new insight into wheat heat adaptation. Front. Plant Sci. 8:1086. doi: 10.3389/fpls.2017.01086
Mahrookashani, A., Siebert, S., Hüging, H., and Ewert, F. (2017). Independent and combined effects of high temperature and drought stress around anthesis on wheat. J. Agron. Crop Sci. 203, 453–463. doi: 10.1111/jac.12218
Mathur, S., Agrawal, D., and Jajoo, A. (2014). Photosynthesis: response to high temperature stress. J. Photochem. Photobiol. B 137, 116–126. doi: 10.1016/j.jphotobiol.2014.01.010
Mayes, S. R., Dubbs, J. M., Vass, I., Hideg, É, Nagy, L., and Barber, J. (1993). Further characterization of the PsbH locus of synechocystis sp. PCC 6803: inactivation of PsbH impairs QA to QB electron transport in photosystem 2. Biochemistry 32, 1454–1465. doi: 10.1021/bi00057a008
Mirosavljevi, M., Miki, S., Upunski, V., Pika, A. K., Trkulja, D., and Ottosen, C. O. (2021). Effects of high temperature during anthesis and grain filling on physiological characteristics of winter wheat cultivars. J. Agron. Crop Sci. 207, 823–832. doi: 10.1111/jac.12546
Narayanan, S., Prasad, P. V. V., Fritz, A. K., Boyle, D. L., and Gill, B. S. (2015). Impact of high night–time and high daytime temperature stress on winter wheat. J. Agron. Crop. Sci. 201, 206–218. doi: 10.1111/jac.12101
Öz, M. T., Turan, Ö, Kayihan, C., Eyidoğan, F., Ekmekçi, Y., Yücel, M., et al. (2014). Evaluation of photosynthetic performance of wheat cultivars exposed to boron toxicity by the JIP fluorescence test. Photosynthetica 52, 555–563. doi: 10.1007/s11099-014-0065-2
Pan, J., Lin, S., and Woodbury, N. W. (2012). Bacteriochlorophyll excited-state quenching pathways in bacterial reaction centers with the primary donor oxidized. J. Phys. Chem. B 116, 2014–2022. doi: 10.1021/jp212441b
Pavel, P. (2011). Molecular mechanisms of production and scavenging of reactive oxygen species by photosystem II. Biochim. Biophys. Acta 1817, 218–231. doi: 10.1016/j.bbabio.2011.05.017
Prasad, P. V. V., Pisipati, S. R., Ristic, Z., Bukovnik, U., and Fritz, A. K. (2008). Impact of nighttime temperature on physiology and growth of spring wheat. Crop Sci. 48, 2372–2380. doi: 10.2135/cropsci2007.12.0717
Rasul, G., Mahmood, A., Sadiq, A., and Khan, S. I. (2012). Vulnerability of the Indus delta to climate change in Pakistan. Pak. J. Meteorol. 8, 89–107.
Ren, G., Guan, Z., Shao, X., and Gong, D. Y. (2011). Changes in climatic extremes over mainland China. Clim. Res. 50, 105–111. doi: 10.3354/cr01067
Ristic, Z., Bukovnik, U., and Prasad, P. V. V. (2007). Correlation between heat stability of thylakoid membranes and loss of chlorophyll in winter wheat under heat stress. Crop Sci. 47, 2067–2073. doi: 10.2135/cropsci2006.10.0674
Rivelli, G. M., Fernández Long, M. E., Abeledo, L. G., Calderini, D. F., and Rondanini, D. P. (2021). Assessment of heat stress and cloudiness probabilities in post-flowering of spring wheat and canola in the Southern Cone of South America. Theor. Appl. Climatol. 145, 1485–1502. doi: 10.1007/s00704-021-03694-x
Sarkar, S., Islam, A. K. M. A., Barma, N. C. D., and Ahmed, J. U. (2021). Tolerance mechanisms for breeding wheat against heat stress: a review. S. Afr. J. Bot. 138, 262–277. doi: 10.1016/j.sajb.2021.01.003
Shew, A. M., Tack, J. B., Nalley, L. L., and Chaminuka, P. (2020). Yield reduction under climate warming varies among wheat cultivars in South Africa. Nat. Commun. 11:4408. doi: 10.1038/s41467-020-18317-8
Shu, S., Yuan, Y., Chen, J., Sun, J., and Guo, S. (2015). The role of putrescine in the regulation of proteins and fatty acids of thylakoid membranes under salt stress. Sci. Rep. 5:14390. doi: 10.1038/srep14390
Simpson, R. J., Lambers, H., and Dalling, M. J. (1983). Nitrogen redistribution during grain growth in wheat (Triticum aestivum L.): IV. Development of a quantitative model of the translocation of nitrogen to the grain. Plant Physiol. 71, 7–14. doi: 10.1007/BF00384560
Strasser, B. J. (1997). Donor side capacity of photosystem II probed by chlorophyll a fluorescence transients. Photosynth. Res. 52, 147–155. doi: 10.1023/A:1005896029778
Sun, H. Y., Zhang, X. Y., Chen, S. Y., Pei, D., and Liu, C. M. (2007). Effects of harvest and sowing time on the performance of the rotation of winter wheat-summer maize in the North China Plain. Ind. Crop. Prod. 25, 239–247. doi: 10.1016/j.indcrop.2006.12.003
Tack, J., Barkley, A., and Nalley, L. L. (2015). Effect of warming temperatures on US wheat yields. Proc. Natl. Acad. Sci. U.S.A. 112, 6931–6936. doi: 10.1073/pnas.1415181112
Takahashi, S., and Badger, M. R. (2011). Photoprotection in plants: a new light on photosystem II damage. Trends Plant Sci. 16, 53–60. doi: 10.1016/j.tplants.2010.10.001
Takahashi, S., and Murata, N. (2008). How do environmental stresses accelerate photoinhibition? Trends Plant Sci. 13, 178–182. doi: 10.1016/j.tplants.2008.01.005
Townsend, A. J., Retkute, R., Chinnathambi, K., Randall, J. W., Foulkes, J., and Carmo-Silva, E. (2018). Suboptimal acclimation of photosynthesis to light in wheat canopies. Plant Physiol. 176, 1233–1246. doi: 10.1104/pp.17.01213
Wang, X., Cai, J., Jiang, D., Liu, F. L., Dai, T. B., and Cao, W. X. (2011). Pre-anthesis high-temperature acclimation alleviates damage to the flag leaf caused by post-anthesis heat stress in wheat. Plant Physiol. 168, 585–593. doi: 10.1016/j.jplph.2010.09.016
Wang, X., Dinler, B. S., Vignjevic, M., Jacobsen, S., and Wollenweber, B. (2015). Physiological and proteome studies of responses to heat stress during grain filling in contrasting wheat cultivars. Plant Sci. 230, 33–50. doi: 10.1016/j.plantsci.2014.10.009
Whaley, J. M., Sparkes, D. L., Foulkes, M. J., Spink, J. H., Semere, T., and Scott, R. K. (2000). The physiological response of winter wheat to relations in plant density. Ann. Appl. Biol. 137, 167–177. doi: 10.1111/j.1744-7348.2000.tb00048.x
Xiao, D., Moiwo, J. P., Tao, F., Yang, Y., Shen, Y., Xu, Q., et al. (2015). Spatiotemporal variability of winter wheat phenology in response to weather and climate variability in China. Mitig Adapt Strateg Glob Change 20, 1191–1202. doi: 10.1007/s11027-013-9531-6
Xiao, D., Tao, F., Liu, Y., Shi, W., Wang, M., Liu, F., et al. (2013). Observed changes in winter wheat phenology in the north china plain for 1981–2009. Int. J. Biometeorol. 57, 275–285. doi: 10.1007/s00484-012-0552-8
Yin, L. J., Dai, X. L., and He, M. R. (2018). Delayed sowing improves nitrogen utilization efficiency in winter wheat without impacting yield. Field Crop. Res. 221, 90–97. doi: 10.1016/j.fcr.2018.02.015
Yin, L. J., Xu, H. C., Dong, S. X., Dai, X. L., and He, M. R. (2019). Optimised nitrogen allocation favours improvement in canopy photosynthetic nitrogen-use efficiency: evidence from late-sown winter wheat. Environ. Exp. Bot. 159, 75–86. doi: 10.1016/j.envexpbot.2018.12.013
Zhao, H., Dai, T. B., Jing, Q., Jiang, D., and Cao, W. X. (2007). Leaf senescence and grain filling affected by post-anthesis heats in two different wheat cultivars. Plant Growth Regul. 51, 149–158. doi: 10.1007/s10725-006-9157-8
Zhao, S. J., Xu, C. C., and Zou, Q. (1994). Improvements of method for measurement of malondialdehvde in plant tissues. Plant Physiol. Commun. 3, 207–210. (In Chinese with English Abstract),
Keywords: heat stress, photosynthetic capacity, proteomics, wheat, delayed sowing
Citation: Fei L, Chu J, Zhang X, Dong S, Dai X and He M (2022) Physiological and Proteomic Analyses Indicate Delayed Sowing Improves Photosynthetic Capacity in Wheat Flag Leaves Under Heat Stress. Front. Plant Sci. 13:848464. doi: 10.3389/fpls.2022.848464
Received: 04 January 2022; Accepted: 28 February 2022;
Published: 24 March 2022.
Edited by:
Michael Moustakas, Aristotle University of Thessaloniki, GreeceReviewed by:
Nafees A. Khan, Aligarh Muslim University, IndiaCopyright © 2022 Fei, Chu, Zhang, Dong, Dai and He. This is an open-access article distributed under the terms of the Creative Commons Attribution License (CC BY). The use, distribution or reproduction in other forums is permitted, provided the original author(s) and the copyright owner(s) are credited and that the original publication in this journal is cited, in accordance with accepted academic practice. No use, distribution or reproduction is permitted which does not comply with these terms.
*Correspondence: Xinglong Dai, YWRhaXNkbnlAMTYzLmNvbQ==; Mingrong He, bXJoZUBzZGF1LmVkdS5jbg==
Disclaimer: All claims expressed in this article are solely those of the authors and do not necessarily represent those of their affiliated organizations, or those of the publisher, the editors and the reviewers. Any product that may be evaluated in this article or claim that may be made by its manufacturer is not guaranteed or endorsed by the publisher.
Research integrity at Frontiers
Learn more about the work of our research integrity team to safeguard the quality of each article we publish.