- 1College of Horticulture and Plant Protection, Yangzhou University, Yangzhou, China
- 2Department of Floriculture, Faculty of Agriculture, Alexandria University, Alexandria, Egypt
Melatonin is a multi-functional molecule that is ubiquitous in all living organisms. Melatonin performs essential roles in plant stress tolerance; its application can reduce the harmful effects of abiotic stresses. Plant melatonin biosynthesis, which usually occurs within chloroplasts, and its related metabolic pathways have been extensively characterized. Melatonin regulates plant stress responses by directly inhibiting the accumulation of reactive oxygen and nitrogen species, and by indirectly affecting stress response pathways. In this review, we summarize recent research concerning melatonin biosynthesis, metabolism, and antioxidation; we focus on melatonin-mediated tolerance to abiotic stresses including drought, waterlogging, salt, heat, cold, heavy metal toxicity, light and others. We also examine exogenous melatonin treatment in plants under abiotic stress. Finally, we discuss future perspectives in melatonin research and its applications in plants.
Introduction
Plants encounter various environmental stresses throughout their lives. As sessile organisms, plants have evolved multiple response mechanisms to cope with adverse conditions, thus ensuring their survival and reproductive success. N-acetyl-5-methoxytryptamine (melatonin), an indolic compound derived from tryptophan, is a universal abiotic stress regulator in plants (Wang et al., 2018). Melatonin was first identified in the bovine pineal gland in 1958 (Lerner et al., 1958). It was later characterized as an essential animal hormone that is involved in multiple biological processes, including antioxidation, circadian rhythms, seasonal reproduction, sleep, sexual behavior, mood, temperature homeostasis, retina physiology, and immunological enhancement (Lerner et al., 1958; Shi et al., 2015b). In 1995, melatonin was first identified in plants (Dubbels et al., 1995; Hattori et al., 1995). Since then, melatonin has been shown to participate in various abiotic stress responses as a pleiotropic signaling molecule. In addition, it is an efficient scavenger of both reactive oxygen species (ROS) and reactive nitrogen species (RNS; Arnao and Hernández-Ruiz, 2019b). Melatonin is present in the leaves, roots, stems, petals, flower buds, fruits and seeds of various plant species. Its biosynthesis typically occurs in chloroplasts and mitochondria (Tan and Reiter, 2020).
Since the discovery of melatonin, numerous studies have been conducted to examine its functions in plants, thus revealing its protective roles against both biotic and abiotic stresses. In adverse environments, melatonin regulates plant growth and development by promoting seed germination, boosting lateral root generation, controlling flowering time and delaying leaf senescence (Arnao and Hernández-Ruiz, 2019a, 2020). Melatonin acts as a direct regulator by scavenging ROS and RNS. It also acts as an indirect regulator by regulating gene expression via stress-responsive transcription factors. Melatonin also functions as an auxin-like regulator, sharing a precursor molecule with auxin. It can mimic auxin activity, acting upstream of the auxin pathway to alter the expression profiles of various auxin-related transcription factors (e.g., WRKY, NAC, MYB, bHLH, and HD-ZIP family transcription factors; Liang et al., 2017; Tan and Reiter, 2020). Melatonin may also interact with other plant hormones such as indoleacetic acid (IAA), gibberellic acid (GA), cytokinin (CK), abscisic acid (ABA), ethylene (ET), salicylic acid (SA), jasmonic acid (JA), brassinosteroid (BR), strigolactones, and polyamines (Arnao and Hernández-Ruiz, 2020). Moreover, the exogenous application of melatonin can enhance plant tolerance (e.g., to drought, salt, heat, cold, waterlogging, and heavy metal toxicity) by modulating the biosynthesis of endogenous melatonin and the activities of antioxidative enzymes (Weeda et al., 2014; Zhang et al., 2015; Moustafa-Farag et al., 2020; Sun et al., 2021).
Considering its protective functions, melatonin has attracted increasing research attention in recent years because of the increasing harmful effects of climate change, soil salinization, and industrial pollution on agriculture, crop production, and food chain security. Over the past decade, considerable progress has been made in the general understanding of melatonin in plants. Therefore, an updated review highlighting recent findings in melatonin-mediated abiotic stress tolerance is needed. In this review, we have summarized the biosynthetic and catabolic pathways of melatonin. We have also focused on the defensive roles of melatonin against various abiotic stresses, as well as its applications for improving plant stress tolerance.
Biosynthesis and Metabolism of Phytomelatonin
Biosynthetic Pathway
Melatonin, which is ubiquitous among most plant species, has a low molecular weight and a stable structure (Wu Y. et al., 2021). Its biosynthetic pathways in model animals and plants have been elucidated. In animals, four main enzymes participate in the classic melatonin biosynthetic pathway. First, tryptophan hydroxylase converts tryptophan into 5-hydroxytryptophan, which is then decarboxylated by aromatic amino acid decarboxylase to form 5-hydroxytryptamine (serotonin). Next, arylalkylamine N-acetyltransferase, also known as serotonin N-acetyltransferase (SNAT), acetylates serotonin to form N-acetyl-5-hydroxytryptamine (N-acetylserotonin). Finally, hydroxyindole-O-methyltransferase, also known as N-acetylserotonin methyltransferase (ASMT), O-methylates N-acetyl-5-hydroxytryptamine to generate melatonin (Axelrod and Weissbach, 1960; Weissbach et al., 1960; Tan et al., 2016).
The melatonin biosynthetic pathway in plants differs from the pathway in animals. It involves at least six biosynthetic enzymes including tryptophan decarboxylase (TDC), tryptophan hydroxylase, tryptamine 5-hydroxylase (T5H), SNAT, ASMT, and caffeic acid O-methyltransferase (COMT; Figure 1; Back et al., 2016; Sun et al., 2021). Among them, SNAT is a key rate-limiting enzyme (Liao et al., 2021). SNAT plant knockout strains exhibit altered phenotypes and become more sensitive to some abiotic stresses (Lee et al., 2019). ASMT and COMT were likely involved in plant terrestrialization. COMT first appeared in bryophytes and is presume to have evolved from ASMT. The increased activity of COMT compared to ASMT may have enhanced melatonin production in plants. In higher plants, COMT acquired an additional function in lignin biosynthesis (Zhao et al., 2021). Moreover, other hormonal pathways influence the expression of key melatonin biosynthesis genes. For example, ET insensitive protein 3 (CcEIN3) activates the expression of CcTDC and CcASMT1 in hickory (Chen W. et al., 2021).
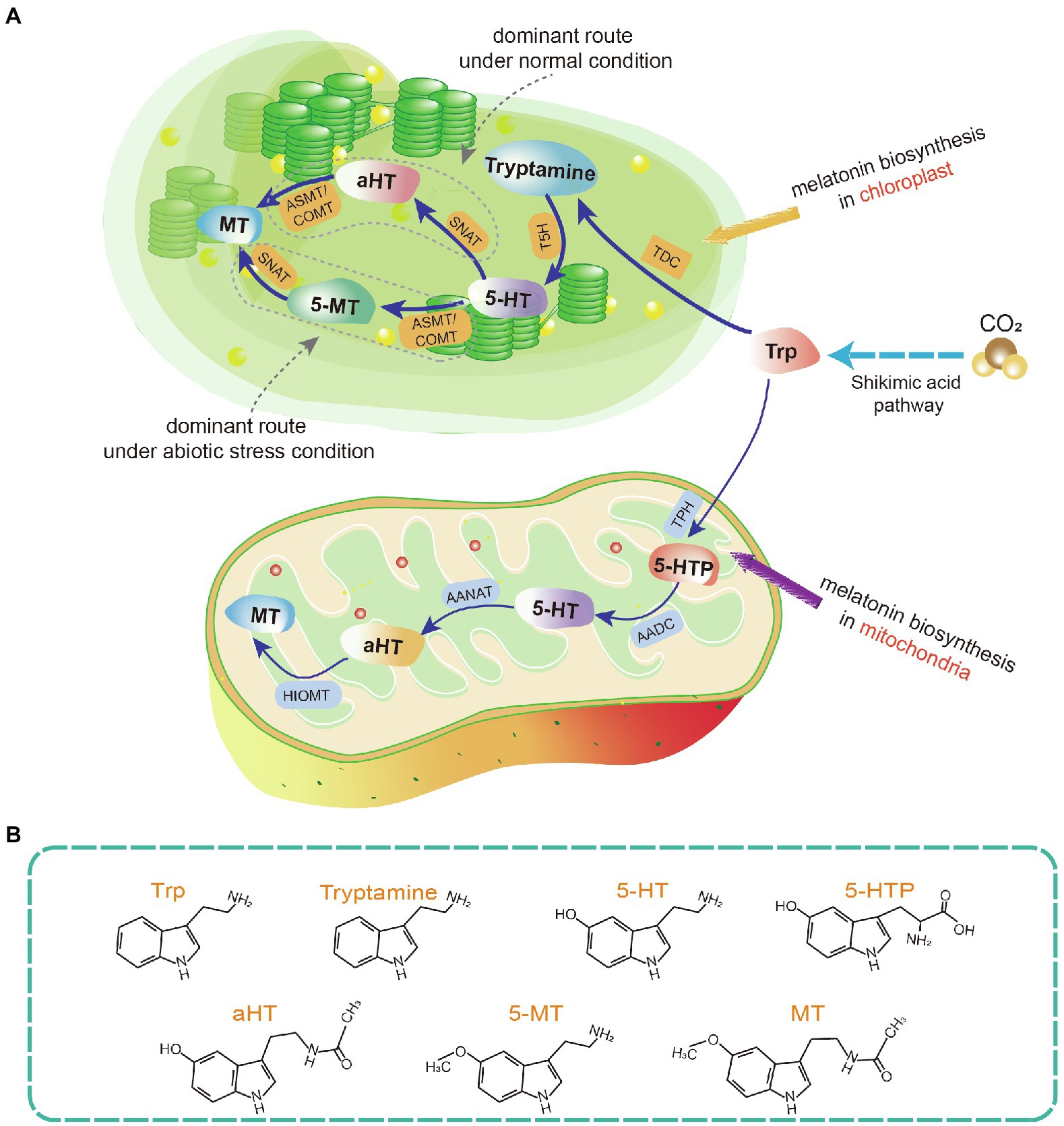
Figure 1. Phytomelatonin biosynthetic pathway in chloroplasts and mitochondria. (A) Schematic of melatonin biosynthetic pathways. (B) Molecular structures of key intermediate products in the melatonin biosynthetic pathway. Plants usually synthesize melatonin in chloroplasts under normal conditions. If this pathway is blocked, melatonin biosynthesis may be switched to the mitochondria. Trp, tryptophan; 5-HT, 5-hydroxytryptamine; 5-HTP, 5-hydroxytryptophan; aHT, N-acetyl-5-hydroxytryptamine; 5-MT, 5-methoxytryptamine; MT, melatonin; TDC, tryptophan decarboxylase; T5H, tryptamine 5-hydroxylase; SNAT, serotonin N-acetyltransferase; ASMT, N-acetylserotonin-O-methyltransferase (plant type SNATs and ASMTs appear to have origins distinct from the origins in animals); COMT, caffeic acid O-methyltransferase; TPH, tryptophan hydroxylase; AADC, aromatic amino acid decarboxylase; AANAT, arylalkylamine N-acetyltransferase (also known as arylamine N-acetyltransferase); and HIOMT, hydroxyindole-O-methyltransferase (also known as N-acetylserotonin O-methyltransferase).
The first two biosynthesis steps in the plant melatonin pathway are the reverse of the first two steps in animals. In plants, tryptophan is decarboxylated by TDC in the first step, after which tryptamine is hydroxylated by T5H (Tan et al., 2016). SNAT and ASMT/COMT catalyze the final two biosynthesis steps, the order of which alternates depending on the environmental conditions (Byeon et al., 2015a). Under abiotic stress, the expression of different ASMT isoforms is induced, so serotonin is first O-methylated to form 5-methoxytryptamine by ASMT, and then acetylated to form melatonin (Ye et al., 2019; Tan and Reiter, 2020). In contrast, under standard conditions, the dominant pathway involves serotonin acetylation to form N-acetyl-5-hydroxytryptamine and then O-methylation to form melatonin (Ye et al., 2019; Figure 1).
In animals, melatonin is biosynthesized in the pineal gland. The specific organs in which melatonin is synthesized in plants are currently unknown. However, melatonin can be transported from roots to shoots as a long-distance signal in some plant species (Mukherjee et al., 2014; Li H. et al., 2017). The cellular location of melatonin biosynthesis in plants is clear. Tan et al. (2013) hypothesized that mitochondria and chloroplasts are the sites of melatonin biosynthesis in plants. The key melatonin synthetase, SNAT, is mainly localized to the chloroplasts in plants such as rice, cucumber, and tomato (Byeon et al., 2014; Wang et al., 2020). COMT and ASMT can be highly expressed in the chloroplast and their overexpression results in enhanced melatonin production (Choi et al., 2017). Taken together, this evidence suggests that the chloroplast may be the main site of melatonin biosynthesis. In addition to chloroplasts, mitochondria are regarded as sites of melatonin biosynthesis. The apple MzSNAT5 gene, which is localized to mitochondria, is more closely related to animal SNAT than to the chloroplast-localized MzSNAT9 or SNATs present in rice, Arabidopsis, or cyanobacteria (Wang et al., 2017; Tan and Reiter, 2020).
Mitochondria and chloroplasts are the biosynthetic sites of melatonin because of their evolutionary histories Hardeland, 2019. Melatonin is produced by several bacterial taxa, including cyanobacteria and α-proteobacteria. Mitochondria evolved from ingested α-proteobacteria, while chloroplasts originated from photosynthetic cyanobacteria. Throughout evolution, both organelles likely retained their ability to produce melatonin (Zhao et al., 2019). Moreover, there is evidence to suggest that plants preferentially perform melatonin biosynthesis in chloroplasts under normal conditions. When the chloroplast pathway is blocked, melatonin biosynthesis takes place in the mitochondria (Tan and Reiter, 2020; Figure 1).
Metabolic Pathway
Melatonin can be degraded via enzymatic and non-enzymatic transformation routes (Tan et al., 2012; Hardeland, 2015; Back, 2021). In the enzymatic transformation route of animals, melatonin is metabolized into 6-hydroxymelatonin (6-OHM) by P450 enzymes. 6-OHM is then sulfated into 6-sulfatoxymelatonin and N1-acetyl-N2-formyl-5-methoxykynuramine (AFMK) by several enzymes (e.g., indoleamine 2,3-dioxygenase; Ma et al., 2005; Semak et al., 2005; Hardeland, 2017). 6-OHM is the major product of enzymatic melatonin degradation in animals. In non-enzymatic transformation routes, melatonin is degraded by free radicals or other oxidants. Examples of melatonin metabolites include cyclic 3-OHM (c3-OHM), 4-OHM, 2-OHM, AFMK, and N-acetyl-5-methoxykynuramine, which are produced non-enzymatically through interactions with ROS and RNS (Hardeland, 2017; Back, 2021).
The enzymatic degradation of melatonin in plants shares some common features with such degradation in vertebrates and other eukaryotes (Sun et al., 2021). For example, indoleamine 2,3-dioxygenase, which converts melatonin to AFMK, was identified in water hyacinth (Eichhornia crassipes) and other phototrophs such as phaeophytes, dinoflagellates, and chlorophyceae (Tan et al., 2007; Hardeland et al., 2009; Okazaki et al., 2010; Hardeland, 2015). Similarly, 2-OHM and c3-OHM are two melatonin hydroxylation metabolites present in the plant metabolic pathway. They are generated by melatonin 2-hydroxylase (M2H) and melatonin 3-hydroxylase (M3H), respectively (Byeon and Back, 2015; Byeon et al., 2015c; Lee et al., 2016; Choi and Back, 2019). Notably, M2H-1, a 2-oxoglutarate-dependent dioxygenase (2-ODD) isogene in rice, is localized to chloroplasts and has a major role in 2-OHM biosynthesis. Other 2-ODD isogenes with moderate M2H activity are expressed in the cytoplasm. The M2H pathway is the most important catabolic pathway, with crucial roles in modulating physiological and biochemical processes under biotic and abiotic stresses (Byeon et al., 2015b; Sun et al., 2021). There is evidence that endogenously triggered 2-OHM production, as well as exogenously applied 2-OHM, can improve plant tolerance to various adverse stresses such as cold, drought, metal stress, and pathogen attack (Lee and Back, 2016a; Shah et al., 2020; Tan and Reiter, 2020).
The gene responsible for producing c3-OHM, another important melatonin hydroxylation metabolite, has been cloned in rice (Lee et al., 2016). c3-OHM production is catalyzed by M3H, which belongs to the 2-ODD superfamily. It is located in the cytoplasm. Of note, c3-OHM levels exhibit a diurnal rhythm in rice (Choi and Back, 2019). Additionally, M2H and M3H are endemic to terrestrial plants, suggesting that the functions of their enzymatic products are specific to land plants (Lee and Back, 2019). Generally, c3-OHM and 2-OHM are the predominant hydroxylated forms of melatonin found in plants, in contrast to the 6-OHM mainly found in animals. Moreover, 5-methoxytryptamine is another bioactive metabolite. It is formed from melatonin by N-acetylserotonin deacetylase (ASDAC) and from serotonin by ASMT in rice seedlings (Back, 2021). For non-enzymatic melatonin degradation, melatonin can be nitrosated at the nitrogen atom of its indole ring, resulting in the formation of N-nitrosomelatonin (Blanchard et al., 2000; Mukherjee, 2019). The melatonin metabolites beta-hydroxymelatonin, cyclic beta-hydroxymelatonin, and cyclic melatonin are also present in corn and cucumber. They may be produced because of interactions between melatonin and oxidizing agents such as ROS and RNS (Kołodziejczyk et al., 2015; Manchester et al., 2015; Figure 2).
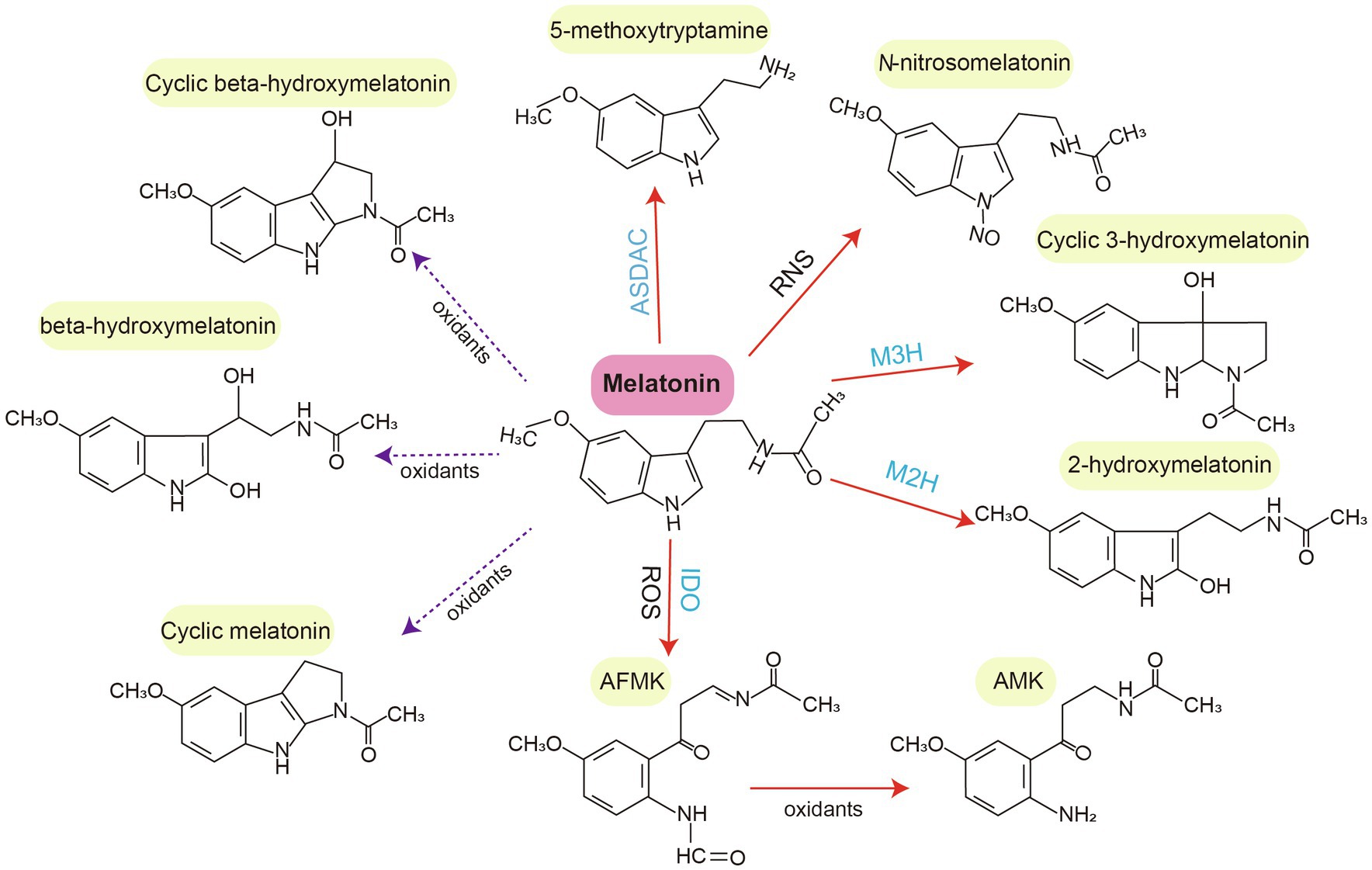
Figure 2. Degradation (catabolism) of melatonin in plants. Melatonin is typically degraded into various metabolites through enzymatic (M3H, M2H, ASDAC, and IDO) and non-enzymatic (oxidants, ROS, and RNS) transformation routes. Red solid arrows indicate confirmed melatonin metabolites. Purple broken arrows indicate potential melatonin metabolites. AFMK, N1-acetyl-N2-formyl-5-methoxykynuramine; AMK, N-acetyl-5-methoxykynuramine; IDO, indoleamine 2,3-dioxygenase; M2H, melatonin 2-hydroxylase; M3H, melatonin 3-hydroxylase; and ASDAC, N-acetylserotonin deacetylase.
Melatonin coexists with its major metabolites (3-OHM, 2-OHM, and 5-methoxytryptamine) in plant cells (Lee and Back, 2019). Both endogenous melatonin and its metabolisms may be affected by exogenous melatonin (Back, 2021). After exogenous melatonin application in plants, the resulting physiological and biochemical changes and stress responses may differ depending on the changed level of melatonin metabolites and endogenous melatonin (Lee and Back, 2016a). Endogenous levels of melatonin metabolites are generally high in plants, where they exhibit high antioxidant activity.
Many melatonin-mediated abiotic stress responses (e.g., to drought, salt, and heat) and physiological process (e.g., flowering, senescence, somatic embryogenesis, sugar metabolism and secondary metabolism) are related to the mitogen-activated protein kinase (MAPK) cascade pathway, melatonin metabolism (Erland et al., 2018; Kobylińska et al., 2018; Qi et al., 2018; Huang et al., 2019; Liu et al., 2019; Zhang H. et al., 2019; Back, 2021), and other more complicated signaling pathways (hormones, ROS, and Ca2+; Arnao and Hernández-Ruiz, 2018; Zhang Y. et al., 2021). Melatonin metabolites can reduce oxidative damage by balancing the redox state (Galano and Reiter, 2018; Yu et al., 2018). However, the signaling pathways of melatonin metabolites in plants have not been fully elucidated.
Melatonin Functions as an Antioxidant in Plants
Melatonin as a ROS and RNS Scavenger
Reactive oxygen species are byproducts in plant aerobic metabolic processes (Apel and Hirt, 2004). ROS consist of radical and non-radical oxygen species. They may be as simple as molecules of superoxide or more complex molecules, like hydrogen peroxide (H2O2) and hydroxyl radical (OḤ; Ray et al., 2012). In plants, H2O2, O2•−, OḤ, and 1O2 are the most common ROS. They are mainly generated in chloroplasts, mitochondria, and peroxisomes under stresses. In chloroplasts, ROS generation depends on the interaction of chlorophyll (chl) and light (Hasanuzzaman et al., 2020). The light energy is captured in photosystem II (PSII) to cause photosynthetic electron transfer. During light-driven photosynthetic electron transport, highly reactive singlet oxygen (1O2) is formed by transferring the absorbed energy to ground-state oxygen of O2 (Li and Kim, 2021). 3Chl and 3P680 are involved in this process. In addition, O2•− is produced in photosystem I (PSI) by Mehler reaction. O2•− can be transferred to H2O2 and O2 by SOD, thus generating OḤ via the interaction of H2O2 with transition metal ions (Fe2+ and Cu+; Hasanuzzaman et al., 2020; Li and Kim, 2021). In mitochondria, Complex I and Complex III are two components of the mitochondrial electron transport chain (mtETC) to covert O2 to O2•−. Then, Mn-SOD and Cu-Zn-SOD catalyze O2•− to H2O2 (Huang et al., 2016; Hasanuzzaman et al., 2020). In addition, oxidative metabolism also exits in peroxisomes. A number of enzymes are implicated in active metabolism of ROS in peroxisomes such as glycolate oxidase, xanthine oxidase and NADPH oxidase (Del Río and López-Huertas, 2016; Kerchev et al., 2016). Peroxisome is also one of the main cellular sites of NO production that exerts a regulatory function of ROS metabolism.
Damage caused by environmental stress in plants typically occurs because of oxidant stress, which manifests as a loss of equilibrium between oxidant and antioxidant chemical species in the cell, thus altering redox homeostasis and accelerating ROS accumulation (Nguyen et al., 2018). ROS can cause oxidative modification of proteins in chloroplasts under stress conditions. For example, excess 1O2 is reported to oxidize PSII core proteins, polyunsaturated fatty acids (PUFAs) and some specific amino acid residues like tryptophan (Trp), tyrosine (Tyr), phenylalanine (Phe), histidine (His), methionine (Met), and cysteine (Cys; Li and Kim, 2021). O2•− and OḤ can oxidize tyrosine (Y) 246 in the D1 protein (Kumar et al., 2021). α-tocopherol plays a significant role in preventing oxidative modification of the D1-Y246 residue (Li and Kim, 2021). 1O2 can also oxidize a disulfide bond (DSB), thus forming a disulfide crosslink with thiol-containing proteins (Jiang et al., 2021; Li and Kim, 2021). In addition, ROS are utilized as signaling agents by plants and trigger a series of responses that protect the plant against stress factors (Nabi et al., 2019). Among ROS signaling pathways, operational retrograde signaling (ORS) pathways are generally associated with stress. ORS pathways can be mediated by β-cyclocitra (βCC), 3′ (2′)-phosphoadenosine-5′-phosphate (PAP), methylerythritol cyclodiphosphate (MEcPP), and EXECUTER1 (EX1; Kim, 2020; Li and Kim, 2021). Moreover, various hormones (JA, SA, ABA) participate in the crosstalk with ROS to active ROS detoxification under environmental stresses (Lemos et al., 2016; Lv et al., 2019; Postiglione and Muday, 2020; Wang Y. et al., 2021). In addition to hormones, trehalose and anthocyanins are also reported to regulate ROS metabolism (Naing and Kim, 2021; Yang et al., 2022).
NO is an important molecular signal and regulates ROS metabolism (Corpas et al., 2019). Interactions between nitric oxide (NO) and ROS induce radical and non-radical RNS generation under unfavorable conditions. This process is known as nitrosative stress (Corpas and Barroso, 2013; Saddhe et al., 2019). RNS include nitroxyl anion (NO−), nitrosonium cation (NO+), higher oxides of nitrogen (NO, NO2 and higher valence), S-nitrosothiols, and dinitrosyl iron complexes (DNICs; Martínez and Andriantsitohaina, 2009). RNS modify protein activity via nitrosylation and interactions between NO and other biomolecules (ferrous heme and catalase; Arora et al., 2016). Moreover, peroxynitrite (ONOO-) and S-nitrosoglutathione (GSNO) derived from NO can modulate the ROS metabolism of peroxisomes through protein posttranslational modifications (Corpas et al., 2019). RNS can also interact with various signaling cascades, such as Ca2+-dependent and phytohormone signaling pathways (Arora et al., 2016; Hasanuzzaman et al., 2018). ROS and RNS have dual roles in plant cells. At low levels, they act as intracellular signaling agents and induce a positive response within the antioxidant system. At high levels, they become toxic and damage both cells and proteins. In plants, the genes of receptor kinase, annexin, and peroxisome biogenesis can be induced by ROS (Apel and Hirt, 2004). ROS and RNS can be neutralized or scavenged by a diverse array of enzymatic and non-enzymatic antioxidants (Arnao and Hernández-Ruiz, 2019b). Enzymatic antioxidant agents include superoxide dismutase (SOD), catalase (CAT), ascorbate peroxidase (APX), glutathione (GSH) peroxidase, GSH S-transferase, monodehydroascorbate reductase (MDHAR), peroxidase, and peroxiredoxin. Non-enzymatic compounds include polyphenols, flavonoids, polyamines, ascorbic acid (AsA), GSH, cysteine, alkaloids, proline, carotenoids, tocopherols, and plastoquinone.
Melatonin is one of ROS-regulated molecules (Pardo-Hernández et al., 2020). It is well known for ROS detoxification and an ecologically friendly antioxidant compound. Melatonin neutralizes free radical species via single-electron and hydrogen transfer (Galano and Reiter, 2018). It can directly interact with ROS to enhance plant resistance to various stresses by scavenging ROS (O2•−, OḤ), RNS (nitric oxide and dioxide radicals, azide radicals and peroxynitrite radicals) and other oxidative agents. The most reactive free radical among ROS is the hydroxyl radical (OḤ), which is mainly responsible for the oxidative damage to DNA (Chatgilialoglu et al., 2009; Galano and Alvarez-Idaboy, 2009). Furthermore, melatonin has superior OḤ scavenging activity, compared to mannitol and GSH (Arnao and Hernández-Ruiz, 2019b). Melatonin reduced nickel-induced oxidative damage by decreasing O2•− and hydrogen peroxide (H2O2) production in tomato leaves and roots (Jahan et al., 2020). Melatonin improved high light tolerance of Arabidopsis by scavenging H2O2 and O2•− (Yang et al., 2021). Under different abiotic stresses, exogenous melatonin can trigger the biosynthesis of endogenous melatonin to suppress the accumulation of H2O2, O2•− and MDA, thus alleviating cell membrane damage and improving photosynthesis (Chen Y. et al., 2021; Imran et al., 2021). In addition, melatonin derivatives such as c3-OHM and AFMK exhibit high OḤ and hydroperoxyl radical scavenging efficiency (Galano et al., 2014; Galano and Reiter, 2018). Therefore, melatonin and its metabolites act as direct ROS and RNS scavengers in plants under stress.
Melatonin as a Signaling Molecule
Melatonin functions as a signaling molecule. Under stress conditions, melatonin can directly enhance antioxidant enzyme activity and indirectly activates the gene expression of stress response systems and antioxidative systems (Figure 3). The expression of antioxidant enzymes (e.g., CAT, POD, and SOD, which detoxify excess H2O2) is induced by melatonin to restore redox homeostasis and control ROS levels. A number of antioxidant enzyme-related genes are induced by melatonin. For example, melatonin can alleviate oxidative damage caused by salt stress through the enhancement of antioxidant enzyme activity and removal of H2O2 in cotton (Zhang Y. et al., 2021). However, melatonin induces the expression of respiratory burst oxidase homologs (RBOH) that generate O2•−, causing an increase in H2O2 levels (Chen et al., 2017; Arnao and Hernández-Ruiz, 2019a). In plants, melatonin signaling triggers abiotic and biotic stress responses by acting on ROS- and RNS-mediated pathways. It also modulates various antioxidant pathways such as the AsA-GSH cycle (Erland et al., 2017). Melatonin can regulate hydrogen peroxide-mediated signaling pathway to detoxify excess ROS (Imran et al., 2021). In addition, Ca2+ signal pathway participates in melatonin-mediated abiotic stress tolerance via the regulation of Ca2+ signal genes like CIPK3 and CIPK9 (Gu et al., 2020). ROS generating enzymes (NADPH oxidase and GOX) are suppressed by the combined application of melatonin and Ca2+ (Zhang Y. et al., 2021). Moreover, melatonin regulates stress-related gene expression. For example, melatonin induced the expression of HSFA2 and heat HSP90, which enhance thermotolerance in tomato seedlings (Jahan et al., 2019). In salt stress, ion-response gene expressions (GhNHX1, GhSOS1 and GhAKT1) were upregulated by melatonin treatment for better salt tolerance of cotton (Shen et al., 2021). In tomato, carbohydrate metabolism is also demonstrated to be regulated by melatonin treatment (Iqbal et al., 2021). Carbon starvation-induced chlorophyll degradation and leaf senescence were alleviated by exogenous melatonin through upregulating transcript levels of miR171b, thus suppressing the expression of α-glucan water dikinase (GWD) gene (Wang et al., 2022).
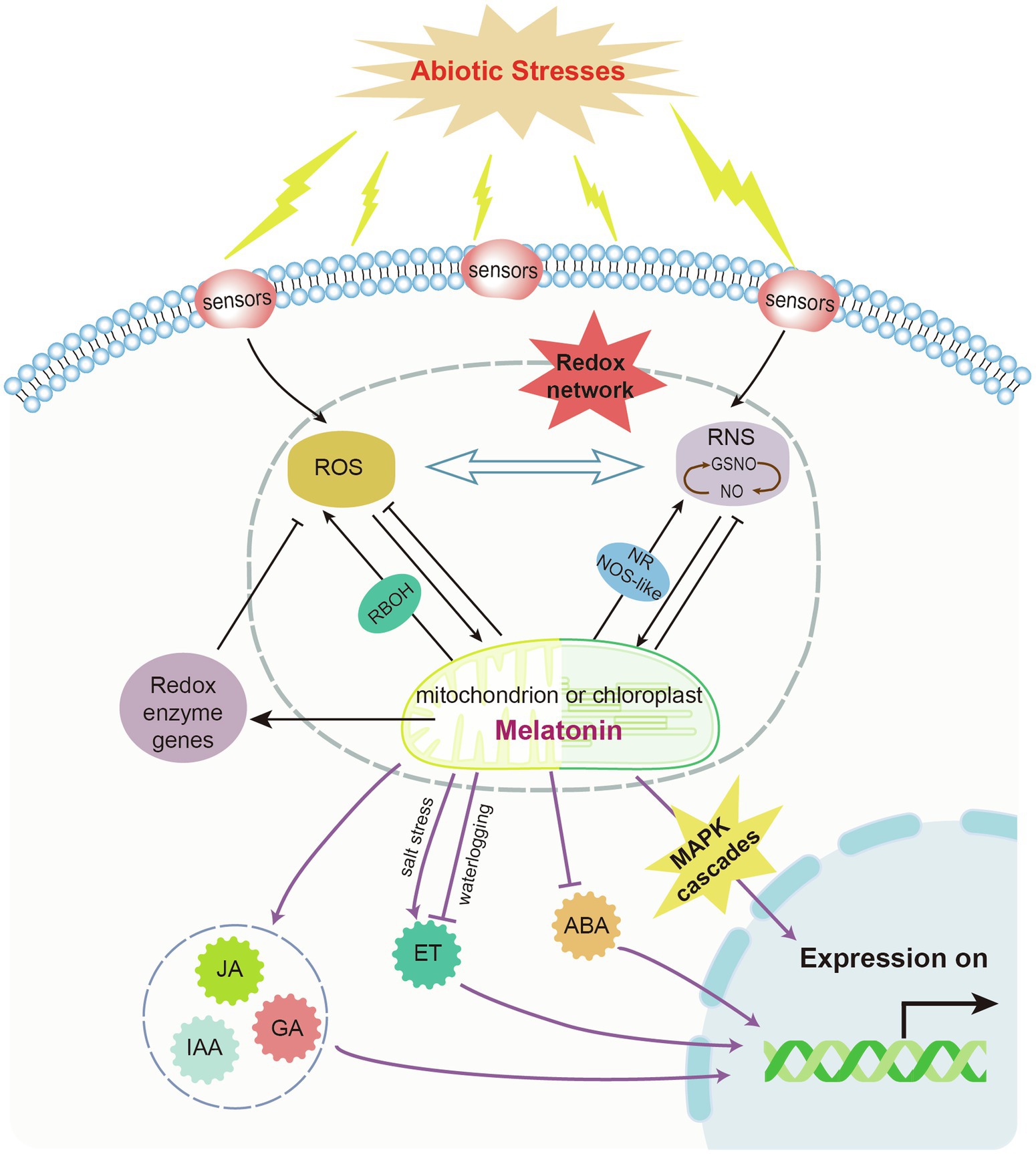
Figure 3. Endogenous melatonin-modulated feedback mechanism in plants under abiotic stresses. Abiotic stresses induce the accumulation of reactive oxygen species (ROS) and reactive nitrogen species (RNS), thus triggering the biosynthesis of endogenous melatonin. RNS and ROS can be scavenged by plant melatonin. Their levels can also be controlled by melatonin-mediated induction of redox enzymes. Mitogen-activated protein kinase (MAPK) cascades and other phytohormone signals are involved in melatonin-modulated transcriptional feedback mechanisms under abiotic stresses. NOS, nitric oxide synthase; GSNO, S-nitrosoglutathione; and RBOH, respiratory burst oxidase homolog.
Melatonin can also mediate the NOS-like system to exert physiological functions and benefit plants under stress conditions (Arnao and Hernández-Ruiz, 2018). For example, it interacts with NO to activate the SA-dependent pathway and MAPK cascades in response to biotic stresses (He and He, 2020). In Arabidopsis, pathogen attack-induced melatonin biosynthesis can activate MAPK kinase kinases such as oxidative signal-induced kinase 1, followed by the MAPK kinase 4/5/7/9 and MAPK3/6 cascades. This upregulates the expression of several defense genes such as pathogenesis-related protein 1 (PR1), isochorismate synthase 1 (ICS1), GSH S-transferase 1 (GST1), and APX1 (Lee et al., 2015; Lee and Back, 2016b, 2017). Similar to melatonin, some melatonin metabolites such as 2-OHM and AFMK can activate MAPKs. However, the induction of MAPK pathways by c3-OHM has not yet been reported (Lee and Back, 2016b).
A controversial topic of melatonin research is the regulation of other plant hormones by melatonin. Melatonin regulates the gene expression of multiple enzymes, receptors, and transcription factors that are associated with the biosynthesis and catabolism of IAA, GA, CK, ABA, ET, JA, SA, and BR. These relationships indicate possible crosstalk between melatonin and other phytohormones (Weeda et al., 2014; Arnao and Hernández-Ruiz, 2019a; Figure 3). Melatonin and IAA are structurally related and have the same precursor, tryptophan. Melatonin also upregulates the expression of auxin signaling and efflux genes (PIN1, PIN3, and PIN7), along with adventitious root formation in tomato (Wen et al., 2016). It also influences the biosynthesis and catabolism of ABA in response to various abiotic stresses (e.g., salt, drought, heat, and cold), possibly acting upstream of the ABA pathway (Li et al., 2015; Fu et al., 2017; Li H. et al., 2017; Arnao and Hernández-Ruiz, 2018). In addition, melatonin reprograms the biosynthesis and metabolism of ET and polyamine biosynthesis to improve waterlogging tolerance in alfalfa (Zhang Q. et al., 2019; Gu et al., 2020). Although there is increasing evidence of crosstalk between melatonin and other plant hormones, details regarding the underlying mechanisms are largely unknown.
Roles of Melatonin in Plant Abiotic Stress Responses
Melatonin has important roles in plant responses to abiotic stress. In this section, recent research concerning melatonin-mediated responses to several major abiotic stress factors will be discussed.
Drought Stress
Drought restricts plant growth and development. It is also a severe threat to crop productivity and quality because of alterations to the morphological, physiological, biochemical, and molecular features of plants (Cherono et al., 2021). Drought induces oxidative stress and activates stress signaling pathways mediated by ROS, Ca2+, and hormones. In addition, drought stress can activate transcription factors such as NACs, MYBs, AP2/EREBPs, bZIPs, HDs, and bHLHs (Zhang J. et al., 2014). Melatonin has an important role in drought response and resistance in plants. Typically, water deficiency promotes melatonin biosynthesis (Shi et al., 2015a). Endogenous melatonin levels change during water deprivation because melatonin biosynthesis genes (e.g., TDC, ASMT, COMT, and SNAT) are upregulated. Drought-induced melatonin biosynthesis has been reported in Arabidopsis, barley, bermudagrass, apple, grapevine, and rice (Moustafa-Farag et al., 2020).
Exogenous melatonin treatment can trigger an increase in endogenous melatonin levels, thereby improving turgor pressure, cell stability, chlorophyll recovery, and photosynthetic machinery stability under drought stress (Meng et al., 2014; Ding et al., 2018). Furthermore, melatonin treatment can promote seed germination, lateral root formation, hypocotyl elongation, vegetative growth, and fruit quality during drought (Hosseini et al., 2021; Sun et al., 2021). In rapeseed, seed priming with melatonin improves stomatal and ultrastructural traits, as well as osmotic adjustment, thus improving drought tolerance (Khan et al., 2019). Melatonin pretreatment in tomato improves dehydration resistance and promotes vegetative growth by decreasing the compatible solute content (e.g., of proline and soluble sugars) and increasing photosynthetic efficiency (Liu et al., 2015; Ibrahim et al., 2020). In lemon verbena plants, melatonin treatment alleviates the adverse effects of drought, while improving growth and essential oil yields by regulating mineral homeostasis and osmolyte accumulation (Hosseini et al., 2021). Moreover, melatonin-mediated drought tolerance in soybean is associated with glucose metabolism.
Melatonin treatment can also alleviate drought-induced oxidative damage in plant species such as apple, tomato, alfalfa, and wheat (Li et al., 2015; Antoniou et al., 2017; Cui et al., 2017). During oxidative stress, antioxidative enzymes such as APX, CAT, SOD, peroxide dismutase, peroxidase, dehydroascorbate reductase (DHAR) and GSH reductase are activated by melatonin, which suppresses ROS accumulation in drought environments (Alharby and Fahad, 2020; Sadak and Bakry, 2020). In Medicago sativa, melatonin serves as a priming agent by modulating nitro-oxidative and osmoprotective homeostasis to resist drought stress (Sun et al., 2021). After melatonin treatment, the AsA-GSH cycle, an integral antioxidant system, is enhanced through the upregulation of related genes (APX, MDHAR, and DHAR) to improve drought stress tolerance (Cui et al., 2017; Tiwari et al., 2021). In addition, MAPKs and transcription factors that improve drought tolerance are regulated by melatonin (Tiwari et al., 2021). In oat seedlings, the expression levels of MAPKs (e.g., Asmap1 and Aspk11) and transcription factor genes (e.g., WRKY1, DREB2, and MYB) are upregulated by melatonin treatment (Gao et al., 2018).
Crosstalk between melatonin and other phytohormones is another crucial drought stress regulatory mechanism. The application of melatonin may alleviate drought-induced suppression of flowering in plants by inducing GA biosynthesis (Tiwari et al., 2020). Drought tolerance is enhanced by interactions between melatonin and ABA, which regulate stomatal closure (Chen et al., 2020; Qi et al., 2021). Melatonin downregulates ABA biosynthesis genes and upregulates ABA catabolism genes (MdCYP707A1 and MdCYP707A2) to maintain stomatal function during drought stress (Li et al., 2015).
Waterlogging Stress
Waterlogging can affect crop survival, growth, and productivity. This phenomenon severely limits gas diffusion, which results in hypoxic stress caused by anaerobic respiration in the roots, thereby promoting ROS accumulation (Zhang Q. et al., 2019; Gu et al., 2020; Wu Q. et al., 2021). This burst of excessive ROS production causes roots to rot and leaves to wilt (Hossain et al., 2009). Melatonin participates in the regulation of plant responses to waterlogging. When plants are faced with waterlogging stress, their endogenous melatonin levels increase because melatonin biosynthesis genes (e.g., TDC, T5H, ASMT, COMT, and SNAT) are upregulated (Moustafa-Farag et al., 2020).
Endogenous melatonin levels are positively correlated with waterlogging resistance. Treatment with exogenous melatonin markedly enhances seedling vitality in peach, apple, and alfalfa by regulating endogenous melatonin levels under waterlogging conditions (Zheng et al., 2017; Zhang Q. et al., 2019; Gu et al., 2020). Additionally, melatonin treatment abates stomatal closure, chlorophyll and photosynthesis reduction, and leaf senescence (Zhang Q. et al., 2019; Gu et al., 2020). In Malus baccata, exogenous melatonin application induces the post-transcriptional regulation of endogenous melatonin levels during waterlogging (Zheng et al., 2017). In addition, waterlogging-induced oxidative damage is alleviated by melatonin treatment. Melatonin application enhanced the activities of several antioxidant enzymes and reduced H2O2 concentrations in both leaves and roots of peach seedlings to maintain redox homeostasis under waterlogging stress (Gu et al., 2020).
Additionally, melatonin plays a beneficial role in the regulation of waterlogging-induced hypoxia stress by optimizing metabolism in roots and regulating hypoxia-related genes (e.g., ET response factors and calcineurin B-like protein-interacting protein kinase) in peach seedlings (Gu et al., 2020). ET is an important signal associated with hypoxia stress. ET synthesis and signaling is affected by melatonin, which inhibits the expression of 1-aminocyclopropane-1-carboxylate synthase, 1-aminocyclopropane-1-carboxylic acid oxidase, and ET response factor (Zhang Q. et al., 2019; Gu et al., 2020). Notably, waterlogging induced-ET production is suppressed by treatment with moderate amounts of melatonin, whereas ET biosynthesis is induced by treatment with high concentrations of melatonin (Zheng et al., 2017). Therefore, the crosstalk between melatonin and ET has an important regulatory role in waterlogging stress tolerance.
Salt Stress
Salt stress has become a severe global problem, limiting agricultural production and leading to substantial economic losses worldwide (Su et al., 2021). Salt has two detrimental effects on plants: osmotic stress and ion poisoning (Van Zelm et al., 2020). Salt stress alters gene expression, mRNA stability, and translational regulation, thus altering the abundances of proteins in plants (Sharma and Dietz, 2009; Van Zelm et al., 2020). Plant phospholipid signals, protein kinase signals, and ABA are involved in responses to salt stress.
Salt tolerance is enhanced by melatonin in various plants such as maize, wheat, cucumber, tomato, cotton, and rice (Liang et al., 2015; Zhou et al., 2016; Chen et al., 2018; Ke et al., 2018; Zhang Y. et al., 2021). Salt stress can affect the expression patterns of key melatonin biosynthetic enzymes and increase endogenous melatonin content (Arnao and Hernández-Ruiz, 2009). Moreover, the overexpression of SNAT can significantly improve plant salt tolerance. For example, VvSNAT1 overexpression Arabidopsis lines exhibit greener leaves, enhanced growth, and higher germination rates under salt stress, compared with wild-type lines (Wu Y. et al., 2021). In contrast, SNAT inhibition lowers endogenous melatonin levels, thereby increasing the sensitivity of rice to salt stress (Byeon and Back, 2016).
Exogenous melatonin treatment helps to protect plants against salt stress by regulating antioxidant enzyme expression and activity, polyamine metabolism, and NO signaling (Zhan et al., 2019). The activation of major antioxidant enzymes decreases salinity-induced ROS and H2O2 levels. In addition, crosstalk between melatonin and NO regulates redox equilibrium via the differential expression of copper/zinc-SOD and manganese-SOD, and by modulating both GSH levels and GSH reductase activity in cotyledons under salt stress (Arora and Bhatla, 2017). Moreover, melatonin induces crosstalk between NO and hydrogen sulfide, thus improving salt stress tolerance in pepper (Kaya et al., 2020). Molecular hydrogen triggers melatonin signaling by activating SNAT-dependent melatonin production in Arabidopsis (Su et al., 2021).
Ion homeostasis is vital for plant survival under salt stress. There is evidence that melatonin assists with ion homeostasis maintenance, particularly concerning K+ and Na+. Melatonin-mediated ion homeostasis is associated with the expression of NHX and AKT, which encode two important ion channels (Li et al., 2012). The NHX family is related to K+ and Na+ antiporters. Na+ accumulates in vacuoles when NHX is upregulated (Munns and Tester, 2008). Under salt stress, the salt overly sensitive signaling pathway is triggered to transport Na+ out of the cells, or to activate an unknown transporter that confines Na+ to the vacuole.
Exogenous melatonin treatment leads to triacylglycerol breakdown, fatty acid β-oxidation, and energy turnover to maintain the activity of the plasma membrane H+-ATPase. These processes mediate K+/Na+ homeostasis and provide evidence for the mediation of seed germination by melatonin in cucumber under salt stress (Zhang N. et al., 2017). In addition, Na+ and Cl− accumulation is inhibited in rice roots and leaves after exogenous melatonin application by enhancing the transcription of OsSOS1 in roots and of OsCLC1 and OsCLC2 in roots and leaves (Li X. et al., 2017).
ET biosynthesis is promoted by exogenous melatonin treatment during salt stress in grapevine. Endogenous ET enhancement is regulated by MYB108A expression, which activates the expression of the ET biosynthesis-related gene ACS1 (Xu et al., 2019). GA and ABA, as well as antioxidant enzymes, are involved in melatonin-mediated seed germination under high salt conditions in cucumber (Zhang H. et al., 2014). Melatonin coordinates with BR during salt stress by regulating genes involved in BR biosynthesis and signal transduction in cotton. In addition, the Ca2+ signal transduction pathway has a pivotal role in melatonin-mediated salt tolerance (Zhang Y. et al., 2021).
Cold (Chilling) Stress
Cold stress exerts destructive effects on crop growth and productivity. It can lead to redox imbalance and induce excessive ROS accumulation. In response to cold stress, plants increase ABA synthesis, thus activating the C-repeat binding factor pathway (Knight et al., 2004). Extreme cold environments typically induce melatonin accumulation to protect plants against fatal injuries. Increasing endogenous melatonin levels can improve plant cold tolerance. For example, SNAT transgenic rice are less sensitive to cold than wild-type plants, indicating the protective role of melatonin in cold resistance (Kang et al., 2010). There is evidence that melatonin functions as a long-distance signal that can be transported from roots to shoots (Tan et al., 2007). In grafted watermelons, melatonin improves cold tolerance via root-to-shoot communication and transportation (Li H. et al., 2021).
Exogenous melatonin treatment can maintain the quality of fruits, vegetables, and cut flowers by conferring chilling tolerance under cold storage. For example, pre-storage treatment of loquat fruit with melatonin triggers the accumulation of phenolic compounds and a reduction in lignin, thereby alleviating flavor and nutrition loss induced by chilling injury under cold storage (Wang D. et al., 2021). Melatonin treatment in Bermuda grass can improve the levels of secondary metabolites including amino acids, organic acids, carbohydrates, and sugars, thereby enhancing cold tolerance (Hu et al., 2016). The application of 2-hydroxymelatonin, a metabolite of melatonin, may also improve cold and drought tolerances in tobacco, tomato, and cucumber (Lee and Back, 2019).
Similar to its effects in the context of other stress factors, exogenous melatonin application can alleviate cold damage through the activation of antioxidant enzymes, as well as reductions of both ROS accumulation and lipid peroxidation (Hu et al., 2016). For example, cut flowers treated with melatonin had lower H2O2 concentrations and increased 2,2-diphenyl-1-picrylhydrazyl (DPPH) scavenging capacity under cold stress than did untreated flowers (Aghdam et al., 2019). Chilling tolerance in tomato increases because of arginine-dependent NO accumulation after melatonin treatment. Under cold stress, melatonin upregulates genes related to stress responsive pathways such as C-repeat binding factor/dehydration-responsive element-binding protein (CBF/DREB), cold regulated 15a (COR15a), calmodulin binding transcription activator 1 (CAMTA1), and zinc finger transcription factors 10 and 12 (ZAT 10 and ZAT 12; Zhao et al., 2017; Guo et al., 2018; Wang et al., 2018). The rhythmic expression patterns of circadian clock genes are also altered by melatonin supplementation in hulless barley seedlings, regulating their growth and enhancing cold resistance (Chang et al., 2021).
Additionally, the expression patterns of phytohormone biosynthesis genes are modulated by exogenous melatonin. For example, the expression levels of a key gene (ClAOC1) in JA biosynthesis, and a key gene (ClAMI1) in IAA biosynthesis are upregulated in watermelon leaves after melatonin application under cold stress (Chang et al., 2020; Li H. et al., 2021). Consequently, the JA and IAA contents increase, thus improving photosynthesis and redox homeostasis (Chang et al., 2021).
Heat Stress
Heat stress is becoming a global concern because of global warming. Frequent heat waves have a profound impact on terrestrial plants. Heat stress affects plants at the physiological (e.g., photosynthesis, cell membrane thermostability, and oxidative damage), transcriptional, post-transcriptional (e.g., non-coding RNAs), and epigenetic (e.g., DNA methylation, histone modification, and chromatin remodeling) levels (Zhao et al., 2020). During heat stress, endogenous melatonin levels increase, thereby improving plant thermotolerance. In tomato, COMT1 and TDC silencing aggravates high temperature-induced oxidative damage by suppressing endogenous melatonin biosynthesis (Ahammed et al., 2019). Furthermore, ASMT and SNAT overexpression significantly improves thermotolerance by increasing endogenous melatonin (Xu et al., 2016; Wang et al., 2020).
Under heat stress, exogenous melatonin treatment can enhance plant antioxidant defense systems via control of ROS accumulation and enhancement of proline metabolism. In tomato seedlings, melatonin treatment promotes thermotolerance by balancing redox homeostasis, while modulating polyamine and nitric oxide biosynthesis (Jahan et al., 2019). In wheat, photosynthetic potential is maintained by interactions between melatonin and hydrogen sulfide under heat stress (Iqbal et al., 2021). Upon exposure to high temperatures, exogenous melatonin treatment can alleviate heat damage in plants by mediating the activity of HSFs, which are the master mediators of heat responses. In Arabidopsis, melatonin-mediated thermotolerance was associated with the upregulation of HSFA2 and HSA32, as well as HSP90 and HSP101 (Shi et al., 2015b). In tomato, melatonin treatment upregulated HSP gene expression and increases HSP production, which then repaired denatured or damaged proteins after heat exposure (Xu et al., 2016). Furthermore, HSP40 interacted with SlSNAT to regulate melatonin biosynthesis and promote thermotolerance by maintaining Rubisco enzyme stability under heat stress (Wang et al., 2020). In addition, heat-induced leaf senescence is suppressed by melatonin treatment, which regulates ABA and CK biosynthesis and signaling pathways (Zhang J. et al., 2017). Exogenous melatonin reduces heat-induced damage by upregulating SA production and downregulating ABA levels in soybean seedlings (Imran et al., 2021).
Heavy Metal Toxicity
Heavy metals are toxic to plants. Heavy metal stress can repress root growth and promote the leaf senescence by inducing ROS accumulation and damaging chloroplasts. In addition, Calvin cycle enzymes, photosynthesis, and carbohydrate metabolism are also inhibited by heavy mental toxicity (Hasan et al., 2015, 2019; Ni et al., 2018). Toxicity caused by lead (Pb), copper, vanadium, and aluminum can disrupt redox homeostasis and accelerate the accumulation of redox agents (Nagajyoti et al., 2010).
Melatonin treatment can alleviate heavy metal-induced suppression of root growth and activity (Ni et al., 2018; Jahan et al., 2020). Endogenous melatonin production can be induced by treatment with exogenous melatonin to strengthen heavy metal tolerance. In wheat seedlings, melatonin treatment promoted endogenous melatonin biosynthesis in shoots and roots. The enhancement of endogenous melatonin alleviated cadmium (Cd) toxicity by balancing H2O2 homeostasis and activating antioxidant enzymes (Ni et al., 2018). Exogenous melatonin treatment also enhances the antioxidant potential of plants and reduces lipid peroxidation under aluminum stress (Sun et al., 2020). In tomato, melatonin effectively ameliorated Cd-induced toxicity by enhancing H+-ATPase activity, increasing GSH and phytochelatin contents, and facilitating Cd sequestration in plant cells (Hasan et al., 2015). In addition, melatonin treatment alleviates nickel phytotoxicity by improving gas exchange, while increasing the contents of photosynthetic pigments, minerals, nutrients, and secondary metabolites (Jahan et al., 2020). NO signaling is involved in melatonin-induced antioxidant defenses. NO has a vital role in re-establishing redox homeostasis in plants under heavy metal stress. Crosstalk between melatonin and NO improves Pb and Cd stress tolerance (Kaya et al., 2019; Okant and Kaya, 2019).
In addition to regulating antioxidant levels, melatonin mediates heavy metal tolerance by enhancing heavy metal uptake and sequestration. Cd, vanadium, and copper are excluded from or sequestered in plant cells after exogenous melatonin treatment (Nawaz et al., 2018; Cao et al., 2019; Hasan et al., 2019). Notably, melatonin treatment balances endogenous GA and ABA levels and improves thiol-mediated detoxification in two indica rice. Furthermore, melatonin reduces Pb damage in radish plants via DNA demethylation of metal transporters and antioxidant genes (Tang et al., 2021).
Light Stress
Light is dispensable to plants. However, it is also an environmental stressor that causes photodamage and overaccumulation of ROS in plants. Ultraviolet (UV) light can induce the generation of free radicals in plants (Demarsy et al., 2018; Nazir et al., 2020). Exposure to high UV radiation for a long time can result in damage of the nuclear membrane and generally cause changes in the ultrastructure of various cellular components in many plant species.
One important regulator of melatonin biosynthesis is light (Tan et al., 2007; Zhang H. et al., 2019). In plants, it was observed that the concentration of endogenous melatonin was increased after UV-B treatment for short time, suggesting that endogenous melatonin is involved in UV-B response (Afreen et al., 2006; Wei et al., 2019). Exogenous melatonin increased the content isoflavone monomers in 4-day-old germinated soybeans under UV-B stress (Yin et al., 2022). In Arabidopsis, melatonin also participated in UV-B signaling pathway by delaying and subsequently enhancing expression of COP1, HY5, HYH, and RUP1/2 to mediate UV-B stress tolerance (Yao et al., 2021). Under high light stress, melatonin can improve photosynthesis, alleviate inhibitory effect of stomatal apertures and relieve the oxidative damage to cells by regulating redox state (Wei et al., 2019; Haskirli et al., 2021; Yang et al., 2021). In contrast, snat1 knockout mutants of Arabidopsis failed to induce melatonin biosynthesis and lower expression of ROS-responsive genes, resulting in sensitivity to high light stress (Lee and Back, 2018). In addition, melatonin may regulate flavonoids to mediate light tolerance (Wei et al., 2019; Nazir et al., 2020). Flavonoids are also important antioxidants (Shen et al., 2022), however, the interaction of melatonin and flavonoids need further investigation.
Other Abiotic Stresses
Melatonin functions on other abiotic stress factors have been discussed. Potassium deficiency tolerance is a vital problem to crop production. Melatonin can enhance potassium content in Malus under different stress conditions and promote potassium deficiency tolerance in wheat (Li et al., 2016; Li G. et al., 2021). TaHAK1 regulated by TaNAC71 played an important role in MT-mediated potassium deficiency tolerance in wheat (Li G. et al., 2021). In addition, plastic pollution has received a great concern worldwide. Melatonin was reported to reduce the nanoplastic uptake by roots and their translocation to shoots by regulating the expression of genes associated with aquaporin (TIP2-9, PIP2, PIP3, PIP1-5 and PIP1.2). Melatonin enhanced the tolerance to nanoplastic toxicity by maintaining a better redox homeostasis and ameliorating the negative effects of nanoplastics on carbohydrate metabolism (Li S. et al., 2021).
Exogenous Melatonin Application
Exogenous melatonin treatment can improve the tolerance of plants to various abiotic stresses (e.g., salt, drought, waterlogging, heat, cold, and heavy metal toxicity) in several plant species. These improvements in stress tolerance are largely associated with the induction of endogenous melatonin production after exogenous melatonin treatment. In addition, these improvements are typically dose-dependent. Treatment with low melatonin concentrations typically induces stronger stress tolerance responses than does treatment with high melatonin concentrations (Zhang et al., 2015).
Overall, melatonin has great potential for use as an anti-stress agent in agricultural crop production applications. Recent reports of exogenous melatonin treatment for abiotic stresses are summarized in Table 1.
Conclusions and Future Perspectives
Plant melatonin is important for alleviating damage caused by various abiotic stresses. In this review, we summarized the regulatory mechanisms that drive melatonin-mediated abiotic stress tolerance in plants. Plant tolerance is typically enhanced by melatonin via the following pathways. Melatonin can act as a direct scavenger of ROS and RNS to improve antioxidant capacity. It also acts as a signaling molecule, regulating the expression of genes involved in stress responses, antioxidant production, and phytohormone pathways (e.g., ABA, ET, and JA; Figure 4). Moreover, melatonin affects the redox network. It can interact with NO to regulate redox homeostasis, thus maintaining antioxidant potential during abiotic stress.
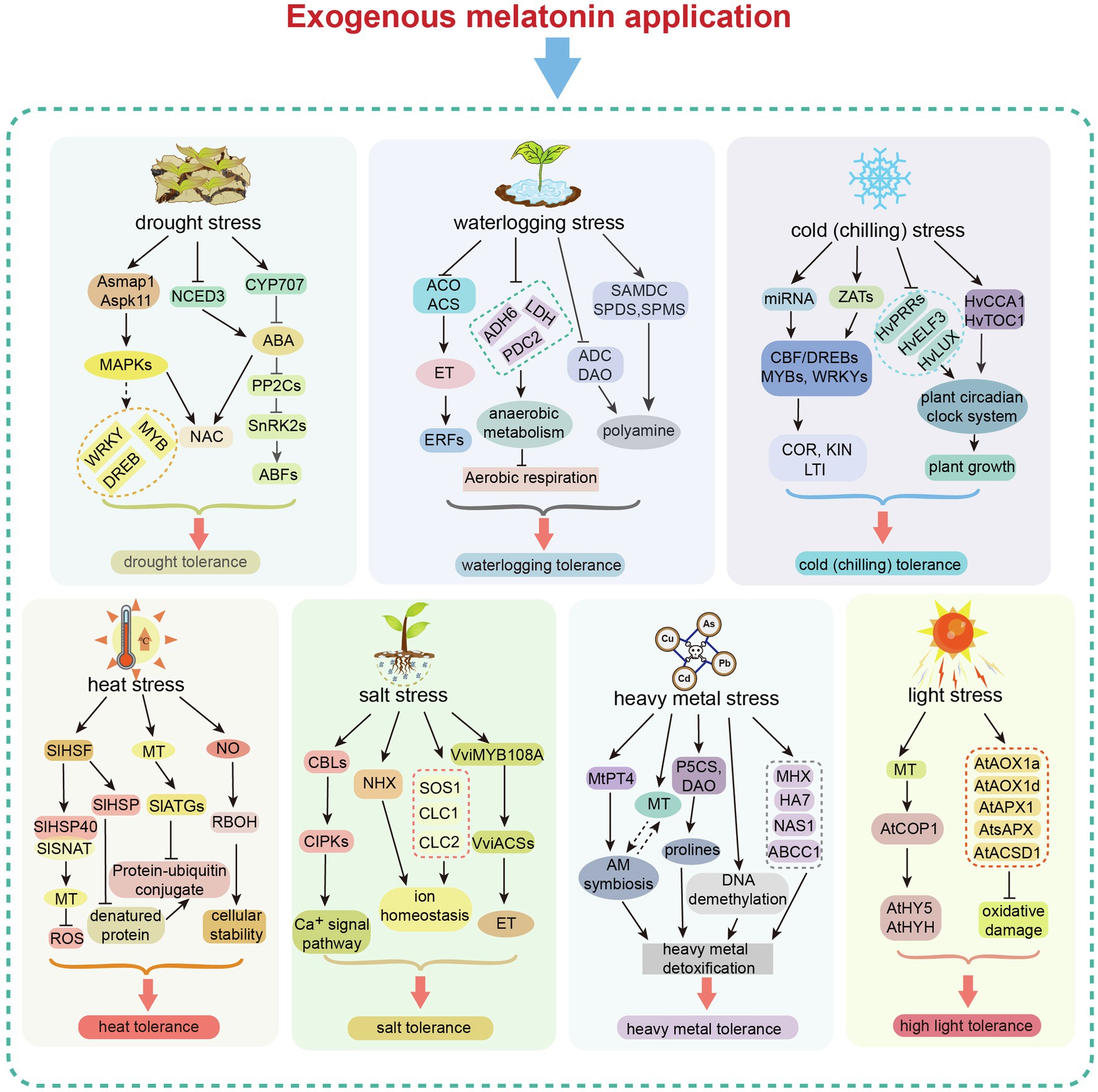
Figure 4. Exogenous melatonin-mediated regulation in response to different abiotic stresses. Exogenous melatonin application induces changes in gene expression in different abiotic stress response pathways, resulting in enhanced tolerance to major abiotic stresses (drought, waterlogging, heat, cold, salt, heavy metal toxicity and light). MAPK, mitogen-activated protein kinase; NCED3, nine-cis-epoxycarotenoid dioxygenase 3; NAC, N-acetylcysteine; DREB, dehydration responsive element binding; CYP, cytochrome P450; PP2C, protein phosphatase 2C; SnRK, sucrose non-fermenting–related related kinase; ABF, abscisic acid responsive element-binding factor; ABA, abscisic acid; ACO, acyl-CoA oxidase; ACS, acetyl-CoA synthetase; ET, ethylene; ERF, ETS2 repressor factor; ADH, alcohol dehydrogenase; LDH, lactate dehydrogenase; PDC2, pyruvate decarboxylase-2; SAMDC, S-adenosylmethionine decarboxylase; SPDS, spermidine synthase; SPMS, spermine synthase; ADC, arginine decarboxylase; DAO, di-amine acid oxidase; CBF/DREB, dehydration responsive element binding/C-repeat binding factor; ZAT, zinc transporter; COR, cold-regulated gene; KIN, Kin17 DNA and RNA binding protein; LTI, low-temperature-induced genes; PRRs, pseudo-response regulators; ELF3, E74 like ETS transcription factor 3; CCA1, circadian colck associated 1; TOC1, timing of CAB expression 1; HSF, heat shock facter; HSP, heat shock protein; SNAT, serotonin N-acetyltransferase; MT, melatonin; RBOH, respiratory burst oxidase homolog; ATG, autophagy-related; CBL, calcineurin B-like proteins; CIPK, CBL-interacting protein kinases; NHX, Na+/H+ exchanger protein; SOS1, SOS Ras/Rac guanine nucleotide exchange factor 1; CLC, chloride channel; PT4, phosphate transporter 4; AM, arbuscular mycorrhizal; P5CS, delta 1-pyrroline-5-carboxylate synthase; DAO, D-amino acid oxidase; MHX, magnesium/proton exchanger; NAS1, nicotianamine synthase 1; nicotianamine synthase 1; COP1, constitutive photomorphogenic 1; HY5, elongated hypocotyl 5; HYHAPX, hy5 homolog; APX, ascorbate peroxidase; AOX, aldehyde oxidase; and ACSD, 2-amino-3-carboxymuconate-6-semialdehyde decarboxylase.
The key melatonin biosynthetic enzymes (TDC, T5H, SNAT, ASMT, COMT) have been identified. The melatonin biosynthetic pathway has been characterized in several plant species. Under normal conditions, the chloroplast is the main biosynthetic site of plant melatonin. Mitochondria are also sites of melatonin biosynthesis in plants when the chloroplast pathway is blocked. The melatonin catabolic pathway and its metabolites have received increasing research attention in recent years because of their beneficial effects on plant resistance to environmental stresses. Exogenous melatonin application can regulate plant physiological and biochemical processes to enhance stress tolerance (Figure 5).
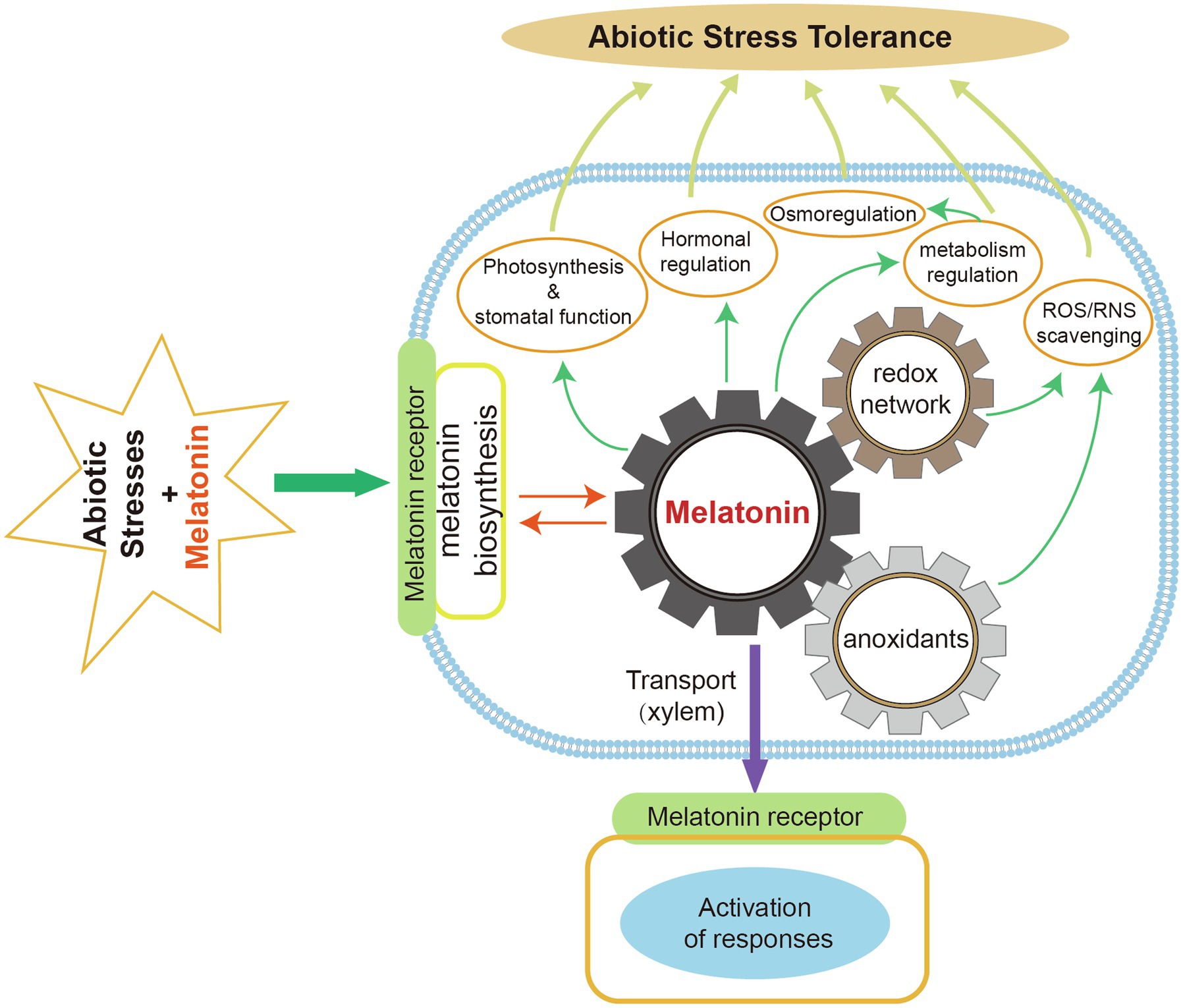
Figure 5. Roles of melatonin in improving plant abiotic stress tolerance. Exogenous melatonin can remove ROS and RNS by interacting with melatonin receptors to induce endogenous melatonin production and increased antioxidant levels. Melatonin facilitates photosynthesis and stomatal functions. It also regulates plant hormones, osmosis, and metabolism in response to abiotic stresses. Melatonin can be transported to various plant organs via the xylem and then activate stress responses.
Several studies have demonstrated the powerful effects of exogenous melatonin on plant stress tolerance enhancement. However, the precise signaling, transcriptional, and epigenetic mechanisms of melatonin remain unclear and require further characterization. Although melatonin can influence the biosynthesis and signaling of other phytohormones, some mechanisms by which melatonin interacts with other phytohormones remain elusive. Furthermore, most melatonin-mediated stress tolerance studies have been performed using model plant systems, as well as some important crops. However, the transferability of these findings to other plant species should be investigated. Considering the role of melatonin in abiotic stress tolerance in plants, melatonin-related transgenic technologies could be developed to enhance stress resistance in plants.
Exogenous melatonin treatment has mainly been applied under laboratory-scale conditions. However, large-scale commercial and agricultural applications of melatonin have rarely been performed. Furthermore, the potential detrimental effects of melatonin treatment on plants grown under standard conditions must be investigated. Therefore, field studies are required to determine the effects of melatonin treatment on final crop yield and quality under regular conditions.
Author Contributions
WZ and BJ: conceptualization. WZ and ZL: writing—original preparation. WZ, SM, and BJ: writing—review and editing. All authors contributed to the article and approved the submitted version.
Conflict of Interest
The authors declare that the research was conducted in the absence of any commercial or financial relationships that could be construed as a potential conflict of interest.
Publisher’s Note
All claims expressed in this article are solely those of the authors and do not necessarily represent those of their affiliated organizations, or those of the publisher, the editors and the reviewers. Any product that may be evaluated in this article, or claim that may be made by its manufacturer, is not guaranteed or endorsed by the publisher.
Acknowledgments
We apologize for not being able to cite all the relevant publications in the literature due to space limitations.
References
Afreen, F., Zobayed, S. M., and Kozai, T. (2006). Melatonin in Glycyrrhiza uralensis: response of plant roots to spectral quality of light and UV-B radiation. J. Pineal Res. 41, 108–115. doi: 10.1111/j.1600-079X.2006.00337.x
Aghdam, M. S., Jannatizadeh, A., Nojadeh, M. S., and Ebrahimzadeh, A. (2019). Exogenous melatonin ameliorates chilling injury in cut anthurium flowers during low temperature storage. Postharvest Biol. Technol. 148, 184–191. doi: 10.1016/j.postharvbio.2018.11.008
Ahammed, G. J., Xu, W., Liu, A., and Chen, S. (2019). Endogenous melatonin deficiency aggravates high temperature-induced oxidative stress in Solanum lycopersicum L. Environ. Exp. Bot. 161, 303–311. doi: 10.1016/j.envexpbot.2018.06.006
Ahmad, S., Muhammad, I., Wang, G. Y., Zeeshan, M., Yang, L., Ali, I., et al. (2021). Ameliorative effect of melatonin improves drought tolerance by regulating growth, photosynthetic traits and leaf ultrastructure of maize seedlings. Plant Biol. 21:368. doi: 10.1186/s12870-021-03160-w
Alharby, H. F., and Fahad, S. (2020). Melatonin application enhances biochar efficiency for drought tolerance in maize varieties: modifications in physio-biochemical machinery. Agron. J. 112, 2826–2847. doi: 10.1002/agj2.20263
Antoniou, C., Chatzimichail, G., Xenofontos, R., Pavlou, J. J., Panagiotou, E., Christou, A., et al. (2017). Melatonin systemically ameliorates drought stress-induced damage in Medicago sativa plants by modulating nitro-oxidative homeostasis and proline metabolism. J. Pineal Res. 62:e12401. doi: 10.1111/jpi.12401
Apel, K., and Hirt, H. (2004). Reactive oxygen species: metabolism, oxidative stress, and signal transduction. Annu. Rev. Plant Biol. 55, 373–399. doi: 10.1146/annurev.arplant.55.031903.141701
Arnao, M. B., and Hernández-Ruiz, J. (2009). Chemical stress by different agents affects the melatonin content of barley roots. J. Pineal Res. 46, 295–299. doi: 10.1111/j.1600-079X.2008.00660.x
Arnao, M. B., and Hernández-Ruiz, J. (2018). Melatonin and its relationship to plant hormones. Ann. Bot. 121, 195–207. doi: 10.1093/aob/mcx114
Arnao, M. B., and Hernández-Ruiz, J. (2019a). Melatonin: a new plant hormone and/or a plant master regulator? Trends Plant Sci. 24, 38–48. doi: 10.1016/j.tplants.2018.10.010
Arnao, M. B., and Hernández-Ruiz, J. (2019b). Melatonin and reactive oxygen and nitrogen species: a model for the plant redox network. Melatonin Res. 2, 152–168. doi: 10.32794/11250036
Arnao, M., and Hernández-Ruiz, J. (2020). Melatonin in flowering, fruit set and fruit ripening. Plant Reprod. 33, 77–87. doi: 10.1007/s00497-020-00388-8
Arora, D., and Bhatla, S. C. (2017). Melatonin and nitric oxide regulate sunflower seedling growth under salt stress accompanying differential expression of cu/Zn SOD and Mn SOD. Free Radic. Biol. Med. 106, 315–328. doi: 10.1016/j.freeradbiomed.2017.02.042
Arora, D., Jain, P., Singh, N., Kaur, H., and Bhatla, S. C. (2016). Mechanisms of nitric oxide crosstalk with reactive oxygen species scavenging enzymes during abiotic stress tolerance in plants. Free Radic. Res. 50, 291–303. doi: 10.3109/10715762.2015.1118473
Axelrod, J., and Weissbach, H. (1960). Enzymatic O-methylation of N-acetylserotonin to melatonin. Science 131:1312. doi: 10.1126/science.131.3409.1312
Back, K. (2021). Melatonin metabolism, signaling and possible roles in plants. Plant J. 105, 376–391. doi: 10.1111/tpj.14915
Back, K., Tan, D. X., and Reiter, R. J. (2016). Melatonin biosynthesis in plants: multiple pathways catalyze tryptophan to melatonin in the cytoplasm or chloroplasts. J. Pineal Res. 61, 426–437. doi: 10.1111/jpi.12364
Blanchard, B., Pompon, D., and Ducrocq, C. (2000). Nitrosation of melatonin by nitric oxide and peroxynitrite. J. Pineal Res. 29, 184–192. doi: 10.1034/j.1600-079X.2000.290308.x
Byeon, Y., and Back, K. (2015). Molecular cloning of melatonin 2-hydroxylase responsible for 2-hydroxymelatonin production in rice (Oryza sativa). J. Pineal Res. 58, 343–351. doi: 10.1111/jpi.12220
Byeon, Y., and Back, K. (2016). Low melatonin production by suppression of either serotonin N-acetyltransferase or N-acetylserotonin methyltransferase in rice causes seedling growth retardation with yield penalty, abiotic stress susceptibility, and enhanced coleoptile growth under anoxic conditions. J. Pineal Res. 60, 348–359. doi: 10.1111/jpi.12317
Byeon, Y., Choi, G. H., Lee, H. Y., and Back, K. (2015a). Melatonin biosynthesis requires N-acetylserotonin methyltransferase activity of caffeic acid O-methyltransferase in rice. J. Exp. Bot. 66, 6917–6925. doi: 10.1093/jxb/erv396
Byeon, Y., Lee, H. Y., Hwang, O. J., Lee, H. J., Lee, K., and Back, K. (2015b). Coordinated regulation of melatonin synthesis and degradation genes in rice leaves in response to cadmium treatment. J. Pineal Res. 58, 470–478. doi: 10.1111/jpi.12232
Byeon, Y., Lee, H. Y., Lee, K., Park, S., and Back, K. (2014). Cellular localization and kinetics of the rice melatonin biosynthetic enzymes SNAT and ASMT. J. Pineal Res. 56, 107–114. doi: 10.1111/jpi.12103
Byeon, Y., Tan, D. X., Reiter, R. J., and Back, K. (2015c). Predominance of 2-hydroxymelatonin over melatonin in plants. J. Pineal Res. 59, 448–454. doi: 10.1111/jpi.12274
Cao, Y. Y., Qi, C. D., Li, S., Wang, Z., Wang, X., Wang, J., et al. (2019). Melatonin alleviates copper toxicity via improving copper sequestration and ROS scavenging in cucumber. Plant Cell Physiol. 60, 562–574. doi: 10.1093/pcp/pcy226
Cao, L., Qin, B., and Zhang, Y. X. (2021). Exogenous application of melatonin may contribute to enhancement of soybean drought tolerance via its effects on glucose metabolism. Biotechnol. Biotechnol. Equip. 35, 964–976. doi: 10.1080/13102818.2021.1941254
Chang, J., Guo, Y., Zhang, Z., Wei, C., Zhang, Y., Ma, J., et al. (2020). CBF-responsive pathway and phytohormones are involved in melatonin-improved photosynthesis and redox homeostasis under aerial cold stress in watermelon. Acta Physiol. Plant. 42:159. doi: 10.1007/s11738-020-03147-4
Chang, T., Zhao, Y., He, H., Xi, Q., Fu, J., and Zhao, Y. (2021). Exogenous melatonin improves growth in hulless barley seedlings under cold stress by influencing the expression rhythms of circadian clock genes. Peer J. 9:e10740. doi: 10.7717/peerj.10740
Chatgilialoglu, C., D’Angelantonio, M., Guerra, M., Kaloudis, P., and Mulazzani, Q. G. (2009). A reevaluation of the ambident reactivity of the guanine moiety towards hydroxyl radicals. Angew. Chem. Int. Ed. Eng. 48, 2214–2217. doi: 10.1002/anie.200805372
Chen, Z., Gu, Q., Yu, X., Huang, L., Xu, S., Wang, R., et al. (2018). Hydrogen peroxide acts downstream of melatonin to induce lateral root formation. Ann. Bot. 121, 1127–1136. doi: 10.1093/aob/mcx207
Chen, K., Li, G. J., Bressan, R. A., Song, C. P., Zhu, J. K., and Zhao, Y. (2020). Abscisic acid dynamics, signaling, and functions in plants. J. Integr. Plant Biol. 62, 25–54. doi: 10.1111/jipb.12899
Chen, Y., Li, R., Ge, J., Liu, J., Wang, W., Xu, M., et al. (2021). Exogenous melatonin confers enhanced salinity tolerance in rice by blocking the ROS burst and improving Na+/K+ homeostasis. Environ. Exp. Bot. 189:104530. doi: 10.1016/j.envexpbot.2021.104530
Chen, Z., Xie, Y., Gu, Q., Zhao, G., Zhang, Y., Cui, W., et al. (2017). The AtrbohF-dependent regulation of ROS signaling is required for melatonin-induced salinity tolerance in Arabidopsis. Free Radic. Biol. Med. 108, 465–477. doi: 10.1016/j.freeradbiomed.2017.04.009
Chen, W., Zhang, J., Zheng, S., Wang, Z., Xu, C., Zhang, Q., et al. (2021). Metabolite profiling and transcriptome analyses reveal novel regulatory mechanisms of melatonin biosynthesis in hickory. Hortic. Res. 8:196. doi: 10.1038/S41438-021-00631-X
Cherono, S., Ntini, C., Wassie, M., Mollah, M. D., Belal, M. A., Ogutu, C., et al. (2021). Exogenous application of melatonin improves drought tolerance in coffee by regulating photosynthetic efficiency and oxidative damage. J. Am. Soc. Hortic. Sci. 146, 24–32. doi: 10.21273/JASHS04964-20
Choi, G. H., and Back, K. (2019). Cyclic 3-hydroxymelatonin exhibits diurnal rhythm and cyclic 3-hydroxymelatonin overproduction increases secondary tillers in rice by upregulating MOC1 expression. Melatonin Res. 2, 120–138. doi: 10.32794/11250034
Choi, G. H., Lee, H. Y., and Back, K. (2017). Chloroplast overexpression of rice caffeic acid O-methyltransferase increases melatonin production in chloroplasts via the 5-methoxytryptamine pathway in transgenic rice plants. J. Pineal Res. 63:e12412. doi: 10.1111/jpi.12412
Corpas, F. J., and Barroso, J. B. (2013). Nitro-oxidative stress vs oxidative or nitrosative stress in higher plants. New Phytol. 199, 633–635. doi: 10.1111/nph.12380
Corpas, F. J., Río, L. A. D., and Palma, J. M. (2019). Impact of nitric oxide (NO) on the ROS metabolism of peroxisomes. Plan. Theory 8:37. doi: 10.3390/plants8020037
Cui, G., Zhao, X., Liu, S., Sun, F., Zhang, C., and Xi, Y. (2017). Beneficial effects of melatonin in overcoming drought stress in wheat seedlings. Plant Physiol. Biochem. 118, 138–149. doi: 10.1016/j.plaphy.2017.06.014
Del Río, L. A., and López-Huertas, E. (2016). ROS generation in peroxisomes and its role in cell signaling. Plant Cell Physiol. 57, 1364–1376. doi: 10.1093/pcp/pcw076
Demarsy, E., Goldschmidt-Clermont, M., and Ulm, R. (2018). Coping with ‘dark sides of the sun’ through photoreceptor signaling. Trends Plant Sci. 23, 260–271. doi: 10.1016/j.tplants.2017.11.007
Ding, F., Wang, G., Wang, M., and Zhang, S. (2018). Exogenous melatonin improves tolerance to water deficit by promoting cuticle formation in tomato plants. Molecules 23:1605. doi: 10.3390/molecules23071605
Dubbels, R., Reiter, R., Klenke, E., Goebel, A., Schnakenberg, E., Ehlers, C., et al. (1995). Melatonin in edible plants identified by radioimmunoassay and by high performance liquid chromatography-mass spectrometry. J. Pineal Res. 18, 28–31. doi: 10.1111/j.1600-079X.1995.tb00136.x
Erland, L. A., Saxena, P. K., and Murch, S. J. (2017). Melatonin in plant signalling and behaviour. Funct. Plant Biol. 45, 58–69. doi: 10.1071/FP16384
Erland, L. A. E., Shukla, M. R., Singh, A. S., Murch, S. J., and Saxena, P. K. (2018). Melatonin and serotonin: mediators in the symphony of plant morphogenesis. J. Pineal Res. 64:e12452. doi: 10.1111/jpi.12452
Feng, Y., Fu, X., Han, L., Xu, C., Liu, C., Bi, H., et al. (2021). Nitric oxide functions as a downstream signal for melatonin-induced cold tolerance in cucumber seedlings. Front. Plant Sci. 12:686545. doi: 10.3389/fpls.2021.686545
Fleta-Soriano, E., Díaz, L., Bonet, E., and Munné-Bosch, S. (2017). Melatonin may exert a protective role against drought stress in maize. J. Agron. Crop Sci. 203, 286–294. doi: 10.1111/jac.12201
Fu, J., Wu, Y., Miao, Y., Xu, Y., Zhao, E., Wang, J., et al. (2017). Improved cold tolerance in Elymus nutans by exogenous application of melatonin may involve ABA-dependent and ABA-independent pathways. Sci. Rep. 7:39865. doi: 10.1038/srep39865
Galano, A., and Alvarez-Idaboy, J. R. (2009). Guanosine + OH radical reaction in aqueous solution: a reinterpretation of the UV-vis data based on thermodynamic and kinetic calculations. Org. Lett. 11, 5114–5117. doi: 10.1021/ol901862h
Galano, A., and Reiter, R. J. (2018). Melatonin and its metabolites vs oxidative stress: from individual actions to collective protection. J. Pineal Res. 65:e12514. doi: 10.1111/jpi.12514
Galano, A., Tan, D. X., and Reiter, R. J. (2014). Cyclic 3-hydroxymelatonin, a key metabolite enhancing the peroxyl radical scavenging activity of melatonin. RSC Adv. 4, 5220–5227. doi: 10.1039/c3ra44604b
Gao, W., Zhang, Y., Feng, Z., Bai, Q., He, J., and Wang, Y. J. (2018). Effects of melatonin on antioxidant capacity in naked oat seedlings under drought stress. Molecules 23:1580. doi: 10.3390/molecules23071580
Gu, X., Xue, L., Lu, L., Xiao, J., Song, G., Xie, M., et al. (2020). Melatonin enhances the waterlogging tolerance of Prunus persica by modulating antioxidant metabolism and anaerobic respiration. J. Plant Growth Regul. 40, 2178–2190. doi: 10.1007/s00344-020-10263-5
Guo, X., Liu, D., and Chong, K. (2018). Cold signaling in plants: insights into mechanisms and regulation. J. Integr. Plant Biol. 60, 745–756. doi: 10.1111/jipb.12706
Hardeland, R. (2015). Melatonin in plants and other phototrophs: advances and gaps concerning the diversity of functions. J. Exp. Bot. 66, 627–646. doi: 10.1093/jxb/eru386
Hardeland, R. (2017). Taxon-and site-specific melatonin catabolism. Molecules 22:2015. doi: 10.3390/molecules22112015
Hardeland, R. (2019). Melatonin in the evolution of plants and other phototrophs. Melatonin Res. 2, 10–36. doi: 10.32794/mr11250029
Hardeland, R., Tan, D. X., and Reiter, R. J. (2009). Kynuramines, metabolites of melatonin and other indoles: the resurrection of an almost forgotten class of biogenic amines. J. Pineal Res. 47, 109–126. doi: 10.1111/j.1600-079X.2009.00701.x
Hasan, M. K., Ahammed, G. J., Sun, S., Li, M., Yin, H., and Zhou, J. (2019). Melatonin inhibits cadmium translocation and enhances plant tolerance by regulating sulfur uptake and assimilation in Solanum lycopersicum L. J. Agric. Food Chem. 67, 10563–10576. doi: 10.1021/acs.jafc.9b02404
Hasan, M. K., Ahammed, G. J., Yin, L., Shi, K., Xia, X., Zhou, Y., et al. (2015). Melatonin mitigates cadmium phytotoxicity through modulation of phytochelatins biosynthesis, vacuolar sequestration, and antioxidant potential in Solanum lycopersicum L. Front. Plant Sci. 6:601. doi: 10.3389/fpls.2015.00601
Hasanuzzaman, M., Bhuyan, M. H. M. B., Parvin, K., Bhuiyan, T. F., Anee, T. I., Nahar, K., et al. (2020). Regulation of ROS metabolism in plants under environmental stress: a review of recent experimental evidence. Int. J. Mol. Sci. 21:8695. doi: 10.3390/ijms21228695
Hasanuzzaman, M., Oku, H., Nahar, K., Bhuyan, M., Mahmud, J. A., Baluska, F., et al. (2018). Nitric oxide-induced saltstress tolerance in plants: ROS metabolism, signaling, and molecular interactions. Plant Biotechnol. Rep. 12, 77–92. doi: 10.1007/s11816-018-0480-0
Haskirli, H., Yilmaz, O., Ozgur, R., Uzilday, B., and Turkan, I. (2021). Melatonin mitigates UV-B stress via regulating oxidative stress response, cellular redox and alternative electron sinks in Arabidopsis thaliana. Phytochemistry 182:112592. doi: 10.1016/j.phytochem.2020.112592
Hattori, A., Migitaka, H., Iigo, M., Itoh, M., Yamamoto, K., Ohtani-Kaneko, R., et al. (1995). Identification of melatonin in plants and its effects on plasma melatonin levels and binding to melatonin receptors in vertebrates. Biochem. Mol. Biol. Int. 35, 627–634.
He, H., and He, L. F. (2020). Crosstalk between melatonin and nitric oxide in plant development and stress responses. Physiol. Plant. 170, 218–226. doi: 10.1111/ppl.13143
He, J., Zhuang, X., Zhou, J., Sun, L., Wan, H., Li, H., et al. (2020). Exogenous melatonin alleviates cadmium uptake and toxicity in apple rootstocks. Tree Physiol. 40, 746–761. doi: 10.1093/treephys/tpaa024
Hossain, Z., López-Climent, M. F., Arbona, V., Pérez-Clemente, R. M., and Gómez-Cadenas, A. (2009). Modulation of the antioxidant system in citrus under waterlogging and subsequent drainage. J. Plant Physiol. 166, 1391–1404. doi: 10.1016/j.jplph.2009.02.012
Hosseini, M. S., Samsampour, D., Zahedi, S. M., Zamanian, K., Rahman, M. M., Mostofa, M. G., et al. (2021). Melatonin alleviates drought impact on growth and essential oil yield of lemon verbena by enhancing antioxidant responses, mineral balance, and abscisic acid content. Physiol. Plant. 172, 1363–1375. doi: 10.1111/ppl.13335
Hu, Z., Fan, J., Xie, Y., Amombo, E., Liu, A., Gitau, M. M., et al. (2016). Comparative photosynthetic and metabolic analyses reveal mechanism of improved cold stress tolerance in bermudagrass by exogenous melatonin. Plant Physiol. Biochem. 100, 94–104. doi: 10.1016/j.plaphy.2016.01.008
Hu, W., Zhang, J., Yan, K., Zhou, Z., Zhao, W., Zhang, X., et al. (2021). Beneficial effects of abscisic acid and melatonin in overcoming drought stress in cotton (Gossypium hirsutum L.). Physiol. Plant. 173, 2041–2054. doi: 10.1111/ppl.13550
Huang, B., Chen, Y. E., Zhao, Y. Q., Ding, C. B., Liao, J. Q., Hu, C., et al. (2019). Exogenous melatonin alleviates oxidative damages and protects photosystem II in maize seedlings under drought stress. Front. Plant Sci. 10:677. doi: 10.3389/fpls.2019.00677
Huang, S., VanAken, O., Schwarzländer, M., Belt, K., and Millar, A. H. (2016). The roles of mitochondrial reactive oxygen species in cellular signaling and stress responses in plants. Plant Physiol. 171, 1551–1559. doi: 10.1104/pp.16.00166
Ibrahim, M. F. M., Elbar, O. H. A., Farag, R., Hikal, M., El-Kelish, A., El-Yazied, A. A., et al. (2020). Melatonin counteracts drought induced oxidative damage and stimulates growth, productivity and fruit quality properties of tomato plants. Plan. Theory 9:1276. doi: 10.3390/plants9101276
Imran, M., Aaqil Khan, M., Shahzad, R., Bilal, S., Khan, M., Yun, B.-W., et al. (2021). Melatonin ameliorates thermotolerance in soybean seedling through balancing redox homeostasis and modulating antioxidant defense, phytohormones and polyamines biosynthesis. Molecules 26:5116. doi: 10.3390/molecules26175116
Iqbal, N., Fatma, M., Gautam, H., Umar, S., Sofo, A., D'ippolito, I., et al. (2021). The crosstalk of melatonin and hydrogen sulfide determines photosynthetic performance by regulation of carbohydrate metabolism in wheat under heat stress. Plan. Theory 10:1778. doi: 10.3390/plants10091778
Jahan, M. S., Guo, S., Baloch, A. R., Sun, J., Shu, S., Wang, Y., et al. (2020). Melatonin alleviates nickel phytotoxicity by improving photosynthesis, secondary metabolism and oxidative stress tolerance in tomato seedlings. Ecotoxicol. Environ. Saf. 197:110593. doi: 10.1016/j.ecoenv.2020.110593
Jahan, M. S., Shu, S., Wang, Y., Chen, Z., He, M., Tao, M., et al. (2019). Melatonin alleviates heat-induced damage of tomato seedlings by balancing redox homeostasis and modulating polyamine and nitric oxide biosynthesis. Plant Biol. 19:414. doi: 10.1186/s12870-019-1992-7
Jannatizadeh, A., Aghdam, M. S., Luo, Z., Razavi, F. J. F., and Technology, B. (2019). Impact of exogenous melatonin application on chilling injury in tomato fruits during cold storage. Food Bioprocess Technol. 12, 741–750. doi: 10.1007/s11947-019-2247-1
Jiang, S., Carroll, L., Mariotti, M., Hagglund, P., and Davies, M. J. (2021). Formation of protein cross-links by singlet oxygen-mediated disulfide oxidation. Redox Biol. 41:101874. doi: 10.1016/j.redox.2021.101874
Kang, K., Lee, K., Park, S., Kim, Y. S., and Back, K. (2010). Enhanced production of melatonin by ectopic overexpression of human serotonin N-acetyltransferase plays a role in cold resistance in transgenic rice seedlings. J. Pineal Res. 49, 176–182. doi: 10.1111/j.1600-079X.2010.00783.x
Kaya, C., Higgs, D., Ashraf, M., Alyemeni, M. N., and Ahmad, P. (2020). Integrative roles of nitric oxide and hydrogen sulfide in melatonin-induced tolerance of pepper (Capsicum annuum L.) plants to iron deficiency and salt stress alone or in combination. Physiol. Plant. 168, 256–277. doi: 10.1111/ppl.12976
Kaya, C., Okant, M., Ugurlar, F., Alyemeni, M. N., Ashraf, M., and Ahmad, P. (2019). Melatonin-mediated nitric oxide improves tolerance to cadmium toxicity by reducing oxidative stress in wheat plants. Chemosphere 225, 627–638. doi: 10.1016/j.chemosphere.2019.03.026
Ke, Q., Ye, J., Wang, B., Ren, J., Yin, L., Deng, X., et al. (2018). Melatonin mitigates salt stress in wheat seedlings by modulating polyamine metabolism. Front. Plant Sci. 9:914. doi: 10.3389/fpls.2018.00914
Kerchev, P., Waszczak, C., Lewandowska, A., Willems, P., Shapiguzov, A., and Li, Z. (2016). Lack of GLYCOLATE OXIDASE1, but not GLYCOLATE OXIDASE2, attenuates the photorespiratory phenotype of CATALASE2- deficient Arabidopsis. Plant Physiol. 171, 1704–1719. doi: 10.1104/pp.16.00359
Khan, M. N., Zhang, J., Luo, T., Liu, J., Rizwan, M., Fahad, S., et al. (2019). Seed priming with melatonin coping drought stress in rapeseed by regulating reactive oxygen species detoxification: antioxidant defense system, osmotic adjustment, stomatal traits and chloroplast ultrastructure perseveration. Ind. Crop. Prod. 140:112165. doi: 10.1016/j.indcrop.2019.111597
Kim, C. (2020). ROS-driven oxidative modification: its impact on chloroplasts-nucleus communication. Front. Plant Sci. 10:1729. doi: 10.3389/fpls.2019.01729
Knight, H., Zarka, D. G., Okamoto, H., Thomashow, M. F., and Knight, M. (2004). Abscisic acid induces CBF gene transcription and subsequent induction of cold-regulated genes via the CRT promoter element. Plant Physiol. 135, 1710–1717. doi: 10.1104/pp.104.043562
Kobylińska, A., Borek, S., and Posmyk, M. M. (2018). Melatonin redirects carbohydrates metabolism during sugar starvation in plant cells. J. Pineal Res. 64:e12466. doi: 10.1111/jpi.12466
Kołodziejczyk, I., Bałabusta, M., Szewczyk, R., and Posmyk, M. M. J. A. P. P. (2015). The levels of melatonin and its metabolites in conditioned corn (Zea mays L.) and cucumber (Cucumis sativus L.) seeds during storage. Acta Physiol. Plant. 37:105. doi: 10.1007/s11738-015-1850-7
Kong, X. M., Ge, W. Y., Wei, B. D., Zhou, Q., Zhou, X., Zhao, Y. B., et al. (2020). Melatonin ameliorates chilling injury in green bell peppers during storage by regulating membrane lipid metabolism and antioxidant capacity. Postharvest Biol. Technol. 170:111315. doi: 10.1016/j.postharvbio.2020.111315
Kumar, A., Prasad, A., Sedlarova, M., Kale, R., Frankel, L. K., Sallans, L., et al. (2021). Tocopherol controls D1 amino acid oxidation by oxygen radicals in photosystem II. Proc. Natl. Acad. Sci. U. S. A. 118:e2019246118. doi: 10.1073/pnas.2019246118
Lee, H. J., and Back, K. (2016a). 2-Hydroxymelatonin promotes the resistance of rice plant to multiple simultaneous abiotic stresses (combined cold and drought). J. Pineal Res. 61, 303–316. doi: 10.1111/jpi.12347
Lee, H. Y., and Back, K. (2016b). Mitogen-activated protein kinase pathways are required for melatonin-mediated defense responses in plants. J. Pineal Res. 60, 327–335. doi: 10.1111/jpi.12314
Lee, H. Y., and Back, K. (2017). Melatonin is required for H2O2-and NO-mediated defense signaling through MAPKKK3 and OXI1 in Arabidopsis thaliana. J. Pineal Res. 62:e12379. doi: 10.1111/jpi.12379
Lee, H. Y., and Back, K. (2018). Melatonin induction and its role in high light stress tolerance in Arabidopsis thaliana. J. Pineal Res. 65:e12504. doi: 10.1111/jpi.12504
Lee, H. J., and Back, K. (2019). 2-Hydroxymelatonin confers tolerance against combined cold and drought stress in tobacco, tomato, and cucumber as a potent anti-stress compound in the evolution of land plants. Melatonin Res. 2, 35–46. doi: 10.32794/mr11250020
Lee, H. Y., Byeon, Y., Tan, D. X., Reiter, R. J., and Back, K. (2015). Arabidopsis serotonin N-acetyltransferase knockout plants exhibit decreased melatonin and salicylic acid levels resulting in susceptibility to an avirulent pathogen. J. Pineal Res. 58, 291–299. doi: 10.1111/jpi.12214
Lee, H. Y., Lee, K., and Back, K. (2019). Knockout of Arabidopsis serotonin N-acetyltransferase-2 reduces melatonin levels and delays flowering. Biomol. Ther. 9:712. doi: 10.3390/biom9110712
Lee, K., Zawadzka, A., Czarnocki, Z., Reiter, R. J., and Back, K. (2016). Molecular cloning of melatonin 3-hydroxylase and its production of cyclic 3-hydroxymelatonin in rice (Oryza sativa). J. Pineal Res. 61, 470–478. doi: 10.1111/jpi.12361
Lemos, M., Xiao, Y., Bjornson, M., Wang, J. Z., Hicks, D., Souza, A., et al. (2016). The plastidial retrograde signal methyl erythritol cyclopyrophosphate is a regulator of salicylic acid and jasmonic acid crosstalk. J. Exp. Bot. 67, 1557–1566. doi: 10.1093/jxb/erv550
Lerner, A. B., Case, J. D., Takahashi, Y., Lee, T. H., and Mori, W. (1958). Isolation of melatonin, the pineal gland factor that lightens melanocytes. J. Am. Chem. Soc. 80:2587. doi: 10.1021/ja01543a060
Li, H., Chang, J., Zheng, J., Dong, Y., Liu, Q., Yang, X., et al. (2017). Local melatonin application induces cold tolerance in distant organs of Citrullus lanatus L. via long distance transport. Sci. Rep. 7:40858. doi: 10.1038/srep40858
Li, H., Guo, Y., Lan, Z., Xu, K., Chang, J., Ahammed, G. J., et al. (2021). Methyl jasmonate mediates melatonin-induced cold tolerance of grafted watermelon plants. Hortic. Res. 8:57. doi: 10.1038/S41438-021-00496-0
Li, S., Guo, J., Wang, T., Gong, L., Liu, F., Brestic, M., et al. (2021). Melatonin reduces nanoplastic uptake, translocation, and toxicity in wheat. J. Pineal Res. 71:e12761. doi: 10.1111/jpi.12761
Li, M., and Kim, C. (2021). Chloroplast ROS and stress signaling. Plant Commun. 3:100264. doi: 10.1016/j.xplc.2021.100264
Li, C., Liang, B., Chang, C., Wei, Z., Zhou, S., and Ma, F. (2016). Exogenous melatonin improved potassium content in Malus under different stress conditions. J. Pineal Res. 61, 218–229. doi: 10.1111/jpi.12342
Li, G. Z., Liu, J., Chen, S. J., Wang, P. F., Liu, H. T., Dong, J., et al. (2021). Melatonin promotes potassium deficiency tolerance by regulating HAK1 transporter and its upstream transcription factor NAC71 in wheat. J. Pineal Res. 70:e12727. doi: 10.1111/jpi.12727
Li, C., Tan, D. X., Liang, D., Chang, C., Jia, D., and Ma, F. (2015). Melatonin mediates the regulation of ABA metabolism, free-radical scavenging, and stomatal behaviour in two Malus species under drought stress. J. Exp. Bot. 66, 669–680. doi: 10.1093/jxb/eru476
Li, C., Wang, P., Wei, Z., Liang, D., Liu, C., Yin, L., et al. (2012). The mitigation effects of exogenous melatonin on salinity-induced stress in Malus hupehensis. J. Pineal Res. 53, 298–306. doi: 10.1111/j.1600-079X.2012.00999.x
Li, X., Yu, B., Cui, Y., and Yin, Y. (2017). Melatonin application confers enhanced salt tolerance by regulating Na+ and Cl− accumulation in rice. Plant Growth Regul. 83, 441–454. doi: 10.1007/s10725-017-0310-3
Liang, D., Gao, F., Ni, Z., Lin, L., Deng, Q., Tang, Y., et al. (2018). Melatonin improves heat tolerance in kiwifruit seedlings through promoting antioxidant enzymatic activity and glutathione S-Transferase transcription. Molecules 23:584. doi: 10.3390/molecules23030584
Liang, C., Li, A., Yu, H., Li, W., Liang, C., Guo, S., et al. (2017). Melatonin regulates root architecture by modulating auxin response in rice. Front. Plant Sci. 8:134. doi: 10.3389/fpls.2017.00134
Liang, C., Zheng, G., Li, W., Wang, Y., Hu, B., Wang, H., et al. (2015). Melatonin delays leaf senescence and enhances salt stress tolerance in rice. J. Pineal Res. 59, 91–101. doi: 10.1111/jpi.12243
Liao, L., Zhou, Y., Xu, Y., Zhang, Y., Liu, X., Liu, B., et al. (2021). Structural and molecular dynamics analysis of plant serotonin N-Acetyltransferase reveal an acid/base-assisted catalysis in melatonin biosynthesis. Angew. Chem. Int. Ed. Eng. 133, 12127–12133. doi: 10.1002/ange.202100992
Liu, D. D., Sun, X. S., Liu, L., Shi, H. D., Chen, S. Y., and Zhao, D. K. (2019). Overexpression of the melatonin synthesis-related gene SlCOMT1 improves the resistance of tomato to salt stress. Molecules 24:1514. doi: 10.3390/molecules24081514
Liu, J., Wang, W., Wang, L., and Sun, Y. (2015). Exogenous melatonin improves seedling health index and drought tolerance in tomato. Plant Growth Regul. 77, 317–326. doi: 10.1007/s10725-015-0066-6
Lv, R., Li, Z., Li, M., Dogra, V., Lv, S., Liu, R., et al. (2019). Uncoupled expression of nuclear and plastid photosynthesis-associated genes contributes to cell death in a lesion mimic mutant. Plant Cell 31, 210–230. doi: 10.1105/tpc.18.00813
Ma, X., Idle, J. R., Krausz, K. W., and Gonzalez, F. J. (2005). Metabolism of melatonin by human cytochromes p450. Drug Metab. Dispos. 33, 489–494. doi: 10.1124/dmd.104.002410
Manchester, L. C., Coto-Montes, A., Boga, J. A., Andersen, L. P. H., Zhou, Z., Galano, A., et al. (2015). Melatonin: an ancient molecule that makes oxygen metabolically tolerable. J. Pineal Res. 59, 403–419. doi: 10.1111/jpi.12267
Martínez, M. C., and Andriantsitohaina, R. (2009). Reactive nitrogen species: molecular mechanisms and potential significance in health and disease. Antioxid. Redox Signal. 11, 669–702. doi: 10.1089/ars.2007.1993
Meng, J., Xu, T., Wang, Z., Fang, Y., Xi, Z., and Zhang, Z. (2014). The ameliorative effects of exogenous melatonin on grape cuttings under water-deficient stress: antioxidant metabolites, leaf anatomy, and chloroplast morphology. J. Pineal Res. 57, 200–212. doi: 10.1111/jpi.12159
Moustafa-Farag, M., Mahmoud, A., Arnao, M. B., Sheteiwy, M. S., Dafea, M., Soltan, M., et al. (2020). Melatonin-induced water stress tolerance in plants: recent advances. Antioxidants 9:809. doi: 10.3390/antiox9090809
Mukherjee, S. (2019). Recent advancements in the mechanism of nitric oxide signaling associated with hydrogen sulfide and melatonin crosstalk during ethylene-induced fruit ripening in plants. Nitric Oxide 82, 25–34. doi: 10.1016/j.niox.2018.11.003
Mukherjee, S., David, A., Yadav, S., Baluška, F., and Bhatla, S. (2014). Salt stress-induced seedling growth inhibition coincides with differential distribution of serotonin and melatonin in sunflower seedling roots and cotyledons. Physiol. Plant. 152, 714–728. doi: 10.1111/ppl.12218
Munns, R., and Tester, M. (2008). Mechanisms of salinity tolerance. Annu. Rev. Plant Biol. 59, 651–681. doi: 10.1146/annurev.arplant.59.032607.092911
Nabi, R. B. S., Tayade, R., Hussain, A., Kulkarni, K. P., Imran, Q. M., Mun, B. G., et al. (2019). Nitric oxide regulates plant responses to drought, salinity, and heavy metal stress. Environ. Exp. Bot. 161, 120–133. doi: 10.1016/j.envexpbot.2019.02.003
Nagajyoti, P. C., Lee, K. D., and Sreekanth, T. V. M. (2010). Heavy metals, occurrence and toxicity for plants: a review. Environ. Chem. Lett. 8, 199–216. doi: 10.1007/s10311-010-0297-8
Naing, A. H., and Kim, C. K. (2021). Abiotic stress-induced anthocyanins in plants: their role in tolerance to abiotic stresses. Physiol. Plant. 172, 1711–1723. doi: 10.1111/ppl.13373
Namdjoyan, S., Soorki, A. A., Elyasi, N., Kazemi, N., and Simaei, M. (2019). Melatonin alleviates lead-induced oxidative damage in safflower (Carthamus tinctorius L.) seedlings. Ecotoxicology 29, 108–118. doi: 10.1007/s10646-019-02136-9
Nawaz, M. A., Jiao, Y., Chen, C., Shireen, F., Zheng, Z., Imtiaz, M., et al. (2018). Melatonin pretreatment improves vanadium stress tolerance of watermelon seedlings by reducing vanadium concentration in the leaves and regulating melatonin biosynthesis and antioxidant-related gene expression. J. Plant Physiol. 220, 115–127. doi: 10.1016/j.jplph.2017.11.003
Nazir, M., Asad Ullah, M., Mumtaz, S., Siddiquah, A., Shah, M., Drouet, S., et al. (2020). Interactive effect of melatonin and UV-C on phenylpropanoid metabolite production and antioxidant potential in callus cultures of purple basil (Ocimum basilicum L. var. purpurascens). Molecules 25:1072. doi: 10.3390/molecules25051072
Nguyen, H., Lin, K., Ho, S., Chiang, C., and Yang, C. (2018). Enhancing the abiotic stress tolerance of plants: from chemical treatment to biotechnological approaches. Physiol. Plant. 164, 452–466. doi: 10.1111/ppl.12812
Ni, J., Wang, Q., Shah, F. A., Liu, W., Wang, D., Huang, S., et al. (2018). Exogenous melatonin confers cadmium tolerance by counterbalancing the hydrogen peroxide homeostasis in wheat seedlings. Molecules 23:799. doi: 10.3390/molecules23040799
Okant, M., and Kaya, C. (2019). The role of endogenous nitric oxide in melatonin-improved tolerance to lead toxicity in maize plants. Environ. Sci. Pollut. Res. Int. 26, 11864–11874. doi: 10.1007/s11356-019-04517-3
Okazaki, M., Higuchi, K., Aouini, A., and Ezura, H. (2010). Lowering intercellular melatonin levels by transgenic analysis of indoleamine 2, 3-dioxygenase from rice in tomato plants. J. Pineal Res. 49, 239–247. doi: 10.1111/j.1600-079X.2010.00788.x
Pardo-Hernández, M., López-Delacalle, M., and Rivero, R. M. (2020). ROS and NO regulation by melatonin under abiotic stress in plants. Antioxidants 9:1078. doi: 10.3390/antiox9111078
Park, H. S., Kazerooni, E. A., Kang, S. M., Al-Sadi, A. M., and Lee, I. J. (2021). Melatonin enhances the tolerance and recovery mechanisms in Brassica juncea (L.) Czern. Under saline conditions. Front. Plant Sci. 12:593717. doi: 10.3389/fpls.2021.593717
Postiglione, A. E., and Muday, G. K. (2020). The role of ROS homeostasis in ABA-induced guard cell signaling. Front. Plant Sci. 11:968. doi: 10.3389/fpls.2020.00968
Qi, Z. Y., Wang, K. X., Yan, M. Y., Kanwar, M. K., Li, D. Y., Wijaya, L., et al. (2018). Melatonin alleviates high temperature-induced pollen abortion in Solanum lycopersicum. Molecules 23:386. doi: 10.3390/molecules23020386
Qi, H., Xia, F. N., and Xiao, S. (2021). Autophagy in plants: physiological roles and post-translational regulation. J. Integr. Plant Biol. 63, 161–179. doi: 10.1111/jipb.12941
Ray, P. D., Huang, B. W., and Tsuji, Y. (2012). Reactive oxygen species (ROS) homeostasis and redox regulation in cellular signaling. Cell. Signal. 24, 981–990. doi: 10.1016/j.cellsig.2012.01.008
Ren, J., Ye, J., Yin, L., Li, G., Deng, X., and Wang, S. (2020). Exogenous melatonin improves salt tolerance by mitigating osmotic, ion, and oxidative stresses in maize seedlings. Agronomy 10:663. doi: 10.3390/agronomy10050663
Sadak, M. S., and Bakry, B. A. (2020). Alleviation of drought stress by melatonin foliar treatment on two flax varieties under sandy soil. Physiol. Mol. Biol. Plants 26, 907–919. doi: 10.1007/s12298-020-00789-z
Saddhe, A. A., Malvankar, M. R., Karle, S. B., and Kumar, K. (2019). Reactive nitrogen species: paradigms of cellular signaling and regulation of salt stress in plants. Environ. Exp. Bot. 161, 86–97. doi: 10.1016/j.envexpbot.2018.11.010
Samanta, S., Banerjee, A., and Roychoudhury, A. (2021). Exogenous melatonin regulates endogenous phytohormone homeostasis and thiol-mediated detoxification in two indica rice cultivars under arsenic stress. Plant Cell Rep. 40, 1585–1602. doi: 10.1007/s00299-021-02711-7
Semak, I., Naumova, M., Korik, E., Terekhovich, V., Wortsman, J., and Slominski, A. (2005). A novel metabolic pathway of melatonin: oxidation by cytochrome C. Biochemistry 44, 9300–9307. doi: 10.1021/bi050202d
Shah, A. A., Ahmed, S., Ali, A., and Yasin, N. A. (2020). 2-Hydroxymelatonin mitigates cadmium stress in cucumis sativus seedlings: modulation of antioxidant enzymes andpolyamines. Chemosphere 243:125308. doi: 10.1016/j.chemosphere.2019.125308
Sharma, S. S., and Dietz, K. J. (2009). The relationship between metal toxicity and cellular redox imbalance. Trends Plant Sci. 14, 43–50. doi: 10.1016/j.tplants.2008.10.007
Shen, J., Chen, D., Zhang, X., Song, L., Dong, J., Xu, Q., et al. (2021). Mitigation of salt stress response in upland cotton (Gossypium hirsutum) by exogenous melatonin. J. Plant Res. 134, 857–871. doi: 10.1007/s10265-021-01284-6
Shen, N., Wang, T., Gan, Q., Liu, S., Wang, L., and Jin, B. (2022). Plant flavonoids: classification, distribution, biosynthesis, and antioxidant activity. Food Chem. 383:132531. doi: 10.1016/j.foodchem.2022.132531
Shi, H., Jiang, C., Ye, T., Tan, D. X., Reiter, R. J., Zhang, H., et al. (2015a). Comparative physiological, metabolomic, and transcriptomic analyses reveal mechanisms of improved abiotic stress resistance in bermudagrass [Cynodon dactylon (L). Pers.] by exogenous melatonin. J. Exp. Bot. 66, 681–694. doi: 10.1093/jxb/eru373
Shi, H., Tan, D. X., Reiter, R. J., Ye, T., Yang, F., and Chan, Z. J. (2015b). Melatonin induces class A1 heat-shock factors (HSFA1s) and their possible involvement of thermotolerance in Arabidopsis. J. Pineal Res. 58, 335–342. doi: 10.1111/jpi.12219
Su, J., Yang, X., Shao, Y., Chen, Z., and Shen, W. (2021). Molecular hydrogen–induced salinity tolerance requires melatonin signalling in Arabidopsis thaliana. Plant Cell Environ. 44, 476–490. doi: 10.1111/pce.13926
Sun, C., Liu, L., Wang, L., Li, B., Jin, C., and Lin, X. (2021). Melatonin: a master regulator of plant development and stress responses. J. Integr. Plant Biol. 63, 126–145. doi: 10.1111/jipb.12993
Sun, C., Lv, T., Huang, L., Liu, X., Jin, C., and Lin, X. (2020). Melatonin ameliorates aluminum toxicity through enhancing aluminum exclusion and reestablishing redox homeostasis in roots of wheat. J. Pineal Res. 68:e12642. doi: 10.1111/jpi.12642
Tan, D. X., Hardeland, R., Back, K., Manchester, L. C., Alatorre-Jimenez, M. A., and Reiter, R. J. (2016). On the significance of an alternate pathway of melatonin synthesis via 5-methoxytryptamine: comparisons across species. J. Pineal Res. 61, 27–40. doi: 10.1111/jpi.12336
Tan, D. X., Hardeland, R., Manchester, L. C., Korkmaz, A., Ma, S., Rosales-Corral, S., et al. (2012). Functional roles of melatonin in plants, and perspectives in nutritional and agricultural science. J. Exp. Bot. 63, 577–597. doi: 10.1093/jxb/err256
Tan, D. X., Manchester, L. C., Di Mascio, P., Martinez, G. R., Prado, F. M., and Reiter, R. J. (2007). Novel rhythms of N1-acetyl-N2-formyl-5-methoxykynuramine and its precursor melatonin in water hyacinth: importance for phytoremediation. FASEB J. 21, 1724–1729. doi: 10.1096/fj.06-7745com
Tan, D. X., Manchester, L. C., Liu, X., Rosales-Corral, S. A., Acuna-Castroviejo, D., and Reiter, R. J. (2013). Mitochondria and chloroplasts as the original sites of melatonin synthesis: a hypothesis related to melatonin’s primary function and evolution in eukaryotes. J. Pineal Res. 54, 127–138. doi: 10.1111/jpi.12026
Tan, D. X., and Reiter, R. J. (2020). An evolutionary view of melatonin synthesis and metabolism related to its biological functions in plants. J. Exp. Bot. 71, 4677–4689. doi: 10.1093/jxb/eraa235
Tang, M., Xu, L., Wang, Y., Dong, J., Zhang, X., Wang, K., et al. (2021). Melatonin-induced DNA demethylation of metal transporters and antioxidant genes alleviates lead stress in radish plants. Hortic. Res. 8:124. doi: 10.1038/s41438-021-00561-8
Tiwari, R. K., Lal, M. K., Kumar, R., Chourasia, K. N., Naga, K. C., Kumar, D., et al. (2021). Mechanistic insights on melatonin-mediated drought stress mitigation in plants. Physiol. Plant. 172, 1212–1226. doi: 10.1111/ppl.13307
Tiwari, R. K., Lal, M. K., Naga, K. C., Kumar, R., Chourasia, K. N., Subhash, S., et al. (2020). Emerging roles of melatonin in mitigating abiotic and biotic stresses of horticultural crops. Sci. Hortic. 272:109592. doi: 10.1016/j.scienta.2020.109592
Van Zelm, E., Zhang, Y., and Testerink, C. (2020). Salt tolerance mechanisms of plants. Annu. Rev. Plant Biol. 71, 403–433. doi: 10.1146/annurev-arplant-050718-100005
Wang, K., Cai, S., Xing, Q., Qi, Z., Fotopoulos, V., Yu, J., et al. (2022). Melatonin delays dark-induced leaf senescence by inducing miR171b expression in tomato. J. Pineal Res. 72:e12792. doi: 10.1111/jpi.12792
Wang, D., Chen, Q., Chen, W., Guo, Q., Xia, Y., Wu, D., et al. (2021). Melatonin treatment maintains quality and delays lignification in loquat fruit during cold storage. Sci. Hortic. 284:110126. doi: 10.1016/j.scienta.2021.110126
Wang, M., Duan, S., Zhou, Z., Chen, S., and Wang, D. (2019). Foliar spraying of melatonin confers cadmium tolerance in Nicotiana tabacum L. Ecotoxicol. Environ. Saf. 170, 68–76. doi: 10.1016/j.ecoenv.2018.11.127
Wang, L., Feng, C., Zheng, X., Guo, Y., Zhou, F., Shan, D., et al. (2017). Plant mitochondria synthesize melatonin and enhance the tolerance of plants to drought stress. J. Pineal Res. 63:e12429. doi: 10.1111/jpi.12429
Wang, Y., Mostafa, S., Zeng, W., and Jin, B. (2021). Function and mechanism of jasmonic acid in plant responses to abiotic and biotic stresses. Int. J. Mol. Sci. 22:8568. doi: 10.3390/ijms22168568
Wang, Y., Reiter, R. J., and Chan, Z. (2018). Phytomelatonin: a universal abiotic stress regulator. J. Exp. Bot. 69, 963–974. doi: 10.1093/jxb/erx473
Wang, X., Zhang, H., Xie, Q., Liu, Y., Lv, H., Bai, R., et al. (2020). SlSNAT interacts with HSP40, a molecular chaperone, to regulate melatonin biosynthesis and promote thermotolerance in tomato. Plant Cell Physiol. 61, 909–921. doi: 10.1093/pcp/pcaa018
Weeda, S., Zhang, N., Zhao, X., Ndip, G., Guo, Y., Buck, G. A., et al. (2014). Arabidopsis transcriptome analysis reveals key roles of melatonin in plant defense systems. PLoS One 9:e93462. doi: 10.1371/journal.pone.0093462
Wei, Z., Li, C., Gao, T., Zhang, Z., Liang, B., Lv, Z., et al. (2019). Melatonin increases the performance of Malus hupehensis after UV-B exposure. Plant Physiol. Biochem. 139, 630–641. doi: 10.1016/j.plaphy.2019.04.026
Weissbach, H., Redfield, B. G., and Axelrod, J. (1960). Biosynthesis of melatonin: enzymic conversion of serotonin to N-acetylserotonin. Biochim. Biophys. Acta 43, 352–353. doi: 10.1016/0006-3002(60)90453-4
Wen, D., Gong, B., Sun, S., Liu, S., Wang, X., Wei, M., et al. (2016). Promoting roles of melatonin in adventitious root development of Solanum lycopersicum L. by regulating auxin and nitric oxide signaling. Front. Plant Sci. 7:718. doi: 10.3389/fpls.2016.00718
Wu, Y., Fan, X., Zhang, Y., Jiang, J., Sun, L., Rahman, F. U., et al. (2021). VvSNAT1 overexpression enhances melatonin production and salt tolerance in transgenic Arabidopsis. Plant Physiol. Biochem. 166, 485–494. doi: 10.1016/j.plaphy.2021.06.025
Wu, Q., Su, N., Huang, X., Cui, J., Shabala, L., Zhou, M., et al. (2021). Hypoxia-induced increase in GABA content is essential for restoration of membrane potential and preventing ROS-induced disturbance to ion homeostasis. Plant Commun. 2:100188. doi: 10.1016/j.xplc.2021.100188
Xu, W., Cai, S. Y., Zhang, Y., Wang, Y., Ahammed, G. J., Xia, X. J., et al. (2016). Melatonin enhances thermotolerance by promoting cellular protein protection in tomato plants. J. Pineal Res. 61, 457–469. doi: 10.1111/jpi.12359
Xu, L., Xiang, G., Sun, Q., Ni, Y., Jin, Z., Gao, S., et al. (2019). Melatonin enhances salt tolerance by promoting MYB108A-mediated ethylene biosynthesis in grapevines. Hortic. Res. 6:114. doi: 10.1038/s41438-019-0197-4
Yan, F., Wei, H., Ding, Y., Li, W., Liu, Z., Chen, L., et al. (2021). Melatonin regulates antioxidant strategy in response to continuous salt stress in rice seedlings. Plant Physiol. Biochem. 165, 239–250. doi: 10.1016/j.plaphy.2021.05.003
Yang, H., Dai, L., Wei, Y., Deng, Z., and Li, D. (2019). Melatonin enhances salt stress tolerance in rubber tree (Hevea brasiliensis) seedlings. Ind. Crop. Prod. 145:111990. doi: 10.1016/j.indcrop.2019.111990
Yang, S. J., Huang, B., Zhao, Y. Q., Hu, D., Chen, T., Ding, C. B., et al. (2021). Melatonin enhanced the tolerance of Arabidopsis thaliana to high light through improving anti-oxidative system and photosynthesis. Front. Plant Sci. 12:752584. doi: 10.3389/fpls.2021.752584
Yang, Y., Yao, Y., Li, J., Zhang, J., Zhang, X., Hu, L., et al. (2022). Trehalose alleviated salt stress in tomato by regulating ROS metabolism, photosynthesis, osmolyte synthesis, and trehalose metabolic pathways. Front. Plant Sci. 13:772948. doi: 10.3389/fpls.2022.772948
Yao, J. W., Ma, Z., Ma, Y. Q., Zhu, Y., Lei, M. Q., Hao, C. Y., et al. (2021). Role of melatonin in UV-B signaling pathway and UV-B stress resistance in Arabidopsis thaliana. Plant Cell Environ. 44, 114–129. doi: 10.1111/pce.13879
Ye, T., Yin, X., Yu, L., Zheng, S. J., Cai, W. J., Wu, Y., et al. (2019). Metabolic analysis of the melatonin biosynthesis pathway using chemical labeling coupled with liquid chromatography-mass spectrometry. J. Pineal Res. 66:e12531. doi: 10.1111/jpi.12531
Yin, Y., Tian, X., Yang, J., Yang, Z., Tao, J., and Fang, W. (2022). Melatonin mediates isoflavone accumulation in germinated soybeans (Glycine max L.) under ultraviolet-B stress. Plant Physiol. Biochem. 175, 23–32. doi: 10.1016/j.plaphy.2022.02.001
Yu, Y., Lv, Y., Shi, Y., Li, T., Chen, Y., Zhao, D., et al. (2018). The role of phyto-melatonin and related metabolites in response to stress. Molecules 23:1887. doi: 10.3390/molecules23081887
Zhan, H., Nie, X., Zhang, T., Li, S., Wang, X., Du, X., et al. (2019). Melatonin: a small molecule but important for salt stress tolerance in plants. Int. J. Mol. Sci. 20:709. doi: 10.3390/ijms20030709
Zhang, J. Y., Cruz De Carvalho, M. H., Torres-Jerez, I., Kang, Y., Allen, S. N., Huhman, D. V., et al. (2014). Global reprogramming of transcription and metabolism in Medicago truncatula during progressive drought and after rewatering. Plant Cell Environ. 37, 2553–2576. doi: 10.1111/pce.12328
Zhang, Y., Fan, Y., Rui, C., Zhang, H., Xu, N., Dai, M., et al. (2021). Melatonin improves cotton salt tolerance by regulating ROS scavenging system and Ca2+ signal transduction. Front. Plant Sci. 12:693690. doi: 10.3389/fpls.2021.693690
Zhang, J., Li, D., Wei, J., Ma, W., Kong, X., Rengel, Z., et al. (2019). Melatonin alleviates aluminum-induced root growth inhibition by interfering with nitric oxide production in Arabidopsis. Environ. Exp. Bot. 161, 157–165. doi: 10.1016/j.envexpbot.2018.08.014
Zhang, Q., Liu, X., Zhang, Z., Liu, N., Li, D., and Hu, L. (2019). Melatonin improved waterlogging tolerance in alfalfa (Medicago sativa) by reprogramming polyamine and ethylene metabolism. Front. Plant Sci. 10:44. doi: 10.3389/fpls.2019.00044
Zhang, J., Shi, Y., Zhang, X., Du, H., Xu, B., and Huang, B. (2017). Melatonin suppression of heat-induced leaf senescence involves changes in abscisic acid and cytokinin biosynthesis and signaling pathways in perennial ryegrass (Lolium perenne L.). Environ. Exp. Bot. 138, 36–45. doi: 10.1016/j.envexpbot.2017.02.012
Zhang, N., Sun, Q., Zhang, H., Cao, Y., Weeda, S., Ren, S., et al. (2015). Roles of melatonin in abiotic stress resistance in plants. J. Exp. Bot. 66, 647–656. doi: 10.1093/jxb/eru336
Zhang, H., Wang, L., Shi, K., Shan, D., Zhu, Y., Wang, C., et al. (2019). Apple tree flowering is mediated by low level of melatonin under the regulation of seasonal light signal. J. Pineal Res. 66:e12551. doi: 10.1111/jpi.12551
Zhang, R., Yue, Z., Chen, X., Wang, Y., Zhou, Y., Xu, W., et al. (2021). Foliar applications of urea and melatonin to alleviate waterlogging stress on photosynthesis and antioxidant metabolism in sorghum seedlings. Plant Growth Regul. 1–10. doi: 10.1007/s10725-021-00705-9
Zhang, N., Zhang, H. J., Sun, Q. Q., Cao, Y. Y., Li, X., Zhao, B., et al. (2017). Proteomic analysis reveals a role of melatonin in promoting cucumber seed germination under high salinity by regulating energy production. Sci. Rep. 7:503. doi: 10.1038/s41598-017-00566-1
Zhang, H., Zhang, N., Yang, R., Wang, L., Sun, Q., Li, D., et al. (2014). Melatonin promotes seed germination under high salinity by regulating antioxidant systems, ABA and GA 4 interaction in cucumber (Cucumis sativus L.). J. Pineal Res. 57, 269–279. doi: 10.1111/jpi.12167
Zhang, X., Zhang, H., Zhang, H., and Tang, M. (2020). Exogenous melatonin application enhances rhizophagus irregularis symbiosis and induces the antioxidant response of Medicago truncatula under lead stress. Front. Microbiol. 11:516. doi: 10.3389/fmicb.2020.00516
Zhao, J., Lu, Z., Wang, L., and Jin, B. (2020). Plant responses to heat stress: physiology, transcription, noncoding RNAs, and epigenetics. Int. J. Mol. Sci. 22:117. doi: 10.3390/ijms22010117
Zhao, D., Yao, Z., Zhang, J., Zhang, R., Mou, Z., Zhang, X., et al. (2021). Melatonin synthesis genes N-acetylserotonin methyltransferases evolved into caffeic acid O-methyltransferases and both assisted in plant terrestrialization. J. Pineal Res. 71:e12737. doi: 10.1111/jpi.12737
Zhao, D., Yu, Y., Shen, Y., Liu, Q., Zhao, Z., Sharma, R., et al. (2019). Melatonin synthesis and function: evolutionary history in animals and plants. Front. Endocrinol. 10:249. doi: 10.3389/fendo.2019.00249
Zhao, H., Zhang, K., Zhou, X., Xi, L., Wang, Y., Xu, H., et al. (2017). Melatonin alleviates chilling stress in cucumber seedlings by up-regulation of CsZat12 and modulation of polyamine and abscisic acid metabolism. Sci. Rep. 7:4998. doi: 10.1038/s41598-017-05267-3
Zhao, G., Zhao, Y., Yu, X., Kiprotich, F., Han, H., Guan, R., et al. (2018). Nitric oxide is required for melatonin-enhanced tolerance against salinity stress in rapeseed (Brassica napus L.) seedlings. Int. J. Mol. Sci. 19:1912. doi: 10.3390/ijms19071912
Zheng, X., Zhou, J., Tan, D. X., Wang, N., Wang, L., Shan, D., et al. (2017). Melatonin improves waterlogging tolerance of Malus baccata (Linn.) Borkh. Seedlings by maintaining aerobic respiration, photosynthesis and ROS migration. Front. Plant Sci. 8:483. doi: 10.3389/fpls.2017.00483
Keywords: melatonin, abiotic stress, stress tolerance, exogenous applications, biosynthesis, metabolism, antioxidants, molecular signaling
Citation: Zeng W, Mostafa S, Lu Z and Jin B (2022) Melatonin-Mediated Abiotic Stress Tolerance in Plants. Front. Plant Sci. 13:847175. doi: 10.3389/fpls.2022.847175
Edited by:
Guo-Liang Jiang, Virginia State University, United StatesReviewed by:
Xin Li, Tea Research Institute (CAAS), ChinaMirza Hasanuzzaman, Sher-e-Bangla Agricultural University, Bangladesh
Copyright © 2022 Zeng, Mostafa, Lu and Jin. This is an open-access article distributed under the terms of the Creative Commons Attribution License (CC BY). The use, distribution or reproduction in other forums is permitted, provided the original author(s) and the copyright owner(s) are credited and that the original publication in this journal is cited, in accordance with accepted academic practice. No use, distribution or reproduction is permitted which does not comply with these terms.
*Correspondence: Zhaogeng Lu, emdsdUB5enUuZWR1LmNu; Biao Jin, YmppbkB5enUuZWR1LmNu