- 1Xinjiang Key Laboratory of Desert Plant Roots Ecology and Vegetation Restoration, Xinjiang Institute of Ecology and Geography, Chinese Academy of Sciences, Urumqi, China
- 2State Key Laboratory of Desert and Oasis Ecology, Xinjiang Institute of Ecology and Geography, Chinese Academy of Sciences, Urumqi, China
- 3Cele National Station of Observation and Research for Desert-Grassland Ecosystems, Cele, China
- 4University of Chinese Academy of Sciences, Beijing, China
The allocation patterns of foliar phosphorus (P) fractions across various vegetation types generally reflect the adaptability to P-impoverished environments. However, the allocation of foliar-P fractions within the desert herb Karelinia caspia (K. caspica) and shrub Tamarix ramosissima (T. ramosissima) in soils with different environment-P availability and the impact of soil and groundwater properties on foliar-P fractions allocation remain unclear. The foliar-P fractions (metabolites-P, nucleic acid-P, structural-P, and residual-P) of K. caspica and T. ramosissima and the properties of 0–60 cm deep soil under their canopy and groundwater were determined at four different environment-P sites. Results found that as environment-P availability decreased, both plants allocated the higher proportions of foliar-P to nucleic acid-P than to metabolites-P and structural-P. With the exception of residual-P, foliar-P fractions were markedly higher for K. caspica than T. ramosissima. Soil Olsen-P, NO3–-N, soil water content, electrical conductivity (EC), groundwater EC, and total dissolved solids (TDSs) played an important role in allocating foliar P-fractions for both K. caspica and T. ramosissima. Compared with K. caspica, the foliar-P fractions of T. ramosissima were more tightly bounded to groundwater than soil properties. Overall, these findings show how desert plants flexibility take advantage of the foliar-P in low environment-P availability and illustrate the foliar-P fractions allocation of desert plants is driven by soil and groundwater properties.
Introduction
Phosphorus (P) is an essential macronutrient for plants, helping to regulate the metabolism, transportation, and improving the drought resistance of plants (Dobrota, 2004). However, P deficiency in desert ecosystems is a common phenomenon (Hou et al., 2020; Xia et al., 2020). The desert-oasis transition zone on the southern edge of the Taklimakan Desert is a hyper-arid and nutrient-impoverished desert ecosystem (Gao et al., 2022). Vegetation in this region is dominated by sparse perennial herbs and shrubs, which form dominant and co-dominant stands and are important to the security of dunes in the region (Bruelheide et al., 2010; Xue et al., 2019; Zhang et al., 2020). A prior research has found that groundwater is the main source of water and nutrients for deep-rooted desert vegetation in this area (Zeng et al., 2013; Li et al., 2021). However, the annual decline of groundwater table in recent years has brought serious challenges to the vegetation safety in this area (Zeng et al., 2013). Thus, it is crucial to understand the mechanisms that allow flora growing in the juncture zone to accommodate P-impoverished conditions to prevent vegetation degradation and maintain the stability of the juncture zone.
Plants generally respond to P-deficient environments by reducing the leaf P content (Epstein and Bloom, 2005). However, leaf P content is the total of various foliar-P fractions in leaf cells, such as metabolites-P, nucleic acid-P, structural-P, and residual-P (Veneklaas et al., 2012). When faced with low soil-P availability, plants acclimate by shifting the allocation of P between foliar-P fractions (Hidaka and Kitayama, 2011; Yan et al., 2019). Gao et al. (2022) investigated foliar-P fractions in the desert plant, Alhagi sparsifolia, along a soil-P availability gradient on the desert-oasis transition zone in Xinjiang, China. In P-impoverished soils, A. sparsifolia reduced the metabolites-P content but increased the nucleic acid-P content. In addition, foliar-P fractions allocation may be related to life history because these fractions are functionally associated with growth and reproduction (Hidaka and Kitayama, 2011; Han et al., 2021). For example, Han et al. (2021) found that faster-growing tree species allocate more foliar-P to nucleic acid-P than slow-growing tree species. According to the growth-rate hypothesis (GRH), fast-growing plants need to allocate more foliar-P for protein synthesis and enzyme-related nucleic acid-P (RNA, especially rRNA) to supply photosynthesis and other physiological needs (Elser and Hamilton, 2007; Reef et al., 2010; Hidaka and Kitayama, 2011). A global study on leaf nutrients showed that the leaf P content of faster-growing herbs was significantly higher than in other functional groups (Yuan and Chen, 2009).
The herbaceous perennial, Karelinia caspia (K. caspica), and the shrub, Tamarix ramosissima (T. ramosissima), are endemic to the Qira oasis in the Taklimakan Desert (Li et al., 2021; Gao et al., 2022). K. caspica is an excellent forage resource that grows relatively fast, and T. ramosissima is relatively slow-growing. T. ramosissima soil is used by residents to produce the parasitic medicinal plant, Cistanche tubulosa (Zhang et al., 2019). Thus, exploring the P utilization of K. caspica and T. ramosissima is beneficial to ecology and the local economy. Previous studies have shown that K. caspica and T. ramosissima play a significant role in preventing the land desertification and reducing sand dune movement attributed to “dune” formation (Thompson et al., 2006; Li et al., 2007; Meglioli et al., 2017). A “dune” is formed by the litter of some perennial herbs or shrubs as well as sand movement in desert ecosystems (Throop and Archer, 2008; Sun et al., 2017). In this process of dune formation, plant litter rapidly releases nutrients in response to the special micro-environment under the canopy and forms a “fertile island” (Titus et al., 2002; Thompson et al., 2006; Yao et al., 2019). The creation of fertile islands has been widely reported worldwide and is shown to improve the chemical [e.g., soil organic carbon and total nitrogen (TN)] and biological (e.g., urease and alkaline phosphatase activity) conditions of soil (Meglioli et al., 2017; Yao et al., 2019). The nutrient status of fertile islands formed by diverse vegetation differs based on the litter nutrient concentration, mineralization capacity, and the unique microbial communities of different plants (Innes et al., 2004; Li et al., 2007). Thus, it is worth considering differences in the soil properties of fertile islands formed by K. caspica and T. ramosissima and how they affect the allocation of foliar-P fractions.
Prior studies have shown that desert ecosystems often have more extensive and deeper roots than vegetation in other ecosystems, likely due to scarce precipitation (Li et al., 2010; Yao et al., 2019). These root characteristics enable desert vegetation to obtain more water and nutrients from both soil and groundwater (Zhang et al., 2018; Li et al., 2021). This feature is attributed to many perennial plants depending on the availability of groundwater in hyper-arid desert ecosystems (Thomas et al., 2006; Bruelheide et al., 2010). Some researchers believe that groundwater is the primary source of water and nutrients for desert vegetation (Gries et al., 2003; Zeng et al., 2013). However, most of the root system of desert vegetation is distributed in the soil, and plant roots only obtain water and nutrients from the ground before they reach the groundwater table. Consequently, the properties of soil and groundwater beneath desert vegetation are equally essential to understand the P nutrition status in desert transition regions.
In this study, the allocation pattern of the relatively fast-growing herb, K. capsica, and the relatively slow-growing shrub, T. ramosissima, and the impact of soil and groundwater properties on the foliar-P fractions allocation of these plants were assessed in four sites with different environment-P availability (soil Olsen-P and groundwater dissolved P concentration) in the desert-oasis transition zone on the southern edge of the Taklimakan Desert. The study investigated (1) variation of the leaf P and foliar-P fractions contents of K. caspica and T. ramosissima under different environment-P availability, (2) the allocation pattern of foliar-P fractions in K. caspica and T. ramosissima at low environment-P availability, and (3) the driving factors of soil and groundwater that influence the allocation of foliar-P fractions in the two desert plants.
Materials and Methods
Study Area Description
This study was conducted in the desert-oasis transition zone on the southern edge of the Taklimakan Desert in August 2020 (Figure 1A). In this region, the climate type is warm temperate desert climate, the groundwater depth ranges from 2 to 16 m, the average annual temperature is 15.85°C, the mean annual precipitation during the growing season, June to September is 42.62 mm (hyper-arid), and the maximum evaporation potential is roughly 2,700 mm (Chen et al., 2019). The soil is primarily aeolian sandy soil (Arenosols in the FAO/ISRIC/ISSS, 1998 taxonomy) with high pH and electrical conductivity (EC), low soil organic matter (SOM), soil water content (SWC), and available soil nutrients (Li et al., 2021; Gao et al., 2022). The natural vegetation on this desert-oasis transition zone is dominated by perennial phreatophytes. The perennial shrub, T. ramosissima, and herb, K. capsica, were selected as study plants for this analysis (Figures 1B,C).
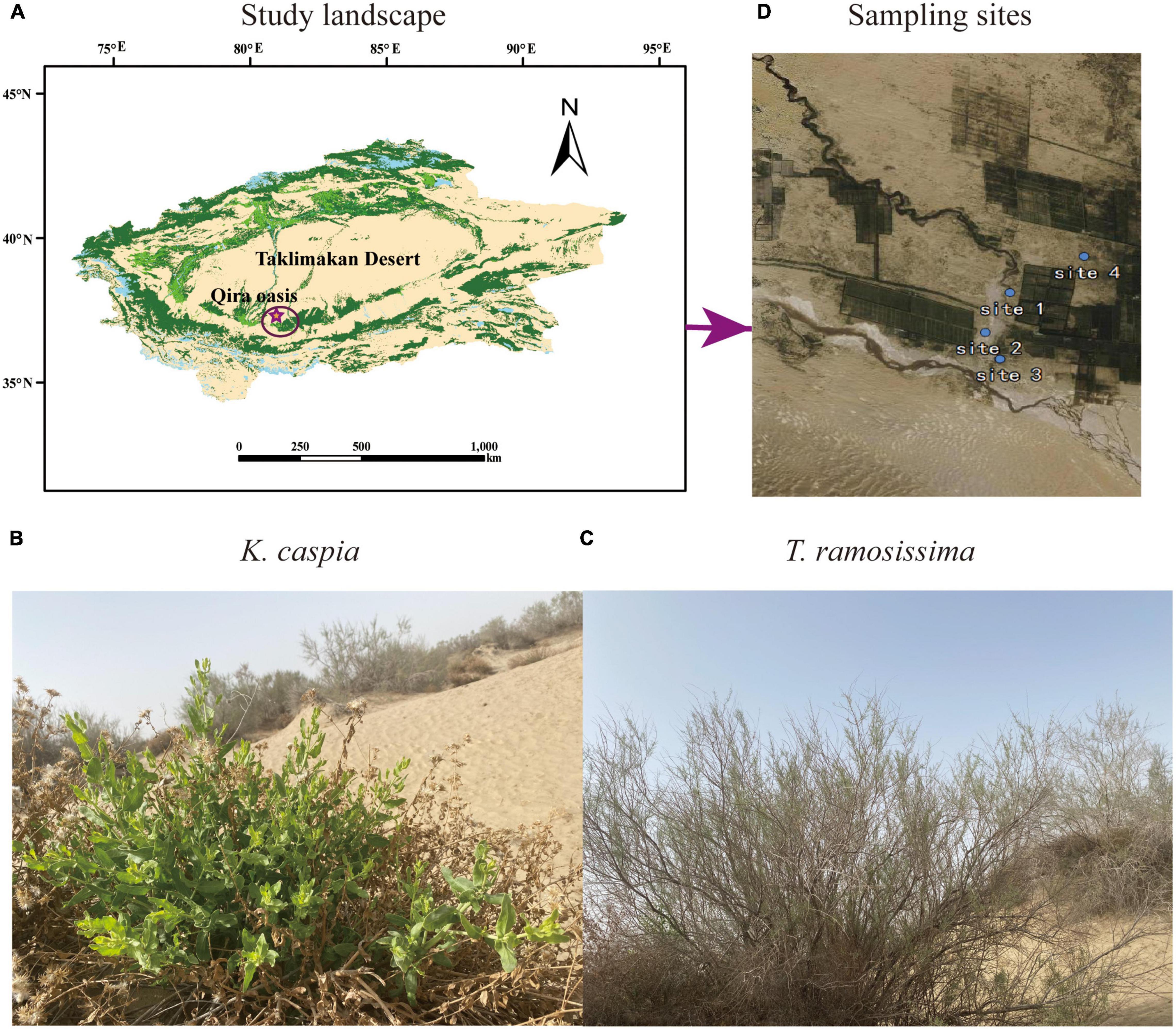
Figure 1. Photos of the study landscape (A), desert species Karelinia caspia (K. caspica) (B), Tamarix ramosissima (T. ramosissima) (C), and four sampling sites (D).
The four study sites had the following longitude, latitude, and groundwater depths: site 1 (N37°01′32′′ E80°42′27′′, 2.5 m), site 2 (N37°00′41′′ E80°42′15′′, 5 m), site 3 (N37°00′34′′ E80°42′28′′, 11 m), and site 4 (N37°00′56′′ E80°43′81′′, 13 m) (Figure 1D). Selection was based on the growth status of the two study plants in each site and the groundwater and soil properties at the depths of 0–60 cm. Soil Olsen-P and groundwater dissolved P concentrations are shown in Tables 1, 2. The distance between two sites was at least 2 km, and the plants selected for each site were at least 500 m far apart.
Plant Samples Collection
At each site, six T. ramosissima and K. caspica plants with consistent growth status were selected from which soil and plant samples were collected in August 2020, respectively. Approximately 3.0 g young and mature K. caspica leaves and 3.0 g mature T. ramosissima leaves with no visible damage or discoloration were collected in the natural conditions of each site to ensure enough for 1.0 g dry samples of each leaf type after freeze-drying. Plant samples were kept fresh by immediately placing them in resealable bags, putting them in a car-refrigerator and storing them at –80°C to determine different foliar-P fractions contents. Other leaf samples from the two desert plants were collected from each site to determine the contents of total leaf N and leaf P.
Soil Samples Collection
After plant sample collection, 0–60 cm deep soil samples were collected along the main root of each plant. The non-decomposed plant litter and sand on the soil surface were removed and a standard vertical soil profile of about 100 cm × 50 cm × 60 cm (length × width × height) was excavated from the center of the main root. The floating soil on the profile was gently swept away, a sample was collected within 2 cm of the main root at every 30 cm soil from bottom to top, and the two soil samples collected on each profile were mixed to obtain a 0–60 cm soil sample. All samples were stored in a car-refrigerator, taken back to the laboratory, and divided into three parts. One part was immediately assessed for soil water content (SWC), the second part was kept at 4°C to measure soil NH4+-N and NO3–-N, and the remaining parts were air-dried and used to measured soil Olsen-P, soil organic matter (SOM), EC and pH.
Groundwater Samples Collection
Groundwater samples were collected from a groundwater observation well at each site. These groundwater observation wells were excavated in 2005. A 1 L plastic bottle with a 2 kg weight at the bottom was extended into the observation well to obtain each groundwater sample and pH, EC, NH4+-N, NO3–-N, dissolved P, TN, total P (TP), and total dissolved solids (TDSs) were measured.
Plant Samples Analysis
Plant leaf P was divided into four fractions: metabolites-P (including inorganic P: Pi), nucleic acid-P, structural-P, and residual-P, and a modified sequential extraction was used for the fractionation procedure (Chapin and Kedrowski, 1983; Hidaka and Kitayama, 2011). In the measurement procedure, a higher weight of each leaf sample was used because of the lower foliar-P content of desert vegetation. Samples were freeze-dried (Alpha 2–4 LD Plus, Christ, Osterode am Harz, Germany), and 1.0 g was weighed into a 10 ml plastic centrifuge tube (tube 1) with 2 ml chloroform: methanol: formic acid (CMF = 12:6:1, v/v/v) and centrifuged for 15 min (5,000 rpm). The supernatant was transferred into another 10 ml plastic centrifuge tube (tube 2), the steps above were repeated, and then, 2.5 ml chloroform: methanol: water (CMW = 1:2:0.8, v/v/v) was added into the initial extraction and the steps above were repeated again. Then, 4 ml water-washed chloroform was added, the tube was centrifuged for 15 min (5,000 rpm), and the lower phase was transferred to 10 ml glass bottle (bottle 1) to determine the structural-P and the upper phase were transferred to another glass bottle (bottle 2). Furthermore, 5 mL methanol (85% v/v) was added into tube 1 and centrifuged for 15 min (5,000 rpm), the supernatant was transferred into bottle 2, and then, 2 ml 5% trichloroacetic acid (TCA) was added and the tube was centrifuged for 10 min (5,000 rpm). These steps were repeated again, the supernatant was transferred into bottle 2 to determine metabolites-P, and another 2 ml 2.5% TCA was added to tube 1. The tube was then placed in a water bath (95°C) for 60 min, cooled, and centrifuged for 10 min (5,000 rpm). These steps were repeated two times before all the supernatant was transferred into a 10 ml glass bottle (bottle 3) to determine nucleic acid-P. Another 5 ml 2.5% TCA was added to tube 1 and transferred to a 10 ml glass bottle (bottle 4) to measure the residual-P. In addition, HNO3:H2SO4 (3:1, v/v) was added into glass bottles 1, 2, 3, and 4 to digest the samples using the molybdenum blue method, and measurement was carried out with a full band spectrophotometer at 620 nm (Scheffer and Pajenkamp, 1952).
Other leaf samples were oven-dried at 75°C for a minimum of 48 h, and then ground and digested in a mixture of concentrated H2SO4 and H2O2 until the sample solution was clear. After cooling, the leaf N content was analyzed using an elemental analyzer (2400 II CHNS; Perkin-Elmer, Boston, MA, United States) and the leaf P content was measured using the method developed by Scheffer and Pajenkamp (1952).
Soil and Groundwater Samples Analysis
Soil Olsen-P (0.5M NaHCO3 as extractant), soil TP, groundwater dissolved P, and TP were measured according to the method developed by Olsen and Sommers (1982). Then, NH4+-N and NO3–-N concentrations were determined using the AA3 continuous flow analytical system (CFA) after extracting by 2 M KCl. Soil organic matter (SOM) was determined by the wet-oxidation technique (Shaw, 1959). The soil moisture content (SWC) was calculated according to the weight difference between wet soil and dry soil after drying at 105°C for 48 h. The pH and EC were measured at a soil/water ratio of 1:5 (w:v). TN concentration was measured with a full automatic Kjeldahl Nitrogen Analyzer (K1100, Jinan Hanon Instruments Co., Ltd., China). TDSs were gained by evaporating groundwater at 105–120°C.
Statistical Analysis
Analysis of variance (ANOVA) was performed using SPSS 20.0 software (SPSS Inc., United States). Soil properties, the mass ratios for leaf N to leaf P, and the amount of P in each foliar P-containing fraction were analyzed using one-way ANOVA and t-test. Groundwater properties and foliar-P fractions were analyzed using one-way ANOVA. Redundancy analysis was used to analyze the top five environmental factors with the closest correlations between foliar-P fractions and soil and groundwater properties at the four sites. Matrix and circle correlation heatmaps were used to analyze the pairwise and sum correlations among foliar P-fractions and soil and groundwater properties.
Results
Soil and Groundwater Properties
Soil Olsen-P, NH4+-N, and NO3–-N concentration increased gradually from site 1 to 4 and was higher in K. capsica than T. ramosissima samples (Table 1). Compared with T. ramosissima, the soil NH4+-N and NO3–-N concentration under K. caspica were increased by 12.4 and 10.4% at site 4, respectively. The higher nutrient concentration of the soil under K. caspica vs. T. ramosissima may be attributed to its lower soil pH and EC (Figure 2). SWC and pH were highest at site 1, but EC was the highest at site 4. SOM concentration under T. ramosissima decreased from site 1 to 4 and was significantly higher than K. caspica. Groundwater dissolved P, NO3–-N, TP, and TN increased from site 1 to 4, while pH, EC, and TDSs decreased (Table 2).
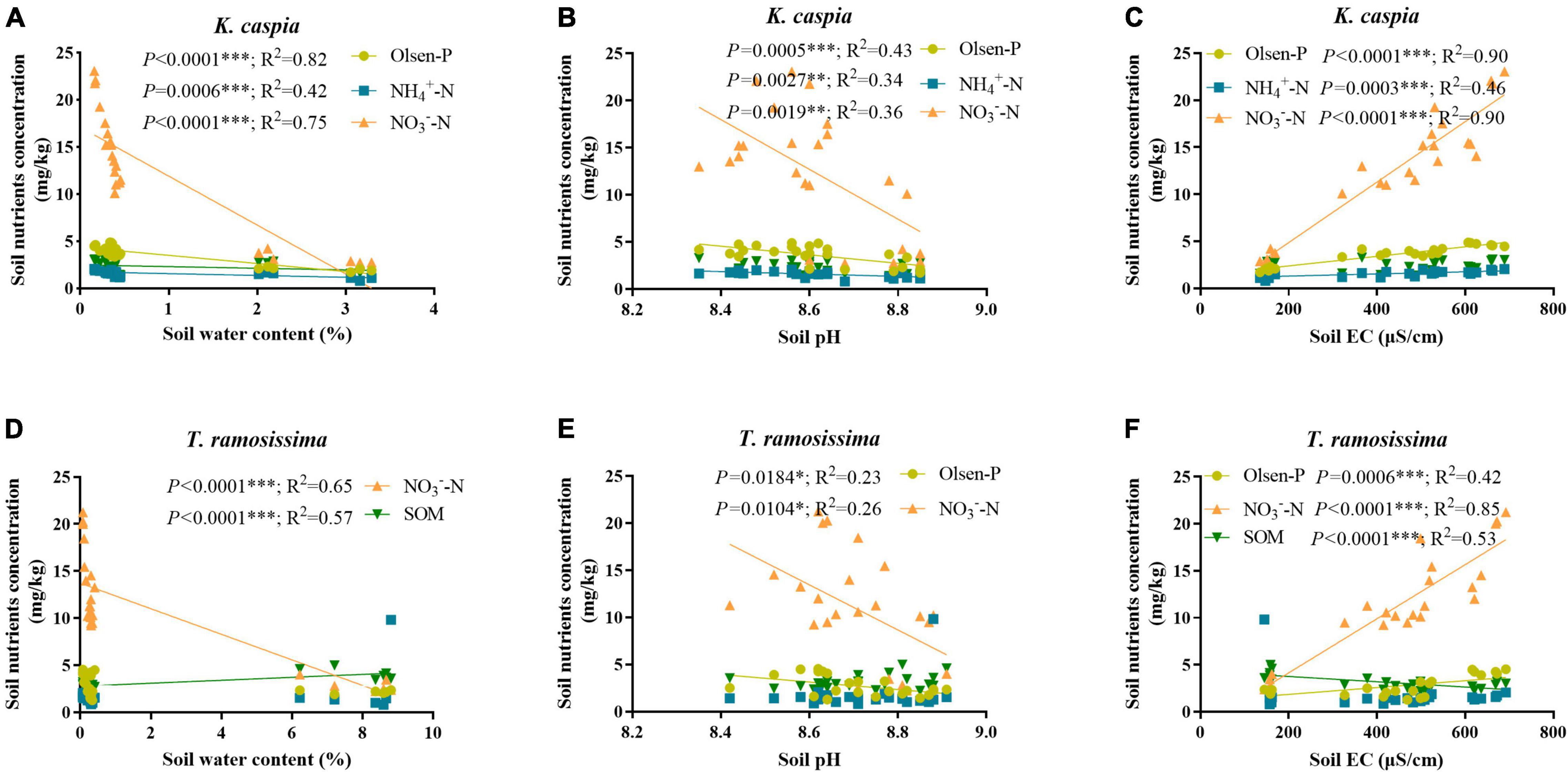
Figure 2. Correlations between the soil basic nutrients and soil water content, pH, and electrical conductivity (EC). The correlation between soil basic nutrients under K. caspia and soil water content (A), pH (B), and EC (C), and under T. ramosissima and soil water content (D), pH (E) and EC (F), respectively. The values of R2 and p are shown only if p < 0.05. *p < 0.05, **p < 0.01, ***p < 0.001.
Leaf N and P Content
Leaves of K. caspica and T. ramosissima had similar patterns of leaf N, P, and P in each foliar-P fraction across the four sites (Table 3). With the increasing soil N and P availability (Table 1), compared with the site 1, leaf N, and P of K. caspica in site 4 were increased by 31.3 and 18.2%, respectively, and leaf N and P of T. ramosissima were increased by 24.8 and 47.1%, respectively. The mean N content in K. caspica was decreased by 7.6% relative to T. ramosissima, while the mean P content increased by 44.5%. The leaf N:P ratio had a different pattern for the two species across the four sites. The ratio was lower in site 4 than site 1 and higher in T. ramosissima than K. caspica in every site, indicating that T. ramosissima growth was likely limited by P. The leaf N:PFraction ratios of K. caspica and T. ramosissima for nucleic acid-P were significantly lower in site 1 than site 4, while the ratios for metabolites-P and structural-P were indistinguishable. The ratios of leaf N:PFraction in each P fraction were higher in T. ramosissima than K. caspica at each site, except that residual-P contributed to the lower leaf N: P ratio.
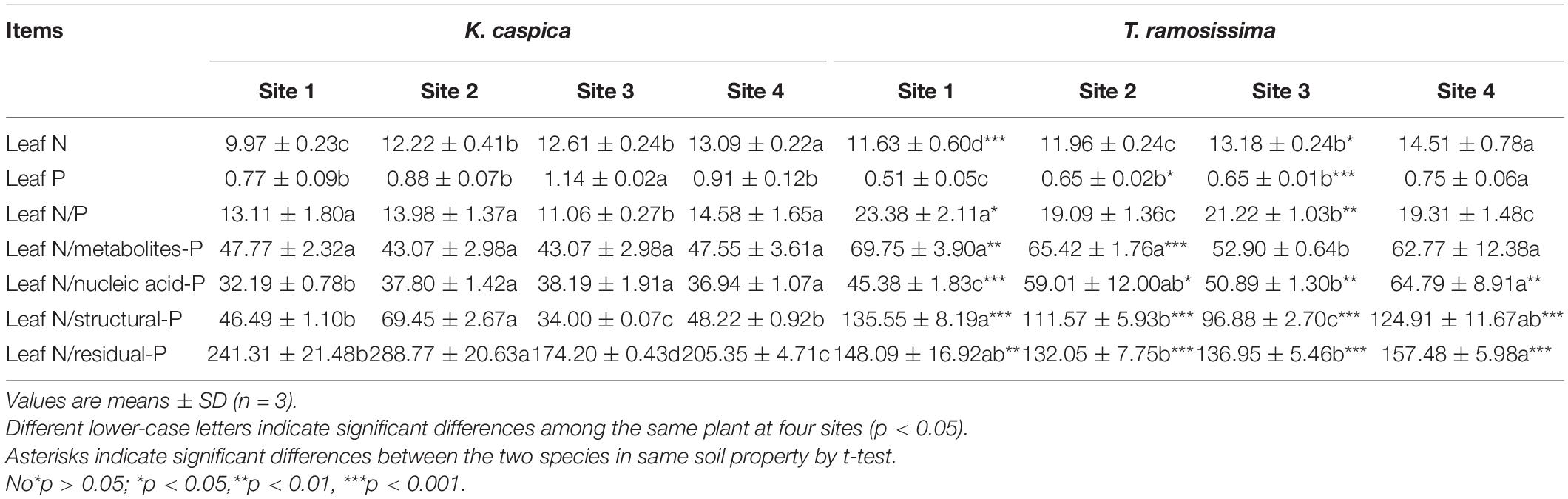
Table 3. Mass ratios for total leaf nitrogen (N) to total leaf phosphorus (P) and to P in each foliar P-containing fraction of Karelinia caspia (K. caspica) and Tamarix ramosissima (T. ramosissima).
Foliar-P Fractions Content
As shown in Figure 3, the foliar-P fractions content of K. caspica was distinctly higher than T. ramosissima, except for residual-P. Overall, the nucleic acid-P contents of K. caspica and T. ramosissima were greater than metabolites-P, structural-P, and residual-P, and the young leaves of K. caspica were higher than mature leaves and T. ramosissima. Meanwhile, the contents of metabolites-P, structural-P, and residual-P in K. caspica young leaves and T. ramosissima increased as raising the soil available-P concentration. Compared with site 1, the metabolites-P and structural-P contents of K. caspica young leaves increased by 50.8 and 34.6% in site 4, while nucleic acid-P reduced by 15.7%. Similarly, the mature leaves of T. ramosissima and young leaves of K. caspica tended to allocate higher foliar-P to nucleic acid-P than to metabolites-P and structural-P with decreasing soil available-P concentration.
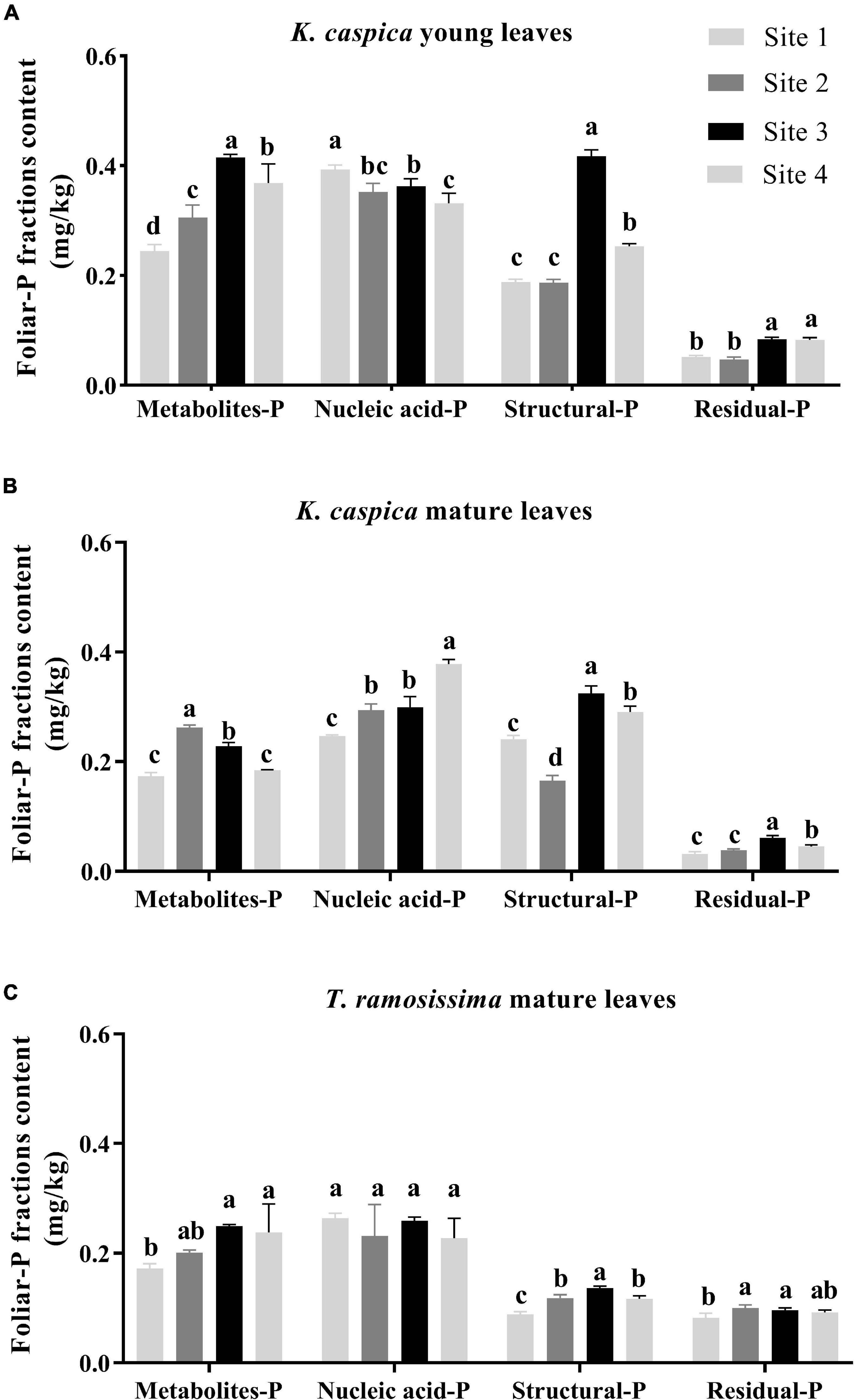
Figure 3. Foliar-P fractions content of two plant species at different environment-P sites. Foliar-P fractions contents of K. caspia young leaves (A), K. caspia mature leaves (B), and T. ramosissima mature leaves (C), respectively. Error bars represent means ± standard deviation (SD, n = 3). Different lower-case letters indicate significant differences of the same foliar-P fraction among the sites (p < 0.05).
Allocation Proportions of Foliar-P Fractions
The allocation proportion of nucleic acid-P in T. ramosissima mature leaf and K. caspica young leaves in site 1 increased by 16 and 18.6% compared with site 4, respectively (Figure 4). In comparison, metabolites-P and structural-P decreased by 33.7, 25.7, 27.8, and 24.2% in site 1. In contrast, the mature leaves of K. caspica reduced the allocation proportion of nucleic acid-P as soil available-P concentration decreased. Notably, the allocation proportion of residual-P and nucleic acid-P of T. ramosissima were markedly higher than K. caspica. The coefficient of variation (CV) of foliar-P fractions at the all sites shows that K. caspica young leaves > K. caspica mature leaves > T. ramosissima, structural-P > residual-P > metabolites-P > nucleic acid-P (Figure 5).
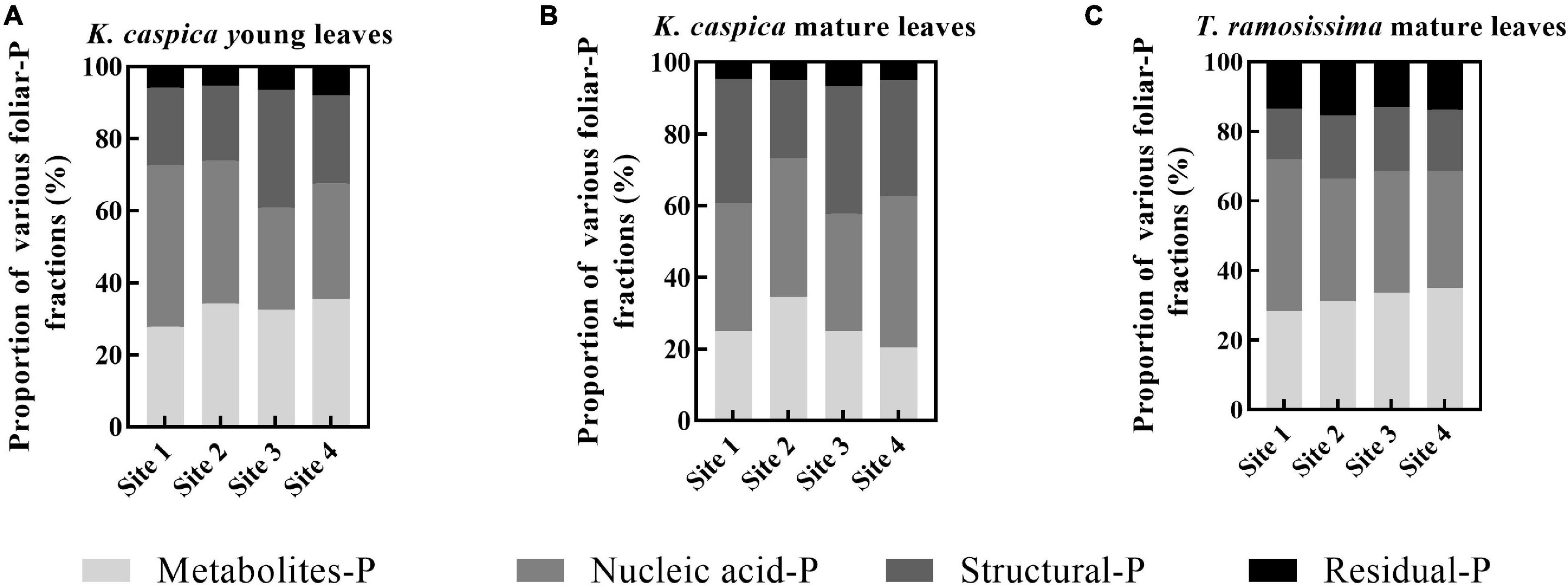
Figure 4. The allocation proportions of foliar-P fractions of two plant species at different environment-P sites. The allocation proportions of foliar-P fractions of K. caspia young leaves (A), K. caspia mature leaves (B), and T. ramosissima mature leaves (C), respectively.
Redundancy Analysis Between the Foliar-P Fractions and Environment Properties
Redundancy analysis was used to analyze the correlations between foliar-P fractions and soil and groundwater properties at four sites (Figure 6). Only the top five environment factors were displayed. The arrow angles between the metabolites-P and residual-P of K. caspica young leaves and soil NO3–-N, EC, and groundwater TN, between nucleic acid-P of K. caspica young leaves and soil pH, between nucleic acid-P of K. caspica mature leaves and soil NO3–-N, EC, groundwater TN, and pH were considerably smaller than 90 degrees, indicating positive relationships (Figures 6A,B). However, the metabolites-P and structural-P of T. ramosissima correlated positively with soil NO3–-N, EC, and groundwater TN, and nucleic acid-P correlated positively with soil pH (Figure 6C). In addition, for K. caspica young leaves, there were positive correlations between structural-P and site 3, nucleic acid-P and site 1, and metabolites-P and site 4, respectively (Figure 6A). However, for T. ramosissima mature leaves, there were strong correlations between structural-P and site 4, nucleic acid-P and site 1, and metabolites-P and site 3, respectively (Figure 6C).
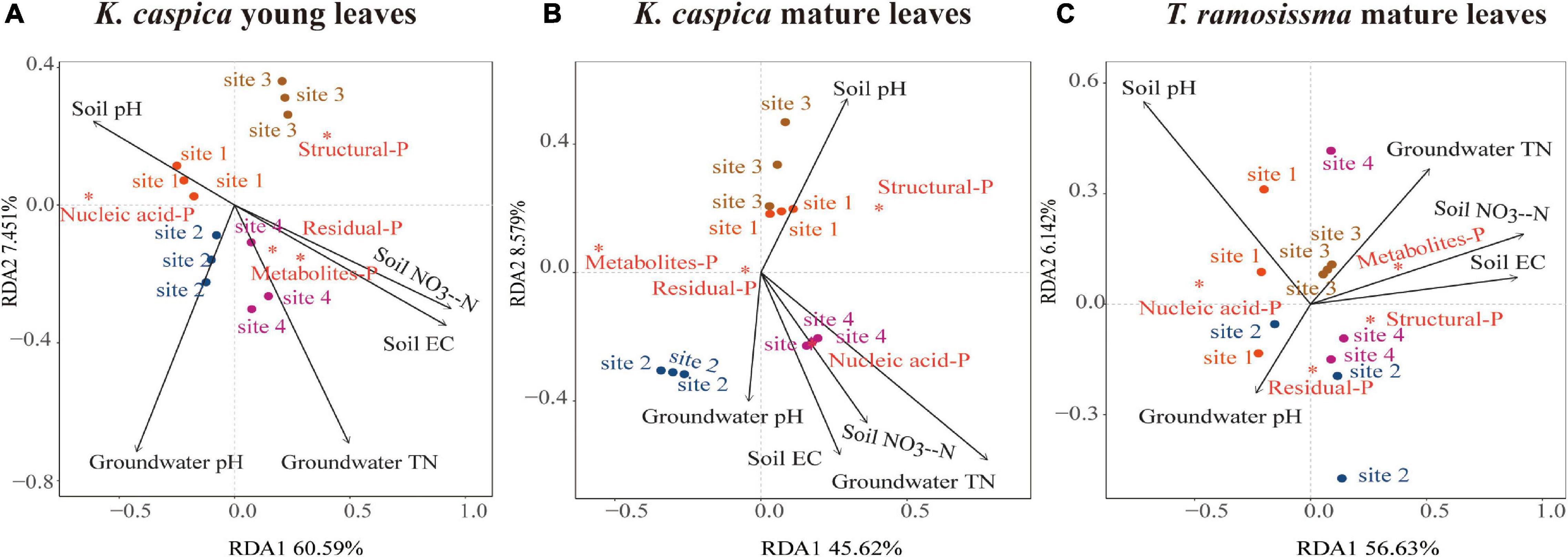
Figure 6. Redundancy analyses of the correlations between foliar-P fractions and soil and groundwater properties. Redundancy analysis on the correlations between soil and groundwater properties and foliar-P fractions of K. caspia young leaves (A), K. caspia mature leaves (B), and T. ramosissima mature leaves (C), respectively. Red asterisk means the foliar-P fractions; black arrow means the soil and groundwater properties. Only the top five environment factors are displayed.
Correlations Between the Foliar-P Fractions and Environment Properties
The sum correlations between foliar P-fractions and groundwater and soil properties were analyzed (Figure 7). There was a significant positive relationship between the metabolites-P of K. caspica young leaves and soil Olsen-P, NH4+-N, NO3–-N, and EC and a negative correlation between the metabolites-P of K. caspica young leaves and SWC, groundwater EC, and TDSs (Figure 7A). The pattern was flipped for the nucleic acid-P of K. caspica young leaves. However, a significant active correlation between the nucleic acid-P of K. caspica mature leaves and soil Olsen-P, NH4+-N, NO3–-N, EC, and groundwater NO3–-N and TN was found (Figure 7C). The metabolites-P and structural-P of T. ramosissima were positively correlated with soil Olsen-P, NH4+-N, NO3–-N, and EC, but negatively correlated with SWC, groundwater EC and TDSs (Figure 7E).
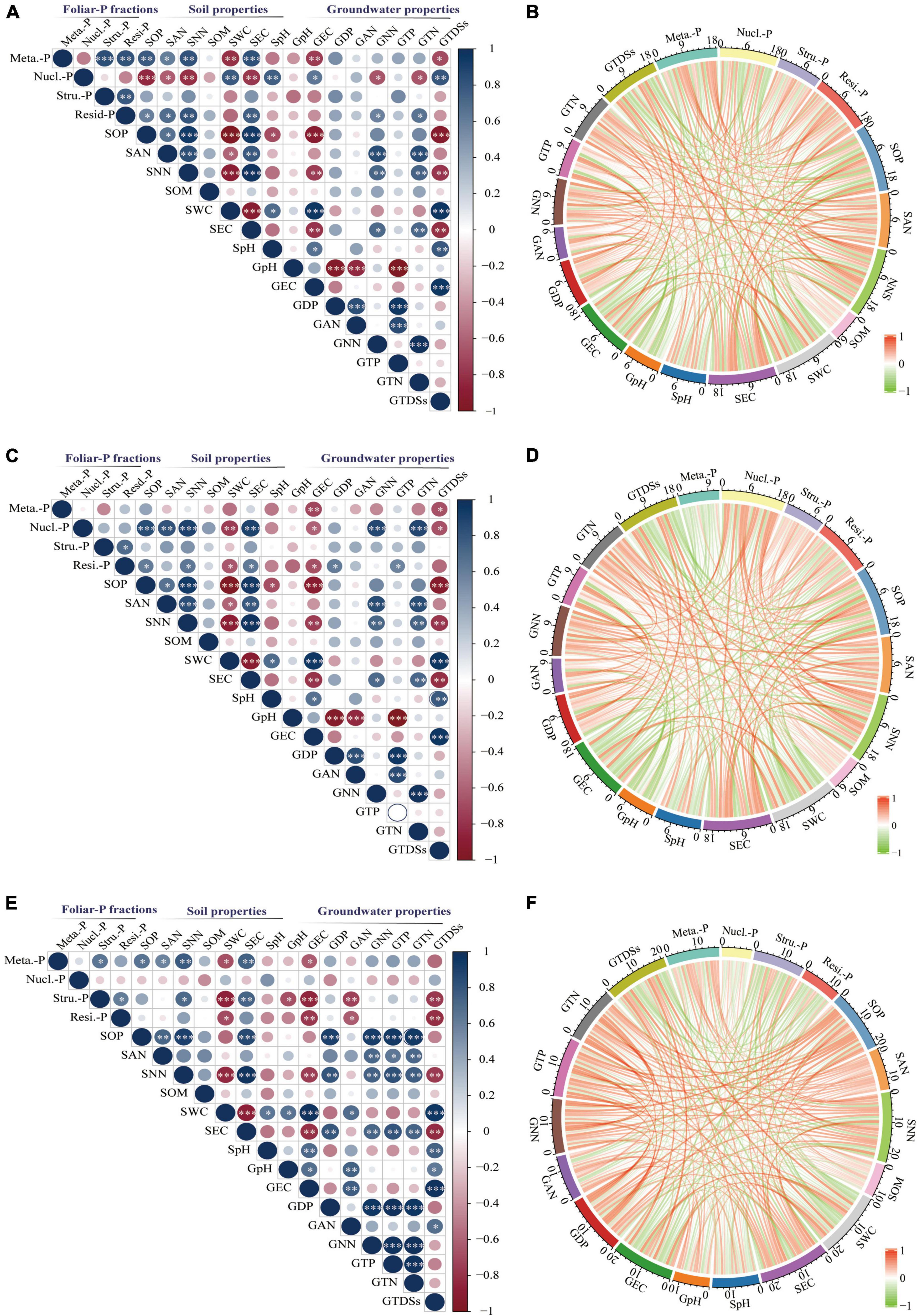
Figure 7. Correlations between foliar-P fractions and soil and groundwater properties. Correlations between soil and groundwater properties and foliar-P fractions of K. caspia young leaves (A,B), K. caspia mature leaves (C,D), T. ramosissima mature leaves (E,F), respectively. Stars represent the level of significance. No*p > 0.05; *p < 0.05, **p < 0.01, ***p < 0.001. The sum correlations coefficient between foliar P-fractions and environment factors are expressed by the scale on each arc. Meta.-P: Metabolites-P; Nucl.-P: Nucleic acid-P; Stru.-P: Structural-P; Resi.-P: Residual-P; S: soil; G: groundwater; OP: Olsen-P; DP: dissolved P; AN: NH4+-N; NN: NO3–-N; TP: total-P; TN: total-N; SOM: soil organic matter; SWC: soil water content; TDSs: total dissolved solids.
Of the four foliar-P fractions from the two desert species in this study, the overall relationship of metabolites-P, nucleic acid-P, and residual-P in K. caspica young leaves, nucleic acid-P, and residual-P in K. caspica mature leaves, and metabolites-P and structural-P in T. ramosissima were greater than those of other foliar-P fractions and were more strongly correlated with soil Olsen-P, NO3–-N, EC, SWC, and groundwater EC and TDSs (Figures 7B,D,F). Furthermore, the overall relationship of foliar-P fractions in K. caspica was greater than T. ramosissima, while the soil and groundwater properties of T. ramosissima were greater than K. caspica.
Discussion
Variations of Leaf N and P Content
Leaf N and P content are strongly related to leaf photosynthesis and carbon assimilation (Yan et al., 2015). In plant leaves, N and P often represent the best performance of plant adaptability to the environment (Drenovsky et al., 2010). In this study, the leaf P of both K. caspica and T. ramosissima decreased with decreasing environment-P availability (Table 3). Low environmental-P availability was found to limit P acquisition by plants explaining why they respond to low leaf P content. Indeed, it was previously reported that leaf P was lowest in the most P-impoverished site (Sulpice et al., 2014; Lambers et al., 2015). Additionally, leaf P of the two species in this study was markedly lower than the mean leaf P content in extensive global datasets due to the hyper-arid climate and P-impoverished soil (Wu et al., 2012; Gao et al., 2022). The leaf P content of K. caspica was higher than T. ramosissima grown in the same site, and K. caspica had higher structural-P content, implying that K. caspica may have a higher photosynthetic utilization rate (Mo et al., 2019).
Compared with the leaf P content in the two species, the leaf N content of T. ramosissima was higher than K. caspica. These findings differs from results on Hakea prostrata (Proteaceae) which showed that P availability does not affect leaf N content (Prodhan et al., 2019), but correlated leaf N and leaf P content was similar, and enhanced as increasing soil available-P concentration or groundwater available-P concentration (Han et al., 2021). The leaf N content of both species was lower than both those in global soils (Cleveland and Liptzin, 2007; Xu et al., 2013), and lower than results previously reported on A. sparsifolia in this area (Zhang et al., 2018; Gao et al., 2022). This is likely explained by the nutrient-impoverished soil in this region, the hyper-arid climate, and the higher soil pH and EC in sandy soil with poor water holding capacity, which reduces the availability, absorption, and transport of nutrients (Figure 2; Gong et al., 2017). In general, the remarkably low leaf N in K. caspica and T. ramosissima suggests that protein content was low, indicating a low demand for P to adapt to low P availability.
Herbaceous plants have higher leaf P than other functional groups explaining why herbs have low leaf N:P ratios (Yuan and Chen, 2009). This is consistent with results from this study where T. ramosissima growth was more likely limited by P while K. caspica growth was not. In addition, the higher leaf N content and leaf N:P ratios suggest that more leaf P was allocated to nucleic acid-P (Figure 4; Han et al., 2021). Interestingly, however, the ratios of leaf N:PFraction in each P fraction, with the exception of residual-P, were higher in T. ramosissima than in K. caspica (Table 3). This suggests that the lower contents of foliar-P fractions with specific functionality may be the main factors limiting P for T. ramosissima growing in this area.
Allocation Patterns of Foliar-P Fractions
Differences in the foliar-P fractions allocation patterns and soil and groundwater properties between the two species reflect distinctions in their nutrient utilization strategies and nutrient source. Aboveground parts of plants, such as herbaceous K. caspica die in the winter and resprout again in the spring (Vonlanthen et al., 2011) and have to grow fast to use limited soil, water, and nutrients quickly and efficiently (Verburg et al., 2013), and thus needs the higher P requirement and flexible allocation ability of foliar-P fractions (de Oliveira et al., 2018; Dissanayaka et al., 2018). Leaf P and the CV of foliar-P fractions of K. caspica were higher than T. ramosissima at four sites with different soil-P availability, which just confirmed this viewpoint (Figure 5). Indeed, the contents of foliar structural-P and nucleic acid-P, the balance of membrane lipid metabolism, and the synthesis of enzymes and proteins were higher in K. caspica than T. ramosissima, to maintain photosynthesis (Figure 3). This strategy may require greater nucleic acid-P investment to support rapid protein synthesis and turnover (Raven, 2012; Jeong et al., 2017). A high protein synthesis capacity may allow plants to acclimate to the variable and changing environments, such as P-impoverished soils or low groundwater dissolved P, and complete their life cycles quickly (Verburg et al., 2013; Mo et al., 2019; Gao et al., 2022). Unlike K. caspica, only the leaves of T. ramosissima withered and fallen in autumn, a strategy associated with a slower relative growth rate (RGR) (Bruelheide et al., 2010; Vonlanthen et al., 2011). Thus, T. ramosissima selection was based on a lower investment in nucleic acid P and the ability to allocate less biomass to deep roots, giving this plant a higher median life span than K. caspica (Li et al., 2010; Liu et al., 2016). It was surprising, however, that relatively slow-growing T. ramosissima did not have a significantly lower proportion of foliar nucleic acid-P allocation than relatively fast-growing K. caspica (Figure 4). The low leaf P content of T. ramosissima is most likely explained by extremely low environmental-P in the study region (Gao et al., 2022). To ensure its normal growth and development, T. ramosissima allocated more foliar-P proportion to nucleic acid-P to increase hydrolyses and protein synthesis. This is confirmed by the high leaf N: P ratios of T. ramosissima (Table 3).
The metabolites-P and structural-P content were significantly greater for K. caspica than T. ramosissima, suggesting that K. caspica has a higher photosynthetic rate and metabolic activity (Figure 3; Mo et al., 2019). Indeed, the content of structural-P is greater in herbs than in other functional groups, and this is attributed to the higher need of herbs to maintain a balance between membrane lipid metabolism and enzyme and protein synthesis (Jeong et al., 2017; Dissanayaka et al., 2018). This may be explained by the larger, fresher, and tenderer leaf characteristics of K. caspica (Figure 1B). However, T. ramosissima had a higher residual-P proportion of total P than K. caspica, and the proportion was lower when grown in the lower soil-P availability of site 1 than sites 2, 3, and 4 (Figure 4). The foliar residual-P differences between the two species may be attributed to a need for more metabolites-P and nucleic acid-P to participate in metabolic activities and the higher photosynthetic demand of fast-growing herbs than slow-growing shrubs (Mo et al., 2019; Gao et al., 2022). Although residual-P concentration is relatively constant in the same species, phosphatases may dephosphorylate phosphorylated proteins in extremely P-impoverished environments (Schlüter et al., 2013), explaining why the proportion of residual-P was lowest in the site with the least environmental-P (site 1).
Effect of Different Soil and Groundwater Properties on Foliar-P Fractions Allocation Patterns
Nucleic acid-P was negatively correlated with soil-P availability and groundwater dissolved P concentration in our study, while metabolites-P was positively correlated (Figures 6, 7). This suggests that low environment-P allows plants to distribute more foliar-P fractions to nucleic acid-P to promote hydrolase and protein synthesis, and then provides more P to metabolites-P fraction (Raven, 2012; Jeong et al., 2017; Dissanayaka et al., 2018).
Foliar-P fractions’ allocation patterns in the two desert plants were tightly related to soil Olsen-P, NO3–-N, SWC, EC, and groundwater EC and TDSs in this area (Figures 6, 7). This indicated that soil and groundwater properties were both crucial drivers of foliar-P fractions allocation by desert plants in a hyper-arid desert ecosystem. This is corroborated with the earlier findings as reported by Gao et al. (2022). However, compared with K. caspica, the foliar-P fractions of T. ramosissima were more closely related to groundwater than soil properties (Figure 7). This may be explained by the root characteristics of shrubs and herbs. Liu et al. (2016) monitored the fine roots of some perennial herbs, shrubs, and tree species in the Taklimakan desert and showed that K. caspica had a primary anatomical structure and higher N content, greater respiration rate, and a shorter life span than T. ramosissima. The fine roots of T. ramosissima showed thicker diameters, no cortex or a collapsed cortex, and distinct secondary growth. These findings suggest that K. caspica can absorb more nutrients and water from the soil than T. ramosissima. Since fine root structure generally determines nutrient and water-absorbing ability (McCormack et al., 2012, 2013, 2015), the stronger vitality of fine roots is conducive to nutrient and water absorption (Liu et al., 2016).
Prior studies found that K. caspica has a deeper root than T. ramosissima, as well as clonal reproduction (Li et al., 2010; Vonlanthen et al., 2011), indicating that K. caspica receives more nutrients and water from both soil and groundwater. Similarly, the results presented here showed that while foliar-P fractions allocation of K. caspica was influenced by many soil and groundwater properties, the foliar-P fractions allocation of T. ramosissima was more closely related to groundwater properties. Overall, the two species have a similar pattern of foliar-P fractions allocation but their responses to soil and groundwater properties are completely different. These differences may be associated with growth strategies designed for the particular ecological niches in which they are found (Figure 8).
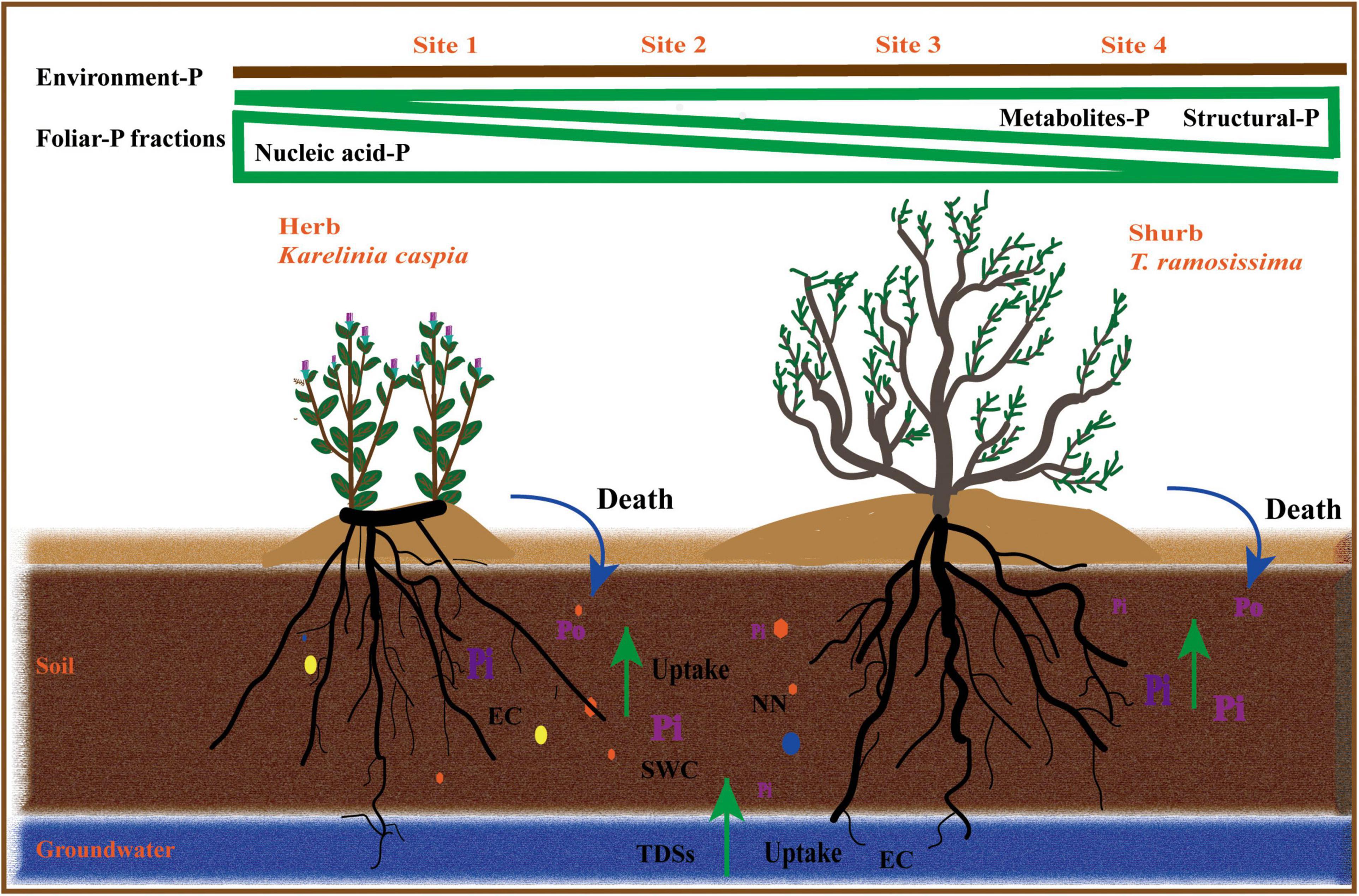
Figure 8. Diagrams summarizing foliar-P fractions allocation of two plant species at different environment-P levels.
Conclusion
This study provides strong evidence that not all desert vegetation growing in P-impoverished soils are limited by environment-P; T. ramosissima was limited, while K. caspica was not. This may be because K. caspica had higher soil-P levels than T. ramosissima. The high content and allocation proportion of nucleic acid-P and the low content and allocation proportions of metabolites-P and structural-P suggested that the allocation patterns of foliar-P fractions by the herb and shrub were similar in the P-impoverished study sites. Groundwater properties were another driver of foliar-P fractions allocation in this study. These results supply an essential theoretical basis for understanding the differences in foliar-P fractions allocation between fast-growing desert herbs and slow-growing desert shrubs in regions with different environmental-P availability. However, understanding of why residual-P was significantly higher in the shrub than the herb, what role residual-P plays in foliar-P fractions allocation, and the correlation between shrub and groundwater nutrients requires further exploration.
Data Availability Statement
The original contributions presented in the study are included in the article/supplementary material, further inquiries can be directed to the corresponding author/s.
Author Contributions
YG and FZ planned and designed the research. YG, MX, and XC conducted the fieldwork. YG, ZZ, HY, and BZ analyzed the data. AT and FZ revised the manuscript. All authors contributed to the article and approved the submitted version.
Funding
This work was supported by the Program of Joint Funds of the National Natural Science Foundation of China and Xinjiang Uygur Autonomous Region of China (No. U1903102) and the National Natural Science Foundation of China (No. 41977050).
Conflict of Interest
The authors declare that the research was conducted in the absence of any commercial or financial relationships that could be construed as a potential conflict of interest.
Publisher’s Note
All claims expressed in this article are solely those of the authors and do not necessarily represent those of their affiliated organizations, or those of the publisher, the editors and the reviewers. Any product that may be evaluated in this article, or claim that may be made by its manufacturer, is not guaranteed or endorsed by the publisher.
Acknowledgments
We would like to thank Yue Zhao, Jianian Wang, Jaoyang Song, Yanying Qi, and the concerned staff of Cele National station for assistance with field work.
References
Bruelheide, H., Vonlanthen, B., Jandt, U., Thomas, F. M., Foetzki, A., Gries, D., et al. (2010). Life on the edge-to which degree does phreatic water sustain vegetation in the periphery of the Taklamakan Desert? Appl. Veg. Sci. 13, 56–71. doi: 10.1111/j.1654-109X.2009.01050.x
Chapin, F. S., and Kedrowski, R. A. (1983). Seasonal changes in nitrogen and phosphorus fractions and autumn retranslocation in evergreen and deciduous taiga trees. Ecology 64, 376–391. doi: 10.2307/1937083
Chen, X. P., Qi, Z. M., Gui, D. W., Gu, Z., Ma, L. W., Zeng, F. J., et al. (2019). Simulating impacts of climate change on cotton yield and water requirement using RZWQM2. Agric. Water Manage. 222, 231–241. doi: 10.1016/j.agwat.2019.05.030
Cleveland, C. C., and Liptzin, D. (2007). C:N:P stoichiometry in soil: is there a “Redfield ratio”for the microbial biomass? Biogeochemistry 85, 235–252. doi: 10.1007/s10533-007-9132-0
de Oliveira, L. B., Marques, A. C. R., de Quadros, F. L. F., Farias, J. G., Piccin, R., Brunetto, G., et al. (2018). Phosphorus allocation and phosphatase activity in grasses with different growth rates. Oecologia 186, 633–643. doi: 10.1007/s00442-018-4059-9
Dissanayaka, D., Plaxton, W. C., Lambers, H., Siebers, M., Marambe, B., and Wasaki, J. (2018). Molecular mechanisms underpinning phosphorus-use efficiency in rice. Plant Cell Environ. 41, 1483–1496. doi: 10.1111/pce.13191
Dobrota, C. (2004). “The biology of phosphorus,” in Phosphorus in Environmental Technology: Principles and Applications, first Edn, ed. E. Valsami-Jones (London: IWA publishing), 51–78.
Drenovsky, R. E., James, J. J., and Richards, J. H. (2010). Variation in nutrient resorption by desert shrubs. J. Arid Environ. 74, 1564–1568. doi: 10.1016/j.jaridenv.2010.05.030
Elser, J. J., and Hamilton, A. (2007). Stoichiometry and the new biology: the future is now. PLoS Biol. 5:e181. doi: 10.1371/journal.pbio.0050181
Epstein, E., and Bloom, A. J. (2005). Mineral Nutrition of Plants: Principles and Perspectives, 2nd Edn. Sunderland, MA: Sinauer Associates.
FAO/ISRIC/ISSS (1998). World Reference Base for Soil Resources (WRB). In: World Soil Resources. 84. Rome: FAO.
Gao, Y. J., Tariq, A., Zeng, F. J., Graciano, C., Zhang, Z. H., Sardans, J., et al. (2022). Allocation of foliar-P fractions of Alhagi sparsifolia and its relationship with soil-P fractions and soil properties in a hyperarid desert ecosystem. Geoderma 407:115546. doi: 10.1016/j.geoderma.2021.115546
Gong, Y. M., Lv, G. H., Guo, Z. J., Chen, Y., and Cao, J. (2017). Influence of aridity and salinity on plant nutrients scales up from species to community level in a desert ecosystem. Sci. Rep. 7:6811. doi: 10.1038/s41598-017-07240-6
Gries, D., Zeng, F. J., Foetzki, A., Arndt, S. K., Bruelheide, H., Thomas, F. M., et al. (2003). Growth and water relations of T. ramosissima and Populus euphratica on Taklamakan desert dunes in relation to depth to a permanent water table. Plant Cell Environ. 26, 725–736. doi: 10.1046/j.1365-3040.2003.01009.x
Han, Z. M., Shi, J. M., Pang, J. Y., Yan, L., Finnegan, P. M., and Lambers, H. (2021). Foliar nutrient-allocation patterns in Banksia attenuata and Banksia sessilis differing in growth rate and adaptation to low-phosphorus habitats. Ann. Bot. 128, 419–430. doi: 10.1093/aob/mcab013
Hidaka, A., and Kitayama, K. (2011). Allocation of foliar phosphorus fractions and leaf traits of tropical tree species in response to decreased soil phosphorus availability on Mount Kinabalu, Borneo. J. Ecol. 99, 849–857. doi: 10.1111/j.1365-2745.2011.01805.x
Hou, E. Q., Luo, Y. Q., Kuang, Y. W., Chen, C. G., Lu, X. K., Jiang, L. F., et al. (2020). Global meta-analysis shows pervasive phosphorus limitation of aboveground plant production in natural terrestrial ecosystems. Nat. Commun. 11:637. doi: 10.1038/s41467-020-14492-w
Innes, L., Hobbs, P. J., and Bardgett, R. D. (2004). The impacts of individual plant species on rhizosphere microbial communities in soils of different fertility. Biol. Fertil. Soils 40, 7–13. doi: 10.1007/s00374-004-0748-0
Jeong, K., Julia, C. C., Waters, D. L. E., Pantoja, O., Wissuwa, M., Heuer, S., et al. (2017). Remobilisation of phosphorus fractions in rice flag leaves during grain filling: implications for photosynthesis and grain yields. PLoS One 12:e0187521. doi: 10.1371/journal.pone.0187521
Lambers, H., Finnegan, P. M., Jost, R., Plaxton, W. C., Shane, M. W., and Stitt, M. (2015). Phosphorus nutrition in Proteaceae and beyond. Nat. Plants 1, 1–9. doi: 10.1038/nplants.2015.109
Li, J., Zhao, C., Zhu, H., Li, Y., and Wang, F. (2007). Effect of plant species on shrub fertile island at an oasis-desert ecotone in the South Junggar Basin, China. J. Arid Environ. 71, 350–361. doi: 10.1016/j.jaridenv.2007.03.015
Li, M. M., Petrie, M. D., Tariq, A., and Zeng, F. J. (2021). Response of nodulation, nitrogen fixation to salt stress in a desert legume Alhagi sparsifolia. Environ. Exp. Bot. 183:104348. doi: 10.1016/j.envexpbot.2020.104348
Li, X. Y., Lin, L. S., Zhao, Q., Zhang, X. M., and Thomas, F. M. (2010). Influence of groundwater depth on species composition and community structure in the transition zone of Cele oasis. J. Arid Land 2, 235–242.
Liu, B., He, J. X., Zeng, F. J., Lei, J. Q., and Arndt, S. K. (2016). Life span and structure of ephemeral root modules of different functional groups from a desert system. New Phytol. 211, 103–112. doi: 10.1111/nph.13880
McCormack, M. L., Adams, T. S., Smithwick, E. A. H., and Eissenstat, D. M. (2012). Predicting fine root lifespan from plant functional traits in temperate trees. New Phytol. 195, 823–831. doi: 10.1111/j.1469-8137.2012.04198.x
McCormack, M. L., Dickie, I. A., Eissenstat, D. M., Fahey, T. J., Fernandez, C. W., Guo, D. L., et al. (2015). Redefining fine roots improves understanding of below-ground contributions to terrestrial biosphere processes. New Phytol. 207, 505–518. doi: 10.1111/nph.13363
McCormack, M. L., Eissenstat, D. M., Prasad, A. M., and Smithwick, E. A. H. (2013). Regional scale patterns of fine root lifespan and turnover under current and future climate. Glob. Change Biol. 19, 1697–1708.
Meglioli, P. A., Aranibar, J. N., Villagra, P. E., and Vega, R. C. (2017). Spatial patterns of soil resources under different land use in Prosopis woodlands of the Monte desert. Catena 149, 86–97. doi: 10.1111/gcb.12163
Mo, Q. F., Li, Z. A., Sayer, E. J., Lambers, H., Li, Y. W., Zou, B., et al. (2019). Foliar phosphorus fractions reveal how tropical plants maintain photosynthetic rates despite low soil phosphorus availability. Funct. Ecol. 33, 503–513. doi: 10.1111/1365-2435.13252
Olsen, R. S., and Sommers, L. E. (1982). “Phosphorus,” in Methods of Soil Analysis (Part 2), eds A. L. Page, R. H. Miller, and D. R. Keeney (Madison, WI: American Society of Agronomy), 15–72.
Prodhan, M. A., Finnegan, P. M., and Lambers, H. (2019). How does evolution in phosphorus-impoverished landscapes impact plant nitrogen and sulfur assimilation? Trends Plant Sci. 24, 69–82.
Raven, J. A. (2012). Protein turnover and plant RNA and phosphorus requirements in relation to nitrogen fixation. Plant Sci. 188–189, 25–35. doi: 10.1016/j.plantsci.2012.02.010
Reef, R., Ball, M. C., Feller, I. C., and Lovelock, C. E. (2010). Relationships among RNA: DNA ratio, growth and elemental stoichiometry in mangrove trees. Funct. Ecol. 24, 1064–1072. doi: 10.1111/j.1365-2435.2010.01722.x
Scheffer, F., and Pajenkamp, H. (1952). Phosphatbestimmung in P flanzenaschen nach der Molybdän Vanadin Methode. J. Plant Nutr. Soil Sci. 56, 2–8. doi: 10.1002/jpln.19520560104
Schlüter, U., Colmsee, C., Scholz, U., Brautigam, A., Weber, A. P., Zellerhoff, N., et al. (2013). Adaptation of maize source leaf metabolism to stress related disturbances in carbon, nitrogen and phosphorus balance. BMC Genomics 14:442. doi: 10.1186/1471-2164-14-442
Shaw, K. (1959). Determination of organic carbon in soil and plant material. Eur. J. Soil Sci. 10, 316–326. doi: 10.1111/j.1365-2389.1959.tb02353.x
Sulpice, R., Ishihara, H., Schlereth, A., Cawthray, G. R., Encke, B., Giavalisco, P., et al. (2014). Low levels of ribosomal RNA partly account for the very high photosynthetic phosphorususe efficiency of Proteaceae species. Plant Cell Environ. 37, 1276–1298. doi: 10.1111/pce.12240
Sun, Y. F., Zhang, Y. Q., Feng, W., Qin, S. G., Liu, Z., Bai, Y. X., et al. (2017). Effects of xeric shrubs on soil microbial communities in a desert in northern China. Plant Soil 414, 281–294. doi: 10.1007/s11104-016-3111-y
Thomas, F. M., Foetzkia, A., Arndt, S. K., Bruelheidea, H., Griesa, D., Li, X. Y., et al. (2006). Water use by perennial plants in the transition zone between river oasis and desert in NW China. Basic Appl. Ecol. 7, 253–267. doi: 10.1016/j.baae.2005.07.008
Thompson, T. L., Zaady, E., Huan, C. P., Wilson, T. B., and Martens, D. A. (2006). Soil C and N pools in patchy shrublands of the Negev and Chihuahuan Deserts. Soil Biol. Biochem. 38, 1943–1955. doi: 10.1016/j.soilbio.2006.01.002
Throop, H. L., and Archer, S. R. (2008). Shrub (Prosopis velutina) encroachment in a semidesert grassland: spatial-temporal changes in soil organic carbon and nitrogen pools. Glob. Chang. Biol. 14, 2420–2431. doi: 10.1111/j.1365-2486.2008.01650.x
Titus, J. H., Nowak, R. S., and Smith, S. D. (2002). Soil resource heterogeneity in the Mojave Desert. J. Arid Environ. 52, 269–292. doi: 10.1006/jare.2002.1010
Veneklaas, E. J., Lambers, H., Bragg, J., Finnegan, P. M., Lovelock, C. E., Plaxton, W. C., et al. (2012). Opportunities for improving phosphorus-use efficiency in crop plants. New Phytol. 195, 306–320. doi: 10.1111/j.1469-8137.2012.04190.x
Verburg, P. S. J., Young, A. C., Stevenson, B. A., Glanzmann, I., Arnone, J. A. III, Marion, G. M., et al. (2013). Do increased summer precipitation and N deposition alter fine root dynamics in a Mojave Desert ecosystem? Glob. Change Biol. 19, 948–956. doi: 10.1111/gcb.12082
Vonlanthen, B., Zhang, X. M., and Bruelheide, H. (2011). Establishment and early survival of five phreatophytes of the Taklamakan Desert. Flora 206, 100–106. doi: 10.1016/j.flora.2010.01.013
Wu, T. G., Yu, M. K., Wang, G. G., Dong, Y., and Cheng, X. R. (2012). Leaf nitrogen and phosphorus stoichiometry across forty-two woody species in Southeast China. Biochem. Syst. Ecol. 44, 255–263. doi: 10.1016/j.bse.2012.06.002
Xia, Z. C., He, Y., Yu, L., Lv, R. B., Helena, K., and Li, C. Y. (2020). Sex-specific strategies of phosphorus (P) acquisition in Populus cathayana as affected by soil P availability and distribution. New Phytol. 225, 782–792.
Xu, X. F., Thornton, P. E., and Post, W. M. (2013). A global analysis of soil microbial biomass carbon, nitrogen and phosphorus in terrestrial ecosystems. Glob. Ecol. Biogeogr. 22, 737–749. doi: 10.1111/geb.12029
Xue, J., Gui, D. W., Lei, J. Q., Sun, H. W., Zeng, F. J., Mao, D. L., et al. (2019). Oasification: an unable evasive process in fighting against desertification for the sustainable development of arid and semiarid regions of China. Catena 179, 197–209. doi: 10.1016/j.catena.2019.03.029
Yan, K., Duan, C., Fu, D., Li, J., Wong, M. H., Qian, L., et al. (2015). Leaf nitrogen and phosphorus stoichiometry of plant communities in geochemically phosphorus-enriched soils in a subtropical mountainous region, SW China. Environ. Earth Sci. 74, 3867–3876. doi: 10.1007/s12665-015-4519-z
Yan, L., Zhang, X. H., Han, Z. G., Pang, J. Y., Lambers, H., and Finnegan, P. M. (2019). Responses of foliar phosphorus fractions to soil age are diverse along a two-million-year dune chronosequence. New Phytol. 223, 1621–1633. doi: 10.1111/nph.15910
Yao, Y., Shao, M., Fu, X., Wang, X., and Wei, X. (2019). Effects of shrubs on soil nutrients and enzymatic activities over a 0-100 cm soil profile in the desert-loess transition zone. Catena 174, 362–370. doi: 10.1016/j.catena.2018.11.031
Yuan, Z., and Chen, H. Y. H. (2009). Global trends in senesced-leaf nitrogen and phosphorus. Glob.Ecol. Biogeogr. 18, 532–542. doi: 10.1111/j.1466-8238.2009.00474.x
Zeng, F. J., Song, C., Guo, H. F., Liu, B., Luo, W. C., Gui, D. W., et al. (2013). Responses of root growth of Alhagi sparsifolia Shap. (Fabaceae) to different simulated groundwater depths in the southern fringe of the Taklimakan Desert, China. J. Arid Land 5, 220–232. doi: 10.1007/s40333-013-0154-2
Zhang, B., Gao, X. P., Li, L., Lu, Y., Muhammad, S., Huang, C. B., et al. (2018). Groundwater depth affects phosphorus but not carbon and nitrogen concentrations of a desert phreatophyte in Northwest China. Front. Plant Sci. 9:338. doi: 10.3389/fpls.2018.00338
Zhang, L., Li, G. J., Dong, G. Q., Wang, M., Di, D. W., Kronzucker, H. J., et al. (2019). Characterization and comparison of nitrate fluxes in T. ramosissima and cotton roots under simulated drought conditions. Tree Physiol. 39, 628–640. doi: 10.1093/treephys/tpy126
Keywords: desert ecosystem, phreatophyte, foliar-P fraction, groundwater, P limitation, soil properties
Citation: Gao Y, Zhang Z, Zhang B, Yin H, Chai X, Xu M, Tariq A and Zeng F (2022) Foliar P-Fractions Allocation of Karelinia caspia and Tamarix ramosissima Are Driven by Soil and Groundwater Properties in a Hyper-Arid Desert Ecosystem. Front. Plant Sci. 13:833869. doi: 10.3389/fpls.2022.833869
Received: 12 December 2021; Accepted: 18 February 2022;
Published: 31 March 2022.
Edited by:
Victoria Fernandez, Polytechnic University of Madrid, SpainReviewed by:
Sehrish Sadia, University of Veterinary and Animal Sciences, PakistanNirmalendu Basak, Central Soil Salinity Research Institute (ICAR), India
Copyright © 2022 Gao, Zhang, Zhang, Yin, Chai, Xu, Tariq and Zeng. This is an open-access article distributed under the terms of the Creative Commons Attribution License (CC BY). The use, distribution or reproduction in other forums is permitted, provided the original author(s) and the copyright owner(s) are credited and that the original publication in this journal is cited, in accordance with accepted academic practice. No use, distribution or reproduction is permitted which does not comply with these terms.
*Correspondence: Akash Tariq, YWthc2gubWFsaWs3ODZAbWFpbHMudWNhcy5hYy5jbg==; Fanjiang Zeng, emVuZ2ZqQG1zLnhqYi5hYy5jbg==