- Department of Biological Sciences, University of Wisconsin-Milwaukee, Milwaukee, WI, United States
In flowering plants, male reproductive development is highly susceptible to heat stress. In this mini-review, we summarized different anomalies in tapetum, microspores, and pollen grains during anther development under heat stress. We then discussed how epigenetic control, particularly DNA methylation, is employed to cope with heat stress in male reproduction. Further understanding of epigenetic mechanisms by which plants manage heat stress during male reproduction will provide new genetic engineering and molecular breeding tools for generating heat-resistant crops.
Introduction
Short- and long-term heat stress have detrimental effects on overall growth and development in plants (Kotak et al., 2007); however, reproductive organs, particularly the male reproductive organ, are more susceptible to elevated temperatures comparing with vegetative organs (Abiko et al., 2005; Sakata et al., 2010; Sato et al., 2014, 2019; Fragkostefanakis et al., 2016; Begcy et al., 2019; He et al., 2019). Heat stress leads to partial or complete male sterility, which in turn causes yield loss in crops (Smith and Zhao, 2016). Being sessile, plants employ various mechanisms to cope with heat stress. Besides the genetic control, transcriptome and genome-wide DNA methylation analyses have revealed that the epigenetic regulation plays a pivotal role in reprogramming expression of genes required for plants to manage heat stress during reproductive development. In this mini-review, we focus on discussing research in epigenetic mechanisms underlying heat stress response in male reproduction.
Plant Male Reproduction Is Highly Sensitive to Heat Stress
Heat stress impairs anther wall cell differentiation, microsporogenesis, and pollen formation, resulting in partial or complete male sterility in various plants. Stamen is the male reproductive organ of flowering plants, comprising of an anther where pollen (the male gametophyte) develops and a filament that anchors the anther to the flower. A typical anther has four lobes (microsporangia; Goldberg et al., 1993; Zhao, 2009; Feng et al., 2013; Walbot and Egger, 2016); within each lobe, the central pollen mother cells (PMC or microsporocytes) are surrounded by four concentrically organized layers of somatic cells: the epidermis, endothecium, middle layer, and tapetum (outside to inside). PMCs give rise to pollen via a series of events. PMCs undergo meiosis to produce tetrads that release microspores. After two rounds of mitosis, microspores eventually become pollen grains which contain a vegetative cell and two sperm cells (Sanders et al., 1999; Figure 1). The somatic anther wall cells, particularly tapetal cells (tapetum), are essential for the normal development and release of pollen. Tapetum, consisting of a monolayer or multilayers of endopolyploid cells, which is associated with successive stages of PMC, tetrads, microspores, and developing pollen as anther development progresses (Goldberg et al., 1993; Scott et al., 2004; Walbot and Egger, 2016; Figure 1). Early on, tapetal cells secrete enzymes required for releasing haploid microspores from tetrads (Pacini et al., 1985; Clément and Pacini, 2001; Hsieh and Huang, 2007; Ishiguro et al., 2010; Parish and Li, 2010). Later, tapetal cells provide energy and materials for pollen development and pollen coat formation (Wu et al., 1997; Wang et al., 2003; Parish and Li, 2010; Huang et al., 2017). Lack of a tapetum or an abnormal tapetum impairs microspore and pollen development, causing male sterility (Mariani et al., 1990; Zhao et al., 2002; Zhang et al., 2014). Furthermore, endothecium is necessary for anther dehiscence (Cecchetti et al., 2013; Murphy et al., 2015).
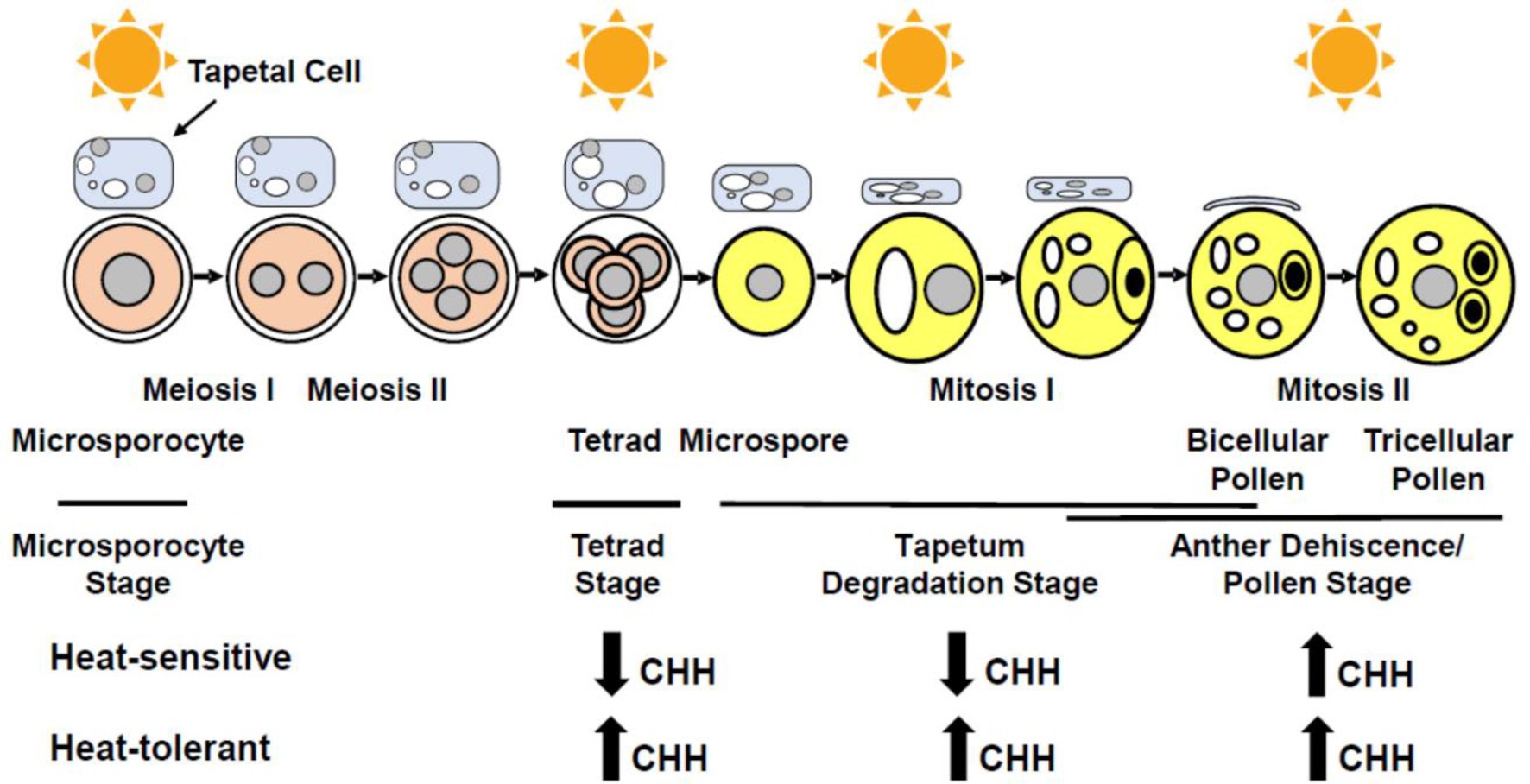
Figure 1. Schematic representation of stages susceptible to heat stress (indicated by sun symbols) during male reproduction and their methylation patterns. CHH methylation is decreased under heat stress at tetrad and tapetum degradation stages in anthers of heat-sensitive plants. CHH methylation is increased under heat stress at tetrad and tapetum degradation stages in anthers of heat-tolerant plants, as well as at the anther dehiscence/pollen stage in anthers of both heat-sensitive and -tolerant plants (H in CHH representing A, T, or G).
Heat stress causes male sterility and seed yield loss are mainly ascribed to aberrant tapetum and pollen development (Parish et al., 2012; De Storme and Geelen, 2014). Decreased pollen viability due to heat stress has been reported in many crops, such as common bean (Gross and Kigel, 1994; Prasad et al., 2002), rice (Endo et al., 2009), cotton (Min et al., 2014; Song et al., 2015), tomato (Pressman et al., 2002; Giorno et al., 2013), pepper (Erickson and Markhart, 2002), wheat (Saini and Aspinall, 1982; Saini et al., 1984), barley (Sakata et al., 2010), cowpea (Ahmed et al., 1992), peanut (Vara Prasad et al., 1999; Zoong Lwe et al., 2020), and flax (Cross et al., 2003; Table 1). In crops, such as wheat, episodes of male sterility were observed upon 3 days of treatment at 30/30°C (day/night, the same thereafter) during meiosis, and irregular tapetum degeneration is a plausible cause for pollen abortion (Saini et al., 1984). In heat-sensitive wheat varieties, elevated temperature (35/24°C) caused tapetum degradation and pollen abortion (Browne et al., 2021). Premature pollen development in common bean at 33/29°C is also a result of early tapetum degeneration (Suzuki et al., 2001). Furthermore, abnormally wavy, looped endoplasmic reticulum (ER) structures were detected in heat-stressed tapetal cells (Suzuki et al., 2001), suggesting that ER malfunction in tapetal cells might cause male sterility under heat stress (De Storme and Geelen, 2014). Heat stress results in DNA fragmentation, cytoplasmic shrinkage, and vacuolation in early tapetal cells of thermosensitive genic male-sterile (TGMS) rice, suggesting that the precocious programmed cell death (PCD) of tapetal cells during heat stress causes male sterility (Ku et al., 2003). Impaired tapetal cells by heat stress also affects callose degradation in PMCs and pollen wall formation, such as exine patterning (Suzuki et al., 2001; Parish et al., 2012; Djanaguiraman et al., 2014). Moderately high temperature (30/25°C) causes aberrant mitochondria, ER, and nuclear membranes in PMCs (Oshino et al., 2007). Moreover, abnormal meiosis occurred in PMCs in heat-stressed wheat (Omidi et al., 2014). Recently, abnormal cross-over was observed in Arabidopsis male meiocytes under high temperature (De Storme and Geelen, 2020). Heat stress (36–38°C) also impaired chromosome segregation and cytokinesis during male meiosis in Arabidopsis (Lei et al., 2020). Moreover, acute heat stress on Arabidopsis causes defects in male germline and sporophytic anther tissues (Hedhly et al., 2020). A recent report showed that pollen abortion was subjected to heat stress (35/25°C) at the pre-meiotic stage in maize with downregulated MAGO (MALE-ASSOCIATED ARGONAUTE-1 and -2) genes (Lee et al., 2021). Further studies revealed that heat stress induced MAGO hypophosphorylation which affects accumulation of 21-nt phasiRNAs and then the activity of retrotransposons in anther wall cells. Thus, the surveillance mechanism mediated by Argonaute is important for protecting male sterility under heat stress.
Anther wall cells and pollen in tomato plants upon heat stress (32/26°C) witness decreased starch and soluble sugar contents (Pressman et al., 2002). In sorghum, heat-stressed (36/26°C) microspores also showed reduced starch content and sucrose deficiency, thus reducing pollen germination (Jain et al., 2007). Moreover, an imbalance in ROS (reactive oxygen species) homeostasis in tapetal cells due to heat stress possibly causes early PCD of tapetal cells (De Storme and Geelen, 2014). In rice anthers, ROS and superoxide dismutase (SOD) are significantly increased at the male meiosis stage (Zhao et al., 2018). In barley, male sterility is possibly attributed to the hyper-phosphorylation of the serine-5 residue at the C-terminal domain of RNA Polymerase (RNA Pol) II, which alters expression of many genes during early anther development under high-temperature conditions (Abiko et al., 2005). Furthermore, auxin synthesis in Arabidopsis and barley anthers are reduced during high temperatures, whereas exogenous application of auxin to anthers improved pollen thermotolerance in barley (Sakata et al., 2010; Higashitani, 2013). Auxin biosynthesis genes, such as YUCCA-YUC2 and YUC6, were suppressed in anthers exposed to high temperatures (33°C; Sakata et al., 2010). Heat stress generally alters expression of various genes which affect cell proliferation, photosynthesis, hormones, starch metabolism, heat shock response, and ROS production (Yang et al., 2006; Yamakawa et al., 2007; Endo et al., 2009; Frank et al., 2009; Bita et al., 2011; Mangelsen et al., 2011; Guan et al., 2013; Min et al., 2014; Song et al., 2014; Fragkostefanakis et al., 2015; González-Schain et al., 2016; Zhang et al., 2017; Zhao et al., 2018; Begcy et al., 2019; Qian et al., 2019b). Here we mainly discuss the epigenetic mechanisms by which plants respond to heat stress during male reproduction.
Epigenetic Modifications During Heat Stress Response
In contrast with the molecular mechanisms underlying heat stress at the transcriptional level, epigenetic regulation during high-temperature stress is not well understood in plants (Ohama et al., 2017). Different plant organs/cells have been studied to understand the role of epigenetic modifications during heat stress. For instance, exposure of soybean root hairs and roots stripped root hairs to heat stress (40°C) caused hypomethylation of CHH (H = A, T or C; Hossain et al., 2017). Heat stress also induced hypomethylation of CG and CHG in cultured microspores of Brassica napus (Li et al., 2016). In maize seedlings, 325 differentially methylated genes (DMG) were identified responding to heat stress (42°C). Interestingly, 9 DMG associated with spliceosome showed the decreased methylation level during heat stress (Qian et al., 2019a). Moreover, the Brassica napus heat-sensitive genotype possesses a higher level of DNA methylation than the heat-tolerant genotype during heat stress (37–45°C; Gao et al., 2014). Collectively, these findings reveal that DNA methylation is responsive to heat stress. The effect of heat stress on methylation in various plants is summarized in Table 2.
Genes involved in DNA methylation, histone modification, chromatin modeling, and small RNA biogenesis were studied for their roles in response to heat stress. Loss-of-function mutant of the NUCLEAR RNA POLYMERASE D2A (NRPD2) gene which encodes the second largest subunit of RNA POL IV and POL V is sensitive to heat stress (Popova et al., 2013). A RPD3-type of histone deacetylase mutant hda6 is sensitive to heat stress. In contrast, DNA methyltransferase mutants, such as domains rearranged methylase1 (drm1), domains rearranged methylase2 (drm2), and chromomethylase3(cmt3), presented less pronounced response to heat stress (Popova et al., 2013). Interestingly in wild-type Arabidopsis plants, heat stress induced expression of the key DNA methyltransferase gene DRM2 as well as NUCLEAR RNA POLYMERASE D1A (NRPD1) and NUCLEAR RNA POLYMERASE D1B (NRPE1) which encode the largest subunit of RNA Pol IV and RNA Pol V, respectively (Naydenov et al., 2015). Conversely, the prolonged heat exposure decreased expression of DNA methyltransferase genes METHYLASE1 (MET1) and CHROMOMETHYLASE3(CMT3; Naydenov et al., 2015). The DRM2 expression during heat stress might be regulated by RNA Pol IV and/or RNA Pol V (Naydenov et al., 2015).
DNA methylation associated with NRPD2 and histone modification mediated by HDA6 might play different roles in transcriptional reprogramming for coping with heat stress. Transcriptomic analysis of directly heat-stressed hda6 mutants revealed a larger set of mis-regulated genes comparing with the heat-stressed nrpd2 mutant, while after recovery from heat stress a much broader transcriptional response was detected in nrpd2 mutants than hda6 mutants and wild-type plants (Popova et al., 2013). In hda6 mutants, mis-regulated genes are involved in diverse functions, such as protein processing, hormone signaling, vegetative and reproductive development, transport, and metabolism; however, GO enrichment analysis found that mis-regulated genes in nrpd2 mutants were associated with starch catabolism, fatty acid oxidation, abiotic stress response, and auxin and cytokinin signaling pathways. A little overlap of mis-regulated gene sets between hda6 and nrpd2 mutants suggests that HDA6 and NRPD2 function differently at different stages of heat response (Popova et al., 2013). Similarly, in the heat-stressed (42°C) maize seedling, some of the key KEGG pathway enrichment involve spliceosome, RNA transport, ubiquitin-mediated proteolysis, and carbon metabolism (Qian et al., 2019a), suggesting that heat stress affects a diverse range of biological pathways which might be regulated via the epigenetic control.
Heat stress activates the ONSEN (“hot spring” in Japanese) retrotransposon and synthesis of extrachromosomal DNA copies in Arabidopsis seedlings (Ito et al., 2011). Heat stress triggers accumulation of ONSEN in mutants lacking RNA Pol IV and RDR2, which are main components in the RdDM pathway. Interestingly, the memory of heat stress (i.e., transgenerational inheritance of ONSEN insertion) can only occur in the progeny of mutant plants defective in siRNA biogenesis. Heat stress induced epigenetic memory associated with hypermethylation of H3K4me2 and H3K4me3 can be maintained for several days in Arabidopsis somatic cells (Lamke et al., 2016). Moreover, transgenerational epigenetic memory induced by heat stress is transmitted via HEAT SHOCK TRANSCRIPTION FACTOR A2 (HSFA2) activated H3K27me3 demethylase in Arabidopsis (Liu et al., 2019; Yamaguchi et al., 2021). Thus, histone modification is essential for thermotolerance memory.
Epigenetic Regulation of Heat Stress During Male Reproduction
Besides genetic regulation, the epigenetic control, particularly DNA methylation, is an important mechanism for plants to manage heat stress during male reproduction. RNA-directed DNA methylation (RdDM) in plants involves various components, such as small interfering RNAs (siRNA) and DNA methyltransferase DRM2 (Law and Jacobsen, 2010). Methylation of DNA occurs at specific sites: symmetric patterns of CpG/CpNpG and asymmetric CpNpN. In plants, methylation of asymmetric cytosine (CpNpG) is regulated by CHROMOMETHYLASE (CMT; Bartee et al., 2001).
Pollen comprises one vegetative nucleus and two sperm nuclei which maintain more stable methylation patterns than leaves and roots (Hsieh et al., 2016). The vegetative nucleus lacks DECREASE IN DNA METHYLATION 1 (DDM1), leading to reactivation of transposable elements. Reduction of DNA methylation in pollen causes transcriptional reprogramming (Slotkin et al., 2009). Cell-specific DNA methylation studies revealed that CG and CHG methylation were retained in microspores and sperm cells, whereas the CHH methylation was lost (Calarco et al., 2012). Interestingly, DNA methylation is reestablished in the vegetative cell via siRNA-mediated RdDM (Calarco et al., 2012). Repetitive elements were found to be active during pollen development (Slotkin et al., 2009), while heat stress can activate repetitive elements in Arabidopsis seedlings by epigenetic regulation (Pecinka et al., 2010). Most key genes required for DNA methylation, such as DRM2, NRPD1, and NRPE1, are upregulated during heat stress in Arabidopsis (Naydenov et al., 2015), supporting the involvement of DNA methylation in heat stress. New findings suggest that sperm cells have asymmetric mCHG, whereas vegetative nuclei and microspores possess symmetric mCHG (Borges et al., 2021). DNA methylation changes during male reproductive development were recently summarized (Papareddy and Nodine, 2021).
Transcriptome studies on heat-treated cotton anthers identified various genes involved in histone modification and DNA methylation. Under heat stress, the heat-tolerant cotton line produces normal anthers and pollen, while the heat-sensitive line is defective in anther dehiscence and fails to form viable pollen. Heat stress decreased expression of DNA CYTOSINE-5-METHYLTRANSFERASE (DRM1) and S-ADENOSYL-l-METHIONINE-DEPENDENT METHYLTRANSFERASE (DRM3) at tetrad and tapetum degradation stages in heat-sensitive cotton anthers, while their expression remains similar in heat-tolerant cotton anthers with an exception of increased expression of DRM3 at the tetrad stage (Min et al., 2014). Similarly, expression of NEEDED FOR RDR2-INDEPENDENT DNA METHYLATION (NERD), NUCLEAR RNA POLYMERASE D1B (NRPD1B), and S-ADENOSYL-L-HOMOCYSTEINE HYDROLASE1 (SAHH1), which are required for normal DNA methylation, is suppressed by heat stress in heat-sensitive cotton anthers (Min et al., 2014). During heat stress, heat-sensitive cotton anthers undergo DNA hypomethylation, while heat-tolerant cotton anthers have a high level of DNA methylation. Furthermore, pollen sterility and defects in anther dehiscence are possibly caused by hypomethylation in the heat-sensitive cotton (Ma et al., 2018). Studies on expression changes of genes associated with DNA methylation in cotton anthers under heat stress provide strong evidence that the epigenetic regulation is required for plants to cope with heat stress.
CHH methylation mediated by RdDM showed more prominent changes comparing to CG and CHG methylation, suggesting that heat stress mainly induces the RdDM activity in anthers. Most of heat-induced CHH methylations were found in promoters and downstream regions of protein-coding genes (Ma et al., 2018). Interestingly, the DNA methylation status varies with anther stages upon heat stress. At tetrad, tapetum degradation, and anther dehiscence/pollen stages, the CHH methylation level in heat-tolerant cotton anthers is increased upon heat stress; however, heat-sensitive cotton anthers depicted hypo-CHH methylation patterns at tetrad and tapetum degradation stages, while an increased CHH methylation level at the anther dehiscence/pollen stage during heat stress (Figure 1). Hence, heat stress may affect RdDM function in an anther stage-specific manner (Ma et al., 2018). Heat stress alters the DNA methylation level, which affects expression of genes involved in sugar metabolism and ROS generation. The abnormal concentration of sugar and ROS therefore impairs anther and pollen development. These discoveries shed light on a novel molecular mechanism by which plants ensure the success of male reproduction under high temperature, thus providing new tools for improving crops to adapt to the challenge of global warming.
Long non-coding RNA (lncRNA) is important for male fertility. In rice, an lncRNA named the long-day-specific male-fertility-associated RNA (LDMAR) is essential for pollen development under the long-day condition (Ding et al., 2012). A single nucleotide mutation in LDMAR increased CG methylation in the LDMAR promoter region, which decreased the LDMAR expression and thus induced PCD in anther cells. The lncRNA expression responds to stresses spatially and temporally in plants (Yu et al., 2019). Among 54 putative heat stress-induced lncRNAs, TahlnRNA27 and TalnRNA5 were highly upregulated by heat stress in wheat (Xin et al., 2011). Differentially expressed lncRNAs were also observed during heat stress in Brassica rapa (Wang et al., 2019), Brassica juncea (Bhatia et al., 2020), and maize (Lv et al., 2019). A recent study in Arabidopsis showed that 131 pollen-specific intergenic expressed loci (XLOC), which mostly encode lncRNAs, are heat stress responsive (Rutley et al., 2021). These results suggest that lncRNAs might play an important role in heat stress response during male reproduction via epigenetic regulation.
MicroRNAs (miRNAs) are another set of non-coding RNAs which are known to regulate gene expression at the post-transcriptional level (Bartel, 2004; Liu et al., 2010; Chen et al., 2016; Huang et al., 2016). In Brassica rapa seedlings, heat stress significantly decreased expression of novel miRNAs bra-miR1885b.3 and bra-miR5716 (Yu et al., 2011). In barley, heat stress induced expression of miR160a, 166a, 167h, and 5175a, while expression levels of their target genes, such as AUXIN RESPONSE TRANSCRIPTION FACTORs (ARFs), were reduced upon heat stress (Kruszka et al., 2014). In Arabidopsis, the miR398 expression was rapidly induced by heat stress, while its target genes like CSD (encoding the copper/zinc SOD) and CCS (encoding a chaperone for CSD) were downregulated by heat stress (Guan et al., 2013). Moreover, heat shock factors HSFA1b and HSFA7b are required for heat stress induced the miR398 expression. Furthermore, the heat stress-induced miR156 plays a crucial role in regulating heat stress memory via repressing expression of SPL (SQUAMOSA-PROMOTER BINDING-LIKE) genes (Stief et al., 2014). These results suggest that miRNAs are generally important for heat stress response in plants.
In both heat-tolerant and heat-sensitive cotton anthers, heat stress repressed the miR156 expression, which consequently increased expression of its target SPL genes (Ding et al., 2017). The miR160 expression was suppressed in heat-tolerant cotton but increased in heat-sensitive cotton under heat stress. MiR160 target genes ARF10 and ARF17 showed opposite expression pattens to miR160. A recent study identified a plethora of miRNAs which respond to heat stress at a stage-specific manner during cotton anther development (Chen et al., 2020). For instance, expression of miR160, miR167, and miR2949 was elevated at the sporogenous cell proliferation stage under high temperature, while miR156 responded to heat stress at male meiosis and microspore release stages. MiRNAs are also involved in epigenetic regulation via controlling DNA methylation and histone modification. MiR165/166 mediates methylation of downstream coding sequences of their target genes PHABULOSA and PHAVOLUTA in Arabidopsis (Bao et al., 2004). MiR156 and its target genes SPLs control transition from juvenile to adult phase in Arabidopsis (Xu et al., 2018; Manuela and Xu, 2020). MIR156A and MIR156C loci are major contributors to the formation of mature miR156. The H2A histone variant H2A.Z promotes expression of MIR156A and MIR156C via increasing the H3K4me3 level in these two loci (Xu et al., 2018). Although lacking direct evidence, it is possible that miRNAs cope with heat stress via epigenetic regulation during male reproduction in plants.
Conclusion and Perspectives
Male reproductive development is highly susceptible to episodes of heat stress. Heat stress leads to impaired tapetum, abnormal microspores, and pollen abortion, which cause male sterility in plants and adversely affect yield due to failure or reduction in fertilization. Different plants respond to heat stress differently, which makes it important to identify key stages susceptible to heat stress during male reproduction. This can help take correct measures to protect plants against heat stress at specific stages during plant male reproduction.
At the molecular level, plants respond to heat stress in multiple ways. Molecular genetics, transcriptomic, and proteomic studies identified a wide array of genes and gene networks associated with heat stress during male reproduction in various crops (Giorno et al., 2013; Zhang et al., 2017; Keller and Simm, 2018; Begcy et al., 2019; Liu et al., 2020; Lohani et al., 2020; Chaturvedi et al., 2021). During male reproduction, heat stress not only affects expression of genes controlling epigenetic modifications, but also ultimately alters DNA methylation status. LncRNA and miRNA also appear important for heat stress response during plant male reproductive development, further suggesting that epigenetic control is a critical means for plants to cope with heat stress.
It is imperative to elucidate functional significance of epigenetic modifications and associated genes in heat stress response during male reproduction in economic plants. Tapetal cells, male meiocytes (microsporocytes), microspores, and pollen are sensitive to high temperature (Figure 1). Tapetal cells are special in terms of their endopolyploidy, formation of unique organelles (i.e., elaioplast, tapetosome, and ubisch body), highly active carbohydrate and lipid metabolism, and PCD. Tapetal cells are required for releasing haploid microspores from tetrads and for supplying energy and materials for pollen development and pollen coat formation. Numerous studies using various plants have found that heat stress affects tapetal cell differentiation and degeneration, which consequently leads to abnormal microspores and pollen abortion. Thus, it is necessary to preform single-cell transcriptomic and proteomic analyses to identify genes, gene networks, as well as particularly DNA methylation and histone modification marks that are responsible for heat stress in tapetal cells, male meiocytes, microspores, and pollen. In addition, it would be worthwhile to investigate transgenerational epigenetic effects (epigenetic memory) on heat tolerance during male reproduction in plants. CRISPR-based targeted modification of epigenetic marks has emerged as a powerful tool for improving plant traits, such as heat tolerance (Ghoshal et al., 2021). Although emerging evidence suggests the importance of epigenetic regulation for heat stress response especially during male reproduction, applying the related findings to generating thermotolerant crops via genetic engineering and molecular breeding is still a challenge.
Author Contributions
SM and DZ conceived the idea and wrote the manuscript. All authors contributed to the article and approved the submitted version.
Funding
The research in the DZ lab is supported by the National Science Foundation (NSF IOS-1322796) and USDA National Institute of Food and Agriculture (NIFA, 2022-67013-36294).
Conflict of Interest
The authors declare that the research was conducted in the absence of any commercial or financial relationships that could be construed as a potential conflict of interest.
Publisher’s Note
All claims expressed in this article are solely those of the authors and do not necessarily represent those of their affiliated organizations, or those of the publisher, the editors and the reviewers. Any product that may be evaluated in this article, or claim that may be made by its manufacturer, is not guaranteed or endorsed by the publisher.
Acknowledgments
DZ also gratefully acknowledges supports by the Shaw Scientist Award from the Greater Milwaukee Foundation, the Research and Creative Activities Support (RACAS) program at the University of Wisconsin-Milwaukee, the Bradley Catalyst Award from the UWM Research Foundation, and WiSys and UW System applied research funding programs.
References
Abiko, M., Akibayashi, K., Sakata, T., Kimura, M., Kihara, M., Itoh, K., et al. (2005). High-temperature induction of male sterility during barley (Hordeum vulgare L.) anther development is mediated by transcriptional inhibition. Sex. Plant Reprod. 18, 91–100. doi: 10.1007/s00497-005-0004-2
Ahmed, F. E., Hall, A. E., and DeMason, D. A. (1992). Heat injury during floral development in cowpea (Vigna unguiculata, Fabaceae). Am. J. Bot. 79, 784–791. doi: 10.1002/j.1537-2197.1992.tb13655.x
Bao, N., Lye, K. W., and Barton, M. K. (2004). MicroRNA binding sites in Arabidopsis class III HD-ZIP mRNAs are required for methylation of the template chromosome. Dev. Cell 7, 653–662. doi: 10.1016/j.devcel.2004.10.003
Bartee, L., Malagnac, F., and Bender, J. (2001). Arabidopsis cmt3 chromomethylase mutations block non-CG methylation and silencing of an endogenous gene. Genes Dev. 15, 1753–1758. doi: 10.1101/gad.905701
Bartel, D. P. (2004). MicroRNAs: genomics, biogenesis, mechanism, and function. Cell 116, 281–297. doi: 10.1016/S0092-8674(04)00045-5
Begcy, K., Nosenko, T., Zhou, L.-Z., Fragner, L., Weckwerth, W., and Dresselhaus, T. (2019). Male sterility in maize after transient heat stress during the tetrad stage of pollen development. Plant Physiol. 181, 683–700. doi: 10.1104/pp.19.00707
Bhatia, G., Singh, A., Verma, D., Sharma, S., and Singh, K. (2020). Genome-wide investigation of regulatory roles of lncRNAs in response to heat and drought stress in Brassica juncea (Indian mustard). Environ. Exp. Bot. 171:103922. doi: 10.1016/j.envexpbot.2019.103922
Bita, C. E., Zenoni, S., Vriezen, W. H., Mariani, C., Pezzotti, M., and Gerats, T. (2011). Temperature stress differentially modulates transcription in meiotic anthers of heat-tolerant and heat-sensitive tomato plants. BMC Genomics 12:384. doi: 10.1186/1471-2164-12-384
Borges, F., Donoghue, M. T. A., LeBlanc, C., Wear, E. E., Tanurdžić, M., Berube, B., et al. (2021). Loss of small-RNA directed DNA methylation in the plant cell cycle promotes germline reprogramming and somaclonal variation. Curr. Biol. 31, 591–600.e594. doi: 10.1016/j.cub.2020.10.098
Browne, R. G., Li, S. F., Iacuone, S., Dolferus, R., and Parish, R. W. (2021). Differential responses of anthers of stress tolerant and sensitive wheat cultivars to high temperature stress. Planta 254:4. doi: 10.1007/s00425-021-03656-7
Calarco, J. P., Borges, F., Donoghue, M. T. A., Van Ex, F., Jullien, P. E., Lopes, T., et al. (2012). Reprogramming of DNA methylation in pollen guides epigenetic inheritance via small RNA. Cell 151, 194–205. doi: 10.1016/j.cell.2012.09.001
Cecchetti, V., Altamura, M. M., Brunetti, P., Petrocelli, V., Falasca, G., Ljung, K., et al. (2013). Auxin controls Arabidopsis anther dehiscence by regulating endothecium lignification and jasmonic acid biosynthesis. Plant J. 74, 411–422. doi: 10.1111/tpj.12130
Chaturvedi, P., Wiese, A. J., Ghatak, A., Záveská Drábková, L., Weckwerth, W., and Honys, D. (2021). Heat stress response mechanisms in pollen development. New Phytol. 231, 571–585. doi: 10.1111/nph.17380
Chen, Y., Müller, F., Rieu, I., and Winter, P. (2016). Epigenetic events in plant male germ cell heat stress responses. Plant Reprod. 29, 21–29. doi: 10.1007/s00497-015-0271-5
Chen, J., Pan, A., He, S., Su, P., Yuan, X., Zhu, S., et al. (2020). Different MicroRNA families involved in regulating high temperature stress response during cotton (Gossypium hirsutum L.) anther development. Int. J. Mol. Sci. 21:1280. doi: 10.3390/ijms21041280
Clément, C., and Pacini, E. (2001). Anther plastids in angiosperms. Bot. Rev. 67:54. doi: 10.1007/BF02857849
Cross, R., McKay, S. G., McHughen, A., and Bonham-Smith, P. (2003). Heat-stress effects on reproduction and seed set in Linum usitatissimum L.(flax). Plant Cell Environ. 26, 1013–1020. doi: 10.1046/j.1365-3040.2003.01006.x
De Storme, N., and Geelen, D. (2014). The impact of environmental stress on male reproductive development in plants: biological processes and molecular mechanisms. Plant Cell Environ. 37, 1–18. doi: 10.1111/pce.12142
De Storme, N., and Geelen, D. (2020). High temperatures alter cross-over distribution and induce male meiotic restitution in Arabidopsis thaliana. Commun. Biol. 3:187. doi: 10.1038/s42003-020-0897-1
Ding, J., Lu, Q., Ouyang, Y., Mao, H., Zhang, P., Yao, J., et al. (2012). A long noncoding RNA regulates photoperiod-sensitive male sterility, an essential component of hybrid rice. Proc. Natl. Acad. Sci. 109, 2654–2659. doi: 10.1073/pnas.1121374109
Ding, Y., Ma, Y., Liu, N., Xu, J., Hu, Q., Li, Y., et al. (2017). microRNAs involved in auxin signalling modulate male sterility under high-temperature stress in cotton (Gossypium hirsutum). Plant J. 91, 977–994. doi: 10.1111/tpj.13620
Djanaguiraman, M., Vara Prasad, P. V., Murugan, M., Perumal, R., and Reddy, U. K. (2014). Physiological differences among sorghum (Sorghum bicolor L. Moench) genotypes under high temperature stress. Environ. Exp. Bot. 100, 43–54. doi: 10.1016/j.envexpbot.2013.11.013
Endo, M., Tsuchiya, T., Hamada, K., Kawamura, S., Yano, K., Ohshima, M., et al. (2009). High temperatures cause male sterility in rice plants with transcriptional alterations during pollen development. Plant Cell Physiol. 50, 1911–1922. doi: 10.1093/pcp/pcp135
Erickson, A., and Markhart, A. (2002). Flower developmental stage and organ sensitivity of bell pepper (Capsicum annuum L.) to elevated temperature. Plant Cell Environ. 25, 123–130. doi: 10.1046/j.0016-8025.2001.00807.x
Feng, X., Zilberman, D., and Dickinson, H. (2013). A conversation across generations: Soma-germ cell crosstalk in plants. Dev. Cell 24, 215–225. doi: 10.1016/j.devcel.2013.01.014
Fragkostefanakis, S., Mesihovic, A., Simm, S., Paupière, M. J., Hu, Y., Paul, P., et al. (2016). HsfA2 controls the activity of developmentally and stress-regulated heat stress protection mechanisms in tomato male reproductive tissues. Plant Physiol. 170, 2461–2477. doi: 10.1104/pp.15.01913
Fragkostefanakis, S., Simm, S., Paul, P., Bublak, D., Scharf, K. D., and Schleiff, E. (2015). Chaperone network composition in Solanum lycopersicum explored by transcriptome profiling and microarray meta-analysis. Plant Cell Environ. 38, 693–709. doi: 10.1111/pce.12426
Frank, G., Pressman, E., Ophir, R., Althan, L., Shaked, R., Freedman, M., et al. (2009). Transcriptional profiling of maturing tomato (Solanum lycopersicum L.) microspores reveals the involvement of heat shock proteins, ROS scavengers, hormones, and sugars in the heat stress response. J. Exp. Bot. 60, 3891–3908. doi: 10.1093/jxb/erp234
Gao, G., Li, J., Li, H., Li, F., Xu, K., Yan, G., et al. (2014). Comparison of the heat stress induced variations in DNA methylation between heat-tolerant and heat-sensitive rapeseed seedlings. Breed. Sci. 64, 125–133. doi: 10.1270/jsbbs.64.125
Ghoshal, B., Picard, C. L., Vong, B., Feng, S., and Jacobsen, S. E. (2021). CRISPR-based targeting of DNA methylation in Arabidopsis thaliana by a bacterial CG-specific DNA methyltransferase. Proc. Natl. Acad. Sci. 118:e2125016118. doi: 10.1073/pnas.2125016118
Giorno, F., Wolters-Arts, M., Mariani, C., and Rieu, I. (2013). Ensuring reproduction at high temperatures: the heat stress response during anther and pollen development. Plan. Theory 2, 489–506. doi: 10.3390/plants2030489
Goldberg, R. B., Beals, T. P., and Sanders, P. M. (1993). Anther development: basic principles and practical applications. Plant Cell 5, 1217–1229. doi: 10.1105/tpc.5.10.1217
González-Schain, N., Dreni, L., Lawas, L. M., Galbiati, M., Colombo, L., Heuer, S., et al. (2016). Genome-wide transcriptome analysis during anthesis reveals new insights into the molecular basis of heat stress responses in tolerant and sensitive rice varieties. Plant Cell Physiol. 57, 57–68. doi: 10.1093/pcp/pcv174
Gross, Y., and Kigel, J. (1994). Differential sensitivity to high temperature of stages in the reproductive development of common bean (Phaseolus vulgaris L.). Field Crop Res. 36, 201–212. doi: 10.1016/0378-4290(94)90112-0
Guan, Q., Lu, X., Zeng, H., Zhang, Y., and Zhu, J. (2013). Heat stress induction of miR398 triggers a regulatory loop that is critical for thermotolerance in Arabidopsis. Plant J. 74, 840–851. doi: 10.1111/tpj.12169
Harsant, J., Pavlovic, L., Chiu, G., Sultmanis, S., and Sage, T. L. (2013). High temperature stress and its effect on pollen development and morphological components of harvest index in the C3 model grass Brachypodium distachyon. J. Exp. Bot. 64, 2971–2983. doi: 10.1093/jxb/ert142
He, J., Jiang, Z., Gao, L., You, C., Ma, X., Wang, X., et al. (2019). Genome-wide transcript and small RNA profiling reveals transcriptomic responses to heat stress. Plant Physiol. 181, 609–629. doi: 10.1104/pp.19.00403
Hedhly, A., Nestorova, A., Herrmann, A., and Grossniklaus, U. (2020). Acute heat stress during. Stamen development affects both the germline and sporophytic lineages in Arabidopsis thaliana (L.) Heynh. Environ. Exp. Bot. 173:103992. doi: 10.1016/j.envexpbot.2020.103992
Higashitani, A. (2013). High temperature injury and auxin biosynthesis in microsporogenesis. Front. Plant Sci. 4:47. doi: 10.3389/fpls.2013.00047
Hossain, M. S., Kawakatsu, T., Kim, K. D., Zhang, N., Nguyen, C. T., Khan, S. M., et al. (2017). Divergent cytosine DNA methylation patterns in single-cell, soybean root hairs. New Phytol. 214, 808–819. doi: 10.1111/nph.14421
Hsieh, P.-H., He, S., Buttress, T., Gao, H., Couchman, M., Fischer, R. L., et al. (2016). Arabidopsis male sexual lineage exhibits more robust maintenance of CG methylation than somatic tissues. Proc. Natl. Acad. Sci. 113, 15132–15137. doi: 10.1073/pnas.1619074114
Hsieh, K., and Huang, A. H. C. (2007). Tapetosomes in brassica Tapetum accumulate endoplasmic reticulum–derived flavonoids and alkanes for delivery to the pollen surface. Plant Cell 19, 582–596. doi: 10.1105/tpc.106.049049
Huang, J., Li, Z., Biener, G., Xiong, E., Malik, S., Eaton, N., et al. (2017). Carbonic anhydrases function in anther cell differentiation downstream of the receptor-Like kinase EMS1. Plant Cell 29, 1335–1356. doi: 10.1105/tpc.16.00484
Huang, J., Li, Z., and Zhao, D. (2016). Deregulation of the OsmiR160 target gene OsARF18 causes growth and developmental defects with an alteration of auxin signaling in rice. Sci. Rep. 6:29938. doi: 10.1038/srep29938
Ishiguro, S., Nishimori, Y., Yamada, M., Saito, H., Suzuki, T., Nakagawa, T., et al. (2010). The Arabidopsis FLAKY POLLEN1 gene encodes a 3-hydroxy-3-methylglutaryl-coenzyme A synthase required for development of tapetum-specific organelles and fertility of pollen grains. Plant Cell Physiol. 51, 896–911. doi: 10.1093/pcp/pcq068
Ito, H., Gaubert, H., Bucher, E., Mirouze, M., Vaillant, I., and Paszkowski, J. (2011). An siRNA pathway prevents transgenerational retrotransposition in plants subjected to stress. Nature 472, 115–119. doi: 10.1038/nature09861
Jain, M., Prasad, P. V. V., Boote, K. J., Hartwell, A. L., and Chourey, P. S. (2007). Effects of season-long high temperature growth conditions on sugar-to-starch metabolism in developing microspores of grain sorghum (Sorghum bicolor L. Moench). Planta 227, 67–79. doi: 10.1007/s00425-007-0595-y
Keller, M., and Simm, S. (2018). The coupling of transcriptome and proteome adaptation during development and heat stress response of tomato pollen. BMC Genomics 19:447. doi: 10.1186/s12864-018-4824-5
Korotko, U., Chwiałkowska, K., Sańko-Sawczenko, I., and Kwasniewski, M. (2021). DNA demethylation in response to heat stress in Arabidopsis thaliana. Int. J. Mol. Sci. 22:1555. doi: 10.3390/ijms22041555
Kotak, S., Larkindale, J., Lee, U., von Koskull-Döring, P., Vierling, E., and Scharf, K.-D. (2007). Complexity of the heat stress response in plants. Curr. Opin. Plant Biol. 10, 310–316. doi: 10.1016/j.pbi.2007.04.011
Kruszka, K., Pacak, A., Swida-Barteczka, A., Nuc, P., Alaba, S., Wroblewska, Z., et al. (2014). Transcriptionally and post-transcriptionally regulated microRNAs in heat stress response in barley. J. Exp. Bot. 65, 6123–6135. doi: 10.1093/jxb/eru353
Ku, S., Yoon, H., Suh, H. S., and Chung, Y.-Y. (2003). Male-sterility of thermosensitive genic male-sterile rice is associated with premature programmed cell death of the tapetum. Planta 217, 559–565. doi: 10.1007/s00425-003-1030-7
Lamke, J., Brzezinka, K., Altmann, S., and Bäurle, I. (2016). A hit-and-run heat shock factor governs sustained histone methylation and transcriptional stress memory. EMBO J. 35, 162–175. doi: 10.15252/embj.201592593
Law, J. A., and Jacobsen, S. E. (2010). Establishing, maintaining and modifying DNA methylation patterns in plants and animals. Nat. Rev. Genet. 11, 204–220. doi: 10.1038/nrg2719
Lee, Y.-S., Maple, R., Dürr, J., Dawson, A., Tamim, S., del Genio, C., et al. (2021). A transposon surveillance mechanism that safeguards plant male fertility during stress. Nat. Plants 7, 34–41. doi: 10.1038/s41477-020-00818-5
Lei, X., Ning, Y., Eid Elesawi, I., Yang, K., Chen, C., Wang, C., et al. (2020). Heat stress. Interferes with chromosome segregation and cytokinesis during male meiosis in Arabidopsis thaliana. Plant Signal. Behav. 15:1746985. doi: 10.1080/15592324.2020.1746985
Li, J., Huang, Q., Sun, M., Zhang, T., Li, H., Chen, B., et al. (2016). Global DNA methylation variations after short-term heat shock treatment in cultured microspores of Brassica napus cv. Topas. Sci. Rep. 6:38401. doi: 10.1038/srep3840
Liu, J., Feng, L., Gu, X., Deng, X., Qiu, Q., Li, Q., et al. (2019). An H3K27me3 demethylase-HSFA2 regulatory loop orchestrates transgenerational thermomemory in Arabidopsis. Cell Res. 29, 379–390. doi: 10.1038/s41422-019-0145-8
Liu, X., Huang, J., Wang, Y., Owen, H. A., and Zhao, D. (2010). The role of floral organs in carpels, an Arabidopsis loss-of-function mutation in MicroRNA160a, in organogenesis and the mechanism regulating its expression. Plant J. 62, 416–428. doi: 10.1111/j.1365-313X.2010.04164.x
Liu, G., Zha, Z., Cai, H., Qin, D., Jia, H., Liu, C., et al. (2020). Dynamic transcriptome analysis of anther response to heat stress during anthesis in thermotolerant rice (Oryza sativa L.). Int. J. Mol. Sci. 21:1155. doi: 10.3390/ijms21031155
Lohani, N., Singh, M. B., and Bhalla, P. L. (2020). High temperature susceptibility of sexual reproduction in crop plants. J. Exp. Bot. 71, 555–568. doi: 10.1093/jxb/erz426
Lv, Y., Hu, F., Zhou, Y., Wu, F., and Gaut, B. S. (2019). Maize transposable elements contribute to long non-coding RNAs that are regulatory hubs for abiotic stress response. BMC Genomics 20:864. doi: 10.1186/s12864-019-6245-5
Ma, Y., Min, L., Wang, M., Wang, C., Zhao, Y., Li, Y., et al. (2018). Disrupted genome methylation in response to high temperature has distinct affects on microspore abortion and anther indehiscence. Plant Cell 30, 1387–1403. doi: 10.1105/tpc.18.00074
Mangelsen, E., Kilian, J., Harter, K., Jansson, C., Wanke, D., and Sundberg, E. (2011). Transcriptome analysis of high-temperature stress in developing barley caryopses: early stress responses and effects on storage compound biosynthesis. Mol. Plant 4, 97–115. doi: 10.1093/mp/ssq058
Manuela, D., and Xu, M. (2020). Juvenile leaves or adult leaves: determinants for vegetative phase change in flowering plants. Int. J. Mol. Sci. 21:9753. doi: 10.3390/ijms21249753
Mariani, C., Beuckeleer, M. D., Truettner, J., Leemans, J., and Goldberg, R. B. (1990). Induction of male sterility in plants by a chimaeric ribonuclease gene. Nature 347, 737–741. doi: 10.1038/347737a0
Min, L., Li, Y. Y., Hu, Q., Zhu, L. F., Gao, W. H., Wu, Y. L., et al. (2014). Sugar and auxin signaling pathways respond to high-temperature stress during anther development as revealed by transcript profiling analysis in cotton. Plant Physiol. 164, 1293–1308. doi: 10.1104/pp.113.232314
Murphy, K. M., Egger, R. L., and Walbot, V. (2015). Chloroplasts in anther endothecium of Zea mays (Poaceae). Am. J. Bot. 102, 1931–1937. doi: 10.3732/ajb.1500384
Naydenov, M., Baev, V., Apostolova, E., Gospodinova, N., Sablok, G., Gozmanova, M., et al. (2015). High-temperature effect on genes engaged in DNA methylation and affected by DNA methylation in Arabidopsis. Plant Physiol. Biochem. 87, 102–108. doi: 10.1016/j.plaphy.2014.12.022
Ohama, N., Sato, H., Shinozaki, K., and Yamaguchi-Shinozaki, K. (2017). Transcriptional regulatory network of plant heat stress response. Trends Plant Sci. 22, 53–65. doi: 10.1016/j.tplants.2016.08.015
Omidi, M., Siahpoosh, M. R., Mamghani, R., and Modarresi, M. (2014). The influence of terminal heat stress on meiosis abnormalities in pollen mother cells of wheat. Cytologia 79, 49–58. doi: 10.1508/cytologia.79.49
Oshino, T., Abiko, M., Saito, R., Ichiishi, E., Endo, M., Kawagishi-Kobayashi, M., et al. (2007). Premature progression of anther early developmental programs accompanied by comprehensive alterations in transcription during high-temperature injury in barley plants. Mol. Gen. Genomics. 278, 31–42. doi: 10.1007/s00438-007-0229-x
Pacini, E., Franchi, G. G., and Hesse, M. (1985). The Tapetum: its form, function, and possible phylogeny in Embryophyta. Plant Syst. Evol. 149, 155–185. doi: 10.1007/BF00983304
Papareddy, R. K., and Nodine, M. D. (2021). Plant epigenetics: propelling DNA methylation variation across the cell cycle. Curr. Biol. 31, R129–R131. doi: 10.1016/j.cub.2020.11.049
Parish, R. W., and Li, S. F. (2010). Death of a tapetum: a programme of developmental altruism. Plant Sci. 178, 73–89. doi: 10.1016/j.plantsci.2009.11.001
Parish, R. W., Phan, H. A., Iacuone, S., and Li, S. F. (2012). Tapetal development and abiotic stress: a centre of vulnerability. Funct. Plant Biol. 39, 553–559. doi: 10.1071/FP12090
Pecinka, A., Dinh, H. Q., Baubec, T., Rosa, M., Lettner, N., and Scheid, O. M. (2010). Epigenetic regulation of repetitive elements is attenuated by prolonged heat stress in Arabidopsis. Plant Cell 22, 3118–3129. doi: 10.1105/tpc.110.078493
Popova, O. V., Dinh, H. Q., Aufsatz, W., and Jonak, C. (2013). The RdDM pathway is required for basal heat tolerance in Arabidopsis. Mol. Plant 6, 396–410. doi: 10.1093/mp/sst023
Prasad, P. V., Boote, K. J., Allen, L. H. Jr., and Thomas, J. M. (2002). Effects of elevated temperature and carbon dioxide on seed-set and yield of kidney bean (Phaseolus vulgaris L.). Glob. Chang. Biol. 8, 710–721. doi: 10.1046/j.1365-2486.2002.00508.x
Pressman, E., Peet, M. M., and Pharr, D. M. (2002). The effect of heat stress on tomato pollen characteristics is associated with changes in carbohydrate concentration in the developing anthers. Ann. Bot. 90, 631–636. doi: 10.1093/aob/mcf240
Qian, Y., Hu, W., Liao, J., Zhang, J., and Ren, Q. (2019a). The dynamics of DNA methylation in the maize (Zea mays L.) inbred line B73 response to heat stress at the seedling stage. Biochem. Biophys. Res. Commun. 512, 742–749. doi: 10.1016/j.bbrc.2019.03.150
Qian, Y., Ren, Q., Zhang, J., and Chen, L. (2019b). Transcriptomic analysis of the maize (Zea mays L.) inbred line B73 response to heat stress at the seedling stage. Gene 692, 68–78. doi: 10.1016/j.gene.2018.12.062
Rutley, N., Poidevin, L., Doniger, T., Tillett, R. L., Rath, A., Forment, J., et al. (2021). Characterization of novel pollen-expressed transcripts reveals their potential roles in pollen heat stress response in Arabidopsis thaliana. Plant Reprod. 34, 61–78. doi: 10.1007/s00497-020-00400-1
Saini, H., and Aspinall, D. (1982). Abnormal sporogenesis in wheat (Triticum aestivum L.) induced by short periods of high temperature. Ann. Bot. 49, 835–846. doi: 10.1093/oxfordjournals.aob.a086310
Saini, H., Sedgley, M., and Aspinall, D. (1984). Development anatomy in wheat of male sterility induced by heat stress, water deficit or abscisic acid. Funct. Plant Biol. 11, 243–253. doi: 10.1071/PP9840243
Sakata, T., Oshino, T., Miura, S., Tomabechi, M., Tsunaga, Y., Higashitani, N., et al. (2010). Auxins reverse plant male sterility caused by high temperatures. Proc. Natl. Acad. Sci. U.S.A. 107, 8569–8574. doi: 10.1073/pnas.1000869107
Sanders, P. M., Bui, A. Q., Weterings, K., McIntire, K. N., Hsu, Y.-C., Lee, P. Y., et al. (1999). Anther developmental defects in Arabidopsis thaliana male-sterile mutants. Sex. Plant Reprod. 11, 297–322. doi: 10.1007/s004970050158
Sato, H., Mizoi, J., Tanaka, H., Maruyama, K., Qin, F., Osakabe, Y., et al. (2014). Arabidopsis DPB3-1, a DREB2A interactor, specifically enhances heat stress-induced gene expression by forming a heat stress-specific transcriptional complex with NF-Y subunits. Plant Cell 26, 4954–4973. doi: 10.1105/tpc.114.132928
Sato, H., Suzuki, T., Takahashi, F., Shinozaki, K., and Yamaguchi-Shinozaki, K. (2019). NF-YB2 and NF-YB3 have functionally diverged and differentially induce drought and heat stress-specific genes. Plant Physiol. 180, 1677–1690. doi: 10.1104/pp.19.00391
Scott, R. J., Spielman, M., and Dickinson, H. G. (2004). Stamen structure and function. Plant Cell 16, S46–S60. doi: 10.1105/tpc.017012
Slotkin, R. K., Vaughn, M., Borges, F., Tanurdžić, M., Becker, J. D., Feijó, J. A., et al. (2009). Epigenetic reprogramming and small RNA silencing of transposable elements in pollen. Cell 136, 461–472. doi: 10.1016/j.cell.2008.12.038
Smith, A. R., and Zhao, D. Z. (2016). Sterility caused by floral organ degeneration and abiotic stresses in Arabidopsis and cereal grains. Front. Plant Sci. 7:1503. doi: 10.3389/fpls.2016.01503
Song, Y., Chen, Q., Ci, D., Shao, X., and Zhang, D. (2014). Effects of high temperature on photosynthesis and related gene expression in poplar. BMC Plant Biol. 14:111. doi: 10.1186/1471-2229-14-111
Song, G., Wang, M., Zeng, B., Zhang, J., Jiang, C., Hu, Q., et al. (2015). Anther response to high-temperature stress during development and pollen thermotolerance heterosis as revealed by pollen tube growth and in vitro pollen vigor analysis in upland cotton. Planta 241, 1271–1285. doi: 10.1007/s00425-015-2259-7
Stief, A., Altmann, S., Hoffmann, K., Pant, B. D., Scheible, W.-R., and Bäurle, I. (2014). Arabidopsis miR156 regulates tolerance to recurring environmental stress through SPL transcription factors. Plant Cell 26, 1792–1807. doi: 10.1105/tpc.114.123851
Suzuki, K., Takeda, H., Tsukaguchi, T., and Egawa, Y. (2001). Ultrastructural study on degeneration of tapetum in anther of snap bean (Phaseolus vulgaris L.) under heat stress. Sex. Plant Reprod. 13, 293–299. doi: 10.1007/s004970100071
Vara Prasad, P. V., Craufurd, P. Q., and Summerfield, R. J. (1999). Fruit number in relation to pollen production and viability in groundnut exposed to short episodes of heat stress. Ann. Bot. 84, 381–386. doi: 10.1006/anbo.1999.0926
Walbot, V., and Egger, R. L. (2016). Pre-meiotic anther development: cell fate specification and differentiation. Annu. Rev. Plant Biol. 67, 365–395. doi: 10.1146/annurev-arplant-043015-111804
Wang, A., Hu, J., Gao, C., Chen, G., Wang, B., Lin, C., et al. (2019). Genome-wide analysis of long non-coding RNAs unveils the regulatory roles in the heat tolerance of Chinese cabbage (Brassica rapa ssp.chinensis). Sci. Rep. 9:5002. doi: 10.1038/s41598-019-41428-2
Wang, A., Xia, Q., Xie, W., Datla, R., and Selvaraj, G. (2003). The classical Ubisch bodies carry a sporophytically produced structural protein (RAFTIN) that is essential for pollen development. Proc. Natl. Acad. Sci. 100, 14487–14492. doi: 10.1073/pnas.2231254100
Wu, S. S., Platt, K. A., Ratnayake, C., Wang, T. W., Ting, J. T., and Huang, A. H. (1997). Isolation and characterization of neutral-lipid-containing organelles and globuli-filled plastids from Brassica napus tapetum. Proc. Natl. Acad. Sci. U. S. A. 94, 12711–12716. doi: 10.1073/pnas.94.23.12711
Xin, M., Wang, Y., Yao, Y., Song, N., Hu, Z., Qin, D., et al. (2011). Identification and characterization of wheat long non-protein coding RNAs responsive to powdery mildew infection and heat stress by using microarray analysis and SBS sequencing. BMC Plant Biol. 11:61. doi: 10.1186/1471-2229-11-61
Xu, M., Leichty, A. R., Hu, T., and Poethig, R. S. (2018). H2A.Z promotes the transcription of MIR156A and MIR156C in Arabidopsis by facilitating the deposition of H3K4me3. Development 145:dev152868. doi: 10.1242/dev.152868
Yamaguchi, N., Matsubara, S., Yoshimizu, K., Seki, M., Hamada, K., Kamitani, M., et al. (2021). H3K27me3 demethylases alter HSP22 and HSP17.6C expression in response to recurring heat in Arabidopsis. Nat. Commun. 12:3480. doi: 10.1038/s41467-021-23766-w
Yamakawa, H., Hirose, T., Kuroda, M., and Yamaguchi, T. (2007). Comprehensive expression profiling of rice grain filling-related genes under high temperature using DNA microarray. Plant Physiol. 144, 258–277. doi: 10.1104/pp.107.098665
Yang, K. A., Lim, C. J., Hong, J. K., Park, C. Y., Cheong, Y. H., Chung, W. S., et al. (2006). Identification of cell wall genes modified by a permissive high temperature in Chinese cabbage. Plant Sci. 171, 175–182. doi: 10.1016/j.plantsci.2006.03.013
Yu, X., Wang, H., Lu, Y., de Ruiter, M., Cariaso, M., Prins, M., et al. (2011). Identification of conserved and novel microRNAs that are responsive to heat stress in Brassica rapa. J. Exp. Bot. 63, 1025–1038. doi: 10.1093/jxb/err337
Yu, Y., Zhang, Y., Chen, X., and Chen, Y. (2019). Plant noncoding RNAs: hidden players in development and stress responses. Annu. Rev. Cell Dev. Biol. 35, 407–431. doi: 10.1146/annurev-cellbio-100818-125218
Zhang, D., Liu, D., Lv, X., Wang, Y., Xun, Z., Liu, Z., et al. (2014). The cysteine protease CEP1, a key executor involved in tapetal programmed cell death, regulates pollen development in Arabidopsis. Plant Cell 26, 2939–2961. doi: 10.1105/tpc.114.127282
Zhang, S.-S., Yang, H., Ding, L., Song, Z.-T., Ma, H., Chang, F., et al. (2017). Tissue-specific transcriptomics reveals an important role of the unfolded protein response in maintaining fertility upon heat stress in Arabidopsis. Plant Cell 29, 1007–1023. doi: 10.1105/tpc.16.00916
Zhao, D. (2009). Control of anther cell differentiation: a teamwork of receptor-like kinases. Sex. Plant Reprod. 22, 221–228. doi: 10.1007/s00497-009-0106-3
Zhao, D. Z., Wang, G. F., Speal, B., and Ma, H. (2002). The EXCESS MICROSPOROCYTES1 gene encodes a putative leucine-rich repeat receptor protein kinase that controls somatic and reproductive cell fates in the Arabidopsis anther. Genes Dev. 16, 2021–2031. doi: 10.1101/gad.997902
Zhao, Q., Zhou, L., Liu, J., Cao, Z., Du, X., Huang, F., et al. (2018). Involvement of CAT in the detoxification of HT-induced ROS burst in rice anther and its relation to pollen fertility. Plant Cell Rep. 37, 741–757. doi: 10.1007/s00299-018-2264-y
Keywords: heat stress, male reproduction, anther, tapetum, and pollen development, epigenetic regulation, DNA methylation
Citation: Malik S and Zhao D (2022) Epigenetic Regulation of Heat Stress in Plant Male Reproduction. Front. Plant Sci. 13:826473. doi: 10.3389/fpls.2022.826473
Edited by:
Seonghoe Jang, World Vegetable Center Korea Office, South KoreaReviewed by:
Biao Jin, Yangzhou University, ChinaRanjith Papareddy, University of California, Los Angeles, United States
Copyright © 2022 Malik and Zhao. This is an open-access article distributed under the terms of the Creative Commons Attribution License (CC BY). The use, distribution or reproduction in other forums is permitted, provided the original author(s) and the copyright owner(s) are credited and that the original publication in this journal is cited, in accordance with accepted academic practice. No use, distribution or reproduction is permitted which does not comply with these terms.
*Correspondence: Dazhong Zhao, ZHpoYW9AdXdtLmVkdQ==
†Present address: Shikha Malik, Department of Plant Pathology and Microbiology, Iowa State University, Ames, IA, United States