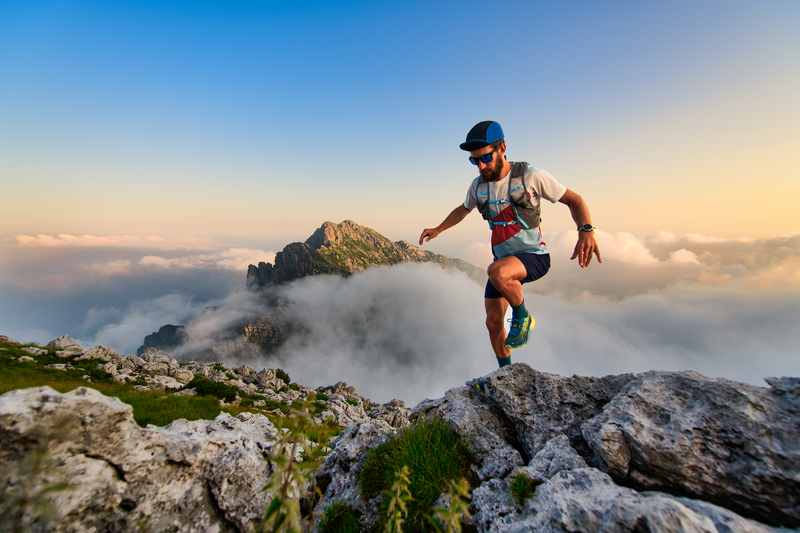
95% of researchers rate our articles as excellent or good
Learn more about the work of our research integrity team to safeguard the quality of each article we publish.
Find out more
ORIGINAL RESEARCH article
Front. Plant Sci. , 11 February 2022
Sec. Plant Development and EvoDevo
Volume 13 - 2022 | https://doi.org/10.3389/fpls.2022.817915
This article is part of the Research Topic XVII Spanish Portuguese Congress on Plant Biology (BP2021) - Plant Growth and Development View all 6 articles
Lipid remodeling of Glycosylphosphatidylinositol (GPI) anchors is required for their maturation and may influence the localization and function of GPI-anchored proteins (GPI-APs). Maturation of GPI-anchors is well characterized in animals and fungi but very little is known about this process in plants. In yeast, the GPI-lipid remodeling occurs entirely at the ER and is initiated by the remodeling enzyme Bst1p (Post-Glycosylphosphatidylinositol Attachment to Proteins inositol deacylase 1 -PGAP1- in mammals and Arabidopsis). Next, the remodeling enzyme Per1p (Post-Glycosylphosphatidylinositol Attachment to Proteins phospholipase 3 -PGAP3- in mammals) removes a short, unsaturated fatty acid of phosphatidylinositol (PI) that is replaced with a very long-chain saturated fatty acid or ceramide to complete lipid remodeling. In mammals, lipid remodeling starts at the ER and is completed at the Golgi apparatus. Studies of the Arabidopsis PGAP1 gene showed that the lipid remodeling of the GPI anchor is critical for the final localization of GPI-APs. Here we characterized loss-of-function mutants of Arabidopsis Per1/PGAP3 like genes (AtPGAP3A and AtPGAP3B). Our results suggest that PGAP3A function is required for the efficient transport of GPI-anchored proteins from the ER to the plasma membrane/cell wall. In addition, loss of function of PGAP3A increases susceptibility to salt and osmotic stresses that may be due to the altered localization of GPI-APs in this mutant. Furthermore, PGAP3B complements a yeast strain lacking PER1 gene suggesting that PGAP3B and Per1p are functional orthologs. Finally, subcellular localization studies suggest that PGAP3A and PGAP3B cycle between the ER and the Golgi apparatus.
GPI-anchored proteins (GPI-APs) are involved in diverse and crucial biological processes, including growth, morphogenesis, reproduction, and disease pathogenesis (Cheung et al., 2014). The GPI anchor is newly synthesized in the ER and is then attached to the protein (also synthesized in the ER) by a GPI transamidase (Desnoyer et al., 2020; Kinoshita, 2020). The nascent protein has a N-terminal secretory peptide and a C-terminal GPI-specifying hydrophobic signal sequence where the GPI anchor will be attached (Yeats et al., 2018). The structure of the GPI anchor is conserved in many eukaryotes and it has a common backbone with a glycan core structure and a lipid moiety composed of phosphatidylinositol (PI). Once the GPI anchor is transferred onto the protein at the ER, the glycan core and the lipid moiety need to be remodeled to the mature form of the GPI anchor which is present in the GPI-APs located at the plasma membrane. The mature GPI anchor structures differ between mammals and yeast. The PI form of mature yeast GPI anchors contains either diacylglycerol (DAG) with a very long chain saturated fatty acid (C26:0) at the sn-2 position or ceramide containing phytosphingosine with a very long chain (C26:0) fatty acid (Kinoshita and Fujita, 2016). In contrast, the major form of mammalian mature GPI anchors has 1-alkyl-2-acyl PI bearing a sn2-linked saturated fatty acid (usually stearic acid) (Kinoshita and Fujita, 2016). In plants, only a single GPI anchor structure has been resolved, the one of PcAGP1, isolated from Pyrus communis (pear) cell suspension culture. The lipid moiety of PcAGP1 consists of a ceramide, as has been detected in yeast (Oxley and Bacic, 1999). A ceramide was also detected as the lipid component of the GPI anchor of an arabinogalactan protein (AGP) isolated from Rosa sp. cell suspension culture (Svetek et al., 1999).
The lipid remodeling is a critical process for the transport and correct cellular localization of GPI-APs. In yeast, lipid remodeling of the GPI anchor occurs entirely at the ER (Figure 1; Pittet and Conzelmann, 2007). This route is initiated with the enzyme Bst1p, which carries out the deacylation at the two position of the inositol ring (Tanaka et al., 2004), making GPI-APs sensitive to bacterial phosphatidylinositol phospholipase C (PI-PLC). Next, the short and unsaturated fatty acid (C18:1) at the sn-2 position of PI is removed by the Per1p enzyme (Fujita et al., 2006a), and then it is replaced with a very long-chain saturated fatty acid (C26:0) by the membrane-bound O-acyltransferase Glycerol uptake 1 (Gup1p; Bosson et al., 2006). Only those GPI-APs destined to be released to the cell wall seem to maintain the C26:0 DAG generated. Indeed, GPI-APs destined to remain at the plasma membrane contain a ceramide moiety (instead of DAG) consisting of phytosphingosine with a C26 fatty acid. Calcofluor white-hypersensitive 43 (Cwh43p) is the enzyme in charge of adding the ceramide (Umemura et al., 2007; Yoko-o et al., 2018). Although the substrate for the ceramide substitution remains elusive, it seems that most lipid moieties of GPI anchors are exchanged from DAG to ceramide types (Ghugtyal et al., 2007).
Figure 1. Scheme of GPI-lipid remodelingin yeast and mammalian cells. The GPI anchor is synthesized in the ER and consists of a glycan core and phosphatidylinositol (PI) with an acyl chain at the two position of the inositol ring (Kinoshita and Fujita, 2016). After protein attachment, the glycan and lipid moieties are remodeled sequentially and this process has been shown to be very important for the transport and final localization of the GPI-APs. The GPI-lipid undergoes a structural remodeling that has the purpose of providing saturated lipids and it is initiated at the ER with the inositol deacylation at the two position of the inositol ring by the remodeling enzyme Bst1p (PGAP1 in mammals). Next, the short and unsaturated fatty acid at the sn-2 position of PI is removed by Per1p (PGAP3 in mammals). In yeast, lipid remodeling of the GPI anchor occurs at the ER. In contrast, in mammals lipid remodeling starts at the ER and is completed at the Golgi. The rest of lipid remodeling enzymes are also indicated and details provided in the text (modified from Muniz and Zurzolo, 2014). Only lipid remodeling enzymes are shown. IPC, inositolphosphoceramide.
Once GPI anchor remodeling is completed at the ER, GPI-APs with long-chain saturated fatty acids have different physical properties and associate to form membrane ordered domains at the ER lipid membrane (Silva et al., 2006), being selectively concentrated at specific ER export sites (ERES) different from those containing other secretory proteins (Muñiz and Riezman, 2016). As the GPI-APs are at the luminal side of the ER, they need a cargo receptor to be sorted within COPII vesicles. This function is carried out by the p24 protein complex (Castillon et al., 2011), which interacts with the remodeled glycan core of GPI-APs and incorporates them into nascent COPII vesicles to transport them to the Golgi apparatus. Once in the Golgi, GPI-APs dissociate from the p24 protein complex and continue their transport to reach their final destination, the plasma membrane or the cell wall.
In mammals, inositol deacylation is mediated by Post-Glycosylphosphatidylinositol Attachment to Proteins inositol deacylase 1 (PGAP1) at the ER (Figure 1; Tanaka et al., 2004). Then, an ethanolamine phosphate (EtNP) side branch linked to the glycan core is removed by an EtNP phosphodiesterase called PGAP5 (Fujita et al., 2009). After these two remodeling reactions, GPI-APs associate with the p24 complex to be transported to the Golgi where the lipid remodeling will continue. In contrast to yeast, mammalian p24 proteins are required not only for packaging GPI-APs into COPII-coated vesicles for ER-Golgi transport but also for concentrating them into the ERESs. This difference may reflect the fact that lipid remodeling of GPI-APs in mammals (which determine their final lipid composition), is not completed at the ER, which may prevent lipid-based sorting into ERES. Once at the Golgi, the unsaturated fatty acid at the sn-2 position of PI of mammalian GPI-APs is replaced by a saturated fatty acid, usually stearic acid. The removal of the unsaturated fatty acid is mediated by the functional ortholog of Per1p, the Golgi enzyme called PGAP3 (Figure 1; Maeda et al., 2007). PGAP3 and Per1p seem to be GPI specific phospholipases A2, although direct demonstration of having this enzyme activity has not been obtained (Pei et al., 2011). The Golgi-resident membrane protein PGAP2 is the enzyme required for the reacylation of the lysoPI with stearic acid (Tashima et al., 2006). Once the GPI anchor is correctly remodeled, some GPI-anchored proteins can also transiently homodimerize (Suzuki et al., 2012) and associate with membrane microdomains or lipid rafts (membrane domains rich in sphingolipids and sterols) (Brown and Rose, 1992; Simons and Gerl, 2010; Zurzolo and Simons, 2016), to be sorted to the apical plasma membrane in polarized cells (Paladino et al., 2006).
In Arabidopsis thaliana, around 300 proteins have been predicted to be GPI-APs and among them there are cell wall structural proteins, proteases, enzymes, receptor-like proteins (RLPs), and lipid transfer proteins. They play important roles in a variety of plant biological processes, including cell wall synthesis, polar cell expansion, stress and hormone signaling responses, stomatal development and pollen tube elongation (Yeats et al., 2018; Zhou, 2019). Complete disruption of GPI-anchor synthesis in Arabidopsis is lethal, as is the case in yeast and mammals (Lalanne et al., 2004; Gillmor et al., 2005; Dai et al., 2014; Bundy et al., 2016), indicating the vital role of these proteins. Disruption of GPI-anchor lipid remodeling catalyzed by Bst1/PGAP1 or Per1p/PGAP3 is not lethal, neither in yeast (Elrod-Erickson and Kaiser, 1996; Fujita et al., 2006a,b) nor in mammals (Ueda et al., 2007; Murakami et al., 2014; Williams et al., 2015; Kinoshita, 2020). Recently, we found that AtPGAP1 is an ER protein involved in deacylation of the inositol ring of GPI-APs in Arabidopsis and this process was shown to be important for the transport and final subcellular localization of GPI-APs. In this work, we have used a loss-of-function approach to initiate the study of the role of Arabidopsis orthologs of mammalian PGAP3 and yeast Per1p, the enzymes involved in the removal of the unsaturated fatty acid at the sn-2 position of the GPI-anchor of GPI-APs.
Nicotiana benthamiana plants were grown from surface-sterilized seeds on soil in the greenhouse at 24°C with 16 h daylength. A. thaliana plants were grown in growth chambers as previously described (Ortiz-Masia et al., 2007) and ecotype Col-0 was used as wild-type. Arabidopsis pgap3A T-DNA insertion mutants used in this study were obtained from the Nottingham Arabidopsis Stock Centre. The T-DNA insertion mutants were characterized by PCR (Supplementary Table 1). Due to the lack of PGAP3B T-DNA insertion mutants in mutant collections, artificial microRNA (amiRNA) was used to knock-down the expression of this gene. The PGAP3B amiRNA construct CSHL_013451 was purchased from Arabidopsis Biological Resource Center (ABRC)1. This construct contained an amiRNA (that we called amiR-PGAP3B) that is targeted to a sequence of the last exon of PGAP3B. After transformation with this construct, transgenic plants were selected by antibiotics and segregation of these lines were analyzed. T3 homozygous generation was used to characterize silencing by RT-PCR. Two independent homozygous lines, amiR-pgap3B-1 and amiR-pgap3B-2, that showed the best silencing for PGAP3B were selected. pgap3A-1 plants were transformed with the amiR-PGAP3B construct to generate amiR-pgap3Bpgap3A double mutants.
To study whether salt tolerance was affected in the AtPGAP3 mutants, seeds of wild-type (Col-0) and mutants were sown on Murashige and Skoog (MS) plates containing 160 mM NaCl. Plates were transferred to a controlled growth chamber after cold treatment in the dark for 3 days at 4°C. After 12 days, the rates of cotyledon greening were scored (Sánchez-Simarro et al., 2020). To study mannitol (300 mM) and MgCl2 (25 mM) tolerance the same protocol was used, but in the case of MgCl2, seedling survival was scored after 18 days.
Total RNA was extracted from seedlings by using a Qiagen RNeasy plant mini kit, and 3 μg of the RNA solution was reverse-transcribed using the maxima first-strand cDNA synthesis kit for quantitative RT-PCR (Fermentas®, Canada) according to the manufacturer’s instructions. Semi-quantitative PCRs (sqPCRs) were performed on 3 μl of cDNA template using Emerald Amp Max PCR Master Mix (Takara®, Japan). The sequences of the primers used for PCR amplifications are included in Supplementary Table 2.
The coding sequence of PGAP3A-RFP, GFP-PGAP3A, PGAP3B-RFP, GFP-PGAP3B, and GFP-PER1p were commercially synthesized de novo (Geneart AG®, Germany) based on the sequence of PGAP3A (AT5G62130.2), PGAP3B (AT1G16560.1), PER1 (YCR044C), RFP and GFP. For the N-terminal tagged constructs, the GFP cDNA was located after the predicted signal peptide sequence. As the representative model gene of PGAP3A, AT5G62130.2, does not include a signal peptide sequence, for the N-terminal GFP-PGAP3A construct, the AT5G62130.1 gene variant was chosen. All the coding sequences were cloned into pCHF3 (pro35S) (Ortiz-Masia et al., 2007). Constructs for yeast expression were obtained as follows: BamHI-SalI inserts containing either GFP or RFP-tagged PGAP3A and PGAP3B previously cloned into pCHF3 were subcloned as BamHI-SalI fragments into pYPGE15 yeast expression vector (Brunelli and Pall, 1993).
A pGreenII 0179 vector backbone (Hellens et al., 2000) was used for constructing V-FLA11 driven by pro35S as previously described (Bernat-Silvestre et al., 2021b). Other constructs used for transient expression experiments were: GFP-AGP4, GFP-GPI, MAP-GFP, and GFP-PAP (Martinière et al., 2012; Bernat-Silvestre et al., 2020, 2021b), GFP-PMA (Kim et al., 2001), PIP2A-RFP (Nelson et al., 2007), RFP-calnexin (Künzl et al., 2016), and GFP-CESA3 (Bernat-Silvestre et al., 2021b). Other constructs have been described previously: RFP−p24δ5 (Langhans et al., 2008; Montesinos et al., 2012), ManI-YFP and ManI-RFP (Nebenführ et al., 1999), ST-YFP (Boevink et al., 1998), GFP-HDEL (Pain et al., 2019), mCherry-HDEL (Nelson et al., 2007), OsSCAMP1-YFP (Lam et al., 2007), GFP-EMP12 (Gao et al., 2012), TIP1.1-GFP (Gattolin et al., 2011), and SPΔCt-mCherry (Pereira et al., 2013).
Wild-type yeast strain BY4742 and the isogenic per1 knock-out mutant were obtained from EUROSCARF with accession numbers Y10000 and Y15768, respectively. The received strains were grown in standard YPD medium. The mutant strain per1 was transformed with GFP or RFP-tagged PGAP3A and PGAP3B constructs in pYPGE15 and selected by URA3 selectable marker in synthetic SD medium supplemented with histidine, lysine and leucine following the lithium acetate method (Ito et al., 1983). Yeast culture conditions were as described previously (Ferrando et al., 1995). For the drop tests, stationary cultures grown for 2–3 days in either rich medium for the wild type and isogenic per1 mutant or in synthetic SD medium without uracil for the per1 transformants, were either directly spotted (5 μL) on the plates or serially diluted ×5 fold in the same medium prior to being spotted on the plates.
To obtain mesophyll protoplasts from Arabidopsis plants, the Tape-Arabidopsis Sandwich method was used, as described in Wu et al. (2009). Protoplasts were isolated from 4-week old rosette leaves. For transient expression, we used the PEG transformation method (Yoo et al., 2007). Transient expression of Arabidopsis seedlings by vacuum infiltration (Bernat-Silvestre et al., 2021a) and N. benthamiana leaves mediated by Agrobacterium tumefaciens (Lerich et al., 2011) were performed as described previously.
Nicotiana benthamiana leaves expressing XFP-Proteins were frozen in liquid N2 and then ground in homogenization buffer (HB, 0.3 M sucrose; 1 mM EDTA; 20 mM KCl; 20 mM HEPES pH 7.5), supplemented with 1 mM DTT and a Protease Inhibitor Cocktail (Sigma®, United States), using a mortar and a pestle. The homogenate was centrifuged for 10 min at 1,200 × g and 4°C, and the post nuclear supernatant (PNS) was collected and analyzed by SDS-PAGE and immunoblotting with GFP/RFP antibodies from Rockland Immunochemicals® (United States). For yeast protein extracts, culture cells were pelleted and resuspended in SDS-PAGE sample buffer. Immunoblots were developed using the SuperSignal West Pico chemiluminescent substrate (Pierce, Thermo Fisher Scientific®, United States) and analyzed using the ChemiDoc XRS+ imaging system (Bio-Rad®, United States)2. Immunoblots in the linear range of detection were quantified using Quantity One software (Bio-Rad Laboratories®).
Confocal fluorescent images were collected using an Olympus FV1000® confocal microscope with 60× oil lens. The GFP signal was visualized with laser excitation at 488 nm and emission at 496–518 nm. The YFP signal was visualized with laser excitation at 514 nm and emission at 539–561 nm. The mRFP/mCherry signal was visualized with laser excitation at 543 nm and emission at 593–636 nm. Sequential scanning was used to avoid any interference between fluorescence channels. Post-acquisition image processing was performed using the FV10-ASW 4.2 Viewer®and ImageJ® (v.1.45).
Differences in stress responses among pgap3A, pgap3B, and pgap3AB mutants compared to Col-0 (Wild-type) were tested using a two samples t-test with unequal variances using Microsoft Excel® 2013.
The lipid remodeling reaction that removes an unsaturated acyl chain at the sn-2 position of the PI moiety is mediated by mammalian PGAP3 and yeast Per1p (Figure 1). Both enzymes belong to the membrane bound hydrolase CREST (alkaline ceramidase, PAQR receptor, Per1, SID-1, and TMEM8) superfamily (Pei et al., 2011). Members of this superfamily share seven predicted core transmembrane segments and a set of conserved serine, histidine, and aspartate residues (Supplementary Figure 1). Two Arabidopsis genes, AT5G62130 and AT1G16560, have been assigned to belong to the Per1/PGAP3 family of fatty acid remodeling hydrolases for GPI-anchored proteins (Pei et al., 2011). They share 60% amino acid sequence identity and both conserve yeast histidines 177 and 326 that have been shown important for the putative function of Per1 proteins (Fujita et al., 2006a; Pei et al., 2011; Supplementary Figure 1). From now on, AT5G62130 and AT1G16560 will be referred as PGAP3A and PGAP3B, respectively. PGAP3A and PGAP3B are predicted to encode a 343-amino acid and 342-amino acid membrane protein, respectively, with an expected subcellular localization at the ER, Golgi apparatus or plasma membrane (Hofmann and Stoffel, 1993)3. Transmembrane topology prediction CCTOP (Dobson et al., 2015) suggests that both proteins have an amino-terminal secretory signal peptide and seven transmembrane domains, as occurs in other members of the Per1 family (Supplementary Figure 1). The cytosolic tail of both PGAP3A and PGAP3B contain a C-terminal dilysine motif which has been shown to be involved in the retrieval of proteins from post ER-membranes to the ER (Gao et al., 2014; Supplementary Figure 1).
To investigate the relative expression of PGAP3 genes, we used the publicly available RNAseq expression database GENEVESTIGATOR (Zimmermann et al., 2004; Hruz et al., 2008). As shown in Supplementary Figure 2, both genes show expression in most tissues throughout plant development with PGAP3B (AT1G16560) having higher mRNA transcript levels than PGAP3A (AT5G62130).
It has been previously described that per1 yeast cells showed increased heat and MgCl2 sensitivity (Paidhungat and Garrett, 1998; Fujita et al., 2006a). To determine if PGAP3A and PGAP3B are functional orthologs of PER1, we introduced plasmids encoding N-terminal GFP or C-terminal RFP tagged PGAP3A and PGAP3B into yeast per1 cells. Tagged proteins of the expected molecular weight were detected in yeast (Supplementary Figure 3A). We examined the sensitivities of the yeast per1 mutant and the complemented lines to 0.4 M MgCl2 and high temperature compared to wild-type cells. We found that both the N-terminal as well as the C-terminal PGAP3B constructs restored MgCl2 and high temperature tolerance of per1 cells to wild-type levels, as it was the case for GFP-Per1p (Figure 2). In contrast, per1 lines complemented with PGAP3A constructs remained sensitive to MgCl2 and high temperature. In addition, the per1 yeast cells were also shown to have a mild phenotype in the presence of 1 M NaCl that could be restored by PGAP3B but not by PGAP3A constructs (Supplementary Figure 3C).
Figure 2. Complementation analysis of the yeast per1 mutant by Arabidopsis PGAP3A/B. Wild-type and per1 (Y15768) mutant strains were grown in YPD medium for 2 days and spotted (upper part) on the indicated YPD medium and temperatures. In parallel, three independent transformants of per1 (lower part) with each of the tagged PGAP3A/B complementation constructs and GFP-Per1 and empty vector as controls (as shown on the left) were grown for 2 days in synthetic medium supplemented with the required amino acids and spotted on the same plates and temperature as indicated. Growth was scored after 2 days. The GFP-Per1 and both PGAP3B constructs were able to recover heat and MgCl2 sensitivity of the per1 mutant whereas PGAP3A constructs were not.
As described in the section “Introduction,” yeast Per1p has been proposed to localize at the ER. However, the mammalian ortholog of Per1p, PGAP3, mainly localizes at the Golgi with a minor ER localization (Maeda et al., 2007; Howard et al., 2014). This is consistent with the fact that mammalian GPI-APs are segregated and sorted at the Golgi apparatus (where the lipid remodeling is completed). Therefore, we sought to investigate subcellular localization of the two isoforms of Arabidopsis PGAP3. In order to localize PGAP3A-B in vivo, PGAP3A and PGAP3B constructs, with N- or C-terminal GFP and RFP, respectively, were used for transient expression in Nicotiana benthamiana leaves. Protein extracts were analyzed by SDS-PAGE and Western Blot with GFP and RFP antibodies to confirm that proteins of the expected size were present (Supplementary Figure 3B). As shown in Figure 3, both GFP-PGAP3A and GFP-PGAP3B showed an ER-like localization pattern and extensively colocalized with the ER markers mCherry-HDEL and RFP-p254δ5. Occasionally, GFP-PGAP3B was also found in punctate structures which partially colocalized with the Golgi marker ManI-RFP (Figures 3L,O,R). When RFP was placed at the C-terminus of both proteins, we observed a shift in the localization of PGAP3A-RFP and PGAP3B-RFP. Both proteins showed a punctate pattern and extensively colocalized with the Golgi markers ManI-YFP and ST-YFP, although some ER localization was also detected (Figure 4). Since PGAP3A and PGAP3B both contain a canonical ER retrieval/retention signal at their C-terminus (KKxx in PGAP3A, KxKxx in PGAP3B) (Supplementary Figure 1), the shift in the localization of the C-terminal tagged proteins may be caused by masking of their ER retrieval/retention signals. These results suggest that, irrespective to their steady-state localization, both PGAP3A and PGAP3B may cycle between ER and Golgi.
Figure 3. Localization of GFP-PGAP3A and GFP-PGAP3B. Transient expression in N. benthamiana leaves of GFP-PGAP3A (B,E,H) and GFP-PGAP3B (K,N,Q) together with mCherry-HDEL (luminal ER marker) (A,J), RFP-p24δ5 (membrane ER marker) (D,M), and ManI-RFP (G,P) (Golgi marker) [see merged images in (C,F,I,L,O,R)]. Scale bars = 10 μm.
Figure 4. Localization of PGAP3A-RFP and PGAP3B-RFP. Transient expression in N. benthamiana leaves of PGAP3A-RFP (B,E,H) and PGAP3B-RFP (K,N,Q) together with GFP-HDEL (luminal ER marker) (A,J), ManI-YFP (D,M), and ST-YFP (G,P) (Golgi markers) [see merged images in (C,F,I,L,O,R)]. Scale bars = 10 μm.
Arabidopsis T-DNA insertion mutants were characterized to further study PGAP3A and PGAP3B function. Two PGAP3A T-DNA insertion mutants from the SALK collection4, pgap3A-1 (SALK_039375), and pgap3A-2 (SALK_069053), were characterized (Figure 5 and Supplementary Figure 4). The mRNA levels of PGAP3 in pgap3A-1 were less than 10% of wild-type levels and no PGAP3 mRNA could be detected in pgap3A-2 by RT-PCR analysis (Figure 5). These results indicate that pgap3A-1 and pgap3A-2 are knock-down and knock-out mutants, respectively. Due to the lack of PGAP3B T-DNA insertion mutants in mutant collections, an artificial microRNA (amiR-PGAP3B) was used to knock-down the expression of this gene (Supplementary Figure 4). A. thaliana transgenic lines were generated by transformation with amiR-PGAP3B. Independent lines were selected and the T3 homozygous generation was used to characterize silencing by RT-PCR as above. Two independent homozygous lines, amiR-pgap3B-1 and amiR-pgap3B-2, that showed the best silencing for PGAP3B (around 20% wild-type mRNA levels) were selected (Figure 5) and from now on, they will be referred as pgap3B-1 and pgap3B-2, respectively. pgap3A-1 plants were transformed with the amiR-PGAP3B construct to generate an amiR-pgap3Bpgap3A double mutant. Independent transgenic lines were selected and the T3 homozygous generation was used to characterize silencing by RT-PCR as above. Two independent homozygous lines, amiR-pgap3Bpgap3A-1 and amiR-pgap3Bpgap3A-2, that showed the best silencing for PGAP3B (less than 70 and 40% of mRNA levels, respectively) were selected (Figure 5) and from now on, they will be referred as pgap3AB-1 and pgap3AB-2, respectively.
Figure 5. Characterization of pgap3 mutants. (A,B) sqPCR analysis of PGAP3A expression in pgap3A mutants (A) and PGAP3B expression in pgap3B and pgap3AB mutants (B). Total RNA from pgap3A-1, pgap3A-2, pgap3B-1, pgap3B-2, pgap3AB-1, pgap3AB-2, and wild-type (Col-0) 4 day-old seedlings were used for the PCRs. In the PCRs, PGAP3A and PGAP3B specific primers were used (Supplementary Tables 1, 2). Actin-7 (ACT7) was used as a control. PCR samples were collected at cycle 22 for ACT7, at cycle 36 for PGAP3A and at cycle 25 for PGAP3B. No wild-type band was detected in pgap3A-2. Quantification of the bands (n = 3) are shown. Values were normalized against the PGAP3A/PGAP3B fragment band intensity in wild-type that was considered to be 100%. Error bars represent SEM. (C,D) Phenotype of pgap3A, pgap3B, and pgap3AB mutants exposed to salt (NaCl) (C) and mannitol (D). Wild type (Col-0), pgap3A-1, pgap3A-2, pgap3B-1, pgap3B-2, pgap3AB-1, and pgap3AB-2 mutants were grown on 0.5 × MS as a control and 0.5 × MS supplemented with 160 mM NaCl or 300 mM mannitol for 12 days. The percentage of seedlings with green cotyledons and seedlings with true leaves was calculated for NaCl and mannitol experiments, respectively. Data shown as the mean ± SEM of five independent experiments (n = 20). Statistical significance: ns, not significant; *p < 0.05; **p < 0.01; ***p < 0.001.
None of the single mutants of PGAP3A, PGAP3B nor the double mutants of PGAP3AB showed any obvious phenotypic alteration under standard growth conditions when compared to wild-type plants (Supplementary Figure 4). However, we found that pgap3A-1 and pgap3A-2 showed enhanced sensitivity to 160 mM NaCl and 300 mM mannitol. The same sensitivity was observed in pgap3AB double mutants (Figures 5C,D). Interestingly, pgap3A and pgap3AB mutants were also more sensitive than wild-type to 25 mM MgCl2 (Supplementary Figure 4F) as yeast per1 cells. In general, smaller differences were detected between pgap3B mutants and wild-type in all the sensitivities tested (Figure 5 and Supplementary Figure 4).
Lipid remodeling enzyme function has been shown to be important for the efficient transport from the ER to the plasma membrane of yeast, mammalian and Arabidopsis GPI-anchored proteins (Tanaka et al., 2004; Bernat-Silvestre et al., 2021b). For that reason, we analyzed the localization of two GPI-anchored proteins in pgap3 mutants. One of them was GFP fused to arabinogalactan protein 4 (AGP4), a GPI-AP proteoglycan that seems to be involved in diverse developmental processes (Ellis et al., 2010; Pereira et al., 2016). This protein was shown previously to localize to the plasma membrane/apoplast (Martinière et al., 2012; Bernat-Silvestre et al., 2020, 2021b). The second one was Venus fused to FLA11 (V-FLA11), a member of fasciclin-like arabinogalactan proteins (FLAs) that have been related to cell adhesion (Johnson, 2003; MacMillan et al., 2010). In addition, we also used a glycosylphosphatidylinositol-anchored GFP (GFP-GPI; Martinière et al., 2012; Bernat-Silvestre et al., 2020, 2021b). As a control, we used a transmembrane plasma membrane protein, the aquaporin PIP2A-RFP (Nelson et al., 2007).
We first analyzed the localization of these proteins by transient expression in Arabidopsis seedlings (Figure 6; Bernat-Silvestre et al., 2021a). GFP-AGP4, V-FLA11, and GFP-GPI were localized to the plasma membrane/cell wall of cotyledon cells of wild-type Arabidopsis seedlings, as it was the case for the transmembrane plasma membrane protein PIP2A-RFP, as shown previously (Bernat-Silvestre et al., 2020, 2021b). In clear contrast, GFP-AGP4 and V-FLA11 showed a predominant ER-like localization pattern, together with a punctate pattern (presumably a Golgi pattern) in the two pgap3A mutants and in the two double pgap3AB mutants (Figure 6). This was not the case in the pgap3B mutants, where both proteins mainly localized to the plasma membrane/cell wall. Interestingly, GFP-GPI localized to the plasma membrane in all mutants, as did the transmembrane protein PIP2A-RFP (Figure 6). This suggests that PGAP3A enzyme is involved in the transport to the plasma membrane of GFP-AGP4 and V-FLA11, and that loss of PGAP3A function does not affect transport from the ER to the plasma membrane of GFP-GPI and the transmembrane protein PIP2A-RFP. The defect in transport of GFP-AGP4 and V-FLA11 in pgap3A mutants was not due to an alteration in the compartments of the secretory pathway, since no obvious defects were observed in the localization pattern of several organelle marker proteins, including GFP-HDEL (ER), GFP-EMP12 (Golgi apparatus), TIP1.1-GFP (tonoplast), SPΔCt-mCherry (vacuole lumen), SCAMP1-YFP (plasma membrane), and GFP-CESA3 (TGN/plasma membrane) (Supplementary Figure 5).
Figure 6. Localization of GFP-AGP4, V-FLA11, GFP-GPI, and PIP2A-RFP in wild-type, pgap3A, pgap3B, and pgap3AB Arabidopsis seedlings. Transient expression of GPI-anchored proteins and a plasma membrane marker in seedlings of wild-type (Col-0) (A–D) or pgap3A-1 (E–H), pgap3A-2 (I–L), pgap3B-1 (M–P), pgap3B-2 (Q–T), pgap3AB-1 (U–X), and pgap3AB-2 (Y–B’) mutants. The three GPI-anchored proteins, GFP-AGP4, V-FLA11, and GFP-GPI, mainly localized to the plasma membrane in cotyledon cells from wild-type (Col-0), pgap3B-1, and pgap3B-2 mutant seedlings, as the transmembrane protein PIP2A-RFP. In the pgap3A-1, pgap3A-2, pgap3AB-1 and pgap3AB-2 mutants, GFP-AGP4 and V-FLA11 showed a predominant ER localization pattern as well as a punctate pattern, probably corresponding to Golgi structures, in contrast to GFP-GPI and PIP2A-RFP, which mainly localized to the plasma membrane. Scale bars = 10 μm.
The localization of GFP-AGP4 and V-FLA11 in pgap3A mutants was confirmed by colocalization experiments. As shown in Figure 7, both GFP-AGP4 and V-FLA11 strongly colocalized with two different ER marker proteins, an ER luminal protein (mCherry-HDEL) and an ER membrane protein (RFP-p24δ5). In addition, GFP-AGP4 and V-FLA11 were also partially found in punctate structures which colocalized with the Golgi marker ManI-RFP, suggesting that these GPI-anchored proteins also localized to the Golgi apparatus in pgap3A mutants.
Figure 7. Colocalization of GPI-APs with ER and Golgi markers in pgap3A-2 seedlings. Transient expression in Arabidopsis seedlings. (A–F) Coexpression of GFP-AGP4 (A) and V-FLA11 (D) with the ER marker mCherry-HDEL (B,E) [see merged images in (C,F)]. (G–L) Coexpression of GFP-AGP4 (G) and V-FLA11 (J) with the ER marker RFP-p24δ5 (H,K) [see merged images in (I,L)]. (M–R) Coexpression of GFP-AGP4 (M) and V-FLA11 (P) with the Golgi marker ManI-RFP (N,Q) [see merged images in (O,R)]. Scale bars = 10 μm. GFP-AGP4 and V-FLA11 colocalized with ER marker proteins and they were also found in punctate structures which colocalized with the Golgi marker.
To confirm these ER/Golgi patterns, we also analyzed the localization of GFP-AGP4 and GFP-GPI by an alternative transient expression system, Arabidopsis protoplasts. In protoplasts from wild-type Arabidopsis plants, GFP-AGP4 and GFP-GPI localized to the plasma membrane, as we have shown previously (Bernat-Silvestre et al., 2020, 2021b). However, as it happened in transient expression in Arabidopsis seedlings, GFP-AGP4 also showed an ER/Golgi localization pattern in protoplasts from the pgap3A-1 and pgap3AB-2 mutants, but not in pgap3B mutants (Supplementary Figure 6) while GFP-GPI localized to the plasma membrane in all pgap3 mutants (Supplementary Figure 6). To corroborate the ER/Golgi patterns of GFP-AGP4 in these mutants, we co-expressed GFP-AGP4 with two different ER-membrane markers (RFP-calnexin and RFP-p24δ5) and a Golgi marker (ManI-RFP). As showed in Supplementary Figure 7, these markers extensively colocalized with GFP-AGP4 in pgap3AB-2 protoplasts, confirming the same ER/Golgi pattern showed in seedlings. Additionally, we could also detect the presence of both GFP-AGP4 and GFP-GPI at the plasma membrane, as shown by colocalization with FM (Fei Mao) styryl dye FM4-64, a lipid probe routinely used to label the plasma membrane (Supplementary Figure 7). This suggests that a fraction of GFP-AGP4 can reach the plasma membrane in pgap3AB mutants.
To test if the lack of PGAP3 enzymes affects the localization of other plasma membrane proteins different from GPI-APs, we used plasma membrane markers without a GPI anchor, including a myristoylated and palmitoylated GFP (MAP-GFP), a prenylated GFP (GFP-PAP) (Martinière et al., 2012) and a transmembrane protein, a GFP fusion with the plasma membrane ATPase (GFP-PMA; Kim et al., 2001). As shown in Supplementary Figure 8, these three proteins mainly localized to the plasma membrane in pgap3A-1, pgap3B-2 and pgap3AB-2 protoplasts, as in protoplasts from wild-type Arabidopsis plants, suggesting that the transport of other plasma membrane proteins is not affected in these mutants.
Since GFP-AGP4 partially localized to the plasma membrane in pgap3A mutants, we postulated that loss of PGAP3A may cause a delay (rather than a block) in its transport to the plasma membrane. Indeed, by inhibiting protein synthesis with cycloheximide, we have previously shown that loss of function of PGAP1 caused a delay in the transport of GFP-AGP4 from the ER to the cell surface with a progressive relocalization of GFP-AGP4 from the ER to the cell surface and ER labeling being almost undetectable after 6 h (Bernat-Silvestre et al., 2021b). To show if GFP-AGP4 was also able to reach the cell surface in pgap3A over time, the localization of GFP-AGP4 was analyzed after inhibition of protein synthesis. Treatment of pgap3A seedlings with 20 μM cycloheximide caused a progressive relocalization of GFP-AGP4 from the ER/Golgi to the cell surface, faster than that observed in pgap1 seedlings, with ER labeling being almost undetectable after 2 h (Figure 8). This indicates that GFP-AGP4 can reach the cell surface in the absence of PGAP3A but with a delayed kinetics and suggests that PGAP3A is involved in efficient transport of GPI-APs from the ER to the cell surface.
Figure 8. Localization of GFP-AGP4 in wild-type and pgap3A Arabidopsis seedlings treated with cycloheximide (CHX). (A–J) Transient expression of GFP-AGP4 in Arabidopsis seedlings of wild-type (Col-0) (A,B), pgap3A-1 (C,E,G,I), and pgap3A-2 (D,F,H,J). Seedlings were incubated in the presence of 20 μM cycloheximide or DMSO (control) for 2 h and analyzed by confocal laser scanning microscopy (CLSM). Scale bars = 10 μM. Note that transport of GFP-AGP4 is delayed in pgap3A-1 and pgap3A-2 mutants and GFP-AGP4 can reach the plasma membrane after 2 h in the presence of CHX.
Up to now, only one plant GPI anchor structure has been resolved, the one of PcAGP1, isolated from Pyrus communis (pear) cell suspension cultures (Oxley and Bacic, 1999). From this structure, it seems that the core structure of GPI anchors is conserved in plant and non-plant eukaryotes. In addition, a survey of the Arabidopsis genome indicates that most of the genes involved in particular steps of GPI anchor assembly and their remodeling have orthologs in Arabidopsis (Luschnig and Seifert, 2011). However, it has to be established whether Arabidopsis orthologs are functional and whether their function is conserved. Null Arabidopsis mutants involved in the biosynthesis and attachment of the GPI anchor showed either gametophytic or embryogenic lethality, indicating that GPI-APs are essential for growth and development in Arabidopsis (Lalanne et al., 2004; Gillmor et al., 2005; Dai et al., 2014; Bundy et al., 2016). Recently, we reported for the first time the characterization of AtPGAP1, an Arabidopsis gene involved in lipid remodeling of the GPI anchor (Bernat-Silvestre et al., 2021b). We found that PGAP1 localizes to the ER and likely functions as the GPI inositol-deacylase that cleaves the acyl chain from the inositol ring of the GPI anchor. Loss of PGAP1 function produced a delayed transport of GPI-APs through the secretory pathway, suggesting that PGAP1 is required for efficient ER export and transport to the cell surface of GPI-APs.
In this study, we have initiated the characterization of Arabidopsis PGAP3A and PGAP3B, orthologs of yeast PER1 and mammalian PGAP3, which have been proposed to function in the removal of the unsaturated fatty acid at the sn-2 position of the GPI-anchor of GPI-APs, although direct evidence of their hydrolase activity is lacking (Pei et al., 2011). AtPGAP3A and AtPGAP3B, together with Per1p and PGAP3, belong to the Per1 family (Pei et al., 2011). PGAP3B fusion proteins were able to rescue heat and salt sensitivity phenotypes of per1 yeast cells, indicating that PGAP3B may be functionally equivalent to yeast Per1p. This was not the case of PGAP3A. It is possible that XFP-tagged PGAP3A is not active in yeast (due to a defect in a posttranslational modification or to different splicing isoforms involved) although it cannot be discarded that PGAP3A plays a distinct and/or plant specific role.
No obvious phenotypic differences were observed between pgap3 mutants and wild-type plants under standard growth conditions. Nevertheless, pgap3A mutants showed enhanced sensitivity to NaCl, MgCl2, and mannitol. This may be due to defects in the localization/concentration of GPI-APs in membrane domains. In MDCK cells, correct lipid remodeling is necessary for proper oligomerization and concentration of GPI-APs in raft microdomains, essential for their transport to the apical or basolateral membranes (Paladino et al., 2004, 2006). Many GPI-APs are signal receptors that function during the response of cells to the extracellular environment (Yeats et al., 2018; Zhou, 2019). Thus, GPI anchor remodeling defects in plants are expected to produce an altered cellular response to salt stress as it has been observed in yeast (Paidhungat and Garrett, 1998; Fujita et al., 2006a). Although PGAP3A did not rescue the salt phenotypes of yeast per1, Arabidopsis pgap3A mutants also show salt sensitivity. In contrast, pgap3B mutants did not show any significant sensitivity to salt and a lower level of sensitivity to mannitol stress than pgap3A mutants. Transcript levels of PGAP3B are higher than those of PGAP3A and are reduced to around 20% of wild-type levels in pgap3B mutants. Therefore, the mild phenotypes observed in response to stress in pgap3B lines and the lack of an effect on GPI-AP trafficking, suggest that there is enough residual PGAP3 activity in those lines.
In yeast, GPI-AP trafficking was altered in per1 mutant cells (Fujita et al., 2006a). GPI-APs accumulated at the ER due to inefficient exit from the ER and levels of cell surface GPI-APs (lacking GPI anchor remodeling) were affected. The trafficking of some GPI-APs to the cell surface was also altered in pgap3A mutants. Similar to per1 yeast cells, pgap3A mutants showed a delay in the transport of GPI-APs. In mammalian cells, a defect of PGAP3 also results in unremodeled GPI-APs at the cell surface and depending on the proteins, cell types and species, can also affect transport to the cell surface (Maeda et al., 2007, 2017; Kinoshita and Fujita, 2016). Similarly, trafficking of GFP-AGP4 and V-FLA11 was altered in pgap3A whereas GFP-GPI was not, suggesting that trafficking of different GPI-APs may be altered to varying degrees depending on the type of protein and the context.
The N-terminally GFP-tagged PGAP3A and B versions mostly localized at the ER. In contrast, the C-terminally mRFP-tagged version localized mostly in the Golgi. This difference could be explained by the presence of a putative dilysine ER retrieval/retention signal at the C-terminal end of both PGAP3A and B that may be masked by the C-terminal RFP tag. The dilysine signals are known to bind to COPI coat proteins and mediate retrieval of proteins from post-ER compartments to the ER by COPI vesicles (Cosson and Letourneur, 1994; Jackson et al., 2012; Gao et al., 2014). It cannot be ruled out that PGAP3 proteins contain additional sorting signals. In addition, putative sorting signals might be altered by post-translational modifications and/or oligomerization, as it happens with the addition of XFP tags. Therefore, it is difficult to predict the real steady-state localization of PGAP3A and PGAP3B, although the results presented here clearly indicate that they may cycle between the ER and the Golgi apparatus (Supplementary Figure 9). This may explain the delay in transport to the plasma membrane/cell wall of GFP-AGP4 and V-FLA11 in pgap3A mutants. Indeed, PGAP3A and PGAP3B localization correlates with GFP-AGP4 and V-FLA11 ER/Golgi patterns observed in transient expression experiments of pgap3A mutants.
Arabidopsis contains two PGAP3 isoforms, in contrast to yeast and mammals that contain only one isoform. pgap3A did not show any growth alterations under standard growth conditions but it is more sensitive to different stress conditions. In addition, the mutant showed a delay in the trafficking of GPI-APs to the cell surface. On the other hand, PGAP3B, but not PGAP3A, was able to complement the yeast per1 mutant. This raises the possibility that PGAP3A and PGAP3B may have different specificities and have not completely redundant functions. In the future, generation of different CRISPR pgap3B mutant lines, for example with substitutions/deletion of putative amino acids of the active site, will assist efforts to understand if the roles of these two proteins are distinct or overlapping. It is intriguing that no PGAP2 gene has been identified yet in Arabidopsis (Luschnig and Seifert, 2011). PGAP2, which acts after PGAP3, is involved in GPI anchor reacylation in mammals and there is evidence that PGAP2 and PGAP3 may form a complex. Nevertheless, as two or more PGAP3 isoforms have been identified in most plant species (Supplementary Table 3) (Thomas et al., 2021), it is exciting to think that these isoforms may reflect differences in plant GPI anchors. To address a major gap in knowledge of key importance, it would be essential to solve other plant GPI-AP structures and gain better understanding of plant GPI biology.
The original contributions presented in the study are included in the article/Supplementary Material, further inquiries can be directed to the corresponding authors.
MM, FA, KJ, and AF: conceptualization. CB-S, YM, AF, MM, and FA: investigation. MM and FA: writing – original draft, supervision, project administration, and funding acquisition. MM, FA, KJ, AF, and CB-S: writing – review and editing. All authors contributed to the article and approved the submitted version.
FA and MM were supported by the Ministerio de Economía y Competitividad (grant no. BFU2016-76607P), Ministerio de Ciencia e Innovación, MICINN/AEI/10.13039/501100011033 (grant no. PID2020-113847GB-100), and Generalitat Valenciana (AICO/2020/187). CB-S was recipient of a fellowship from Ministerio de Ciencia, Innovación y Universidades (FPU program). CB-S was also recipient of an EMBO short-term fellowship and a short-term fellowship from Ministerio de Ciencia, Innovación y Universidades.
The authors declare that the research was conducted in the absence of any commercial or financial relationships that could be construed as a potential conflict of interest.
All claims expressed in this article are solely those of the authors and do not necessarily represent those of their affiliated organizations, or those of the publisher, the editors and the reviewers. Any product that may be evaluated in this article, or claim that may be made by its manufacturer, is not guaranteed or endorsed by the publisher.
We thank John Runions for the GFP-AGP4, GFP-GPI, MAP-GFP, and GFP-PAP constructs, Inhwan Hwang for the GFP-PMA construct, Liwen Jiang for the GFP-EMP12 and OsSCAMP1-YFP constructs, Lorenzo Frigerio for the TIP1.1-GFP construct, Claudia Pereira for the SPΔCt-mCherry construct, Morihisa Fujita for his help with rescue experiments with per1 yeast cells, and Judit Sanchez-Simarro and Javier Montero-Pau for helpful discussions. We also thank the Salk Institute Genomic Analysis Laboratory for providing the sequence-indexed Arabidopsis T-DNA insertion mutants and the greenhouse, genomic and microscopy sections of SCSIE (Serveis Centrals de Suport a la Investigació Experimental, University of Valencia).
The Supplementary Material for this article can be found online at: https://www.frontiersin.org/articles/10.3389/fpls.2022.817915/full#supplementary-material
Bernat-Silvestre, C., Sánchez-Simarro, J., Ma, Y., Montero-Pau, J., Johnson, K., Aniento, F., et al. (2021b). AtPGAP1 functions as a GPI inositol-deacylase required for efficient transport of GPI-anchored proteins. Plant Physiol. 187, 2156–2173. doi: 10.1093/plphys/kiab384
Bernat-Silvestre, C., De Sousa Vieira, V., Sánchez-Simarro, J., Aniento, F., and Marcote, M. J. (2021a). “Transient transformation of A. thaliana seedlings by vacuum infiltration,” in Arabidopsis Protocols: Methods in Molecular Biology, eds J. J. Sanchez-Serrano and J. Salinas (New York, NY: Humana Press Inc), 147–155. doi: 10.1007/978-1-0716-0880-7_6
Bernat-Silvestre, C., Vieira, V. D. S., Sanchez-Simarro, J., Pastor-Cantizano, N., Hawes, C., Marcote, M. J., et al. (2020). P24 Family proteins are involved in transport to the plasma membrane of GPI-anchored proteins in plants. Plant Physiol. 184, 1333–1347. doi: 10.1104/pp.20.00880
Boevink, P., Oparka, K., Cruz, S. S., Martin, B., Betteridge, A., and Hawes, C. (1998). Stacks on tracks: the plant Golgi apparatus traffics on an actin/ER network. Plant J. 15, 441–447. doi: 10.1046/j.1365-313X.1998.00208.x
Bosson, R., Jaquenoud, M., and Conzelmann, A. (2006). GUP1 of Saccharomyces cerevisiae encodes an O-acyltransferase involved in remodeling of the GPI anchor. Mol. Biol. Cell 17, 2636–2645. doi: 10.1091/mbc.e06-02-0104
Brown, D. A., and Rose, J. K. (1992). Sorting of GPI-anchored proteins to glycolipid-enriched membrane subdomains during transport to the apical cell surface. Cell 68, 533–544. doi: 10.1016/0092-8674(92)90189-J
Brunelli, J. P., and Pall, M. L. (1993). A series of yeast/Escherichia coli λ expression vectors designed for directional cloning of cDNAs and cre/lox-mediated plasmid excision. Yeast 9, 1309–1318. doi: 10.1002/yea.320091204
Bundy, M. G. R., Kosentka, P. Z., Willet, A. H., Zhang, L., Miller, E., and Shpak, E. D. (2016). A mutation in the catalytic subunit of the glycosylphosphatidylinositol transamidase disrupts growth, fertility, and stomata formation. Plant Physiol. 171, 974–985. doi: 10.1104/pp.16.00339
Castillon, G. A., Aguilera-Romero, A., Manzano-Lopez, J., Epstein, S., Kajiwara, K., Funato, K., et al. (2011). The yeast p24 complex regulates GPI-anchored protein transport and quality control by monitoring anchor remodeling. Mol. Biol. Cell 22, 2924–2936. doi: 10.1091/mbc.e11-04-0294
Cheung, A. Y., Li, C., Zou, Y. J., and Wu, H. M. (2014). Glycosylphosphatidylinositol anchoring: control through modification. Plant Physiol. 166, 748–750. doi: 10.1104/pp.114.246926
Cosson, P., and Letourneur, F. (1994). Coatomer interaction with Di-Lysine endoplasmic reticulum retention motifs. Science 263, 1629–1631. doi: 10.1126/science.8128252
Dai, X. R., Gao, X.-Q., Chen, G. H., Tang, L. L., Wang, H., and Zhang, X. S. (2014). Abnormal pollen tube guidance1, an endoplasmic reticulum-localized mannosyltransferase homolog of glycosylphosphatidylinositol10 in yeast and phosphatidylinositol glycan anchor biosynthesis b in human, is required for Arabidopsis pollen tube micropylar gu. Plant Physiol. 165, 1544–1556. doi: 10.1104/pp.114.236133
Desnoyer, N., Howard, G., Jong, E., and Palanivelu, R. (2020). AtPIG-S, a predicted glycosylphosphatidylinositol transamidase subunit, is critical for pollen tube growth in Arabidopsis. BMC Plant Biol. 20:380. doi: 10.1186/s12870-020-02587-x
Dobson, L., Reményi, I., and Tusnády, G. E. (2015). CCTOP: a consensus constrained topology prediction web server. Nucleic Acids Res. 43, W408–W412. doi: 10.1093/nar/gkv451
Ellis, M., Egelund, J., Schultz, C. J., and Bacic, A. (2010). Arabinogalactan-proteins: key regulators at the cell surface? Plant Physiol. 153, 403–419. doi: 10.1104/pp.110.156000
Elrod-Erickson, M. J., and Kaiser, C. A. (1996). Genes that control the fidelity of endoplasmic reticulum to Golgi transport identified as suppressors of vesicle budding mutations. Mol. Biol. Cell 7, 1043–1058. doi: 10.1091/mbc.7.7.1043
Ferrando, A., Kron, S. J., Rios, G., Fink, G. R., and Serrano, R. (1995). Regulation of cation transport in Saccharomyces cerevisiae by the salt tolerance gene HAL3. Mol. Cell Biol. 15, 5470–5481. doi: 10.1128/mcb.15.10.5470
Fujita, M., Maeda, Y., Ra, M., Yamaguchi, Y., Taguchi, R., and Kinoshita, T. (2009). GPI glycan remodeling by pgap5 regulates transport of GPI-anchored proteins from the ER to the golgi. Cell 139, 352–365. doi: 10.1016/j.cell.2009.08.040
Fujita, M., Umemura, M., Yoko-o, T., and Jigami, Y. (2006a). PER1 is required for GPI-phospholipase A2 activity and involved in lipid remodeling of GPI-anchored proteins. Mol. Biol. Cell 17, 5253–5264. doi: 10.1091/mbc.e06-08-0715
Fujita, M., Yoko, O. T., and Jigami, Y. (2006b). Inositol deacylation by Bst1p is required for the quality control of glycosylphosphatidylinositol-anchored proteins. Mol. Biol. Cell 17, 834–850. doi: 10.1091/mbc.E05-05-0443
Gao, C., Cai, Y., Wang, Y., Kang, B. H., Aniento, F., Robinson, D. G., et al. (2014). Retention mechanisms for ER and Golgi membrane proteins. Trends Plant Sci. 19, 508–515. doi: 10.1016/j.tplants.2014.04.004
Gao, C., Yu, C. K. Y., Qu, S., San, M. W. Y., Li, K. Y., Lo, S. W., et al. (2012). The golgi-localized Arabidopsis endomembrane protein12 contains both endoplasmic reticulum export and golgi retention signals at its c terminus. Plant Cell 24, 2086–2104. doi: 10.1105/tpc.112.096057
Gattolin, S., Sorieul, M., and Frigerio, L. (2011). Mapping of tonoplast intrinsic proteins in maturing and germinating Arabidopsis seeds reveals dual localization of embryonic TIPs to the tonoplast and plasma membrane. Mol. Plant 4, 180–189. doi: 10.1093/mp/ssq051
Ghugtyal, V., Vionnet, C., Roubaty, C., and Conzelmann, A. (2007). CWH43 is required for the introduction of ceramides into GPI anchors in Saccharomyces cerevisiae. Mol. Microbiol. 65, 1493–1502. doi: 10.1111/j.1365-2958.2007.05883.x
Gillmor, C. S., Lukowitz, W., Brininstool, G., Sedbrook, J. C., Hamann, T., Poindexter, P., et al. (2005). Glycosylphosphatidylinositol-anchored proteins are required for cell wall synthesis and morphogenesis in Arabidopsis. Plant Cell 17, 1128–1140. doi: 10.1105/tpc.105.031815
Hellens, R. P., Anne Edwards, E., Leyland, N. R., Bean, S., and Mullineaux, P. M. (2000). pGreen: a versatile and flexible binary ti vector for agrobacterium-mediated plant transformation. Plant Mol. Biol. 42, 819–832. doi: 10.1023/A:1006496308160
Hofmann, K., and Stoffel, W. (1993). TMbase: a database of membrane spanning protein segments. Biol. Chem. 374:166.
Howard, M. F., Murakami, Y., Pagnamenta, A. T., Daumer-Haas, C., Fischer, B., Hecht, J., et al. (2014). Mutations in PGAP3 impair GPI-anchor maturation, causing a subtype of hyperphosphatasia with mental retardation. Am. J. Hum. Genet. 94, 278–287. doi: 10.1016/j.ajhg.2013.12.012
Hruz, T., Laule, O., Szabo, G., Wessendorp, F., Bleuler, S., Oertle, L., et al. (2008). Genevestigator V3: a reference expression database for the meta-analysis of transcriptomes. Adv. Bioinformatics 2008, 1–5. doi: 10.1155/2008/420747
Ito, H., Fukuda, Y., Murata, K., and Kimura, A. (1983). Transformation of intact yeast cells treated with alkali cations. J. Bacteriol. 153, 163–168. doi: 10.1128/jb.153.1.163-168.1983
Jackson, L. P., Lewis, M., Kent, H. M., Edeling, M. A., Evans, P. R., Duden, R., et al. (2012). Molecular basis for recognition of dilysine trafficking motifs by COPI. Dev. Cell 23, 1255–1262. doi: 10.1016/J.DEVCEL.2012.10.017
Johnson, K. L. (2003). The fasciclin-like arabinogalactan proteins of Arabidopsis. a multigene family of putative cell adhesion molecules. Plant Physiol. 133, 1911–1925. doi: 10.1104/pp.103.031237
Kim, D. H., Eu, Y.-J., Yoo, C. M., Kim, Y.-W., Pih, K. T., Jin, J. B., et al. (2001). Trafficking of phosphatidylinositol 3-phosphate from the trans -Golgi network to the lumen of the central vacuole in plant cells. Plant Cell 13, 287–301. doi: 10.1105/tpc.13.2.287
Kinoshita, T. (2020). Biosynthesis and biology of mammalian GPI-anchored proteins. Open Biol. 10:190290. doi: 10.1098/rsob.190290
Kinoshita, T., and Fujita, M. (2016). Biosynthesis of GPI-anchored proteins: special emphasis on GPI lipid remodeling. J. Lipid Res. 57, 6–24. doi: 10.1194/jlr.r063313
Künzl, F., Früholz, S., Fäßler, F., Li, B., and Pimpl, P. (2016). Receptor-mediated sorting of soluble vacuolar proteins ends at the trans-Golgi network/early endosome. Nat. Plants 2:16017. doi: 10.1038/nplants.2016.17
Lalanne, E., Honys, D., Johnson, A., Borner, G. H. H., Lilley, K. S., Dupree, P., et al. (2004). SETH1 and SETH2, two components of the glycosylphosphatidylinositol anchor biosynthetic pathway, are required for pollen germination and tube growth in Arabidopsis. Plant Cell 16, 229–240. doi: 10.1105/tpc.014407
Lam, S. K., Siu, C. L., Hillmer, S., Jang, S., An, G., Robinson, D. G., et al. (2007). Rice SCAMP1 defines clathrin-coated, trans -golgi–located tubular-vesicular structures as an early endosome in Tobacco BY-2 Cells. Plant Cell 19, 296–319. doi: 10.1105/tpc.106.045708
Langhans, M., Marcote, M. J., Pimpl, P., Virgili-López, G., Robinson, D. G., and Aniento, F. (2008). In vivo trafficking and localization of p24 proteins in plant cells. Traffic 9, 770–785. doi: 10.1111/j.1600-0854.2008.00719.x
Lerich, A., Langhans, M., Sturm, S., and Robinson, D. G. (2011). Is the 6 kDa tobacco etch viral protein a bona fide ERES marker? J. Exp. Bot. 62, 5013–5023. doi: 10.1093/jxb/err200
Luschnig, C., and Seifert, G. J. (2011). Posttranslational modifications of plasma membrane proteins and their implications for plant growth and development. Plant Cell Monogr. 19, 109–128. doi: 10.1007/978-3-642-13431-9_5
MacMillan, C. P., Mansfield, S. D., Stachurski, Z. H., Evans, R., and Southerton, S. G. (2010). Fasciclin-like arabinogalactan proteins: specialization for stem biomechanics and cell wall architecture in Arabidopsis and Eucalyptus. Plant J 62, 689–703. doi: 10.1111/j.1365-313X.2010.04181.x
Maeda, Y., Murakami, Y., and Kinoshita, T. (2017). “Synthesis, genetics, and congenital diseases of GPI-anchored proteins,” in Paroxysmal Nocturnal Hemoglobinuria, eds Y. Kanakura, T. Kinoshita, and J. Nishimura (Tokyo: Springer Japan), 11–54. doi: 10.1007/978-4-431-56003-6_2
Maeda, Y., Tashima, Y., Yoko-o, T., Jigami, Y., Fujita, M., Kinoshita, T., et al. (2007). Fatty acid remodeling of GPI-anchored proteins is required for their raft association. Mol. Biol. Cell 18, 1497–1506. doi: 10.1091/mbc.e06-10-0885
Martinière, A., Lavagi, I., Nageswaran, G., Rolfe, D. J., Maneta-Peyret, L., Luu, D.-T., et al. (2012). Cell wall constrains lateral diffusion of plant plasma-membrane proteins. Proc. Natl. Acad. Sci. U.S.A. 109, 12805–12810. doi: 10.1073/pnas.1202040109
Montesinos, J. C., Sturm, S., Langhans, M., Hillmer, S., Marcote, M. J., Robinson, D. G., et al. (2012). Coupled transport of Arabidopsis p24 proteins at the ER-golgi interface. J. Exp. Bot. 63, 4243–4261. doi: 10.1093/jxb/ers112
Muñiz, M., and Riezman, H. (2016). Trafficking of glycosylphosphatidylinositol anchored proteins from the endoplasmic reticulum to the cell surface. J. Lipid Res. 57, 352–360. doi: 10.1194/jlr.r062760
Muniz, M., and Zurzolo, C. (2014). Sorting of GPI-anchored proteins from yeast to mammals – common pathways at different sites? J. Cell Sci. 127, 2793–2801. doi: 10.1242/jcs.148056
Murakami, Y., Tawamie, H., Maeda, Y., Büttner, C., Buchert, R., Radwan, F., et al. (2014). Null Mutation in PGAP1 Impairing Gpi-Anchor Maturation in Patients with Intellectual Disability and Encephalopathy. PLoS Genet 10:e1004320. doi: 10.1371/journal.pgen.1004320
Nebenführ, A., Gallagher, L. A., Dunahay, T. G., Frohlick, J. A., Mazurkiewicz, A. M., Meehl, J. B., et al. (1999). Stop-and-Go movements of plant golgi stacks are mediated by the acto-myosin system. Plant Physiol. 121, 1127–1141. doi: 10.1104/pp.121.4.1127
Nelson, B. K., Cai, X., and Nebenführ, A. (2007). A multicolored set of in vivo organelle markers for co-localization studies in Arabidopsis and other plants. Plant J. 51, 1126–1136. doi: 10.1111/j.1365-313X.2007.03212.x
Ortiz-Masia, D., Perez-Amador, M. A., Carbonell, J., and Marcote, M. J. (2007). Diverse stress signals activate the C1 subgroup MAP kinases of Arabidopsis. FEBS Lett. 581, 1834–1840. doi: 10.1016/j.febslet.2007.03.075
Oxley, D., and Bacic, A. (1999). Structure of the glycosylphosphatidylinositol anchor of an arabinogalactan protein from Pyrus communis suspension-cultured cells. Proc. Natl. Acad. Sci. U.S.A. 96, 14246–14251. doi: 10.1073/pnas.96.25.14246
Paidhungat, M., and Garrett, S. (1998). Cdc1 and the vacuole coordinately regulate Mn2+ homeostasis in the yeast Saccharomyces cerevisiae. Genetics 148, 1787–1798. doi: 10.1093/genetics/148.4.1787
Pain, C., Kriechbaumer, V., Kittelmann, M., Hawes, C., and Fricker, M. (2019). Quantitative analysis of plant ER architecture and dynamics. Nat. Commun. 10:984. doi: 10.1038/s41467-019-08893-9
Paladino, S., Pocard, T., Catino, M. A., and Zurzolo, C. (2006). GPI-anchored proteins are directly targeted to the apical surface in fully polarized MDCK cells. J. Cell Biol. 172, 1023–1034. doi: 10.1083/jcb.200507116
Paladino, S., Sarnataro, D., Pillich, R., Tivodar, S., Nitsch, L., and Zurzolo, C. (2004). Protein oligomerization modulates raft partitioning and apical sorting of GPI-anchored proteins. J. Cell Biol. 167, 699–709. doi: 10.1083/jcb.200407094
Pei, J., Millay, D. P., Olson, E. N., and Grishin, N. V. (2011). CREST - a large and diverse superfamily of putative transmembrane hydrolases. Biol. Direct. 6:37. doi: 10.1186/1745-6150-6-37
Pereira, A. M., Lopes, A. L., and Coimbra, S. (2016). JAGGER, an AGP essential for persistent synergid degeneration and polytubey block in Arabidopsis. Plant Signal. Behav. 11:e1209616. doi: 10.1080/15592324.2016.1209616
Pereira, C., Pereira, S., Satiat-Jeunemaitre, B., and Pissarra, J. (2013). Cardosin A contains two vacuolar sorting signals using different vacuolar routes in tobacco epidermal cells. Plant J. 76, 87–100. doi: 10.1111/tpj.12274
Pittet, M., and Conzelmann, A. (2007). Biosynthesis and function of GPI proteins in the yeast Saccharomyces cerevisiae. Biochim. Biophys. Acta Mol. Cell Biol. Lipids 1771, 405–420. doi: 10.1016/j.bbalip.2006.05.015
Sánchez-Simarro, J., Bernat-Silvestre, C., Gimeno-Ferrer, F., Selvi-Martínez, P., Montero-Pau, J., Aniento, F., et al. (2020). Loss of Arabidopsis β-COP function affects golgi structure, plant growth and tolerance to salt stress. Front. Plant Sci. 11:430. doi: 10.3389/fpls.2020.00430
Silva, L., De Almeida, R. F. M., Fedorov, A., Matos, A. P. A., and Prieto, M. (2006). Ceramide-platform formation and -induced biophysical changes in a fluid phospholipid membrane. Mol. Membr. Biol. 23, 137–148. doi: 10.1080/09687860500439474
Simons, K., and Gerl, M. J. (2010). Revitalizing membrane rafts: new tools and insights. Nat. Rev. Mol. Cell Biol. 11, 688–699. doi: 10.1038/nrm2977
Suzuki, K. G. N., Kasai, R. S., Hirosawa, K. M., Nemoto, Y. L., Ishibashi, M., Miwa, Y., et al. (2012). Transient GPI-anchored protein homodimers are units for raft organization and function. Nat. Chem. Biol. 8, 774–783. doi: 10.1038/nchembio.1028
Svetek, J., Yadav, M. P., and Nothnagel, E. A. (1999). Presence of a glycosylphosphatidylinositol lipid anchor on rose arabinogalactan proteins. J. Biol. Chem. 274, 14724–14733. doi: 10.1074/jbc.274.21.14724
Tanaka, S., Maeda, Y., Tashima, Y., and Kinoshita, T. (2004). Inositol deacylation of glycosylphosphatidylinositol-anchored proteins is mediated by mammalian PGAP1 and yeast Bst1p. J. Biol. Chem. 279, 14256–14263. doi: 10.1074/jbc.M313755200
Tashima, Y., Taguchi, R., Murata, C., Ashida, H., Kinoshita, T., and Maeda, Y. (2006). PGAP2 is essential for correct processing and stable expression of GPI-anchored proteins. Mol. Biol. Cell 17, 1410–1420. doi: 10.1091/mbc.e05-11-1005
Thomas, P. D., Ebert, D., Muruganujan, A., Mushayahama, T., Albou, L., and Mi, H. (2021). PANTHER : making genome-scale phylogenetics accessible to all. Protein Sci. 31, 8–22. doi: 10.1002/pro.4218
Ueda, Y., Yamaguchi, R., Ikawa, M., Okabe, M., Morii, E., Maeda, Y., et al. (2007). PGAP1 knock-out mice show otocephaly and male infertility. J. Biol. Chem. 282, 30373–30380. doi: 10.1074/jbc.M705601200
Umemura, M., Fujita, M., Yoko-O, T., Fukamizu, A., and Jigami, Y. (2007). Saccharomyces cerevisiae CWH43 is involved in the remodeling of the lipid moiety of GPI anchors to ceramides. Mol. Biol. Cell 18, 4304–4316. doi: 10.1091/mbc.e07-05-0482
Williams, C., Jiang, Y. H., Shashi, V., Crimian, R., Schoch, K., Harper, A., et al. (2015). Additional evidence that PGAP1 loss of function causes autosomal recessive global developmental delay and encephalopathy. Clin. Genet. 88, 597–599. doi: 10.1111/cge.12581
Wu, F.-H., Shen, S.-C., Lee, L.-Y., Lee, S.-H., Chan, M.-T., and Lin, C.-S. (2009). Tape-Arabidopsis sandwich – a simpler Arabidopsis protoplast isolation method. Plant Methods 5:16. doi: 10.1186/1746-4811-5-16
Yeats, T. H., Bacic, A., and Johnson, K. L. (2018). Plant glycosylphosphatidylinositol anchored proteins at the plasma membrane-cell wall nexus. J. Integr. Plant Biol. 60, 649–669. doi: 10.1111/jipb.12659
Yoko-o, T., Umemura, M., Komatsuzaki, A., Ikeda, K., Ichikawa, D., Takase, K., et al. (2018). Lipid moiety of glycosylphosphatidylinositol-anchored proteins contributes to the determination of their final destination in yeast. Genes Cells 23, 880–892. doi: 10.1111/gtc.12636
Yoo, S. D., Cho, Y. H., and Sheen, J. (2007). Arabidopsis mesophyll protoplasts: a versatile cell system for transient gene expression analysis. Nat. Protoc. 2, 1565–1572. doi: 10.1038/nprot.2007.199
Zhou, K. (2019). Glycosylphosphatidylinositol-anchored proteins in Arabidopsis and one of their common roles in signaling transduction. Front. Plant Sci. 10:1022. doi: 10.3389/fpls.2019.01022
Zimmermann, P., Hirsch-Hoffmann, M., Hennig, L., and Gruissem, W. (2004). GENEVESTIGATOR. Arabidopsis microarray database and analysis toolbox. Plant Physiol. 136, 2621–2632. doi: 10.1104/pp.104.046367
Keywords: Glycosylphosphatidylinositol (GPI), GPI-anchored proteins, Per1p, PGAP3, lipid remodeling, Arabidopsis
Citation: Bernat-Silvestre C, Ma Y, Johnson K, Ferrando A, Aniento F and Marcote MJ (2022) Characterization of Arabidopsis Post-Glycosylphosphatidylinositol Attachment to Proteins Phospholipase 3 Like Genes. Front. Plant Sci. 13:817915. doi: 10.3389/fpls.2022.817915
Received: 18 November 2021; Accepted: 07 January 2022;
Published: 11 February 2022.
Edited by:
Pilar Cubas, National Center for Biotechnology, Spanish National Research Council (CSIC), SpainReviewed by:
Giovanni Stefano, University of Florence, ItalyCopyright © 2022 Bernat-Silvestre, Ma, Johnson, Ferrando, Aniento and Marcote. This is an open-access article distributed under the terms of the Creative Commons Attribution License (CC BY). The use, distribution or reproduction in other forums is permitted, provided the original author(s) and the copyright owner(s) are credited and that the original publication in this journal is cited, in accordance with accepted academic practice. No use, distribution or reproduction is permitted which does not comply with these terms.
*Correspondence: Fernando Aniento, RmVybmFuZG8uYW5pZW50b0B1di5lcw==; María Jesús Marcote, bWFyaWFqZXN1cy5tYXJjb3RlQHV2LmVz
†These authors have contributed equally to this work and share senior authorship
Disclaimer: All claims expressed in this article are solely those of the authors and do not necessarily represent those of their affiliated organizations, or those of the publisher, the editors and the reviewers. Any product that may be evaluated in this article or claim that may be made by its manufacturer is not guaranteed or endorsed by the publisher.
Research integrity at Frontiers
Learn more about the work of our research integrity team to safeguard the quality of each article we publish.