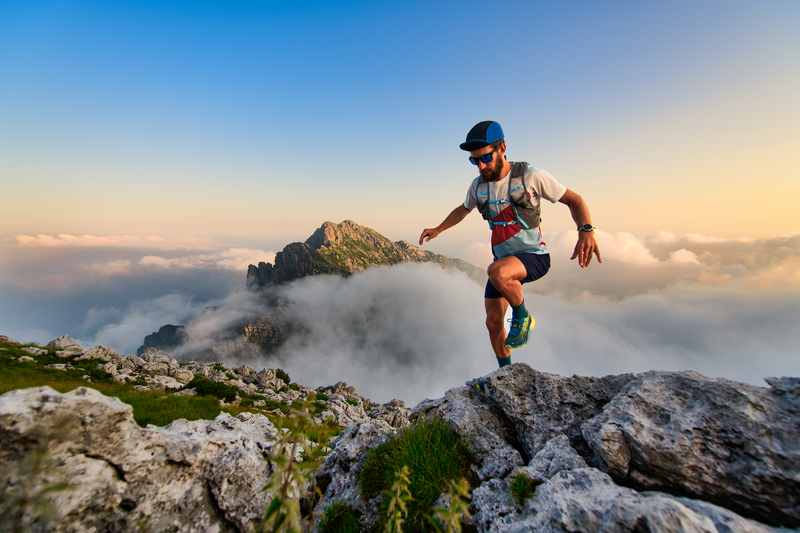
95% of researchers rate our articles as excellent or good
Learn more about the work of our research integrity team to safeguard the quality of each article we publish.
Find out more
ORIGINAL RESEARCH article
Front. Plant Sci. , 27 April 2022
Sec. Plant Development and EvoDevo
Volume 13 - 2022 | https://doi.org/10.3389/fpls.2022.815131
This article is part of the Research Topic Mechanisms Underlying Phenotypic Convergence in Plants View all 5 articles
Gravity is known as an important environmental factor involved in the regulation of plant architecture. To identify genes related to the gravitropism of Tartary buckwheat, a creeping line was obtained and designated as lazy1 from the mutant bank by 60Co-γ ray radiation. Genetic analysis indicated that the creeping phenotype of lazy1 was attributed to a single recessive locus. As revealed by the horizontal and inverted suspension tests, lazy1 was completely lacking in shoot negative gravitropism. The creeping growth of lazy1 occurred at the early seedling stage, which could not be recovered by exogenous heteroauxin, hormodin, α-rhodofix, or gibberellin. Different from the well-organized and equivalent cell elongation of wild type (WT), lazy1 exhibited dilated, distorted, and abnormally arranged cells in the bending stem. However, no statistical difference of indole-3-acetic acid (IAA) levels was found between the far- and near-ground bending sides in lazy1, which suggests that the asymmetric cell elongation of lazy1 was not induced by auxin gradient. Whereas, lazy1 showed up-expressed gibberellin-regulated genes by quantitative real-time PCR (qRT-PCR) as well as significantly higher levels of gibberellin, suggesting that gibberellin might be partly involved in the regulation of creeping growth in lazy1. RNA sequencing (RNA-seq) identified a number of differentially expressed genes (DEGs) related to gravitropism at stages I (before bending), II (bending), and III (after bending) between WT and lazy1. Venn diagram indicated that only Pectate lyase 5 was down-expressed at stages I [Log2 fold change (Log2FC): −3.20], II (Log2FC: −4.97), and III (Log2FC: −1.23) in lazy1, compared with WT. Gene sequencing revealed that a fragment deletion occurred in the coding region of Pectate lyase 5, which induced the destruction of a pbH domain in Pectate lyase 5 of lazy1. qRT-PCR indicated that Pectate lyase 5 was extremely down-expressed in lazy1 at stage II (0.02-fold of WT). Meanwhile, lazy1 showed the affected expression of lignin- and cellulose-related genes and cumulatively abnormal levels of pectin, lignin, and cellulose. These results demonstrate the possibility that Pectate lyase 5 functions as the key gene that could mediate primary cell wall metabolism and get involved in the asymmetric cell elongation regulation of lazy1.
Gravity, an important environmental factor, is involved in the regulation of plant architecture. However, the gravitropism of plants is highly complex and related to multistep biological processes, including gravity perception, signal formation in the gravity perceptive cells, intracellular and intercellular signal transduction, and asymmetric cell elongation between the upper and lower sides of the responding organs (Fukaki and Tasaka, 1999; Digby and Firn, 2010). According to the Cholodny-Went theory, the endodermal cells in shoot and columella cells in root have been regarded as the statocytes that mainly perceive gravity (Moritoshi, 1995; Blancaflor and Gilroy, 1998; Fukaki et al., 2002). Besides, statocytes also serve as the site for the formation of biochemical signals (Hensel, 1987). The occurrence of amyloplast sedimentation in statocytes triggers signal transduction and then the asymmetrical distribution of auxin, thus leading to asymmetric cell elongation (Iversen and Larsen, 2010). So far, there has been plenty of evidence collected to support these hypotheses. For example, Arabidopsis phosphoglucomutase (pgm) mutant exhibits the defect of gravitropism in root and inflorescence caused by the lack of amyloplasts (Toyota et al., 2013). Arabidopsis shoot gravitropism 2 (sgr2), sgr3, sgr4, sgr6, sgr8, and sgr9 exhibit the abnormal gravitropism of inflorescence and hypocotyl induced by aberrant amyloplast sedimentation (Fukaki and Tasaka, 1996; Yoshiro et al., 1997; Kato et al., 2002; Yano et al., 2003; Nakamura et al., 2011; Hashiguchi et al., 2013). However, there is high complexity in the mechanisms for signal transduction and transmission process of gravity signal from amyloplast sedimentation. A variety of secondary messengers, such as Ca2+, Insp3, and pH, may be involved in the process of signal transduction and transmission, which transform physical signals into physiological and biochemical signals (Perera, 2006; Urbina et al., 2006; Monshausen et al., 2011). In addition, it has been discovered that the phosphatidylinositol signaling pathway plays a role in regulating gravity signal and auxin polar transport (Mei et al., 2012).
The transport, signaling, and response of auxin have been identified as crucial for plant gravitropism (Salisbury et al., 1988; Zhen et al., 2010; Geisler et al., 2014). In general, there are three families of plant plasma membrane-associated transporters, namely, AUXIN RESISTANT1/LIKE AUX1 (AUX1/LAX) influx carriers, PIN-FORMED (PIN) efflux carriers, and P-GLYCOPROTEIN ATP-binding cassette (PGP) auxin transporters associated with polar auxin transport (Friml, 2003). Arabidopsis aux1 shows root agravitropic mutation phenotype by reducing auxin uptake within root apex, which indicates the involvement of auxin influx carrier protein AUX1 in root gravitropism regulation (Bennett et al., 1996). Besides, auxin efflux carrier mutants, such as pin2, pin3, pin7, and pin8, exhibit various defects in gravitropism (Bosco et al., 2012; Haga and Sakai, 2013; Rigo et al., 2013). Moreover, there are many modifier proteins, such as GOLVEN secretory peptides and stigmasterol binding protein ROSY1, involved in plant gravitropism by modulating the trafficking dynamics of auxin efflux carriers (Fernandez et al., 2013; Dalal et al., 2016). Arabidopsis DII-VENUS is a sensor involved in the regulation of gravitropism and plant growth by mapping auxin response and distribution (Brunoud et al., 2012).
At present, it remains incompletely understood that the mechanism is for the regulation of asymmetric cell elongation by auxin gradient. A two-phase model for maize stem gravitropism has been proposed through the function research of gravity-stimulated acid invertase gene (Ivr2) (Cosgrove, 1999; Long et al., 2002). In general, phase I is regarded as a signaling phase that requires a continuous gravity signal to induce a sufficient indole-3-acetic acid (IAA) gradient. Also, phase II is regarded as the growth phase that involves IAA signal transduction, protein synthesis, Ivr2 transcripts differentially stimulation, and the differential accumulation of hexose sugars, which leads to differential cell elongation (Cosgrove, 1999; Long et al., 2002). However, this model is unsuited to the explanation of other phenomena, including graviperception and cell wall loosening in maize (Cosgrove, 1999; Long et al., 2002). With experimental and computational approaches taken in combination, a model has been constructed for the apical hook guided by differential growth. The signaling pathways of auxin and ethylene play a role in coordinating both cell division and differential cell growth by mediating various cell division regulatory genes, including CYCA2;1, CYCA2;2, CYCA2;3, CYCA2;4, and SAMBA (Žádníková et al., 2016).
Crop architecture has a significant impact on yield, such as branching (tiller) number, angle, and plant height, which has long been considered for crop improvement (Coyne, 1980). The plant with prostrate growth phenotype has been first designated as “lazy” in maize mutant due to the defect of gravitropism (Jenkins and Gerhardt, 1931; Van, 1936). Subsequently, there have been many lazy mutants discovered in crops, such as rice oslazy1 (Jones and Adair, 1938) and tomato lelazy-1 and lelazy-2 (Roberts, 1984; Hasenstein and Kuznetsov, 1999). Although OsLAZY1 is an uncharacteristic protein with no functional domains, the biochemical function of OsLAZY1 has been proposed to participate in the downstream gravity-sensing mechanism (Abe et al., 1994, 1996, 2010; Li, 2007; Takeshi and Moritoshi, 2007; Akie et al., 2019). The overexpression of OsPIN2 suppresses OsLAZY1 expression, thus affecting tiller numbers, angle, and plant height (Chen et al., 2012). In addition, Brevis Radix Like 4 (OsBRXL4), as an interacting protein of OsLAZY1, is also involved in shoot gravitropism and tiller angle regulation by affecting polar auxin transport (Li et al., 2019). As the homolog gene of OsLAZY1, maize ZmLAZY1 can mediate the complex interactions between gravity, auxin, light, and plant growth (Dong et al., 2013; Howard et al., 2014). As a functional ortholog of the OsLAZY1 gene, LjLAZY3 plays a vital role in the regulation of root gravitropism (Chen et al., 2019).
Tartary buckwheat [Fagopyrum tataricum (L.) Gaertn] is a dicotyledonous plant that belongs to the genus Fagopyrum of Polygonaceae. In fact, Tartary buckwheat is usually classified as a cereal that is widely planted in southwest China (Bulan et al., 2017). Modern people prefer to consume Tartary buckwheat for its high nutritional value and health benefits (Zhang et al., 2017; Kalinová et al., 2018; Luo et al., 2020). To gain insight into Tartary buckwheat plant architecture for the research of gene function and high yield breeding, a series of mutation lines were created by 60Co-γ ray radiation. Herein, a creeping mutant is reported with impaired shoot gravitropism, designated as lazy1. To reveal the mechanisms of lazy1 for the lack of shoot gravitropism, the phenotypic observation, exogenous hormone treatment, endogenous hormone determination, cell wall structural components measurement, RNA sequencing (RNA-seq), and quantitative real-time PCR (qRT-PCR) analysis were performed. The existing results are expected to provide more insight into the regulation mechanisms for lazy1 creeping phenotype.
There were approximately 30,000 seeds of Tartary buckwheat “cv. Jinqiaomai 2” [wild type (WT)] exposed to 60Co-γ ray radiation in the Radiation Center of Guizhou Academy of Agricultural Sciences at 200, 400, and 600 Gy, respectively. All seeds were planted in 2015 during autumn growth season at the Research Center of Buckwheat Industry Technology in Guizhou Province, China (908 m, 26°35′N, 106°52′E) for collection by the individual plant. M2 plants were grown in 2016 during spring growth seasons for mutants screening. Subsequently, a creeping mutant, temporarily designated as lazy1, was screened through phenotypic observation. For the purpose of genetic stability test, the plants of M3, M4, and M5 were grown in 2016 and 2017 during spring and autumn growth seasons at the Research Center of Buckwheat Industry Technology in Guizhou Province. Besides, the plants of M6 were grown in 2018 during winter growth season at the Hainan Breeding and Research Farm of Guizhou Province, China (10 m, 18°45′N, 108°99′E). The plants of M4 were backcrossed to WT in 2017, and the progenies of F2 were grown in 2018 at the Research Center of Buckwheat Industry Technology in Guizhou Province.
The M5 seeds of lazy1 and WT were planted in 96 cylindrical pots (18.5 cm × 16.2 cm × 13 cm) in 2018, 2019, and 2020 during spring and autumn growth seasons for the purpose of phenotypic and microscopic observation, RNA-sequencing, and metabolites determination. The M5 seeds of lazy1 and WT were planted in 12 rectangle pots (68.5 cm × 38.0 cm × 25.0 cm) in 2018 and 2019 during autumn growth seasons for the investigation into major agronomic traits.
The plants of lazy1 and WT at 5 days after germination were horizontally placed, and the stem curvature angle was measured by an angulometer at a 30-min interval for the first 4 h and on a daily basis for the following 2 weeks. The plants were photographed 14 days after treatment. The plants of lazy1 and WT were inverted by 180° at the time of flowering and photographed 24 h after treatment.
The seeds of lazy1 and WT were immersed into 50 and 150 mmol/L of heteroauxin, hormodin, α-rhodofix, and gibberellin, respectively. Later, the plants were sprayed with 50 and 150 mmol/L of heteroauxin, hormodin, α-naphthylacetic acid, and gibberellin, respectively, and photographed 3 days after spraying.
The curved part of lazy1 stem and the parallel erect part of WT stem at 10 days after germination (bending stage) were sampled and prefixed with a mixed solution of 3% glutaraldehyde. Then, they were postfixed in 1% osmium tetroxide, dehydrated in series acetone, infiltrated in a graded series of acetone solution and propylene oxide, and embedded in Spurr’s epoxy resin. The ultrathin sections were cut with a diamond knife and stained with uranyl acetate and lead citrate. Finally, the microstructure of plant cells was photographed using a Transmission Electron Microscope (JEM-1400PLUS, Tokyo, Japan).
The far- and near-ground bending sides of lazy1 curved stem and the parallel erect parts of WT stem at 10 days after germination were sampled and frozen in liquid nitrogen immediately. Then, the levels of auxin (IAA) and gibberellin (GA3) were detected using high-performance liquid chromatography (Rigol L3000, Beijing, China) following the protocol recommended by the manufacturer. The parameters used for IAA measurement are detailed as follows: the ratio at which mobile phase was prepared by mixing methanol and aqueous acetic acid solution is 400:600 ml, the injection volume is 10 μl, the flow rate is 0.8 ml/min, the column temperature is 35°C, the aliasing time is 40 min, the excitation wavelength is 275 nm, and the emission wavelength is 345 nm. The parameters used for GA3 measurement are detailed as follows: the ratio at which mobile phase was prepared by mixing methanol and aqueous acetic acid solution is 35:65, the injection volume is 10 μl, the flow rate is 10 ml/min, the column temperature is 30°C, the aliasing time is 30 min, and the detection wavelength is 254 nm.
The curved part of lazy1 stem and the parallel erect part of WT stem at 10 days after germination were sampled for the measurement of major structural components of cell walls. The contents of cellulose, lignin, and pectin were assayed using Cellulose Detection Kit “CLL-1-Y” (Suzhou Comin Biotechnology, Suzhou, China), Lignin Detection Kit “BC4205” (Beijing Solarbio Science & Technology Co., Ltd., Beijing, China), and Pectin Detection Kit “BC1405” in accordance with the instructions from the manufacturer, respectively.
A total of 18 plants for each repetition were sampled at harvest for the investigation into branch number, stem node number, and internode length.
The target stem of lazy1 and WT at 5 (before bending), 10 (bending), and 15 days (after bending) after germination were sampled and frozen in liquid nitrogen immediately. Total RNAs were extracted using a Plant RNA Kit (Tiangen Biotech, Beijing, China) in the line with the instructions from the manufacturer. The quality of RNAs was examined on 1% agarose gel. RNA concentrations were detected using NanoDrop 2000 spectrophotometer (Thermo Fisher Scientific, Waltham, MA, United States). The sequencing of the library was performed on an Illumina HiSeq platform. The raw data were filtered to trim adaptor sequences while removing low-quality sequences (Q < 20) with >10% uncertain (N) bases. The NCBI database was used to annotate gene function. The criteria of Log2 fold change (Log2FC) ≥ 1 (or ≤ –1) and false discovery rate (FDR) < 0.01 were applied for the identification of differentially expressed genes (DEGs). DEGs were analyzed using DESeq software. The gene functions of DEGs were annotated by Gene Ontology (GO).1 The GO enrichment analysis was conducted using GO:TermFinder software. The Kyoto Encyclopedia of Genes and Genomes (KEGG) database was adopted for DEG pathway enrichment analysis.
The cDNA was synthesized using the total RNA of the curved stem in lazy1 and the parallel erect part in WT at 10 days after germination (bending stage) for the detection of qRT-PCR. To explain the phenotype of lazy1 and verify the reliability of RNA-seq, a total of 25 genes were selected to perform qRT-PCR using SoFast™ EvaGreen® Supermix and CFX96™ (Takara, Dalian, China). Transcript levels were normalized against Actin. The cDNA was amplified under the following cycling conditions: (1) one cycle of 95°C for 30 s, (2) 40 cycles of 95°C for 5 s, 60°C for 30 s, and (3) melt-curve from 65 to 95°C by 5 s per step with a 0.5°C increment. The sequences of gene-specific primers are listed in Supplementary Table 1.
The gene sequence of Pectate lyase 5 from RNA-seq was applied as the template for PCR analysis. The Pectate lyase 5 cDNA was amplified with primers F: 5′-ATGAAAAATCTCCATAAATTCTCTCTTC-3′ and R: 5′-TCAGCATTTTTTCCCCTTATTACA-3′. The sequencing of PCR products was performed by Sangong Biotech Co., Ltd. (Shanghai).
The cDNA was synthesized using the total RNA of root, leaf, and flower in WT for the analysis of PCR and gel electrophoresis (2% agarose). The Pectate lyase 5 cDNA was amplified with primers F: 5′-GATCCAAGACGAGCCATTGT-3′ and R: 5′-ATCACCGTCCGATCTTGTTC-3′ under the following cycling conditions: (1) one cycle of 98°C for 2 min, (2) 35 cycles of 98°C for 10 s, 55°C for 15 s, and 72°C for 1 min, and (3) 72°C for 1 min.
Data were analyzed using SPSS software (IBM SPSS for Windows, version 19.0, United States). Results are presented as mean ± standard deviation.
A total of 8,613 lines derived from the mutation bank were screened, and the line “LW600-47-1” was identified as a creeping mutant. Then, 60 seeds of this mutant were sowed, with all of the sprouted plants identified as having the homozygous mutant genotype. Meanwhile, the genetic stability of the creeping phenotype was demonstrated through growth in different seasons (i.e., spring, autumn, and winter) and at various altitudes, latitudes, and longitudes (Guizhou and Hainan). In addition, the creeping and erect plants in the self-progeny of heterozygote plants were segregated at a ratio of 1:3, suggesting that the creeping phenotype of LW600-47-1 was attributed to a single recessive locus (Supplementary Table 2). As the creeping phenotype had been often reported as “lazy” in rice, maize, and tomato, we, therefore, designated the creeping mutant LW600-47-1 as a “lazy” mutant. The “lazy” mutants are often linked with gravity deficiency in rice, maize, and tomato (Jenkins and Gerhardt, 1931; Van, 1936; Jones and Adair, 1938; Roberts, 1984; Hasenstein and Kuznetsov, 1999).
Compared with WT, the stem of lazy1 was inflected due to the lack of negative geotropism at the early seedling stage and gradually twisted during growth (Figures 1A,B). At maturity, lazy1 exhibited a complete creeping phenotype, indicating that the extremely serious defect of gravity response occurred in lazy1. Meanwhile, lazy1 displayed more stem nodes and branches, with branch angle and the length of upper internodes being larger at harvest (Supplementary Figure 1).
Figure 1. Phenotypic observation of lazy1 plant at 5 (A) and 35 days (B) after germination, 14 days after horizontal treatment (C), 0 and 24 h after inverted suspension treatment (D), and 3 days after exogenous hormone treatment (E). Red arrows point to the bending stem. Scale bar = 5 cm.
Different from the well-organized and equivalent cell elongation of WT, the cells of the curved side of lazy1 were found to be dilated, distorted, and abnormally arranged during observation by transmission electron microscope, which indicates the occurrence of asymmetric cell elongation in lazy1 bending stem (Figure 2).
Figure 2. Microscopic observation of lazy1 bending stem. Red arrows point to the impaired cells wall and blue arrows point to the starch granules. Scale bar = 5 μm.
To identify the gravity response of lazy1, seedlings at 5 days were horizontally placed. A rapid gravity response of WT stem was observed after 1 h of treatment. However, the angle of the lazy1 stem was unaffected by gravity even after 2 weeks of treatment (Figure 1C and Supplementary Figure 2). Furthermore, an inverted suspension test was performed to confirm the gravity response, and we found rapid negative geotropism in the top organs of WT plants but not lazy1 mutants after 24-h treatment (Figure 1D). These observations suggested that lazy1 lacked shoot negative gravitropism.
To determine the effects of the exogenous hormone on the gravity response of lazy1 mutant, 50 and 150 mmol/L of heteroauxin, hormodin, α-rhodofix, and gibberellin were applied. As shown in Figure 1E, the creeping phenotype of lazy1 was failed to be recovered by either high or low concentrations of these exogenous hormones.
To determine the effects of endogenous hormone distribution on the creeping growth of lazy1, the auxin (IAA) and gibberellin (GA3) on the far- and near-ground bending sides were detected. Surprisingly, no statistical difference of IAA levels was found between the far- and near-ground bending sides in lazy1, which suggests that the creeping phenotype of lazy1 was not attributable to the asymmetric distribution of IAA (Figure 3A). Also, the levels of GA3 were similar between the far- and near-ground bending sides in lazy1 (Figure 3A). Compared with WT, lazy1 exhibited similar levels of IAA but significantly higher levels of GA3 on the both far- and near-ground bending sides of lazy1 (Figure 3A). Moreover, the contents of major cell wall structural materials in the lazy1 bending stem were detected. Compared with WT, lazy1 showed significantly higher contents of pectin and lignin but significantly lower content of cellulose (Figure 3B), indicating a disruption caused to the metabolism related to cell wall structure in lazy1.
Figure 3. Hormone levels and major cell wall structural components content of lazy1 bending stem. (A) The levels of GA3 and IAA in near-ground (lazy1-n) and far-ground (lazy1-f) side of lazy1 bending stem. (B) The contents of pectin, lignin and cellulose in lazy1 bending stem. Bars indicate standard deviation (SD, n = 4). “ns” indicates no significant difference. “*” and “**” indicate the significantly difference at 0.05 and 0.01 levels, respectively.
To gain insights into the molecular mechanisms of creeping phenotype in lazy1, the RNA-seq of the stem was performed at stages I (before bending), II (bending), and III (after bending), respectively. Based on the threshold values of Log2 ratio ≥ 1 and FDR < 0.05, a total of 85, 104, and 31 genes were identified as DEGs at stages I, II, and III, respectively, between lazy1 and WT, out of which 55, 84, and 11 DEGs were found up-expressed and 30, 20, and 20 DEGs were shown to be down-expressed in lazy1, respectively (Figure 4A, Supplementary Figure 3, and Supplementary Table 3). The Venn diagram indicated one common DEG among stages I, II, and III, six common DEGs between stages I and II, two common DEGs between stages I and III, and two common DEGs between stages I and III (Figure 4B).
Figure 4. Analysis of DEGs and Venn between WT and lazy1 at stage I (before bending), II (bending), and III (after bending) by RNA-seq. (A) The number of up-expressed and down- expressed DEGs between WT and lazy1. (B) The number of common DEGs between WT and lazy1 by Venn analysis.
The GO analysis was further performed to identify the significantly enriched categories of genes (Figure 5). The “metabolic process,” “cellular process,” and “single-organism process” were identified as the most dominant GO terms enriched in the biological process category; the “cell,” “cell part,” and “organelle and membrane” were determined as dominant in the cellular component category; the “catalytic activity” and “binding” were revealed to be dominant in the molecular function category (Figure 6).
Figure 5. Gene ontology (GO) analysis of DEGs at stage I (before bending), II (bending), and III (after bending).
Figure 6. Quantitative real-time PCR analysis of DEGs related to lazy1 phenotype. (A) The FKPM value of genes by RNA-seq. (B) The relative expression levels of gene by qRT-PCR. Bars indicate standard deviation (SD, n = 4). * and ** indicate the significant difference at 0.05 and 0.01 levels, respectively between WT and lazy1.
Compared with WT, lazy1 has 5 down-expressed and 3 up-expressed common DEGs that are altered in two or three examined stages (Figure 7). Among them, only Pectate lyase 5, which is capable to catalyze the dissolution of pectin chains and regulate primary cell walls (Palusa et al., 2007), was found to be down-expressed at stages I, II, and III in lazy1 stem. Sulfate transporter 3;5, a low-affinity sulfate transporter, was found to be down-expressed at stages I and II in lazy1 stem (Kataoka et al., 2004). In contrast, there were another two up-expressed common DEGs, namely, spermidine hydroxycinnamoyl transferase and glycolate oxidase. In addition, some common DEGs have not yet been functionally characterized, including Ft_newGene_4601, FtPinG0007358500.01, FtPinG0004018000.01, and Ft_newGene_3426 (Figure 7).
Figure 7. Analysis of DEGs at stage I (before bending), II (bending), and III (after bending). “ns” indicates no significant difference of gene expression was detected between WT and lazy1. “-” indicates the gene expression level was not detected.
Compared with WT, lazy1 has 10 up-expressed and four down-expressed DEGs belonging to the transcription factor families, including MADS-box, BTB and TAZ, MYB, AP2/ERF, KNOX, NLI AP2/ERF, NLP FAR1, PHD-finger, and RAD (Figure 7). Among them, BT1 and BT2 were found to be up-expressed in lazy1 at stage I, which positively regulated the auxin signaling in the initial response to the gravity of lazy1 (Mandadi et al., 2009; Robert et al., 2009). Meanwhile, TIM50 and KNOX7 were found to be up-expressed in lazy1 at stage II, which positively regulated the cell wall biosynthesis and asymmetry cell elongation of lazy1, respectively (Kumar et al., 2012; Wang et al., 2020). Furthermore, RAD-like 3 was found to be down-expressed in lazy1 at stage III, which negatively regulated the asymmetry cell elongation of lazy1 (Baxter et al., 2007). In addition, many genes related to plant meristems development and organ growth were found to be up-expressed in lazy1 at stages I, II, and III, respectively, including MYB3R1, MYB86 (Kobayashi et al., 2015), AIL5, AIL6 (Ouakfaoui et al., 2010), ACS1 (Rodrigues-Pousada et al., 1993), and AGL8 homolog (Mandel and Yanofsky, 1995), while the shoot meristem-related PHD-finger gene OBE3 (Ta-Fang et al., 2016), transposase-derived transcription factor gene FAR1-RS5L (Lin and Li, 2018), and ERF118-like (Seyfferth et al., 2018) were found to be down-expressed in lazy1.
Compared with WT, lazy1 has five up-expressed and two down-expressed DEGs involved in signal transduction (Figure 7). Among them, Indole-3-acetic acid-amido synthetase was found to be down-expressed in lazy1 at stage I, and AIR12 was found to be up-expressed in lazy1 at stage II, suggesting that the metabolism and signaling of auxin were affected in lazy1 (Zhang et al., 2009; Gibson and Todd, 2015). Meanwhile, the genes related to plant growth and development were found to be up-expressed in lazy1 at stages II and III, respectively, including NsLTP2 (Thoma et al., 1994), RLPK (Kanamoto et al., 2002), RALF (Liam and Turner, 2017), and IRE1A-like (Bao et al., 2019). The chloroplast differentiation-related gene STY17 (Eisa et al., 2019) and cytokinin-related gene CKI1 (Dobisova et al., 2017) were found to be down-expressed in lazy1 at stages I and II, respectively.
Compared with WT, lazy1 has plenty of DEGs involved in cell cycle, cell wall, cell elongation, and cytoskeleton (Figure 7). Among them, Glycine-rich cell wall structural protein 1 was found to be down-expressed in lazy1 at stage I, while Expansion A10 (Hur et al., 2014), WAT1-related protein gene (Ranocha et al., 2010; Grones and Friml, 2015), ARAF1 (Chavez Montes et al., 2008), and LRX4 (Herger et al., 2020) were found to be up-expressed in lazy1 at stages I and II, respectively, suggesting that the regulation of cell wall structure was affected in lazy1. The abnormal expression of genes related to the metabolism of pectin, lignin, and cellulose was identified in lazy1 at stages I, II, and III, respectively, including Pectate lyase 5 (Palusa et al., 2007), Pectate lyase 15 (Palusa et al., 2007), PBP1 (Benjamins, 2003), TBL3 (Bischoff et al., 2010), Laccase-12, Laccase-12-like, Laccase-15-like, Laccase-4 (Liu et al., 1994; Berthet et al., 2011), beta-galactosidase 5 (Gantulga et al., 2008), IRX15-LIKE (Brown et al., 2011), XTH6, XTH31 (Ryusuke and Kazuhiko, 2001), CSLE1 (Liepman et al., 2005), and CSLG2 (Xu et al., 2020). Meanwhile, VIN3-like 1 was found to be down-expressed in lazy1 at stage II, which regulated the branching angle of lazy1 (Zhao et al., 2010). UGT75B1 was found to be up-expressed in lazy1 at stage II, which modulated the auxin-ethylene cross-talk for gravitropism (Nziengui et al., 2018). TOR2 was found to be up-expressed in lazy1 at stage III, which regulated the twisting growth of lazy1 (Buschmann et al., 2009). In addition, some genes related to cell cycle and redox were found to be abnormally expressed in lazy1 at stages I and II, respectively, including TPX2 (Beáta et al., 2013), Cyclin-D3-1 (Bartkova et al., 1998; Randall et al., 2015), NACK1 (Tanaka et al., 2004), L-ascorbate oxidase homolog (Lim, 2012), and Peroxidase 72 (Fernández-Pérez et al., 2015).
To identify the genes that are probably related to the creeping growth of lazy1 mutant, a total of 25 genes, which are responsible for regulating signal transduction, cell cycle, cell wall, and cell elongation, were selected for qRT-PCR detection at the bending stage of lazy1. As expected, the expression of all selected genes between lazy1 and WT showed a trend that is consistent with the transcriptome data (Figure 6). Compared with WT, the auxin response factor genes AIR12, ARF9-like, and ARF22 were up-expressed in lazy1 stem, suggesting that the auxin response was enhanced in lazy1. In addition, the gibberellin-regulated protein genes, including GA20ox1, GRP4, GRP6-like, Snakin-1, and Snakin-2-like, were up-expressed, implying that both GA biosynthesis and response were enhanced in lazy1. Furthermore, the cell cycle-related gene Cyclin-D3-1 was up-expressed, but SPO11-2-like was down-expressed. Besides, the cellulose-related genes CSLG2 and CSI1-like were down-expressed, while the xyloglucan- and lignin-related genes GE13BG12-like, XTH 31, UGT75B1, IRX15-like, Laccase 4, and Fructokinase 7 were up-expressed in lazy1 stem. Notably, Pectate lyase 5 was extremely down-expressed in lazy1 stem, the level of which was only one fifties of WT.
To analyze the sequence of Pectate lyase 5 between lazy1 and WT, PCR-based cloning was performed. Pectate lyase 5 encodes a 231-amino acid protein containing two pbH domains. According to nucleotide sequencing, a fragment deletion was detected in the coding region of Pectate lyase 5 in lazy1, which induced the destruction of pbH domain 1 and the changes of three-dimensional structure in Pectate lyase 5, as compared to WT (Figure 8A and Supplementary Figures 4, 5).
Figure 8. Alignment of Pectate lyase 5 amino acid sequence (A) and the tissue specific expression of Pectate lyase 5 (B). Red letters indicate the different amino acid sequences between WT and lazy1. Blue lines are the locations of pbH domains.
Meanwhile, the PCR-based gene expression of Pectate lyase 5 was performed using WT as material. According to the analysis of gene tissue-specific expression, Pectate lyase 5 was identified to be rarely expressed in root, leaf, and flower but highly expressed in stem (Figure 8B).
The negative geotropic growth of crop shoot is crucial for plant architecture, colony growth, and yield formation (Coyne, 1980). At present, prostrate or creeping phenotype has been reported as a “lazy” growth habit in various plants, such as rice, tomato, maize, barley, and Arabidopsis (Jones and Adair, 1938; Roberts, 1984; Türkan and Suge, 1991; Howard et al., 2014; Taniguchi et al., 2017). Herein, a novel Tartary buckwheat mutant (lazy1) with a “lazy” growth habit was reported by means of 60Co-γ ray radiation. Compared with WT, lazy1 displayed curving stem at the early seedling stage and fully prostrated during growth due to the extremely serious defect of shoot gravitropism. In addition, lazy1 exhibited impervious growth during both horizontal and inverted suspension tests, which indicates that lazy1 was completely lacking in shoot negative gravitropism.
It is widely known that hormones are responsible for the regulation of plant gravitropism (Venbrinkab and Kiss, 2019; Yin et al., 2019). According to the Cholodny-Went theory, the asymmetric distribution of auxin plays a crucial role in the regulation of plant gravitropism (Zhen et al., 2010; Venbrinkab and Kiss, 2019). Auxin has been demonstrated as the major hormone that is effective not only in triggering lignin deposition and cell wall strengthening in the far-ground bending side (Naoki and Yoshio, 1978) but also in inducing pectate lyase to remodel the cell wall during the course of cell elongation and differentiation (Domingo et al., 1998). Lignin, cellulose, and pectin are known as the major cell wall structural components in the shoot of plants. In this study, it was discovered that the contents of lignin, cellulose, and pectin in the bending stem of lazy1 were significantly affected, compared with WT (Figure 3). However, there was no asymmetric distribution of auxin detected on both far- and near-ground bending sides in lazy1. Despite this, lazy1 did show dilated, distorted, and abnormally arranged cells in the bending stem. Therefore, it was speculated that the creeping phenotype of lazy1 was regulated by the abnormal metabolism of cell wall structural components.
Despite insufficient evidence related to the regulation of gravitropism by gibberellins, the role of gibberellins in the differential shoot growth following gravitropism has been demonstrated (Rood et al., 1987). Probably, gibberellins could act as the modulator of auxin sensitivity, which reduces the response to gravity but increases the variance of this process (Rodrigo et al., 2011). In this study, lazy1 exhibited not only significantly higher levels of GA3 but also a larger length of upper internodes, compared to WT. Meanwhile, qRT-PCR revealed that the gibberellin-regulated genes were up-expressed in the bending stem of lazy1 (Figure 6B), which suggests the possible involvement of gibberellins in the process of shoot gravitropism.
As a directional response to gravity stimulus, plant gravitropism is regulated by complex signaling and metabolic networks (Venbrinkab and Kiss, 2019). The secondary messenger Ca2+ is suggested to be involved in the transduction and transmission of the signal after the gravity signal (Monshausen et al., 2011). Calcium and BTB proteins play their specific roles in auxin transport (Mandadi et al., 2009; Robert et al., 2009). In this study, the calcium-binding protein gene PBP1 and BTB protein genes BT1 and BT2 were found to be up-expressed before bending in lazy1 (Figure 7). Despite no detection of DEGs related to auxin transport between lazy1 and WT, the auxin-related gene Indole-3-acetic acid-amido synthetase was down-expressed in lazy1 before bending. Rice tled1-D mutant shows an increase in both leaf angle and tiller numbers, which is attributed to the defective function of Indole-3-acetic acid-amido synthetase (Zhang et al., 2009). Therefore, it is inappropriate to completely rule out the roles of auxin signaling in the initial response to gravity in lazy1 before bending.
In addition to the Cholodny-Went theory, some other new hypotheses have been put forward in relation to the principal mechanism of gravitropic growth regulation. Not only does gravistimulation inhibit the infiltration of auxin-induced wall-loosening factors into the extension-restricting epidermal walls, but it also leads to differential growth temporarily (Edelmann, 2010). It is suggested that plant gravitropism is subjected to regulation by the different sensitivity between the upper and lower sides under gravity stimulus (Evans, 1992). The lateral distribution of gravity-induced growth inhibitors is suspected to inhibit auxin activity in the upper side organ (Tokiwa et al., 2006). Based on RNA-seq and qRT-PCR, the signaling and metabolic networks of asymmetric cell elongation regulation for lazy1 are illustrated in Figure 9.
Figure 9. The model for the regulation of shoot gravitropism in lazy1 based on RNA-seq and qRT-PCR. Red arrows represent the elevated contents of metabolite or up-expressed levels of gene in lazy1. Green arrows represent the decreased contents of metabolite or down-expressed levels of gene in lazy1.
It was common for the asymmetric cell elongation to occur in the mutants lacking in gravitropism. Plant cell elongation is determined by not only turgor pressure but also mechanical characteristics of the cell wall (Taiz, 1984). In this study, lazy1 showed a large number of DEGs related to cell division, cell wall, cell elongation, and cytoskeleton (Figure 7), as well as the abnormal cell structure on the bending side (Figure 2). Particularly, the abnormal expression of Glycine-rich cell wall structural protein 1, Expansion A10, WAT1-related protein gene, ARAF1, and LRX4 was detected in lazy1 at stages I and II, respectively, suggesting that the regulation of cell wall structure was affected in lazy1 (Ryser et al., 1997; Chavez Montes et al., 2008; Ranocha et al., 2010; Hur et al., 2014; Grones and Friml, 2015; Herger et al., 2020). Cell-wall polysaccharides play an important role in the control of the extensibility of the cell wall (Taiz, 1984). The cell wall metabolism is closely associated with gravity response (Ikushima et al., 2008). The degradation of cell wall xyloglucan accelerates stem elongation and blocks upright-stem gravitropism in poplar through the overexpression of xyloglucanase (Hayashi and Kaida, 2011). Compared to WT, lazy1 exhibited up-expressed xyloglucan endotransglucosylase/hydrolases gene XTH6 and xyloglucan acetylation gene TBL3 before bending and also IRX15-like and XTH31 at the bending stage (Figure 7). Cellulose is involved in the hypocotyl graviresponses regulated by brassinosteroids in Arabidopsis (Marc et al., 2021). Lignin plays a vital role in the generation of maturation stress for normal and compression wood by increasing the compressive stress applied to the cell wall matrix (Okuyama et al., 1998). The viscosity of pectin is negatively correlated with the growth rate in mung bean hypocotyl (Taiz, 1984). There were many DEGs related to the metabolism of lignin, pectin, and cellulose, as identified between lazy1 and WT (Figure 7), which is consistent with the results of the change to lignin, pectin, and cellulose contents in lazy1 bending stem (Figure 3). Therefore, it is suggested that the disrupted cell wall metabolism induces the occurrence of asymmetric cell elongation and leads to the defect in the ability of plants to withstand gravity, which enhances the creeping growth of lazy1.
LAZYs are involved in gravitropism regulation when environmental signals are converted into differential growth, both after amyloplast sedimentation (Abe et al., 1994) and before auxin gradient formation (Godbolé et al., 1999; Li, 2007; Takeshi and Moritoshi, 2007). Among various LAZYs, only LAZY1 was reported to regulate shoot gravitropism in rice (Li, 2007; Takeshi and Moritoshi, 2007), maize (Dong et al., 2013), and Arabidopsis (Taniguchi et al., 2017). Rice OsLAZY1 functions as a negative regulator of polar auxin transport (Li, 2007). However, it is possible for the target gene of Tartary buckwheat lazy1 not to be the homologous gene of OsLAZY1, because the indistinguishable levels of auxin were detected between WT and lazy1.
A time-series gene expression data were screened based on RNA-seq (Figure 7 and Supplementary Table 3). Compared with WT, only Pectate lyase 5 down-expressed at stages I, II, and III in lazy1. That is, the metabolism of pectin in lazy1 was affected throughout the gravity response. Pectin molecules can be used to build cross-links through calcium involved in the regulation of cell wall strength and growth for expanding organs (Yamaoka and Chiba, 1983; Knox et al., 1990; Peaucelle et al., 2011). Pectate lyase catalyzes the dissolution of pectin chains for the loosening, remodeling, and rearrangement of the cell wall (Baker et al., 1990; Palusa et al., 2007). Pectate lyase genes play important and diverse roles in the development of different plant tissues (Palusa et al., 2007; Wieczorek et al., 2014; Sun et al., 2018; Yang et al., 2020). Most Pectate lyase genes are preferentially expressed in flowers of the plants (Palusa et al., 2007; Sun et al., 2018), while Tartary buckwheat Pectate lyase 5 exhibited tissue-specific expression in stem (Figure 8B). Meanwhile, qRT-PCR revealed that Pectate lyase 5 was rarely expressed at stage II (0.02-fold of WT) in the bending stem of lazy1 (Figure 6B). As expected, Tartary buckwheat lazy1 harbors genetic mutation of Pectate lyase 5 according to gene sequencing (Figure 8A and Supplementary Figure 4). Rice rbh1-1 mutant shows twisty and whitish spikelet due to the defective function of pectate lyase (He et al., 2021). In this study, Tartary buckwheat lazy1 exhibited twisty and groveling stem with increased pectin content. The expression of Pectate lyase has been confirmed to be significantly up-regulated by auxin induction (Domingo et al., 1998). The pectin-related genes, including Pectin lyase-like, Pectin lyase 2, Pectinesterase-like, and Pectin-glucuronyltransferase, are differentially expressed between compression wood and opposite wood (Li et al., 2013). Therefore, it is speculated that the genetic mutation of Pectate lyase 5 could induce the defect function of pectate lyase and disrupt cell wall metabolism, which leads to asymmetric cell elongation growth and affects the shoot gravitropism of lazy1.
The original contributions presented in the study are publicly available. This data can be found here: National Center for Biotechnology Information (NCBI) BioProject database under accession number, PRJNA007201.
YW conceived the original research plan and designed the experiment. CL, CW, and LW wrote the manuscript, whereas YW reviewed and edited the manuscript. All authors performed the experiments, analyzed the data, and approved the submitted version.
This study was supported by the National Natural Science Foundation of China (32060511 and 32160428), the Natural Science Foundation of Guizhou Province [QKH ZC (2019) 2298], the High-level Innovative Talents Project of Guizhou Province (111/0920008), and the Graduate Education Innovation Project of Guizhou Province [QJH KY Zi (2020) 100].
The authors declare that the research was conducted in the absence of any commercial or financial relationships that could be construed as a potential conflict of interest.
All claims expressed in this article are solely those of the authors and do not necessarily represent those of their affiliated organizations, or those of the publisher, the editors and the reviewers. Any product that may be evaluated in this article, or claim that may be made by its manufacturer, is not guaranteed or endorsed by the publisher.
We thank Beijing Biomarker Technologies Co. Ltd. for providing excellent technical service in RNA-sequencing.
The Supplementary Material for this article can be found online at: https://www.frontiersin.org/articles/10.3389/fpls.2022.815131/full#supplementary-material
Abe, K., Takahashi, H., and Suge, H. (1994). Localization of cells containing sedimented amyloplasts in the shoots of normal and lazy rice seedlings. Biol. Sci. Space 8, 221–225. doi: 10.2187/bss.8.221
Abe, K., Takahashi, H., and Suge, H. (1996). Lazy gene (la) responsible for both an agravitropism of seedlings and lazy habit of tiller growth in rice (Oryza sativa L.). J. Plant Res. 109, 381–386. doi: 10.1007/BF02344553
Abe, K., Takahashi, H., and Suge, H. (2010). Graviresponding sites in shoots of normal and ‘lazy’ rice seedlings. Physiol. Plant. 3, 371–374. doi: 10.1111/j.1399-3054.1994.tb08823.x
Akie, K., Hye-Jeong, K., Yuta, T., Yutaka, M., Nobuharu, F., Sachiko, Y., et al. (2019). Circumnutational movement in rice coleoptiles involves the gravitropic response: analysis of an agravitropic mutant and space grown seedlings. Physiol. Plant. 165, 464–475. doi: 10.1111/ppl.12824
Baker, C. J., Mock, N., Atkinson, M. M., and Hutcheson, S. (1990). Inhibition of the hypersensitive response in tobacco by pectate lyase digests of cell wall and of polygalacturonic acid. Physiol. Mol. Plant Pathol. 37, 155–167. doi: 10.1016/0885-5765(90)90008-L
Bao, Y., Bassham, D. C., and Howell, S. H. (2019). A functional unfolded protein response is required for normal vegetative development. Plant Physiol. 179, 1834–1843. doi: 10.1104/pp.18.01261
Bartkova, J., Lukas, J., Strauss, M., and Bartek, J. (1998). Cyclin D3: requirement for G1/S transition and high abundance in quiescent tissues suggest a dual role in proliferation and differentiation. Oncogene 17, 1027–1037. doi: 10.1038/sj.onc.1202016
Baxter, C. E. L., Costa, M. M. R., and Coen, E. S. (2007). Diversification and co-option of RAD-like genes in the evolution of floral asymmetry. Plant J. 52, 105–113. doi: 10.1111/j.1365-313X.2007.03222.x
Beáta, P., Hana, J., Lucie, K., Věra, C., Žaneta, P., Zuzana, G., et al. (2013). Overexpressed TPX2 causes ectopic formation of microtubular arrays in the nuclei of acentrosomal plant cells. J. Exp. Bot. 64, 4575–4587. doi: 10.1093/jxb/ert271
Benjamins, R. (2003). PINOID-mediated signaling involves calcium-binding proteins. Plant Physiol. 132, 1623–1630. doi: 10.1104/pp.103.019943
Bennett, M. J., Marchant, A., Green, H. G., May, S. T., Ward, S. P., and Millner, P. A. (1996). Arabidopsis AUX1 gene: a permease-like regulator of root gravitropism. Science 273, 948–950. doi: 10.1126/science.273.5277.948
Berthet, S., Demont-Caulet, N., Pollet, B., Bidzinski, P., Cezard, L., Bris, P. L. N., et al. (2011). Disruption of LACCASE4 and 17 results in tissue-specific alterations to lignification of Arabidopsis thaliana stems. Plant Cell 23, 1124–1137. doi: 10.1105/tpc.110.082792
Bischoff, V., Nita, S., Neumetzler, L., Schindelasch, D., Urbain, A., Eshed, R., et al. (2010). TRICHOME BIREFRINGENCE and its homolog AT5G01360 encode plant-specific DUF231 proteins required for cellulose biosynthesis in Arabidopsis. Plant Physiol. 153, 590–602. doi: 10.1104/pp.110.153320
Blancaflor, E. B., and Gilroy, F. S. (1998). Mapping the functional roles of cap cells in the response of Arabidopsis primary roots to gravity. Plant Physiol. 116, 213–222. doi: 10.1104/pp.116.1.213
Bosco, C. D., Dovzhenko, A., Xing, L., Woerner, N., Rensch, T., Eismann, M., et al. (2012). The endoplasmic reticulum localized PIN8 is a pollen: pecific auxin carrier involved in intracellular auxin homeostasis. Plant J. 71, 860–870. doi: 10.1111/j.1365-313X.2012.05037.x
Brown, D., Wightman, R., Zhang, Z., Gomez, L. D., and Turner, S. (2011). Arabidopsis genes IRREGULAR XYLEM (IRX15) and IRX15L encode DUF579-containing proteins that are essential for normal xylan deposition in the secondary cell wall. Plant J. 66, 401–413. doi: 10.1111/j.1365-313X.2011.04501.x
Brunoud, G., Wells, D. M., Oliva, M., Larrieu, A., Mirabet, V., Burrow, A. H., et al. (2012). A novel sensor to map auxin response and distribution at high spatio-temporal resolution. Nature 482, 103–106. doi: 10.1038/nature10791
Bulan, M. T. S., Posner, J. L., Peng, D., Emshwiller, E., Wang, X., and Li, J. (2017). Old crop, new society: persistence and change of Tartary buckwheat farming in Yunnan, China. Hum. Ecol. 45, 37–51. doi: 10.1007/s10745-016-9871-4
Buschmann, H., Hauptmann, M., Niessing, D., Lloyd, C. W., and Schffner, A. R. (2009). Helical growth of the Arabidopsis mutant tortifolia2 does not depend on cell division patterns but involves handed twisting of isolated cells. Plant Cell 21, 2090–2106. doi: 10.1105/tpc.108.061242
Chavez Montes, R. A., Ranocha, P., Martinez, Y., Minic, Z., Jouanin, L., Marquis, M., et al. (2008). Cell wall modifications in Arabidopsis plants with altered alpha-L-arabinofuranosidase activity. Plant Physiol. 147, 63–77. doi: 10.1104/pp.107.110023
Chen, Y., Fan, X., Song, W., Zhang, Y., and Xu, G. (2012). Over-expression of OsPIN2 leads to increased tiller numbers, angle and shorter plant height through suppression of OsLAZY1. Plant Biotechnol. J. 10, 139–149. doi: 10.1111/j.1467-7652.2011.00637.x
Chen, Y., Xu, S., Tian, L., Liu, L., and Wu, G. (2019). The LjLAZY3 gene plays a distinct role in the positive root gravitropism in Lotus japonicus. J. Exp. Bot. 71, 168–177. doi: 10.1093/jxb/erz429
Cosgrove, D. J. (1999). Enzymes and other agents that enhance cell wall extensibility. Annu. Rev. Plant Physiol. Plant Mol. Biol. 50, 391–417. doi: 10.1146/annurev.arplant.50.1.391
Coyne, D. P. (1980). Modification of plant architecture and crop yield by breeding. Hortscience 15, 244–247. doi: 10.1007/BF00023230
Dalal, J., Lewis, D. R., Tietz, O., Brown, E. M., Brown, C. B., Palme, K., et al. (2016). ROSY1, a novel regulator of gravitropic response is a stigmasterol binding protein. J. Plant Physiol. 196-197, 28–40. doi: 10.1016/j.jplph.2016.03.0ll
Digby, J., and Firn, R. D. (2010). The gravitropic set-point angle (GSA): the identification of an important developmentally controlled variable governing plant architecture. Plant Cell Environ. 18, 1434–1440. doi: 10.1111/j.1365-3040.1995.tb00205.x
Dobisova, T., Hrdinova, V., Cuesta, C., Michlickova, S., and Hejatko, J. (2017). Light-regulated expression of sensor histidine kinase CKI1 controls cytokinin-related development. Plant Physiol. 174, 387–404. doi: 10.1104/pp.16.01964
Domingo, C., Roberts, K., Stacey, N. J., Connerton, I., Teran, F. R., and Mccann, M. C. (1998). A pectate lyase from Zinnia elegans is auxin inducible. Plant J. 13, 17–28. doi: 10.1046/j.1365-313X.1998.00002.x
Dong, Z., Jiang, C., Chen, X., Zhang, T., Ding, L., Song, W., et al. (2013). Maize LAZY1 mediates shoot gravitropism and inflorescence development through regulating auxin transport, auxin signaling, and light response. Plant Physiol. 163, 1306–1322. doi: 10.4161/psb.27452
Edelmann, H. G. (2010). Lateral redistribution of auxin is not the means for gravitropic differential growth of coleoptiles: a new model. Physiol. Plant. 112, 119–126. doi: 10.1034/j.1399-3054.2001.1120116.x
Eisa, A., Blter, B., and Schwenkert, S. (2019). The ACT domain in chloroplast precursor-phosphorylating STY kinases binds metabolites and allosterically regulates kinase activity. J. Biol. Chem. 294, 17278–17288. doi: 10.1074/jbc.AAC119.012166
Evans, M. L. (1992). What remains of the Cholodny-Went theory? Plant Cell Environ. 15, 767–768. doi: 10.1105/tpc.4.9.1157
Fernandez, A., Drozdzecki, A., Hoogewijs, K., Nguyen, A., Beeckman, T., and Hilson, M. P. (2013). Transcriptional and functional classification of the GOLVEN/ROOT GROWTH FACTOR/CLE-like signaling peptides reveals their role in lateral root and hair formation. Plant Physiol. 161, 954–970. doi: 10.1104/pp.112.206029
Fernández-Pérez, F., Pomar, F., Pedreo, M. A., and Novo-Uzal, E. (2015). Suppression of Arabidopsis peroxidase 72 alters cell wall and phenylpropanoid metabolism. Plant Sci. 239, 192–199. doi: 10.1016/j.plantsci.2015.08.001
Friml, J. (2003). Auxin transport-shaping the plant. Curr. Opin. Plant Biol. 6, 7–12. doi: 10.1016/S1369526602000031
Fukaki, H., and Tasaka, F. M. (1996). SGR1, SGR2, SGR3: novel genetic loci involved in shoot gravitropism in Arabidopsis thaliana. Plant Physiol. 110, 945–955. doi: 10.1104/pp.110.3.945
Fukaki, H., and Tasaka, M. (1999). Gravity perception and gravitropic response of inflorescence stems in Arabidopsis thaliana. Adv. Space Res. 24, 763–770. doi: 10.1016/s0273-1177(99)00410-x
Fukaki, H., Wysocka-Diller, J., Kato, T., Fujisawa, H., Benfey, P. N., and Tasaka, M. (2002). Genetic evidence that the endodermis is essential for shoot gravitropism in Arabidopsis thaliana. Plant J. 14, 425–430. doi: 10.1046/j.1365-313x.1998.00137.x
Gantulga, D., Turan, Y., Bevan, D. R., and Esen, A. (2008). The Arabidopsis At1g45130 and At3g52840 genes encode beta-galactosidases with activity toward cell wall polysaccharides. Phytochemistry 69, 1661–1670. doi: 10.1016/j.phytochem.2008.01.023
Geisler, M., Wang, B., Zhu, J., and Legué, V. (2014). Auxin transport during root gravitropism: transporters and techniques. Plant Biol. 16, 50–57. doi: 10.1111/plb.12030
Gibson, S. W., and Todd, C. D. (2015). Arabidopsis AIR12 influences root development. Physiol. Mol. Biol. Plants 21, 479–489. doi: 10.1007/s12298-015-0323-1
Godbolé, R., Takahashi, H., and Hertel, R. (1999). The lazy mutation in rice affects a step between statoliths and gravity-induced lateral auxin transport. Plant Biol. 1, 379–381. doi: 10.1111/j.1438-8677.1999.tb00719.x
Grones, P., and Friml, J. (2015). Auxin transporters and binding proteins at a glance. J. Cell Sci. 128, 1–7. doi: 10.1242/jcs.159418
Haga, K., and Sakai, T. (2013). Differential roles of auxin efflux carrier PIN proteins in hypocotyl phototropism of etiolated Arabidopsis seedlings depend on the direction of light stimulus. Plant Signal. Behav. 8:e22556. doi: 10.4161/psb.22556
Hasenstein, K. H., and Kuznetsov, O. A. (1999). The response of lazy-2 tomato seedlings to curvature-inducing magnetic gradients is modulated by light. Planta 208, 59–65. doi: 10.1007/s004250050534
Hashiguchi, Y., Tasaka, M., and Morita, M. T. (2013). Mechanism of higher plant gravity sensing. Am. J. Bot. 100, 91–100. doi: 10.3732/ajb.1200315
Hayashi, T., and Kaida, R. (2011). Functions of xyloglucan in plant cells. Mol. Plant 4, 17–24. doi: 10.1093/mp/ssq063
He, D., Liang, R., Long, T., Yang, Y., and Wu, C. (2021). Rice RBH1 encoding a pectate lyase is critical for apical panicle development. Plants 10:271. doi: 10.3390/plants10020271
Hensel, W. (1987). Cytodifferentiation of polar plant cells: formation and turnover of endoplasmic reticulum in root statocytes. Exp. Cell Res. 172, 377–384. doi: 10.1016/0014-4827(87)90395-8
Herger, A., Gupta, S., Kadler, G., Franck, C. M., and Ringli, C. (2020). Overlapping functions and protein-protein interactions of LRR-extensins in Arabidopsis. PLoS Genet. 16:e1008847. doi: 10.1371/journal.pgen.1008847
Howard, T. P., Hayward, A. P., Tordillos, A., Fragoso, C., Moreno, M. A., Tohme, J., et al. (2014). Identification of the maize gravitropism gene lazy plant1 by a transposon-tagging genome resequencing strategy. PLoS One 9:e87053. doi: 10.1371/journal.pone.0087053
Hur, Y. S., Um, J. H., Kim, S., Kim, K., Park, H. J., and Lim, J. S. (2014). Arabidopsis thaliana homeobox 12 (ATHB12), a homeodomain-leucine zipper protein, regulates leaf growth by promoting cell expansion and endoreduplication. New Phytol. 205, 316–328. doi: 10.1111/nph.12998
Ikushima, T., Soga, K., Hoson, T., and Shimmen, T. (2008). Role of xyloglucan in gravitropic bending of azuki bean epicotyl. Physiol. Plant. 132, 552–565. doi: 10.1111/j.1399-3054.2007.01047.x
Iversen, T. H., and Larsen, P. (2010). Movement of amyloplasts in the statocytes of geotropically stimulated roots. The pre-inversion effect. Physiol. Plant. 28, 172–181. doi: 10.1111/j.1399-3054.1973.tb01171.x
Jenkins, M., and Gerhardt, F. (1931). A gene influencing the composition of the culm in maize. Iowa Agic. Exp. Sta. Res. Bull. 138, 121–151.
Jones, J. W., and Adair, C. R. (1938). A “LAZY” mutation in rice. J. Hered. 8, 315–318. doi: 10.1093/oxfordjournals.jhered.a104527
Kalinová, J. P., Vrchotová, N., and Tříska, J. (2018). Contribution to the study of rutin stability in the achenes of Tartary Buckwheat (Fagopyrum tataricum). Food Chem. 258, 314–320. doi: 10.1016/j.foodchem.2018.03.090
Kanamoto, H., Jun-Ichiro, H., Takemura, M., Yokota, A., and Kohchi, T. (2002). Molecular cloning and characterization of a gene coding for a putative receptor-like protein kinase with a leucine-rich repeat expressed in inflorescence and root apices from Arabidopsis. Plant Biotechnol. 19, 113–120. doi: 10.5511/plantbiotechnology.19.113
Kataoka, T., Hayashi, N., Yamaya, T., and Hideki, T. (2004). Root-to-shoot transport of sulfate in Arabidopsis. Evidence for the role of SULTR3;5 as a component of low-affinity sulfate transport system in the root vasculature. Plant Physiol. 136, 4198–4204. doi: 10.1104/pp.104.045625
Kato, T., Morita, M. T., Fukaki, H., Yamauchi, Y., Uehara, M., and Tasaka, N. M. (2002). SGR2, a Phospholipase-Like protein, and ZIG/SGR4, a SNARE, are involved in the shoot gravitropism of Arabidopsis. Plant Cell 14, 33–46. doi: 10.1105/tpc.010215
Knox, J. P., Linstead, P. J., King, J., Cooper, C., and Roberts, K. (1990). Pectin esterification is spatially regulated both within cell walls and between developing tissues of root apices. Planta 181, 512–521. doi: 10.1007/BF00193004
Kobayashi, K., Suzuki, T., Iwata, E., Nakamichi, N., Suzuki, T., Chen, P., et al. (2015). Transcriptional repression by MYB3R proteins regulates plant organ growth. EMBO J. 34, 1992–2007. doi: 10.15252/embj.201490899
Kumar, S., Yoshizumi, T., Hongo, H., Yone Da, A., Hara, H., Hamasaki, H., et al. (2012). Arabidopsis mitochondrial protein TIM50 affects hypocotyl cell elongation through intracellular ATP level. Plant Sci. 183, 212–217. doi: 10.1016/j.plantsci.2011.08.014
Li, B. (2007). LAZY1 controls rice shoot gravitropism through regulating polar auxin transport. Cell Res. 17, 402–410. doi: 10.1038/cr.2007.38
Li, X., Yang, X., and Wu, H. X. (2013). Transcriptome profiling of Radiata pine branches reveals new insights into reaction wood formation with implications in plant gravitropism. BMC Genomics 14:768. doi: 10.1186/1471-2164-14-768
Li, Z., Liang, Y., Yuan, Y., Wang, L., and Wang, Y. (2019). OsBRXL4 regulates shoot gravitropism and rice tiller angle through affecting LAZY1 nuclear localization. Mol. Plant 12, 1143–1156. doi: 10.1016/j.molp.2019.05.014
Liam, C., and Turner, S. R. (2017). A comprehensive analysis of RALF proteins in green plants suggests there are two distinct functional groups. Front. Plant Sci. 8:37. doi: 10.3389/fpls.2017.00037
Liepman, A. H., Wilkerson, C. G., and Keegstra, K. (2005). Expression of cellulose synthase-like (Csl) genes in insect cells reveals that CslA family members encode mannan synthases. Proc. Natl. Acad. Sci. U.S.A. 10, 2221–2226. doi: 10.1073/pnas.0409179102
Lim, C. K. (2012). The Function of Ascorbate Oxidase in Arabidopsis thaliana. Exeter: University of Exeter.
Lin, M., and Li, G. (2018). FAR1-RELATED SEQUENCE (FRS) and FRS-RELATED FACTOR (FRF) family proteins in Arabidopsis growth and development. Front. Plant Sci. 7:9692. doi: 10.3389/fpls.2018.00692
Liu, L., Dean, J. D. F., Friedman, W. E., and Eriksson, K. E. L. (1994). A laccase-like phenoloxidase is correlated with lignin biosynthesis in Zinnia elegans stem tissues. Plant J. 6, 213–224. doi: 10.1046/j.1365-313X.1994.6020213.x
Long, J. C., Zhao, W., Rashotte, A. M., Muday, G. K., and Huber, S. C. (2002). Gravity-stimulated changes in auxin and invertase gene expression in maize pulvinal cells. Plant Physiol. 128, 591–602. doi: 10.1104/pp.010579
Luo, X., Fei, Y., Xu, Q., Lei, T., Mo, X., Wang, Z., et al. (2020). Isolation and identification of antioxidant peptides from Tartary buckwheat albumin (Fagopyrum tataricum Gaertn.) and their antioxidant activities. J. Food Sci. 85, 611–617. doi: 10.1111/1750-3841.15004
Mandadi, K. K., Misra, A., Ren, S., and McKnight, T. D. (2009). BT2, a BTB protein, mediates multiple responses to nutrients, stresses, and hormones in Arabidopsis. Plant Physiol. 150, 1930–1939. doi: 10.1104/pp.109.139220
Mandel, M. A., and Yanofsky, M. F. (1995). The Arabidopsis AGL8 MADS box gene is expressed in inflorescence meristems and is negatively regulated by APETALA1. Plant Cell 7, 1763–1771. doi: 10.2307/3870185
Marc, S., Filip, V., Alexander, I., Norma, F., Colin, R., Kris, V., et al. (2021). Brassinosteroids influence arabidopsis hypocotyl graviresponses through changes in mannans and cellulose. Plant Cell Physiol. 4, 678–692. doi: 10.1093/pcp/pcab024
Mei, Y., Jia, W. J., Chu, Y. J., and Xue, H. W. (2012). Arabidopsis phosphatidylinositol monophosphate 5-kinase 2 is involved in root gravitropism through regulation of polar auxin transport by affecting the cycling of PIN proteins. Cell Res. 22, 581–597. doi: 10.1038/cr.2011.150
Monshausen, G. B., Miller, N. D., Murphy, A. S., and Gilroy, S. (2011). Dynamics of auxin-dependent Ca2+ and pH signaling in root growth revealed by integrating high-resolution imaging with automated computer vision-based analysis. Plant J. 65, 309–318. doi: 10.1111/j.1365-313X.2010.04423.x
Moritoshi, I. (1995). Gravitropism and phototropism of maize coleoptiles: evaluation of the Cholodny-Went Theory through effects of auxin application and decapitation. Plant Cell Physiol. 2, 361–367. doi: 10.1093/oxfordjournals.pcp.a078768
Nakamura, M., Toyota, M., and Morita, T. (2011). An Arabidopsis E3 ligase, SHOOT GRAVITROPISM9, modulates the interaction between statoliths and F-Actin in gravity sensing. Plant Cell 23, 1830–1848. doi: 10.1105/tpc.110.079442
Naoki, S., and Yoshio, M. (1978). Auxin-induced extension, cell wall loosening and changes in the wall polysaccharide content of barley coleoptile segments. Plant Cell Physiol. 7, 1225–1233. doi: 10.1093/oxfordjournals.pcp.a075703
Nziengui, H., Lasok, H., Kochersperger, P., Ruperti, A., and Rébeillé, B. (2018). Root gravitropism is regulated by a crosstalk between para-aminobenzoic acid, ethylene, and auxin. Plant Physiol. 178, 1370–1389. doi: 10.1104/pp.18.00126
Okuyama, T., Takeda, H., Yamamoto, H., and Yoshida, M. (1998). Relation between growth stress and lignin concentration in the cell wall: ultraviolet microscopic spectral analysis. J. Wood Sci. 44, 83–89. doi: 10.1007/BF00526250
Ouakfaoui, S. E., Schnell, J., Abdeen, A., Colville, A., Labbé, H., Han, S., et al. (2010). Control of somatic embryogenesis and embryo development by AP2 transcription factors. Plant Mol. Biol. 74, 313–326. doi: 10.1007/s11103-010-9674-8
Palusa, S. G., Golovkin, M., Shin, S. B., Richardson, D. N., and Reddy, A. S. N. (2007). Organ-specific, developmental, hormonal and stress regulation of expression of putative pectate lyase genes in Arabidopsis. New Phytol. 174, 537–550. doi: 10.1111/j.1469-8137.2007.02033.x
Peaucelle, A., Braybrook, S., Le Guillou, L., Bron, E., Kuhlemeier, C., and Höfte, H. (2011). Pectin-induced changes in cell wall mechanics underlie organ initiation in Arabidopsis. Curr. Biol. 21, 1720–1726. doi: 10.1016/j.cub.2011.08.057
Perera, Y. I. (2006). A universal role for inositol 1,4,5-trisphosphate-mediated signaling in plant gravitropism. Plant Physiol. 140, 746–760. doi: 10.1104/pp.105.075119
Randall, R. S., Miyashima, S., Blomster, T., Zhang, J., Elo, A., Karlberg, A., et al. (2015). AINTEGUMENTA and the D-type cyclin CYCD3;1 regulate root secondary growth and respond to cytokinins. Biol. Open 4, 1229–1236. doi: 10.1242/bio.013128
Ranocha, P., Denancé, N., Vanholme, R., Freydier, A., Martinez, Y., Hoffmann, L., et al. (2010). Walls are thin1 (WAT1), an Arabidopsis homolog of Medicago truncatula NODULIN21, is a tonoplast-localized protein required for secondary wall formation in fibers. Plant J. 63, 469–483. doi: 10.1111/j.1365-313X.2010.04256.x
Rigo, G., Ayaydin, F., Tietz, O., Zsigmond, L., Kovacs, H., Pay, A., et al. (2013). Inactivation of plasma membrane-localized CDPK-RELATED KINASE5 decelerates PIN2 exocytosis and root gravitropic response in Arabidopsis. Plant Cell 25, 1592–1608. doi: 10.1105/tpc.113.110452
Robert, H. S., Quint, A., Brand, D., Smith, A. V., and Offringa, R. (2009). BTB and TAZ domain scaffold proteins perform a crucial function in Arabidopsis development. Plant J. 58, 109–121. doi: 10.1111/j.1365-313X.2008.03764.x
Roberts, J. A. (1984). Tropic responses of hypocotyls from normal tomato plants and the gravitropic mutant Lazy-1. Plant Cell Environ. 7, 515–520. doi: 10.1111/1365-3040.ep11616210
Rodrigo, G., Jaramillo, A., and Blázquez, M. A. (2011). Integral control of plant gravitropism through the interplay of hormone signaling and gene regulation. Biophys. J. 101, 757–763. doi: 10.1016/j.bpj.2011.06.047
Rodrigues-Pousada, R. A., Rycke, R. D., Dedonder, A., Caeneghem, W. V., and Straeten, D. (1993). The Arabidopsis 1-aminocyclopropane-1-carboxylate synthase gene 1 is expressed during early development. Plant Cell 5, 897–911. doi: 10.2307/3869658
Rood, S., Abe, H., and Pharis, R. (1987). Gibberellins and gravitropism in maize shoots: endogenous gibberellin-like substances and movement and metabolism of [3H]Gibberellin A20. Plant Physiol. 83, 645–651. doi: 10.1104/pp.83.3.645
Ryser, U., Schorderet, M., Zhao, G. A., Studer, D., and Keller, B. (1997). Structural cell-wall proteins in protoxylem development: evidence for a repair process mediated by a glycine-rich protein. Plant J. 12, 97–111. doi: 10.1046/j.1365-313X.1997.12010097.x
Ryusuke, Y., and Kazuhiko, N. (2001). A comprehensive expression analysis of all members of a gene family encoding cell-wall enzymes allowed us to predict cis-regulatory regions involved in cell-wall construction in specific organs of Arabidopsis. Plant Cell Physiol. 42, 1025–1033. doi: 10.1093/pcp/pce154
Salisbury, F. B., Gillespie, L., and Rorabaugh, P. (1988). Gravitropism in higher plant shoots. V. Changing sensitivity to auxin. Plant Physiol. 88, 1186–1194. doi: 10.1104/pp.88.4.1186
Seyfferth, C., Wessels, B., Jokipii-Lukkari, S., Sundberg, B., Delhomme, N., Felten, J., et al. (2018). Ethylene-related gene expression networks in wood formation. Front. Plant Sci. 9:272. doi: 10.3389/fpls.2018.00272
Sun, H., Hao, P., Ma, Q., Zhang, M., Qin, Y., Wei, H., et al. (2018). Genome-wide identification and expression analyses of the pectate lyase (PEL) gene family in cotton (Gossypium hirsutum L.). BMC Genomics 19:661. doi: 10.1186/s12864-018-5047-5
Ta-Fang, L., Shunsuke, S., Mitsutomo, A., Thomas, L., and Hector, C. (2016). OBE3 and WUS interaction in shoot meristem stem cell regulation. PLoS One 11:e0155657. doi: 10.1371/journal.pone.0155657
Taiz, S. (1984). Plant cell expansion: regulation of cell wall mechanical properties. Annu. Rev. Plant Physiol. 35, 585–657. doi: 10.1146/annurev.pp.35.060184.003101
Takeshi, Y., and Moritoshi, I. (2007). Identification of the gravitropism-related rice gene LAZY1 and elucidation of LAZY1-dependent and -independent gravity signaling pathways. Plant Cell Physiol. 48, 678–688. doi: 10.1093/pcp/pcm042
Tanaka, H., Ishikawa, M., Kitamura, S., Takahashi, Y., Soyano, T., Machida, C., et al. (2004). The AtNACK1/HINKEL and STUD/TETRASPORE/AtNACK2 genes, which encode functionally redundant kinesins, are essential for cytokinesis in Arabidopsis. Genes Cells 9, 1199–1211. doi: 10.1111/j.1365-2443.2004.00798.x
Taniguchi, M., Furutani, M., Nishimura, T., Nakamura, M., Fushita, T., Iijima, K., et al. (2017). The Arabidopsis LAZY1 family plays a key role in gravity signaling within statocytes and in branch angle control of roots and shoots. Plant Cell 29, 1984–1999. doi: 10.1105/tpc.16.00575
Thoma, S., Hecht, U., Kippers, A., Botella, J., and Somerville, V. C. (1994). tissue-specific expression of a gene encoding a cell wall-localized lipid transfer protein from Arabidopsis. Plant Physiol. 105, 35–45. doi: 10.1104/pp.105.1.35
Tokiwa, H., Hasegawa, T., Yamada, K., Shigemori, H., and Hasegawa, K. (2006). A major factor in gravitropism in radish hypocotyls is the suppression of growth on the upper side of hypocotyls. J. Plant Physiol. 163, 1267–1272. doi: 10.1016/j.jplph.2005.09.006
Toyota, M., Ikeda, N., Sawai-Toyota, S., Kato, T., and Morita, M. T. (2013). Amyloplast displacement is necessary for gravisensing in Arabidopsis shoots as revealed by a centrifuge microscope. Plant J. 76, 648–660. doi: 10.1111/tpj.12324
Türkan, I., and Suge, H. (1991). Survey of endogenous gibberellins in a barley mutant showing abnormal response to gravity. Jpn. J. Genet. 66, 41–48. doi: 10.1266/jjg.66.41
Urbina, D. C., Silva, H., and Meisel, L. A. (2006). The Ca2+ pump inhibitor, thapsigargin, inhibits root gravitropism in Arabidopsis thaliana. Biol. Res. 39, 289–296. doi: 10.4067/S0716-97602006000200011
Van, O. J. (1936). LAZY,” AN A-GEOTROPIC FORM OF MAIZE: gravitational indifference” rather than structural weakness accounts for prostrate growth-habit of this form. J. Hered. 27, 93–96. doi: 10.1093/oxfordjournals.jhered.a104191
Venbrinkab, J. P., and Kiss, J. Z. (2019). Plant responses to gravity. Semin. Cell Dev. Biol. 92, 122–125. doi: 10.1016/j.semcdb.2019.03.011
Wang, S., Yamaguchi, M., Grienenberger, E., Martone, P. T., Samuels, A. L., and Mansfield, S. D. (2020). The Class II KNOX genes KNAT3 and KNAT7 work cooperatively to influence deposition of secondary cell walls that provide mechanical support to Arabidopsis stems. Plant J. 101, 293–309. doi: 10.1111/tpj.14541
Wieczorek, K., Elashry, A., Quentin, M., Grundler, F., Favery, B., Seifert, G. J., et al. (2014). A distinct role of pectate lyases in the formation of feeding structures induced by cyst and root-knot nematodes. Mol. Plant Microbe Interact. 27, 901–912. doi: 10.1094/MPMI-01-14-0005-R
Xu, Y., Zhao, X., Aiwaili, P., Mu, X., and Hong, B. (2020). A zinc finger protein BBX19 interacts with ABF3 to negatively affect drought tolerance in chrysanthemum. Plant J. 103, 1783–1795. doi: 10.1111/tpj.14863
Yamaoka, T., and Chiba, N. (1983). Changes in the coagulating ability of pectin during the growth of soybean hypocotyls. Plant Cell Physiol. 24, 1281–1290. doi: 10.1094/Phyto-73-1674
Yang, Z., Feng, S., Tang, D., Zhang, L., and Zhang, C. (2020). The mutation of a pectate lyase-like gene is responsible for the yellow margin phenotype in potato. Theor. Appl. Genet. 133, 1123–1131. doi: 10.1007/s00122-020-03536-w
Yano, D., Sato, M., Saito, C., Sato, M. H., Morita, M. T., and Tasaka, M. (2003). A SNARE complex containing SGR3/AtVAM3 and ZIG/VTI11 in gravity-sensing cells is important for Arabidopsis shoot gravitropism. Proc. Natl. Acad. Sci. U.S.A. 100, 8589–8594. doi: 10.1073/pnas.1430749100
Yin, W., Dong, N., Niu, M., Zhang, X., Li, L., Liu, J., et al. (2019). Brassinosteroid-regulated plant growth and development and gene expression in soybean. Crop J. 7, 411–418. doi: 10.1016/j.cj.2018.10.003
Yoshiro, Y., Hidehiro, F., Hisao, F., and Masao, T. (1997). Mutations in the SGR4, SGR5 and SGR6 loci of Arabidopsis thaliana alter the shoot gravitropism. Plant Cell Physiol. 38, 530–535. doi: 10.1093/oxfordjournals.pcp.a029201
Žádníková, P., Wabnik, K., Abuzeineh, A., Gallemi, M., Straeten, D. V. D., Smith, R. S., et al. (2016). A model of differential growth-guided apical hook formation in plants. Plant Cell 28, 2464–2477. doi: 10.1105/tpc.15.00569
Zhang, S. W., Chen, H. L., Zhang, S. Q., Xia, Y. F., Xia, Y. F., Sun, D. Y., et al. (2009). Altered architecture and enhanced drought tolerance in rice via the down-regulation of indole-3-acetic acid by TLD1/OsGH3.13 activation. Plant Physiol. 1889–1901. doi: 10.1104/pp.109.146803
Zhang, X. Y., Chen, J., Li, X. L., Yi, K., Ye, Y., Liu, G., et al. (2017). Dynamic changes in antioxidant activity and biochemical composition of tartary buckwheat leaves during Aspergillus niger fermentation. J. Funct. Foods 32, 375–381. doi: 10.1016/j.jff.2017.03.022
Zhao, S. Q., Hu, J., Guo, L. B., Qian, Q., and Xue, H. W. (2010). Rice leaf inclination2, a VIN3-1ike protein, regulates leaf angle through modulating cell division of the collar. Cell Res. 20, 935–947. doi: 10.1038/cr.2010.109
Keywords: gravitropism, Pectate lyase 5, hormone, cellular morphology, transcriptome
Citation: Liang C, Wei C, Wang L, Guan Z, Shi T, Huang J, Li B, Lu Y, Liu H and Wang Y (2022) Characterization of a Novel Creeping Tartary Buckwheat (Fagopyrum tataricum) Mutant lazy1. Front. Plant Sci. 13:815131. doi: 10.3389/fpls.2022.815131
Received: 15 November 2021; Accepted: 02 March 2022;
Published: 27 April 2022.
Edited by:
Jinshun Zhong, South China Agricultural University, ChinaReviewed by:
Sarah E. Wyatt, Ohio University, United StatesCopyright © 2022 Liang, Wei, Wang, Guan, Shi, Huang, Li, Lu, Liu and Wang. This is an open-access article distributed under the terms of the Creative Commons Attribution License (CC BY). The use, distribution or reproduction in other forums is permitted, provided the original author(s) and the copyright owner(s) are credited and that the original publication in this journal is cited, in accordance with accepted academic practice. No use, distribution or reproduction is permitted which does not comply with these terms.
*Correspondence: Yan Wang, MjAxNjA2MDAyQGd6bnUuZWR1LmNu
†These authors have contributed equally to this work and share first authorship
Disclaimer: All claims expressed in this article are solely those of the authors and do not necessarily represent those of their affiliated organizations, or those of the publisher, the editors and the reviewers. Any product that may be evaluated in this article or claim that may be made by its manufacturer is not guaranteed or endorsed by the publisher.
Research integrity at Frontiers
Learn more about the work of our research integrity team to safeguard the quality of each article we publish.