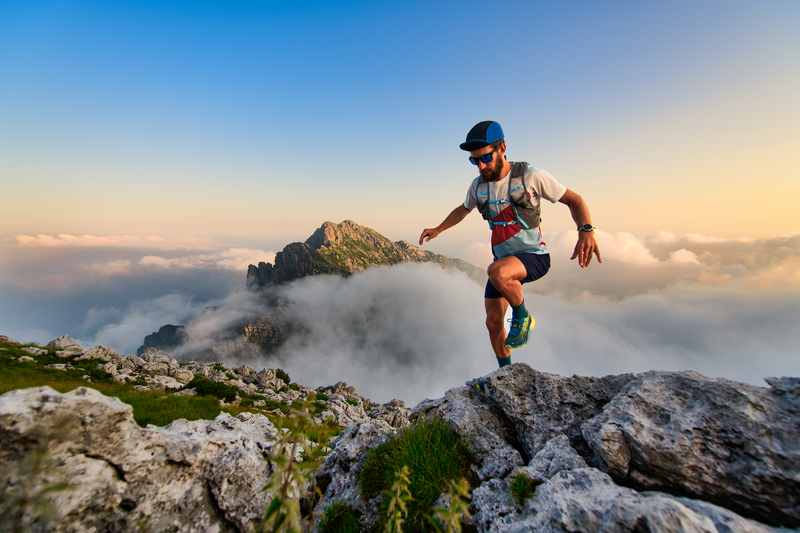
95% of researchers rate our articles as excellent or good
Learn more about the work of our research integrity team to safeguard the quality of each article we publish.
Find out more
REVIEW article
Front. Plant Sci. , 07 April 2022
Sec. Plant Abiotic Stress
Volume 13 - 2022 | https://doi.org/10.3389/fpls.2022.781524
This article is part of the Research Topic Harnessing Resilience of Orphan Crops to the Effects of Drought and Heat: A Route Towards a Sustainable Agriculture View all 5 articles
Pearl millet [Pennisetum glaucum (L.) R. Br.] is a C4 crop cultivated for its grain and stover in crop-livestock-based rain-fed farming systems of tropics and subtropics in the Indian subcontinent and sub-Saharan Africa. The intensity of drought is predicted to further exacerbate because of looming climate change, necessitating greater focus on pearl millet breeding for drought tolerance. The nature of drought in different target populations of pearl millet-growing environments (TPEs) is highly variable in its timing, intensity, and duration. Pearl millet response to drought in various growth stages has been studied comprehensively. Dissection of drought tolerance physiology and phenology has helped in understanding the yield formation process under drought conditions. The overall understanding of TPEs and differential sensitivity of various growth stages to water stress helped to identify target traits for manipulation through breeding for drought tolerance. Recent advancement in high-throughput phenotyping platforms has made it more realistic to screen large populations/germplasm for drought-adaptive traits. The role of adapted germplasm has been emphasized for drought breeding, as the measured performance under drought stress is largely an outcome of adaptation to stress environments. Hybridization of adapted landraces with selected elite genetic material has been stated to amalgamate adaptation and productivity. Substantial progress has been made in the development of genomic resources that have been used to explore genetic diversity, linkage mapping (QTLs), marker-trait association (MTA), and genomic selection (GS) in pearl millet. High-throughput genotyping (HTPG) platforms are now available at a low cost, offering enormous opportunities to apply markers assisted selection (MAS) in conventional breeding programs targeting drought tolerance. Next-generation sequencing (NGS) technology, micro-environmental modeling, and pearl millet whole genome re-sequence information covering circa 1,000 wild and cultivated accessions have helped to greater understand germplasm, genomes, candidate genes, and markers. Their application in molecular breeding would lead to the development of high-yielding and drought-tolerant pearl millet cultivars. This review examines how the strategic use of genetic resources, modern genomics, molecular biology, and shuttle breeding can further enhance the development and delivery of drought-tolerant cultivars.
Pearl millet [Pennisetum glaucum (L.) R. Br. syn. Cenchrus americanus L.] has been widely grown in Sahelian West Africa and the Indian subcontinent since time immemorial. Archeological evidence (Manning et al., 2011) as well as modern genome sequence analysis (Burgarella et al., 2018) indicated that pearl millet originated and was domesticated in West Africa about 4,000 to 5,000 years ago. Pearl millet is grown in an agriculturally harsh environment where other staple crops are likely to fail in producing a reasonable grain yield. Pearl millet is a momentous food crop around the globe after maize (Zea mays), wheat (Triticum aestivum), rice (Oryza sativa), sorghum (Sorghum bicolar), and barley (Hordeum vulgare). It is an important eco-friendly field crop in conventional farming, which is good for human nutrition and health, and the planet. Pearl millet may counter several aversive impacts of looming climate change on food grain production better than other staple cereals and plays a significant role in food and nutritional security. To enhance genetic gains in terms of grain yield, nutritional values, climate resilience, and multiple disease resistance, constant efforts will be needed to develop more potential genomic resources for the pearl millet species (Srivastava et al., 2020a).
Usually, pearl millet is grown not only for nutritious grains but also for grazing, silage, hay, green chop, poultry feed, dry stover, and bio-energy. Pearl millet grains have been consumed as a staple food for thousands of years to make a diversity of food stuffs, and continue to be used as a staple grain by millions of people in African and Asian countries. Pearl millet is a perfect alternative for low-input sustainable agricultural systems. Regardless of its lower productivity owing to various environmental stresses (terminal drought and flowering-stage heat) and biotic constraints (diseases like downy mildew, blast, rust, ergot, and smut), pearl millet produces nutritive grains, which are a resource for addressing malnutrition among the poor in marginal areas of the world (Rai et al., 2013).
Pearl millet has a very efficient energy production system (C4 photosynthesis) for rapid production of biomass that partly has enabled it to adapt to suboptimal arid and semi-arid environments around the world (Wang et al., 2012). The high degree of tolerance to high temperature during the early and reproductive stages also makes it a promising genetic resource for isolation of candidate genes governing adaptation to suboptimal growing conditions (Gupta et al., 2015). High tillering capacity and cross-pollination that produces heterogeneous plants contributed in its resilience to hot and dry environments (Kumar and Andrews, 1993). Pearl millet tillers freely and compensates for any stand irregularity due to early drought, and it produces several heads per plant depending on moisture availability. Today, pearl millet is grown in over 30 million hectares of land and supports more than 100 million people as a staple food, and different forms are found in the Sahel extending from Senegal to Sudan (Brunken, 1977).
Limited soil moisture content is one of the key environmental stresses affecting grain yield and nutritional quality. The looming climate change is expected to make environmental stresses even more aggravated (Kholová et al., 2010a). Hence, sustainable food and nutritional security will rely on breeding cultivars with higher adaptation traits in water-limited environments. Several efforts have been attempted to enhance water use efficiency in dry lands, and have resulted in significant improvements in adaptation to drought stress in pearl millet cultivars (Yadav et al., 2002; 2004; 2011; Serraj et al., 2005; Bidinger et al., 2007; Vadez et al., 2012; Varshney et al., 2017). Genetic improvement in several key traits has lagged behind other staple cereals, but there is good potential to enhance pearl millet resilience to environmental stresses. Availability of the pearl millet whole-genome sequence (Varshney et al., 2017) paves the way to exploit its wide genetic variability to develop cultivars and hybrids well suited to present and environmental stresses (Debieu et al., 2017). This review attempts to assess the progress made in mapping of drought environments in the pearl millet growing ecology, understand the response of pearl millet to various types of drought, comprehend the mechanism of pearl millet drought adaptation, scrutinize procedures and advanced techniques to dissect drought tolerance, and assess the role of genomic resources in breeding for drought tolerance. We contend that understanding these aspects would greatly advance the development of drought-tolerant pearl millet cultivars at a faster rate.
The future climate will likely see increased drought frequency, varied precipitation patterns, and unpredictability of monsoon rains, which can cause drought in different stages of plant growth in different environments. It will make the breeding process even more difficult if studies on traits that drive adaptation are not growth stage-specific and well-characterized for each stage. Accordingly, cost-effective phenotyping of identified traits can be meticulously integrated with pearl millet breeding programs focusing on environment-specific strategy.
Seedling stage drought is prominent in crop production regions receiving rainfall lower than 400 mm per annum such as Thar desert (Western Rajasthan, India) where seedling establishment itself is a major challenge (Howarth et al., 1996). Most breeding programs fail to deliver products for drier regions mainly because of narrow genetic variations for seedling establishment and vast variations in microclimate (day and night temperature variations and humidity) and water-holding capacity of the soil; apart from rainfall, which requires proper quantification. Early season soil moisture stress results in seedling mortality, leading to poor crop establishment and reduced yield in pearl millet (Carberry et al., 1985; Yadav et al., 2012a). Genetic variation has been reported for root and shoot length and its ratio, and leaf formation and secondary root development in early season moisture stress (Gregory, 1983; Govindaraj et al., 2010; Shivhare and Lata, 2019; Mukesh Sankar et al., 2021). Agronomic management plays very little role in reducing seedling mortality, but it was reported that early seedling vigor along with larger seed size could enhance crop stand establishment contributing to drought adaptation (Howarth et al., 1996).
Drought stress in the vegetative stage is of frequent occurrence and seriously affects pearl millet growth (Kholová et al., 2010a; Medina et al., 2017; Shivhare and Lata, 2017), tillering ability (van Oosterom et al., 2003, 2006), and flowering (Bidinger et al., 1987a; Mahalakshmi et al., 1987), which are important components of crop productivity. The high-tillering nature of pearl millet compensates for loss of panicles in the main shoot under intermittent drought provided that water is available at a later season. This feature helps pearl millet to adapt to drought as it takes advantage of late rain to produce additional productive tillers (Siband, 1983; Mahalakshmi et al., 1987). Also, early flowering is an important attribute of pearl millet for drought escape, however, constraints in predicting rainfall events in drier regions limit the breeding process for early flowering traits (Vadez et al., 2012).
Soil moisture stress in the flowering and post-flowering stages are common because of early cessation of rainfall in the growing season. In fact, collectively, terminal drought stress accounts for a huge impact on pearl millet grain and stover yields (Mahalakshmi et al., 1987; Winkel et al., 1997; Bidinger and Hash, 2004; Kholová and Vadez, 2013). Grain yield penalty is maximum when drought strikes during the flowering and grain filling stages of the crop (Lahiri and Kumar, 1966; Mahalakshmi et al., 1987) owing to decrease in the number of fertile florets per panicle and grain size in pearl millet (Bidinger et al., 1987a; Fussell et al., 1991). Reduction in grain size is mainly due to shortening of the period for grain filling rather than reduction in the rate of grain growth (Henson et al., 1984; Bieler et al., 1993), as pearl millet also has an exceptional capacity to compensate for reduction in the supply of assimilates to the grains by mobilizing stored soluble sugars in stems and leaves (Fussell et al., 1980). The contribution of stored assimilates to grain growth during drought stress has, however, not been quantified (Yadav et al., 2012c). Connection between grain development and transfer of assimilates from the leaves has been reported as the central adaptation mechanism in pearl millet to terminal drought stress (Winkel and Do, 1992). However, more recent evidence shows that pearl millet adaptation to terminal drought stress depends on soil moisture availability during the grain-filling stage. Therefore, water-saving mechanisms like transpiration efficiency, restricted transpiration during high atmospheric demand, and leaf expansion at a lower threshold of soil moisture can sustain photosynthesis for continued carbon supply to the grains during the critical period (Vadez et al., 2013; Choudhary et al., 2019).
It has been convincingly shown that the effects of drought are influenced by the developmental stage of the crop in which drought stress occurs, which is environment-specific. Therefore, the development of drought-tolerant products requires proper quantification of the environment and then fit-for-purpose product development through innovative breeding.
Grain yield is a complex process influenced by several component traits at the bottom of plant structural organization and is the consequence of interaction among the environment, management, and genotype. Below are several physiologically important traits reported to play a critical role in drought adaptation of pearl millet.
Availability of an adequate amount of water during critical growth stages determines the productivity of crop plants. Terminal drought-adapted genotypes have built-in plant traits such as lower transpiration rate (Figure 1) and lower water extraction pattern (Figure 2) that promote conservation of soil water in early growth stages to allow for more water to be available for effective grain filling during terminal drought (Vadez et al., 2013). There is a significant relationship between transpiration rate and soil moisture content observed in pearl millet (Kholová et al., 2010a).
Figure 1. Pearl millet genotypes contrasting for drought adaptation were shown to differ in their transpiration response to increase in vapor pressure deficit (VPD, adopted from Kholová et al., 2010b).
Figure 2. Water extraction pattern in H77 and PRLT before and after flowering under water deficit conditions (adopted from Vadez et al., 2013).
Water extraction pattern in critical crop growth stages: the pattern of water extraction in pearl millet has been explored using the lysimetric facility simulating field conditions (Vadez et al., 2013). When irrigation was withheld in the late vegetative stage, the drought-adapted genotype PRLT-2/89-33 showed less water extraction before flowering, and conserved soil moisture was hypothesized to support grain filling, whereas the drought-sensitive genotype H77/833-2 extracted more water before flowering, and depleted soil moisture could not support grain filling (Tharanya et al., 2017).
Plants do suffer from a type of stress called atmospheric drought when the evaporative demand (vapor pressure deficit, VPD) of the environment is high especially under midday conditions irrespective of soil moisture status. During high VPD conditions, transpirational water loss is higher, and photosynthetic efficiency is low; in other words, plants spend a lot of water molecules in transpirational cooling with very minimum CO2 fixation (Figure 3). Limited TR under increasing atmospheric VPD was identified as one of the water-saving mechanisms and genotypic variations were reported in pearl millet (Kholová et al., 2010b; Medina et al., 2017). The limited TR trait in pearl millet operates by hydraulic regulation and partial closure of stomata contributing to water conservation. Drought-adapted genotypes have lower transpiration rates at high atmospheric evaporative demand than terminal drought-sensitive lines (Kholová et al., 2010a,b; Medina et al., 2017; Tharanya et al., 2018; Choudhary et al., 2019). The genotypes contrasting for transpiration rate under elevated VPD condition was found to differ in their dependence on water channeling pathway mediated through aquaporins which play an important role in hydraulic regulation (Tharanya et al., 2018).
Figure 3. Genotypic difference in transpiration response of H77/833-2 (high Tr, open circle) and PRLT-2/89-33 (low Tr, closed circle) to AQP and apoplastic inhibition (adopted from Tharanya et al., 2018).
Canopy development traits are closely associated with crop water use (Tharanya et al., 2018) and are a combination of vigor and size (Vadez et al., 2015). Vadez et al. (2015) looked at the difference in canopy development of pearl millet hybrids bred for different agro-ecological zones of India and hypothesized that these hybrids may have a difference in canopy development resulting in variation in leaf area. F1 hybrids developed for the A1 dry zone produced smaller leaf areas and lower biomass than materials bred for the relatively wetter A and B zones. These differences in canopy development were also reported to result in water conservation under water deficit conditions (Vadez et al., 2015; Choudhary et al., 2019), which had a genetic basis (Yadav et al., 2005; Kholová et al., 2012; Tharanya et al., 2018).
Transpiration is known to be regulated by a combination of hydraulic signals and biochemical mediators like abscisic acid (ABA) (Comstock, 2002). It is postulated that although higher production of ABA may decrease stomatal conductance, it can increase root hydraulic conductivity (Thompson et al., 2007). Grantz (1990) reported that leaf stomatal closure at high evaporative demand from the atmosphere could be mediated by ABA. Under well-watered conditions, the drought-adapted pearl millet genotype PRLT-2/89-33 was found to have high leaf ABA during the vegetative stage.
The interaction of genotypes with the environment restrains genetic gain and insights into drought adaptation. Therefore, it is important to characterize the environment in which a crop is grown. A few decades back, the All-India Coordinated Pearl Millet Improvement Project (AICPMIP) defined the pearl millet growing area of the country into three separate zones, A1 (the arid zone of NW India), A (the higher rainfall areas of north and central India), and B (semi-arid peninsular India). Each of these zones covers areas with high variation in agro-climatic conditions that have, in these decades, also changed dramatically. Recognizing this current variation in existing zones redefining pearl millet-growing area and identifying crop production constraints (drought stress patterns) will enable breeders to understand the need for breed-specific cultivars for each target agro-ecology using a suitable breeding strategy. Crop modeling is also highly useful for designing ideal plant ideotypes based on evaluation of past genetic improvement for the selected environment. The efficiency of crop improvement for constantly changing environments can be improved if the physiological and morphological traits associated with drought adaptation are identified and integrated into breeding programs. Singh et al. (2017) used a modified CSM-CERES-Pearl millet model to study the effect of altered traits determining the maturity of the crop, its yield and adaptation to heat and drought prevailing in semi-arid regions of India and Africa. It was found that decreasing crop maturity duration had a negative impact on yield, and that increasing the maturity duration benefited yield in few locations under current and future predicted climatic conditions. In addition, increasing radiation use efficiency (RUE) resulted in higher yields under climate change conditions. Also, improving the length and depth of roots is recommended as an important mechanism for drought adaptation and achievement of better yield (Vadez et al., 2012). The interaction of genotypes with the environment usually results in hampering of the progress of crop breeding programs. Therefore, it is essential to understand the underlying physiological process behind the interaction (Basford and Cooper, 1998). It was reported that pearl millet hybrids suitable for the arid zone must have a smaller canopy than hybrids bred for non-arid regions, and it was related to water use (Vadez et al., 2015). Differences in pearl millet genotypic transpiration responses and growth showed that breeding for various agroecological zones reflected in breeding of specific plant strategies associated plant water use related traits (Medina et al., 2017). van Oosterom et al. (2003) explained the local landraces’ adaptation to drought environments by analyzing the yield components of pearl millet and concluded that landraces benefited from limited water availability as they produced small-sized main shoots panicles. Tharanya et al. (2018) showed that in environments with unlimited water access, pearl millet genotypes with high tillering and biomass accumulation led to increase in water use and eventually higher yield. On the contrary, low plant vigor with less tiller and size along with stay-green trait favored yield in drier regions. In this regard, it is evident that the effect of the interaction of genotype with the environment determines the success of drought adaptation. It is also demonstrated that traits that favored yield in a specific environment might bring a production penalty in another (Yadav and Weltzien, 2000). Therefore, it is essential to characterize the environment for a better understanding of genotype-environment interactions. A detailed characterization of pearl millet growing area through a modeling approach and identification of crop production constraints (drought stress patterns) will enable breeders to understand each target agro-ecology for breeding specific cultivars.
Drought is a complex trait, and breeding for sustainable drought products requires knowledge of the type of drought stress to target the environment and systematic screening method/tool relevant to the stress. Screening methods should be precise and reproducible to accommodate the different number of breeding lines for selection in order to integrate in the breeding pipeline. A combination of screening methods can be integrated in different stages of the breeding pipeline for drought product development.
Integration of sensor-based smart digital technologies like HTP phenotyping platform and UAV based field phenotyping methods can accelerate the breeding process in early stages (F4, F5) of breeding schema for simple component traits of adaptation like early vigor, canopy index, plant height, and tiller number can be useful for discarding non-desirable phenotypes not only for agronomic traits but also for adaptation traits. One such high-throughput and automated phenotyping platform, “LeasyScan,” was established at ICRISAT1 to screen various plant materials in a non-destructive manner during the vegetative stage using an optical system (PlantEye®2). This can be used for precise measurements of plant canopy traits such as digital biomass, 3D-leaf area, plant height, leaf area index, leaf angle, leaf inclination, and light penetration depth (Vadez et al., 2015; Sivasakthi et al., 2018, 2019; Tharanya et al., 2018). This phenotyping platform is also capable of detecting plant diurnal variations in water use parameters using 1,500 analytical scales (Kar et al., 2020). A pearl millet fine-mapping population (n = 162), segregated for QTLs for drought adaptation (Tharanya et al., 2018), was used for phenotyping canopy development [3D-leaf area (3DLA), projected leaf area (PLA), plant height, leaf area index, canopy structure] and conductance (evapotranspiration, transpiration, and transpiration rate)-related traits (Tharanya et al., 2018). This study revealed a large genotypic variation for most of the traits which were not clear in the pot culture experiment. In addition, the principal component analysis revealed an effect of canopy structure on early water use (Lysimetric study) and grain yield (field) obtained under moderate or no water stress (Tharanya et al., 2018). There are no reports on UAV-based characterization in pearl millet under drought conditions.
Selected lines from large scale screening can be taken for detail and precise characterization for drought type pertaining to targeted ecologies (Figure 4). This can be done by artificial imposition of water stress and water uptake measurements under rainout shelter or glasshouse conditions. Screening using the lysimeter platform3 is a gravimetric system of transpiration measurement providing individual soil profile to each plant, and water use could be measured throughout the crop cycle (Vadez et al., 2013). This system allows for the measurement of highly relevant agronomic assessment of yield and yield components. At the same time, total plant water use (the sum of water use data across the entire life of the crop), dynamics of plant water use (water used during crops development), and transpiration efficiency [TE, the ratio between total biomass produced (pods and vegetative parts) and total water use] can be followed. Lysimetric assessment allows us to evaluate temporal variations in water requirements of individual genotypes (Vadez et al., 2011, 2013; Zaman-Allah et al., 2011; Vadez and Ratnakumar, 2016; Tharanya et al., 2018; Sivasakthi et al., 2019) and different crops like sorghum, pearl millet, finger millet, chickpea, and peanut. Using this facility, genetic variations for TE and grain yield in pearl millet were reported (Vadez et al., 2013; Tharanya et al., 2018). In addition, genotypic differences in water extraction pattern in critical crop growth stages were found using the lysimeter facility where drought-adapted genotypes were found to extract lesser quantity of water before flowering and to extract more water post-anthesis, which might confer adaptation to terminal drought (Vadez et al., 2013). Selection can be based on phenology, TE, grain yield, and pre- and post-flowering water utilization patterns. Screening for drought adaptation using pots has a distinct advantage to create fully controlled water stress and to record observations using complex and sensitive equipment. Several experiments assessed the transpiration response of pearl millet to atmospheric and soil drought under fully watered and water deficit conditions where drought-adapted genotypes showed a lower transpiration rate than drought-sensitive genotypes (Kholová et al., 2010a,b, 2016; Choudhary et al., 2019). Although phenotyping through pot culture is simple and cost-effective, it is very difficult to screen large populations with sufficient replication for traits like leaf area, as it involves a destructive method, and assessing transpiration could be laborious.
Figure 4. Phenotyping principle supporting drought breeding programs.LAI, leaf area index; TL No, tiller number; SLN, specific leaf nitrogen; Rint, radiation intercept; RUE, radiation use efficiency; RADN, radiation; kl, crop rooting parameters; T, transpiration; TE, transpiration efficiency; –VPD, vapor pressure deficit; Grain N, grain s; A, assimilation; UAV, unmanned aerial vehicle; MLT, multilocation testing; MSE, multisite evaluation.
Screening under field conditions is performed by evaluating the test material through multi-locational trials conducted in drought-prone regions (Yadav et al., 2012b) or by growing pearl millet during a rain-free season with adequate water supply but withholding irrigations to expose pearl millet to drought in the desired stage (Bidinger et al., 2000).
Assessing millet crop stress using Earth observation data in public domains, such as the Normalized Difference Vegetation Index (NDVI) product derived from the Moderate Resolution Imaging Spectroradiometer (MODIS), challenges multidisciplinary teams to understand different stresses in millet-growing areas. The process involves analyzing historical time series satellite imagery (Figure 5). Use of MODIS NDVI and LSWI products to map crop stress areas and changes in millet areas is due to rainfall. A methodology was developed to identify crop stress-affected areas using the MODIS-based NDVI and LSWI, and compare these crop areas with those during a normal year (Gumma et al., 2015). A long-term average of NDVI during the rainy (Kharif) season (June-October) is compared to a the current crop year. Rainy season NDVI and crop area changes are identified. Secondary data are used to support the crop stress analysis. Spectral matching techniques are utilized to categorize crop stress-affected cropland areas into three classes, severe, moderate, and mild, based on the intensity of damage assessed by field sampling. Spectral signatures are generated based on these ground survey samples (Gumma et al., 2019).
Although pearl millet is an inherently drought-tolerant species, breeding programs have targeted to further increase drought tolerance utilizing available genetic variations in its germplasm. Adaptive evolution concurrent with climate change in its center of diversity and area of production shaped the crop to successfully grow in areas of limited soil moisture (Serba and Yadav, 2016).
Breeding programs have targeted mostly the mid-season and terminal drought stresses in pearl millet, assessed the importance of drought-adapted germplasm, and explored the usefulness of genetic introgression.
Tillering ability is the most important trait that has been strategically manipulated in mid-season drought stress breeding. There is a huge amount of variation in the tillering capacity of pearl millet and it has been reported to be a moderately heritable trait (Appa Rao et al., 1986; Rai et al., 1997; Yadav et al., 2017). Greater tillering provides elasticity to the growth and development of pearl millet and is part of its mechanism for adaptation to severe drought conditions. Several drought tolerance studies conducted in the Sahel have indicated that pearl millet is tolerant to water deficit until early grain filling, predominantly because the main shoot can be compensated by basal tillering (Winkel et al., 1997).
Earliness and short and rapid grain filling period are the two most important traits that have been manipulated for enhancing tolerance to terminal drought. Early flowering essentially determines grain productivity in water stress (Bidinger et al., 1987b; Fussell et al., 1991; Van Oosterom et al., 1995). Genetic variation for earliness is widely available in the germplasm (Rai et al., 1997; Yadav et al., 2017) and phenotype-based selection was accomplished (Rattunde et al., 1989). The frequently exploited basis of earliness is the Iniadi-type landraces collected from western Africa (Andrews and Kumar, 1996). Promising lines with the early flowering trait have been developed from Iniadi landraces and adopted in Indian and African agro-ecologies.
The proportion of panicle threshing denotes seed setting potential under low soil moisture conditions and integrating the effects of assimilation and translocation of photosynthates in drought environments is a measure of drought tolerance (Bidinger et al., 1987b). It exhibits a wide amount of variation in grain yield (Fussell et al., 1991; Bidinger and Mahalakshmi, 1993), is highly heritable (Yadav, 1994), and selection is effective (Bidinger and Mahalakshmi, 1993). Some mathematical models have also been used to recognize lines that are performing well under adverse conditions by comparing grain yields between stress and non-stress (optimum) conditions (Bidinger et al., 1987b; Yadav and Bhatnagar, 2001). Accordingly, multi-environmental data from a diverse range of growing conditions are used to identify drought-tolerant genotypes.
Successful growth of pearl millet in water stress is more of a reflection of its adaptation to drought stress than genetic yield potential (Bidinger et al., 1994). As adaptation is much less understood physiologically and genetically, it is more difficult to improve for adaptation than for yield potential. Pearl millet landraces that evolved in dry areas as a result of natural and man-made selection over thousands of years demonstrate better adaptability to water stress (Yadav et al., 2000; Yadav, 2010, 2014). Efforts were made to utilize these landraces in pearl millet conventional breeding approaches. Cycles of mass selection in genetically heterogeneous landraces are found to increase yield considerably (Bidinger et al., 1995; Yadav and Manga, 1999; Yadav and Bidinger, 2007) and have been revealed as a valuable germplasm source to breed drought-tolerant lines (Yadav, 2004) and develop inbred pollinator lines for hybrid breeding (Yadav et al., 2009, 2012a).
A huge amount of genetic variation in the global germplasm of pearl millet is available for traits that are constitutive and responsive to drought (Table 1). Murty et al. (1967) characterized 1,532 accessions and reported variation in days to flowering among Indian (52–77 days) and exotic (53–85 days) collections. In another study, days to flowering showed much wider variability (33–140 days) among a world collection of 16,968 pearl millet accessions (Harinarayana et al., 1988). IP 4021 (Bhilodi), collected from Gujarat, was the earliest to flower with 33 days to 50% flowering in rainy season and 34 days in post-rainy season. Furthermore, screening efforts identified two promising drought-tolerant lines among 509 accessions that were screened (Harinarayana et al., 1988). Landraces identified from Rajasthan, India exhibited wide variability for different characters. SR 15, SR 17, and SR 54 were three landraces that were identified for earliness. Three other unique landraces that showed earliness were “Chadi” from Rajasthan, “Bhilodi” from Gujarat, and “Pittaganti” from Eastern Ghats of India (Kumar and Appa Rao, 1987). Furthermore, germplasm accessions IP 4066, IP 9496, and IP 9426 were identified as promising early lines. In a detailed study conducted on a wide range of environmental conditions, 105 landraces were evaluated, a wide range in drought response was observed, and 15 landraces (IP 3243, IP 3228, IP 3424, IP 3296, IP 3362, IP 3180, IP 3272, IP 3303, IP 3252, IP 3258, IP 11141, IP 3318, IP 3123, IP 3363, and IP 3244) having a high degree of drought tolerance have been identified for use in developing drought tolerant cultivars (Yadav et al., 2003).
Differential response of landraces and elite genetic materials across contrasting drought and non-drought environments provided need of amalgamating drought tolerance of landraces and high-yielding potential of elite genetic material (Yadav, 2006, 2010; Yadav and Rai, 2011). Crosses between adapted landraces and elite genetic materials showed enhanced adaptation to drought and high productivity (Presterl and Weltzien, 2003; Yadav, 2008; Yadav and Rai, 2011; Patil et al., 2020). Selection of specific landraces and elite materials for hybridization is governed by combinations of phenotypic traits (like tillering, panicle size, seed size, height, and earliness), drought adaptation, and yield potential in two groups of materials (Patil et al., 2020).
The development of hybrids using strategic use of a diverse range of germplasm has been a top priority in pearl millet breeding, especially in India. However, it is generally argued that hybrids are more suitable for optimum environments than heterogeneous OPVs, as the latter has a population buffering effect to provide stable performance in unpredictable drought environments. A comprehensive study reported a yield advantage of hybrids ranging from 19 to 35% over OPVs (Yadav et al., 2012b). The current seed delivery system for pearl millet in India also favors hybrids, but this is not the case in Africa. Nevertheless, hybrids are more likely to play a key role than composites in enhancing pearl millet productivity in water stress environments.
During the past more than two decades, hundreds of thousands of molecular (DNA) markers have been developed and used in pearl millet genetic studies in different research stations around the world (Srivastava et al., 2020a). Different sorts of DNA-based markers like; RFLP, AFLP, RAPD, STSs, gSSRs EST-SSRs, DArTs, CISP, SSCP-SNP, and SNP markers have been devised and used to accelerate the pace of pearl millet breeding programs around the world (Liu et al., 1994; Devos et al., 1995, 2000; Allouis et al., 2001; Qi et al., 2004; Bertin et al., 2005; Senthilvel et al., 2008, 2010; Rajaram et al., 2010; Supriya et al., 2011; Sehgal et al., 2012, 2015; Tara Satyavathi et al., 2021). Several different molecular markers were used to assess genetic variability and population structure and detect genomic regions associated with agriculturally important traits through genome-wide association study (GWAS), genomic selection (GS), marker-aided breeding (MAB), and other genetic studies to speed up breeding programs in pearl millet (Table 2). DNA markers help determine the degree and quantum of genomic diversity in pearl millet germplasm to identify promising lines for hybridization and analyze population structure and QTLs linked with environmental stress tolerance (Vadez et al., 2012).
Table 2. Details of molecular markers developed for pearl millet related to genetic diversity, genome mapping, micronutrient, grain yield, fodder biomass, and stress estimation.
In pearl millet, molecular mapping work started with the RFLP technique (Liu et al., 1992, 1994). One set of potential AFLP markers was developed and employed for genetic diversity assessment in south-western Niger (Allinne et al., 2008) and genomic regulation adaptation in new environments using the advantage of genome scan and association mapping (Saïdou et al., 2009; Mariac et al., 2011). Mariac et al. (2006) developed novel microsatellite markers in pearl millet using public expressed sequence tags (ESTs) available on GenBank.
Subsequently, 21 EST-derived SSRs and six genome-based SSRs were devised from 3,520 EST sequences and employed in genetic mapping in pearl millet (Senthilvel et al., 2008). The design of 277 DArT markers from 6,900 DArT clones utilized PstI/BanII complexity reduction using RIL population (Senthilvel et al., 2010). In another study, a set of robust DArT-based SNPs were detected from several hundred DArT clones generated from different accessions through the PstI/BanII complexity reduction protocol in millet inbred lines (Supriya et al., 2011). Seven LGs covering 1,749 cM with inter marker distance of 5.73 cM were determined by the mapping of DArT and SSR markers; 2 co-localized QTLs associated with grain Fe and Zn content were detected in LG 3 in pearl millet species (Kumar et al., 2016).
Genomic resources have been exploited to generate CISP and SNP markers employed in the discovery of candidate genes pertaining to drought-tolerant QTLs in different accessions in pearl millet (Sehgal et al., 2012). Under another breakthrough, thousands of SNPs were developed through genotyping-by-sequencing (GBS) protocols in global germplasm collections and landraces in pearl millet lines (Hu et al., 2015; Serba et al., 2019; Kanfany et al., 2020). Besides, ISSR-derived SCAR markers have been developed for the analysis of genetic diversity among parental lines used to develop mapping populations for studies on downy mildew (DM) resistance in pearl millet. A distinct locus has been detected in ICMR 01004 line, which was confirmed with PCR technique. The detected SCAR marker was ultimately endorsed in different accessions collected from wide-geographical locations, and the findings indicate its linkage to DM resistance in pearl millet (Jogaiah et al., 2014). A linkage map was constructed by integrating DArT and SSR markers, which were employed in the detection of rust resistance QTLs in LG1 in pearl millet (Ambawat et al., 2016). With the advancement of next-generation sequencing (NGS) technologies, rapid decoding of the crop has enabled the availability of a huge number of genome-wide single nucleotide polymorphism (SNP) markers for trait mapping, trait selection, and trait improvement gains in pearl millet (Table 3). These markers are proved to be essential genomic tools for various genetic studies on pearl millet for crop improvement by molecular breeding (Srivastava et al., 2020b).
Table 3. Key sequence-based markers and their application in various genomic studies on pearl millet.
The first milestone was achieved with the development of the shortest map (303 cM) with 181 RFLP markers using an F2 pearl millet mapping population (Liu et al., 1994). Later, an integrated consensus linkage map of all 7 genetic maps was developed using different crosses with RFLP and SSRs (Qi et al., 2004). In an ICMB 841 × 863 B cross, 91 loci were incorporated using F2 progenies with a 617.4-cM map length (Yadav et al., 2004). A total of 21 informative SSR loci were mapped using previously developed two bi-parental populations derived from crossing an ICMB 841-P3 line with 863B-P2 and 81B-P8 × IPC 804 lines showed that all functional SSRs mapped on distant regions of LGs cover the preceding gaps (Senthilvel et al., 2008). To construct a linkage map with uniform marker distribution with improved coverage of gaps in existing genetic maps, a linkage map was developed using 196 different types of molecular markers with a mean inter-marker distance of 9.2 cM (Pedraza-Garcia et al., 2010). A linkage map was developed with a set of 258 DArT and 63 polymorphic SSR genotyping data using a 140 F7 recombinant inbred line (RIL) population derived from the H77/833-2 × PRLT 2/89-33 cross to construct a dense linkage map by integrating DArT and SSRs (Supriya et al., 2011). This relatively improved linkage map consisted of 321 loci spanning 1,148 cM with a mean inter-marker distance of 3.7 cM, and the length of individual LGs varied between 78 (LG 3) and 370 cM (LG 2) (Supriya et al., 2011). A longer skeleton linkage map of 1,019 cM length for F2-bred F3 progenies spanning 44 uniformly distributed loci with an inter-marker distance of 14 cM (LG1) to 38 cM (LG6) and an overall average distance of 23 cM has been reported by Vengadessan et al. (2013).
Genetic linkage maps were constructed with 99 in-house designed novel EST-SSRs and 17 previously mapped SSRs, 53 gSSRs, and 2 informative STS markers (Rajaram et al., 2013). A consensus map spanning 1,74 loci covering 899 cM genetic distance was constructed by integrating different linkage maps with the MergeMap tool, which revealed an evolutionarily conserved locus order for almost each LG (Rajaram et al., 2013). Then, a genetic linkage map was constructed comprising SNPs with F2 population of a wild × domesticated cross with improved coverage and improved consistency of loci compared to formerly released maps. The linkage map was developed with a revised GBS protocol involving two (PstI and MspI) restriction enzymes and PCR amplification with primers comprising 3 discerning nucleotides to generate thousands of SNPs for open access (Moumouni et al., 2015). Linkage maps were developed with 305 markers (96 codominant SSRs and 208 dominant DArTs), which detected seven LGs spanning 1,749 cM with a mean distance of 5.73 cM among loci. A panel of 106 RILs (F6) derived from a cross between ICMB 841-P3 and 863B-P2 lines was used in the study of Kumar et al. (2016). Additionally, a genetic linkage map was also constructed with DArT and SSR markers using 317 RIL progenies derived from ICMS 8511-S1-17-2-1-1-B-P03 × AIMP 92901-S1-183-2-2-B-08, a bi-parental cross of ICMS 8511-S1-17-2-1-1-B-P03, and AIMP 92901-S1-183-2-2-B-08 lines. The base map (LGs) of 196 loci was 964.2 cM in length (Kumar et al., 2018). The calling of a huge number of SNPs and the availability of saturated genetic maps may accelerate the pace of progress in linkage studies using cross populations considerably and make easy genetic studies on collections of diverse lines.
Thousands of informative DNA markers have been deployed to dissect QTLs for investigation of the molecular and biochemical bases of tolerance to abiotic stress in millets, and to devise an efficient approach for crop improvement for yields under drought stresses. Subsequently, dissection of QTLs pertaining to drought tolerance (DT-QTLs) and high grain yield was identified in independent studies using different pearl millet mapping populations (Yadav et al., 2002, 2004; Bidinger et al., 2007). The milestone breakthrough in detection of major DT-QTL in LG2 related to grain yield explaining 32% phenotypic variance was carried out (Yadav et al., 2002) using bi-parental populations of individuals of different crosses. Afterward, a minor QTL in LG5 linked with drought tolerance and explaining 14.8% phenotypic variance was also detected (Yadav et al., 2004). The putative drought-tolerant QTL in LG2 was evaluated using near-isogenic lines derived from H 77/833-2 into which a DT-QTL has been introgressed from PRLT 2/89-33 (Serraj et al., 2005). In the same direction, three major QTLs (positioned in LG2, LG3, and LG4) pertaining to grain yield with limited QTL × environment interactions were analyzed as key components of MAB for improved grain yield under variable post-flowering water stresses in pearl millet (Serraj et al., 2005; Bidinger et al., 2007). Major QTLs mapped on LG2 and LG3 accounted for a wide (13-25%) range of phenotypic variations for grain yield traits under drought stress conditions. At the same time, minor QTLs were also co-mapped for harvest index under drought stress, and QTLs for both grain number and individual grain mass in water deficits were identified (Bidinger et al., 2007).
These QTLs mapping findings have been validated in linkage mapping investigations that mapped QTLs linked to high grain yield and its related traits under terminal drought stresses in pearl millet (Yadav et al., 2011). In this study, one major QTL associated with grain yield and drought tolerance of grain yield under water stress conditions was detected in LG2, which explains about 32% of phenotypic variance in test cross progenies (Yadav et al., 2011). The effect of this major QTL that explained 32% of the variance under drought stress was confirmed in different marker-aided backcross programs, where a 30% increment for grain yield general combining ability (GCA) anticipated of DT-QTL in water deficits was recovered in QTL introgression lines (Yadav et al., 2011). Millet species have evolved with an inherited potential for climate resilience to biotic and abiotic stresses, and they may serve as an important source of key genes underlying stress tolerance, which have to be further investigated and characterized (Jangra et al., 2019). The close taxonomic relationship of millets with other staple cereal crop species may allow for introgression of untapped alleles, key genes, genomic regions, or QTLs detected in millets for improved agro-economic traits into other cereals to ensure sustainable food and nutritional security under climate changes and increasing global warming. Details of the genomic regions/QTLs associated with drought stress and other component agronomic traits in pearl millet are provided in Table 4. To dissect the components of drought-tolerant QTLs, 75 SNPs and CISP markers have been designed using an open-access EST database created for some elite pearl millet lines (Sehgal et al., 2012). Some key regulatory genes encoding photosystem I (PSI) reaction center component proteins were identified from DT-QTL regions mapped on LG2 (Sehgal et al., 2012); these candidate genes are supposed to contribute to QTL regions associated with yield and flowering traits under water stress in pearl millet.
Table 4. Details of genomic regions/quantitative trait loci (QTLs) associated with drought stress and other component agronomic traits related to drought in pearl millet.
The key function of a major DT-QTL was tested, and one major terminal DT-QTL was found to be controlling minimum salt uptake and better adaptation under salt-induced oxidative conditions in pearl millet. An illustration of restrained salt acquisition in a drought-resilient accession was found to invariably be linked with improved plant growth with grain yield in salt stresses. There was evidence that the DT-QTL conferred positive impacts on luxurious crop development and yield characters under saline conditions by reducing the accumulation of sodium ions in aerial parts of plants (Sharma et al., 2011). The major QTL linked with improved drought tolerance in the post-flowering and grain-filling stages has also been found to be associated with limited salt uptake and transport in pearl millet (Sharma et al., 2014). Reduced levels of toxic (Na+) ionic accumulation and compartmentalization have been recorded in leaves, while access accumulation of toxic ion Na + was diminished in plant roots of pearl millet. It may be concluded that ionic uptake is controlled by genes pertaining to the DT-QTL in LG2 (Sharma et al., 2014). The QTL associated with low transpiration rates that contributes to water stress tolerance by lodging soil water contents to be used in the grain filling stage by limiting moisture loss in the vegetative phase has been co-mapped with the terminal DT-QTL (Kholová et al., 2012). Low rate of transpiration is maintained by physiological and morphological interactions (Kholová et al., 2012). To unravel the physiological mechanisms behind DT-QTL, fine mapping population segregating for DT-QTL was used to co-map the QTL for transpiration rate (TR) and established that by minimizing water loss during vegetative stage, DT-QTL conserves soil moisture and, thus, contributes to terminal drought tolerance (Kholová and Vadez, 2013). Furthermore, these DT-QTLs associated with terminal drought tolerance have been introgressed into elite pearl millet lines to improve terminal drought tolerance (Jangra et al., 2019). Marker-aided foreground selection has been performed with robust SSR markers mapped on LG2 and LG5 to select plants harboring alleles that render resistance to bi-parental progenies (BC4F2) along with rigorous phenotypic selection to recover the genome of the recurrent parent in pearl millet (Jangra et al., 2019).
Transcriptomic investigation with next-generation sequencing (NGS) technologies has emerged as an efficient proficient approach for analyzing gene expression profiling in plant species. However, RNA sequencing (RNA-seq) utilizing the NGS technique can detect differentially expressed genes (DEGs) owing to their dynamic range of expression levels (Jongeneel et al., 2001). A de novo assembly based transcriptome analysis under drought stress was published and reported several key genes, transcription factors, gene regulatory networks that regulate water stresses in pearl millet (Jaiswal et al., 2018). Some informative SNPs were identified controlling nucleic acid and amino acid metabolic pathways under environmental stresses. Since the pearl millet whole-genome sequence has been published (Varshney et al., 2017), attempts were made to unveil the genetic basis of tolerance to water stresses in pearl millet through the RNA-seq approach (Dudhate et al., 2018). More recently, an important study on RNA-seq analysis was performed to assess the comparative transcriptomics in the vegetative and reproductive stages to discover key genes involved in drought response in a pearl millet drought-tolerant (PRLT2/89-33) line (Shivhare et al., 2020). Several underlying genes, pathways involved in lignin, flavonoids, pigments, and other important secondary metabolites for biosynthesis were enriched and activated in pearl millet (Shivhare et al., 2020).
Genomics provides opportunities to develop DNA-based tools to mitigate the problems of ever-increasing food and fodder yield, nutritional security, and sustainable crop production by modern breeding approaches. The development and deployment of DNA markers for plant genetic advancements through marker-assisted selection (MAS) have been established in bi-parental breeding. Advances in plant genomics offer high-density sequence-based molecular tools exploiting NGS technology for genetic evaluation of breeding populations, and are important to accelerate the pace of pearl millet breeding programs (Varshney et al., 2017). A genomics-driven breeding scheme for breeding of climate-resilient varieties (Varshney et al., 2005) has been initiated by dissecting abiotic stress that will likely influence grain yield and productivity in prevailing climate change due to global warming. Information derived from multi-environment trials offers an opportunity for studying the impacts of abiotic stress on crop plants and populations under investigation. Crop scientists are involved in the exploration of morphological and physiological traits in plants that could improve crop adaptation to this looming climate change. In the present context, plant physiology facilitates the description of ideotypes to be practiced for improving salient adaptation under such harsh climate scenarios. Additionally, the application of geographic and passport information permits the selection of lines thriving well in abiotic stress-prone locations, while existing techniques such as genetic profiling, data analysis, and mapping of agriculturally important genes and genomic regions (QTLs) help in identifying potential lines for next-level screening for certain abiotic stresses (Kole et al., 2015). Moreover, accurate phenotyping for morphological traits and proper biometric evaluation facilitate the identification of certain responses of a panel of accessions in the prevailing growth stage affected by variable weather conditions. Such type of inputs has further been utilized in genomics-assisted breeding (GAB) techniques including genome-wide selection of promising accessions for further deployment in breeding and variety development (Srivastava et al., 2020a). Genetic mapping and QTL dissection using bi-parental and association mapping (AM) populations have facilitated the analysis of genomic regulation of expression agronomic traits. It also precisely permits marker-assisted selection (MAS), linkage mapping (QTLs), and GWAS and GS of promising lines to be developed in the perspective of breeding strategies (Kulwal et al., 2012; Kumar et al., 2018; Singh et al., 2020).
Until recently, the use of GWAS and GS has been impeded because of unavailability of high-density sequence-based DNA markers (Srivastava et al., 2020a). Invention and advancement in genomic technologies with NGS protocols have paved the way for rapid development of genome-wide molecular markers including SNPs and insertion-deletions (InDels) in pearl millet and other orphan plant species (Varshney et al., 2017). Identification of candidate genes and untapped alleles for target agronomic traits has been accomplished through GBS–based genetic approaches (Debieu et al., 2018). In the same way, GWAS has been conducted to detect genomic regions controlling agronomically useful traits and determine the statistical association between genetic polymorphisms and trait variations in area-wide germplasm collection subjected to genotypic and phenotypic characterizations. NGS in association with GWAS enhances high-resolution QTL/gene/s mapping (Varshney et al., 2017; Debieu et al., 2018; Serba et al., 2019). NGS-based technologies with cost-efficient genotyping assays, determination of genome-wide genetic variations, fine linkage mapping, and discovery of candidate genes for drought tolerance and natural allelic variants for QTLs controlling key agronomic traits have gained increased attention in routine plant breeding programs. For instance, three major QTLs linked to grain yield with low QTL and environmental (QTL × E) interactions were detected across different post-flowering water stresses in pearl millet (Bidinger et al., 2007). One of the major QTLs explained ∼32% of phenotypic variation in grain yield under water deficit conditions. The impacts of these dominant QTLs have been validated in marker-assisted back cross (MABC) programs wherein 30% enhancement of the general combining ability for grain yield was anticipated from this QTL under terminal drought stress. It was recovered in introgression lines using informative data generated with markers flanking the QTL (Yadav et al., 2011). This QTL has been fine-mapped using the LG2 QTL NIL-derived F2 mapping population with ddRAD SNPs (Srivastava et al., 2017). Out of 52,028 SNPs that were identified among NILs, a total of 10 SNPs were anchored to the QTL interval and are being used in forward breeding programs using the HTPG platform.
Many potential marker-assisted backcrossing (MABC) methods are devised, which have been employed in QTL introgression from a donor to an elite recurrent parent, while pearl millet lines have been used to develop base mapping populations. ICRISAT (Patancheru, India), with the collaboration of United Kingdom-based scientists supported by the Plant Sciences Programme, Department for International Development (DFID), has made substantial efforts to develop and use genomic tools for increasing grain yield, yield stability, and productivity of hybrid cultivars in pearl millet (Hash et al., 1999). They described the use of MABC methods in pearl millet genetic improvement and attempted to enhance the drought tolerance of an elite inbred pollinator (H 77/833-2) line using a donor PRLT 2/89-33 line and the elite inbred seed parent maintainer genotype ICMB 841 using the 863B line as a donor. MABC methods have played a significant role in the considerable boost of grain yields, production, and quality in pearl millet, which has been achieved in India over the past many years. Development of a marker flanking QTLs governing yield and related traits under limited soil water contents has been a major focus of such type of research activities in the last several decades (Yadav, 2010, 2014). Several validated QTLs have been introgressed into elite hybrid parental lines (A-/ B-, R-), resulting in improved version of hybrids or essentially derived varieties (EDVs). Over two million people enjoy improved food security because of an output from these collaborative research programs using genomic resources in the breeding of genetically advanced pearl millet lines with high resilience to downy mildew. Efforts involving ICRISAT, John Innes Centre, Norwich; Institute of Grassland and Environmental Research, Aberystwyth University, University of Wales, Bangor, United Kingdom, and Chaudhary Charan Singh Haryana Agricultural University (CCSHAU), Hisar, India have detected QTLs associated with DM resistance in the pearl millet H 77/833-2 line, and 2 more QTLs were introgressed using MABC to design a male parental line with improved DM-resistance. The popular hybrid HHB 67 Improved was bred with a cross between improved DM resistant seed parent 843-22A and improved restorer parent H 77/833-2-202 lines. HHB 67 Improved is currently grown in more than 10% of the entire crop area every year in India and is one of the most impactful research outputs in crop plants globally. Recently, efforts were made to further improve the HHB 67 Improved hybrid, as it has started to show signs of breakdown to DM-resistance again. Several downy mildew resistance double QTL introgression lines were generated in the genetic background of restorer parent H 77/833-2-202. These improved introgression lines were used to produce HHB 67 Improved-like test-cross hybrids with enhanced levels of downy mildew resistance (Srivastava et al., 2015, 2020b). These second-cycle improved versions are being tested in EDV trials conducted by AICRP-PM. The MAS has been extensively employed in pearl millet to breed advanced versions of the previous lines (Sharma, 2001; Yadav et al., 2005; Hash et al., 2006; Sehgal et al., 2015). The validated LG2 terminal drought-tolerant QTL (Kholová et al., 2010a,b; Bidinger et al., 2007) from drought tolerant parent PRLT 2/8933 has been moved to H 77/8332 using MABC.
Improvement of genetic gains in terms of grain yield in harsh environments needs knowledge-based selection approaches that use phenotypic and genotypic data. Such type of marker-aided selection has been regarded as genomic selection (Meuwissen et al., 2001). The efficacy of genomic selection in augmenting the degree of genetic gain in crops and evaluation of the implementation of genomic models are limited to other millet crop species (Srivastava et al., 2020a). A first GS study on pearl millet was carried out by Varshney et al. (2017) at ICRISAT, Patancheru to predict grain yield for test crosses and then to predict the performance of single cross hybrids derived from a cytoplasmic-genetic male sterility (CMS) system in individual trials (Liang et al., 2018). High-throughput genotyping methods and genomic sequencing techniques have enabled the generation of rapid and cost-efficient genotypic assays, which facilitate the use of genomic prediction (GP) and genomic selection (GS) in plant and animal breeding. GS exploits DNA marker data to determine genomics-estimated breeding values (GEBVs) for a complex trait, and final selection is based directly on GEBVs exclusive of further field-based phenotypic characterization (Crossa et al., 2017). The key principle is that the genotypic data generated from several markers are uniformly distributed in plant genomes having the potential to unveil genomic polymorphism, and evaluate breeding values without prior information about where the selected alleles are located (Crossa et al., 2017).
Genomic selection (GS) in crop species is advantageous owing to its potential to predict selection decisions even in the off-season, hence enhancing significant genetic gain (Heffner et al., 2010). A simulation analysis was accomplished on maize and established the advantage of GS in place of the conventional marker-assisted selection approach (Crossa et al., 2013). Since then, several studies have exploited the benefits of GS in several crops including maize (Shikha et al., 2017), wheat (Norman et al., 2017), barley (Nielsen et al., 2016), sorghum (Fernandes et al., 2018), and pearl millet (Liang et al., 2018). Plant breeding studies have been reported on assessing the prediction ability of GS in various commercially useful crops, using different genotyping platforms and densities, and applying different parametric and non-parametric genomic prediction models to different agronomically important traits. Recently generated resequencing data have been used to perform GS to predict grain yield for test crosses in pearl millet. Different situations of prediction were explored in GS, for instance, the performance of grain yield within and across environments. High prediction accuracy has been recorded in terms of Pearson correlation coefficient among the predicted and observed values, optimized using the square root of the heritability for performance in diverse environments (Varshney et al., 2017).
Genome-wide molecular markers are potential tools for QTL discovery and deployment (Mohan et al., 1997; Bevan and Uauy, 2013). Moreover, high-density DNA markers are useful in the assessment of genetic variations and population structures of plant germplasm collections for systematic utilization in the breeding of elite varieties and genetic resource conservation and management. With the current progress made in NGS technologies and cost-efficient genotyping platforms, determination of genome-wide genetic variability, fine QTL mapping, and discovery of candidate genes for drought tolerance and natural allelic variants for QTLs controlling key agronomic traits have gained increased attention in routine plant breeding programs.
More than 70% of the pearl millet area would continue to be rainfed where moisture availability is seldom adequate, and the crop will always be prone to facing intermittent drought in the critical growth stage. Increasing climate variability in pearl millet production ecology and the burgeoning population in south Asia and sub-Saharan Africa imply a greater competition for water use in staple crop production besides the industry and domestic sectors. Current defined production ecologies are either vague or quite old, with high variations in agro-climatic conditions. Pearl millet target production environments require rigorous quantification of dynamic TPE with high resolution advanced MET database and crop coefficients to accelerate breeding programs. Enhancing crop productivity with less amount of water, the so-called concept of “more crop per drop,” is becoming a global necessity. Advancements in genomic tools and their effective integration improve the prospects of drought tolerance pipelines through target traits introgression and rapid recycling using speed breeding platform of adapted pearl millet germplasm/parents in conventional breeding. The availability of germplasm collections is relatively meager for diversifying the cultivar base in target regions. Therefore, the inclusion of West Africa and western India warrants more drought-adapted breeding populations. Adding new germplasm collections to drought-tolerant crossing programs to broaden the genetic base ensures the least disturbing maturity period (70–75 days) that is preferred by farmers. There existed a need of putting great thrust on the development of hybrid parents for drought-affected areas as hybrids have a distinct yield advantage of 20–30% over OPVs. ICRISAT exclusive drought-tolerant product profiles established in 2020 will provide the opportunity to build resources to the mainstream of validated traits, linked markers, and genomic selections with high-throughput screening facilities in target environments or platforms at the breeding center that mimic target environments.
Further success will depend on the development of repeatable, cost-efficient, high-throughput phenotyping facilities that reliably characterize genetic variation for drought tolerance and its contributing traits. Currently, hyperspectral remote sensing UAV technologies are an alternative to the manual collection of crop data, offering information on traits and factors influencing crop development and productivity with relatively shorter time and lower cost. Use of ultra-high spatial resolution hyperspectral remote sensing technology aided by machine learning and artificial intelligence (AI) have the potential to revolutionize the drought tolerance breeding process. These will aid in faster selection process and make more inclusive many traits that are complex or time consuming in assessment, like component traits for adaptation. Several new techniques for genomics offer a great opportunity to accelerate the cultivar development process. NGS-based genomic technologies have the potential to revolutionize the way crop breeding for drought tolerance is being attempted. Rapid trait mapping, validation, and deployment may be possible by combining advances made in our understanding of the underlying physiological factors and their interaction with environmental indices, and precision phenotyping. Marker-assisted deciphering of heterotic gene pools and development and diversification of the genetic base of seed and pollen parents will be critical in the development of heterotic and resilient drought-adapted cultivars. The heterotic group-based breeding strategy may bring viable breeding pipelines in the next few years using appropriate elite germplasm in crossing, rapid generation (RapidGen) advancement, and consortium-based drought trial network testing to stage-gate approaches. All such integrated programs with network trailing established and led by ICRISAT in association with NARS will accelerate the drought-tolerant product pipelines in India. Whole-genome resequencing-based selection approaches may help reduce the complexity associated with drought and its underlying component traits, and may provide useful insights into favorable haplotypes associated with drought adaptation. These can help us move toward breeding strategies based on haplotype selection leading to an enhanced rate of genetic gains in drought agro-ecologies.
RKS, RV, and OY planned and coordinated the study. RKS, OY, MaG, SG, DS, JK, SK, TM, SC, CS, RBS, SB, RG, and RV contributed to this work and drafted the manuscript. All authors contributed to the article and approved the submitted version.
This funding support from the Department of Biotechnology (DBT), GCRF/BBSRC TIGR2ESS programme (BB/P027970/1), Department of Science and Technology-Science and Engineering Research Board (DST-SERB; PDF/2018/001919), and the CGIAR Research Program on Grain Legumes and Dryland Cereals (CRP-GLDC) was gratefully acknowledged. This study has been published as part of the CGIAR Research Program on Grain Legumes and Dryland Cereals.
The authors declare that the research was conducted in the absence of any commercial or financial relationships that could be construed as a potential conflict of interest.
All claims expressed in this article are solely those of the authors and do not necessarily represent those of their affiliated organizations, or those of the publisher, the editors and the reviewers. Any product that may be evaluated in this article, or claim that may be made by its manufacturer, is not guaranteed or endorsed by the publisher.
Allinne, C., Mariac, C., Vigouroux, Y., Bezançon, G., Couturon, E., Moussa, D., et al. (2008). Role of seed flow on the pattern and dynamics of pearl millet (Pennisetum glaucum [L.] R. Br.) genetic diversity assessed by AFLP markers: a study in south-western Niger. Genetica 133, 167–178. doi: 10.1007/s10709-007-9197-7
Allouis, S., Qi, X., Lindup, S., Gale, M. D., and Devos, K. M. (2001). Construction of a BAC library of pearl millet, Pennisetum glaucum. Theor. Appl. Genet. 102, 1200–1205. doi: 10.1007/s001220100559
Ambawat, S., Senthilvel, S., Hash, C. T., Nepolean, T., Rajaram, V., Eshwar, K., et al. (2016). QTL mapping of pearl millet rust resistance using an integrated DArT-and SSR-based linkage map. Euphytica 209, 461–476. doi: 10.1007/s10681-016-1671-9
Andrews, D. J., and Kumar, A. (1996). Use of the West African pearl millet landrace Iniadi in cultivar development. Plant Genet. Resour. Newsl. 105, 15–22.
Aparna, K., Nepolean, T., Srivastsava, R. K., Kholová, J., Rajaram, V., Kumar, S., et al. (2015). Quantitative trait loci associated with constitutive traits control water use in pearl millet [Pennisetum glaucum (L.) R. Br.]. Plant Biol. 17, 1073–1084. doi: 10.1111/plb.12343
Appa Rao, S., Mengesha, M. H., Vyas, K. L., and Rajagopal Reddy, C. (1986). Evaluation of pearl millet germplasm from Rajasthan. Indian J. Agric. Sci. 56, 4–9.
Arya, L., Verma, M., Gupta, V. K., and Karihaloo, J. L. (2009). Development of EST SSRs in finger millet (Eleusine coracana ssp coracana) and their transferability to pearl millet (Pennisetum glaucum). J. Plant Biochem. Biotechnol. 18, 97–100. doi: 10.1007/BF03263303
Basford, K. E., and Cooper, M. (1998). Genotype x environment interactions and some considerations of their implications for wheat breeding in Australia. Aust. J. Agric. Res. 49, 153–174. doi: 10.1071/A97035
Berthouly-Salazar, C., Thuillet, A. C., Rhoné, B., Mariac, C., Ousseini, I. S., Couderc, M., et al. (2016). Genome scan reveals selection acting on genes linked to stress response in wild pearl millet. Mol. Ecol. 25, 5500–5512. doi: 10.1111/mec.13859
Bertin, I., Zhu, J. H., and Gale, M. D. (2005). SSCP-SNP in pearl millet-a new marker system for comparative genetics. Theor. Appl. Genet. 110, 1467–1472. doi: 10.1007/s00122-005-1981-0
Bevan, M. W., and Uauy, C. (2013). Genomics reveals new landscapes for crop improvement. Genome Biol. 14:206. doi: 10.1186/gb-2013-14-6-206
Bhattacharjee, R., Bramel, P., Hash, C., Kolesnikova-Allen, M., and Khairwal, I. (2002). Assessment of genetic diversity within and between pearl millet landraces. Theor. Appl. Genet. 105, 666–673. doi: 10.1007/s00122-002-0917-1
Bieler, P., Fussell, L. K., and Bidinger, F. R. (1993). Grain growth of Pennisetum glaucum (L.) R. Br. under well watered and drought stressed conditions. Field Crops Res. 21, 41–54.
Bidinger, F. R., Chandra, S., and Mahalakshmi, V. (2000). “Genetic improvement of tolerance to terminal drought stress in pearl millet (Pennisetum glaucum (L) R Br,” in Molecular Approaches for the Genetic Improvement of Cereals for Stable Production in Water-Limited Environments. A Strategic Planning Workshop held at CIMMYT, El Batan, Mexico, eds J.-M. Ribaut and D. Polland (Mexico: CIMMYT), 59–63.
Bidinger, F. R., and Hash, C. T. (2004). “Pearl millet,” in Physiology and Biotechnology Integration for Plant Breeding, eds H. T. Nguyen and A. Blum (New York: Marcel Dekker), 225–270.
Bidinger, F. R., and Mahalakshmi, V. (1993). Selection for drought tolerance. In, Cereals Program, ICRISAT 1993. Annual Report 1992. Patancheru: ICRISAT, 57–59.
Bidinger, F. R., Mahalakshmi, V., and Rao, G. D. P. (1987a). Assessment of drought resistance in pearl millet [Pennisetum amencanum (L.) Leeke]: I. Factors affecting yield under stress. Aust. Agric. Res. 38, 37–48. doi: 10.1071/AR9870037
Bidinger, F. R., Mahalakshmi, V., and Rao, G. D. P. (1987b). Assessment of drought resistance in pearl millet [Pennisetum americanum (L.) Leeke]. II. Estimation of genotype response to stress. Aust. J. Agric. Res. 38, 49–59. doi: 10.1071/ar9870049
Bidinger, F. R., Mahalakshmi, V., Talukdar, B. S., and Sharma, R. K. (1995). Improvement of landrace cultivars of pearl millet for arid and semi-arid environments. Ann. Arid Zone 34, 105–110.
Bidinger, F. R., Nepolean, T., Hash, C. T., Yadav, R. S., and Howarth, C. J. (2007). Identification of QTL for grain yield of pearl millet [Pennisetum glaucum (L.) R. Br.] in environments with variable moisture during grain filling. Crop Sci. 47, 969–980. doi: 10.2135/cropsci2006.07.0465
Bidinger, F. R., Serraj, R., Rizvi, S. M. H., Howarth, C., Yadav, R. S., and Hash, C. T. (2005). Field evaluation of drought tolerance QTL effects on phenotype and adaptation in pearl millet [Pennisetum glaucum (L.) R. Br.] topcross hybrids. Field Crops Res. 94, 14–32. doi: 10.1016/j.fcr.2004.11.006
Bidinger, F. R., Weltzien, E., Mahalakshmi, V., Singh, S. D., and Rao, K. P. (1994). Evaluation of landrace topcross hybrids of pearl millet for arid zone environments. Euphytica 76, 215–226. doi: 10.1007/bf00022166
Brunken, J. N. (1977). A systematic study of Pennisetum sect. Pennisetum (Gramineae). Am. J. Bot. 64, 161–176. doi: 10.1002/j.1537-2197.1977.tb15715.x
Budak, H., Pedraza, F., Cregan, P. B., Baenziger, P. S., and Dweikat, I. (2003). Development and utilization of SSRs to estimate the degree of genetic relationships in a collection of pearl millet germplasm. Crop Sci. 43, 2284–2290. doi: 10.2135/cropsci2003.2284
Burgarella, C., Cubry, P., Kane, N. A., Varshney, R. K., Mariac, C., Liu, X., et al. (2018). A Western Sahara centre of domestication inferred from pearl millet genomes. Nat. Ecol. Evol. 2, 1377–1380. doi: 10.1038/s41559-018-0643-y
Busso, C. S., Devos, K. M., Ross, G., Mortimore, M., Adams, W. M., Ambrose, M. J., et al. (2000). Genetic diversity within and among landraces of pearl millet (Pennisetum glaucum) under farmer management in West Africa. Genet. Resour. Crop Evol. 47, 561–568. doi: 10.1023/A:1008767220320
Carberry, P. S., Cambell, L. E., and Bidinger, F. R. (1985). The growth and development of pearl millet as affected by plant population. Field Crops Res. 11, 193–220. doi: 10.1016/0378-4290(85)90102-9
Choudhary, S., Guha, A., Kholova, J., Pandravada, A., Messina, C. D., Cooper, M., et al. (2019). Maize, sorghum, and pearl millet have highly contrasting species strategies to adapt to water stress and climate change-like conditions. Plant Sci. 295:110297. doi: 10.1016/j.plantsci.2019.110297
Comstock, J. P. (2002). Hydraulic and chemical signaling in the control of stomatal conductance and transpiration. J. Exp. Bot. 53, 195–200. doi: 10.1093/jexbot/53.367.195
Crossa, J., Beyene, Y., Kassa, S., Pérez, P., and Hickey, J. M. (2013). Genomic prediction in maize breeding populations with genotyping-by-sequencing. G3 3, 1903–1926. doi: 10.1534/g3.113.008227
Crossa, J., Pérez-Rodríguez, P., Cuevas, J., Montesinos-López, O., Jarquín, D., de Los Campos, G., et al. (2017). Genomic selection in plant breeding: methods, models, and perspectives. Trends Plant Sci. 22, 961–975. doi: 10.1016/j.tplants.2017.08.011
Debieu, M., Kanfany, G., and Laplaze, L. (2017). Pearl millet genome: lessons from a tough crop. Trends Plant Sci. 22, 911–913. doi: 10.1016/j.tplants.2017.09.006
Debieu, M., Sine, B., Passot, S., Grondin, A., Akata, E., Gangashetty, P., et al. (2018). Response to early drought stress and identification of QTLs controlling biomass production under drought in pearl millet. PLoS One 13:e0201635. doi: 10.1371/journal.pone.0201635
Devos, K. M., Pittaway, T. S., Busso, C. S., Gale, M. D., Witcombe, J. R., and Hash, C. T. (1995). Molecular tools for the pearl millet nuclear genome. Int. SorghumMillets Newsl. 36, 64–66.
Devos, K. M., Pittaway, T. S., Reynolds, A., and Gale, M. D. (2000). Comparative mapping reveals a complex relationship between the pearl millet genome and those of foxtail millet and rice. Theor. Appl. Genet. 100, 190–198. doi: 10.1007/s001220050026
Diack, O., Kanfany, G., Gueye, M. C., Sy, O., Fofana, A., Tall, H., et al. (2020). GWAS unveils features between early-and late-flowering pearl millets. BMC Genomics 21:777. doi: 10.1186/s12864-020-07198-2
Dudhate, A., Shinde, H., Tsugama, D., Liu, S., and Takano, T. (2018). Transcriptomic analysis reveals the differentially expressed genes and pathways involved in drought tolerance in pearl millet [Pennisetum glaucum (L.) R. Br]. PLoS One 13:e0195908. doi: 10.1371/journal.pone.0195908
Fernandes, S. B., Dias, K. O., Ferreira, D. F., and Brown, P. J. (2018). Efficiency of multi-trait, indirect, and trait-assisted genomic selection for improvement of biomass sorghum. Theor. Appl. Genet. 131, 747–755. doi: 10.1007/s00122-017-3033-y
Fussell, L. K., Bidinger, F. R., and Bieler, P. (1991). Crop physiology and breeding for drought tolerance. Research and development. Field Crops Res. 27, 183–199. doi: 10.1016/0378-4290(91)90061-y
Fussell, L. K., Pearson, C. J., and Norman, M. J. T. (1980). Effect of temperature during various growth stages on grain development and yield of Pennisetum americanum. J. Exp. Bot. 31, 621–633. doi: 10.1093/jxb/31.2.621
Govindaraj, M., Selvi, B., and Rajarathinam, S. (2009). Correlation studies for grain yield components and nutritional quality traits in pearl millet (Pennisetum glaucum (L.) R. Br.) germplasm. World J. Agric. Sci. 5, 686–689.
Govindaraj, M., Shanmugasundaram, P., Sumathi, P., and Muthiah, A. R. (2010). Simple, rapid and cost effective screening method for drought resistant breeding in pearl millet. Electron. J. Plant Breed. 1, 590–599.
Grantz, D. A. (1990). Plant response to atmospheric humidity. Plant Cell Environ. 13, 667–679. doi: 10.1111/j.1365-3040.1990.tb01082.x
Gregory, P. J. (1983). Response to temperature in a stand of pearl millet (Pennisetum typhoides S. & H.): III. Root development.]. Exp. Bot. 34, 744–756. doi: 10.1093/jxb/34.6.744
Gumma, M. K., Mohanty, S., Nelson, A., Arnel, R., Mohammed, I. A., and Das, S. R. (2015). Remote sensing based change analysis of rice environments in odisha, india. J. Environ. Manage. 148, 31–41. doi: 10.1016/j.jenvman.2013.11.039
Gumma, M. K., Nelson, A., and Yamano, T. (2019). Mapping drought-induced changes in rice area in india. Int. J. Remote Sens. 40, 8146–8173. doi: 10.1080/01431161.2018.1547456
Gupta, S. K., Patil, K. S., Rathore, A., Yadav, D. V., Sharma, L. D., Mungra, K. D., et al. (2020). Identification of heterotic groups in South-Asian-bred hybrid parents of pearl millet. Theor. Appl. Genet. 133, 873–888. doi: 10.1007/s00122-019-03512-z
Gupta, S. K., Rai, K. N., Singh, P., Ameta, V. L., Gupta, S. K., Jayalekha, A. K., et al. (2015). Seed set variability underhigh temperatures during flowering period in pearlmillet [Pennisetum glaucum L. (R.) Br.]. Field Crops Res. 171, 41–53. doi: 10.1016/j.fcr.2014.11.005
Harinarayana, G., Appa Rao, S., and Mengesha, M. H. (1988). “Prospects of utilizing genetic diversity in pearl millet,” in Genetic Resources, Indian Perspective, eds R. S. Paroda and R. K. Arora (India: NBPGR), 170–182. doi: 10.3389/fpls.2021.599649
Hash, C. T., Sharma, A., Kolesnikova-Allen, M. A., Singh, S. D., Thakur, R. P., Bhasker Raj, A. G., et al. (2006). Teamwork delivers biotechnology products to Indian small-holder crop-livestock producers: pearl millet hybrid “HHB 67 Improved” enters seed delivery pipeline. J. SAT Agric. Res. 2, 1–3.
Hash, C. T., Yadav, R. S., Cavan, G. P., Howarth, C. J., Liu, H., Qi, X., et al. (1999). “Marker-assisted backcrossing to improve terminal drought tolerance in pearl millet,” in Molecular approaches for the genetic improvement of cereals for stable production in water-limited environments, ed. J.-M. Ribaut (Mexico: CIMMYT).
Heffner, E. L., Lorenz, A. J., Jannink, J. L., and Sorrells, M. E. (2010). Plant breeding with genomic selection: gain per unit time and cost. Crop Sci. 50, 1681–1690. doi: 10.2135/cropsci2009.11.0662
Henson, I. E., Mahalakshmi, V., Alagarswamy, G., and Bidinger, F. R. (1984). The effect of flowering on stomatal response to water stress in pearl millet (Pennisetum americanum (L.) Leeke). J. Exp. Bot. 35, 219–226. doi: 10.1093/jxb/35.2.219
Howarth, C. J., Rattunde, E. W., Bidinger, F. R., and Harris, D. (1996). “Seedling Survival of Abiotic Stress: Sorghum and Pearl Millet,” in Proceedings of the International Conference on Genetic Enhancement of Sorghum and Pearl Millet (New Delhi: ICAR).
Hu, Z., Mbacké, B., Perumal, R., Guèye, M. C., Sy, O., Bouchet, S., et al. (2015). Population genomics of pearl millet (Pennisetum glaucum (L.) R. Br.): comparative analysis of global accessions and Senegalese landraces. BMC Genomics 16:1048. doi: 10.1186/s12864-015-2255-0
Jaiswal, S., Antala, T. J., Mandavia, M. K., Chopra, M., Jasrotia, R. S., Tomar, R. S., et al. (2018). Transcriptomic signature of drought response in pearl millet (Pennisetum glaucum (L.) and development of web-genomic resources. Sci. Rep. 8:3382. doi: 10.1038/s41598-018-21560-1
Jangra, S., Rani, A., Yadav, R. C., Yadav, N. R., and Yadav, D. (2019). Introgression of terminal drought stress tolerance in advance lines of popular pearl millet hybrid through molecular breeding. Plant Physiol. Rep. 24, 359–369. doi: 10.1007/s40502-019-00464-w
Jarquin, D., Howard, R., Liang, Z., Gupta, S. K., Schnable, J. C., and Crossa, J. (2020). Enhancing hybrid prediction in pearl millet using genomic and/or multi-environment phenotypic information of inbreds. Front. Genet. 10:1294. doi: 10.3389/fgene.2019.01294
Jogaiah, S., Sharathchandra, R. G., Raj, N., Vedamurthy, A. B., and Shetty, H. S. (2014). Development of SCAR marker associated with downy mildew disease resistance in pearl millet (Pennisetum glaucum L.). Mol. Biol. Rep. 41, 7815–7824. doi: 10.1007/s11033-014-3675-7
Jongeneel, V., Estreicher, A., Baxevanis, A. D., Ouellette, B. F. F., Wolfsberg, T. G., Landsman, D., et al. (2001). Expressed sequence tags (ESTs). Bioinforma A Pract Guid to Anal Genes Proteins 10, 57–63.
Kanfany, G., Serba, D. D., Rhodes, D., St Amand, P., Bernardo, A., Gangashetty, P. I., et al. (2020). Genomic diversity in pearl millet inbred lines derived from landraces and improved varieties. BMC Genomics 21:469. doi: 10.1186/s12864-020-06796-4
Kar, S., Tanaka, R., Korbu, L. B., Kholová, J., Iwata, H., Durbha, S. S., et al. (2020). Automated discretization of ‘transpiration restriction to increasing VPD’ features from outdoors high-throughput phenotyping data. Plant Methods 16:140. doi: 10.1186/s13007-020-00680-8
Kholová, J., Hash, C. T., Kakkera, A., Kočová, M., and Vadez, V. (2010a). Constitutive water-conserving mechanisms are correlated with the terminal drought tolerance of pearl millet [Pennisetum glaucum (L.) R. Br.]. J. Exp. Bot. 61, 369–377. doi: 10.1093/jxb/erp314
Kholová, J., Hash, C. T., Kumar, P. L., Yadav, R. S., Kočová, M., and Vadez, V. (2010b). Terminal drought-tolerant pearl millet [Pennisetum glaucum (L.) R. Br.] have high leaf ABA and limit transpiration at high vapour pressure deficit. J. Exp. Bot. 61, 1431–1440. doi: 10.1093/jxb/erq013
Kholová, J., Nepolean, T., Hash, C. T., Supriya, A., Rajaram, V., Senthilvel, S., et al. (2012). Water saving traits co-map with a major terminal drought tolerance quantitative trait locus in pearl millet [Pennisetum glaucum (L.) R. Br.]. Mol. Breed. 30, 1337–1353. doi: 10.1007/s11032-012-9720-0
Kholová, J., and Vadez, V. (2013). Water extraction under terminal drought explains the genotypic differences in yield, not the anti-oxidant changes in leaves of pearl millet (Pennisetum glaucum). Funct. Plant Biol. 40, 44–53. doi: 10.1071/FP12181
Kholová, J., Zindy, P., Malayee, S., Baddam, R., Murugesan, T., Kaliamoorthy, S., et al. (2016). Component traits of plant water use are modulated by vapour pressure deficit in pearl millet (Pennisetum glaucum (L.) R. Br.). Funct. Plant Biol. 43, 423–437. doi: 10.1071/FP15115
Kole, C., Muthamilarasan, M., Henry, R., Edwards, D., Sharma, R., Abberton, M., et al. (2015). Application of genomics-assisted breeding for generation of climate resilient crops: progress and prospects. Front. Plant Sci. 6:563. doi: 10.3389/fpls.2015.00563
Kulwal, P., Ishikawa, G., Benscher, D., Feng, Z., Yu, L. X., Jadhav, A., et al. (2012). Association mapping for pre-harvest sprouting resistance in white winter wheat. Theor. Appl. Genet. 125, 793–805. doi: 10.1007/s00122-012-1872-0
Kumar, A., and Andrews, D. J. (1993). Genetics of quantitative traits in pearl millet: a review. Crop Sci. 33, 1–20. doi: 10.2135/cropsci1993.0011183x003300010001x
Kumar, A. K., and Appa Rao, S. (1987). “Diversity and utilization of pearl millet germplasm,” in Proceedings of the International Pearl Millet Workshop, eds J. R. Witcombe and S. R. Beckerman (India: ICRISAT Centre), 69–82.
Kumar, S., Hash, C., Nepolean, T., Mahendrakar, M., Satyavathi, C., Singh, G., et al. (2018). Mapping grain iron and zinc content quantitative trait loci in an iniadi-derived immortal population of pearl millet. Genes 9:248. doi: 10.3390/genes9050248
Kumar, S., Hash, C. T., Thirunavukkarasu, N., Singh, G., Rajaram, V., and Rathore, A. (2016). Mapping quantitave trait loci controlling high iron and zinc in self and open pollinated grains of pearl millet [Pennisetum glaucum (L) R. Br.]. Front. Plant Sci. 7:1636. doi: 10.3389/fpls.2016.01636
Lahiri, A. N., and Kumar, V. (1966). Studies on plant water relationship III. Further studies on the drought mediated alterations in the performance of bulrush millet. Proc. Natl. Acad. Sci. India B 32, 116–129.
Liang, Z., Gupta, S. K., Yeh, C. T., Zhang, Y., Ngu, D. W., Kumar, R., et al. (2018). Phenotypic data from inbred parents can improve genomic prediction in pearl millet hybrids. G3 8, 2513–2522. doi: 10.1534/g3.118.200242
Liu, C. J., Witcombe, J. R., Pittaway, T. S., Nash, M., Hash, C. T., Busso, C. S., et al. (1994). An RFLP-based genetic map of pearl millet (Pennisetum glaucum). Theor. Appl. Genet. 89, 481–487. doi: 10.1007/BF00225384
Liu, C. J., Witcombe, J. R., Pittaway, T. S., Nash, M., Hash, C. T., and Gale, M. D. (1992). “Restriction fragment length polymorphism in pearl millet, Pennisetum glaucum,” in International Colloquium. Plant species complexes, gene flow and genetic resources, eds J. C. Mounolou and A. Sarr (Paris: Bureau des Ressources Génétiques), 233–241.
Mahalakshmi, V., Bidinger, F. R., and Raju, D. S. (1987). Effect of timing of water deficit on pearl millet (Pennisetum americanum). Field Crops Res. 15, 327–339. doi: 10.1016/0378-4290(87)90020-7
Manning, K., Pelling, R., Higham, T., Schwenniger, J. L., and Fuller, D. Q. (2011). 4500-Year old domesticated pearl millet (Pennisetum glaucum) from the Tilemsi Valley, Mali: new insights into an alternative cereal domestication pathway. J. Arch. Sci. 38, 312–322. doi: 10.1016/j.jas.2010.09.007
Mariac, C., Jehin, L., Saïdou, A. A., Thuillet, A. C., Couderc, M., and Sire, P. (2011). Genetic basis of pearl millet adaptation along an environmental gradient investigated by a combination of genome scan and association mapping. Mol. Ecol. 20, 80–91. doi: 10.1111/j.1365-294X.2010.04893.x
Mariac, C., Luong, V., Kapran, I., Mamadou, A., Sagnard, F., Deu, M., et al. (2006). Diversity of wild and cultivated pearl millet accessions (Pennisetum glaucum [L.] R. Br.) in Niger assessed by microsatellite markers. Theor. Appl. Genet. 114, 49–58. doi: 10.1007/s00122-006-0409-9
Medina, S., Gupta, S. K., and Vadez, V. (2017). Transpiration Response and Growth in Pearl Millet Parental Lines and Hybrids Bred for Contrasting Rainfall Environments. Front. Plant Sci. 8:1846. doi: 10.3389/fpls.2017.01846
Meuwissen, T. H. E., Hayes, B. J., and Goddard, M. E. (2001). Prediction of total genetic value using genome-wide dense marker maps. Genetics 157, 1819–1829. doi: 10.1093/genetics/157.4.1819
Mohan, M., Nair, S., Bhagwat, A., Krishna, T. G., Yano, M., Bhatia, C. R., et al. (1997). Genome mapping, molecular markers and marker-assisted selection in crop plants. Mol. Breed. 3, 87–103. doi: 10.1023/A:1009651919792
Moumouni, K. H., Kountche, B. A., Jean, M., Hash, C. T., Vigouroux, Y., Haussmann, B. I. G., et al. (2015). Construction of a genetic map for pearl millet, Pennisetum glaucum (L.) R. Br., using a genotyping-by-sequencing (GBS) approaches. Mol. Breed. 35:5. doi: 10.1007/s11032-015-0212-x
Mukesh Sankar, S., Tara Satyavathi, C., Barthakur, S., Singh, S. P., Bharadwaj, C., and Soumya, S. L. (2021). Differential modulation of heat-inducible genes across diverse genotypes and molecular cloning of a sHSP from pearl millet [Pennisetum glaucum (L.) R. Br.]. Front. Plant Sci. 12:659893. doi: 10.3389/fpls.2021.659893
Murty, B. R., Upadhyaya, M. K., and Manchanda, P. L. (1967). Classification and cataloguing of a world collection of genetic stocks of Pennisetum. Indian J. Genet. 27, 313–394.
Nielsen, N. H., Jahoor, A., Jensen, J. D., Orabi, J., Cericola, F., Edriss, V., et al. (2016). Genomic prediction of seed quality traits using advanced barley breeding lines. PLoS One 11:e0164494. doi: 10.1371/journal.pone.0164494
Norman, A., Taylor, J., Tanaka, E., Telfer, P., Edwards, J., Martinant, J. P., et al. (2017). Increased genomic prediction accuracy in wheat breeding using a large Australian panel. Theor. Appl. Genet. 130, 2543–2555. doi: 10.1007/s00122-017-2975-4
Patil, K. S., Gupta, S. K., Marathi, B., Danam, S., Thatikunta, R., Rathore, A., et al. (2020). African and Asian origin pearl millet populations: genetic diversity pattern and its association with yield heterosis. Crop Sci. 60, 3035–3048. doi: 10.1002/csc2.20245
Pedraza-Garcia, F., Specht, J. E., and Dweikat, I. (2010). A new PCR-based linkage map in pearl millet. Crop Sci. 50, 1754–1760. doi: 10.1016/j.ab.2008.07.012
Ponnaiah, G., Gupta, S. K., Blümmel, M., Marappa, M., Pichaikannu, S., Das, R. R., et al. (2019). Utilization of molecular marker based genetic diversity patterns in hybrid parents to develop better forage quality multi-cut hybrids in pearl millet. Agriculture 9:97. doi: 10.3390/agriculture9050097
Presterl, T., and Weltzien, E. (2003). Exploiting heterosis in pearl millet population breeding in arid environments. Crop Sci. 43, 767–776. doi: 10.2135/cropsci2003.0767
Pucher, A., Hash, C. T., Wallace, J. G., Han, S., Leiser, W. L., and Haussmann, B. I. (2018). Mapping a male-fertility restoration locus for the A 4 cytoplasmic-genic male-sterility system in pearl millet using a genotyping-by-sequencing-based linkage map. BMC Plant Biol. 18:65. doi: 10.1186/s12870-018-1267-8
Punnuri, S. M., Wallace, J. G., Knoll, J. E., Hyma, K. E., Mitchell, S. E., Buckler, E. S., et al. (2016). Development of a high-density linkage map and tagging leaf spot resistance in pearl millet using genotyping-by-sequencing markers. Plant Genome 9, 2015–2010. doi: 10.3835/plantgenome2015.10.0106
Qi, X., Lindup, S., Pittaway, T. S., Allouis, S., Gale, M. D., and Devos, K. M. (2001). Development of simple sequence repeat markers from bacterial artificial chromosomes without subcloning. BioTechniques 31, 355–362. doi: 10.2144/01312st08
Qi, X., Pittaway, T. S., Lindup, S., Liu, H., Waterman, E., Padi, F. K., et al. (2004). An integrated genetic map and a new set of simple sequence repeat markers for pearl millet (Pennisetum glaucum). Theor. Appl. Genet. 109, 1485–1493. doi: 10.1007/s00122-004-1765-y
Rai, K. N., Kumar, A. K., Andrews, D. J., Gupta, S. C., and Ouendeba, B. (1997). “Breeding pearl millet for grain yield and stability,” in Proceedings of international conference on genetic improvement of sorghum pearl millet, Lubbock, Texas, Lubbock, Texas (Lincoln: INTSORMIL), 71–83.
Rai, K. N., Yadav, O. P., Rajpurohit, B. S., Patil, H. T., Govindaraj, M., Khairwal, I. S., et al. (2013). Breeding pearl millet cultivars for high iron density with zinc density as an associated trait. J. SAT Agric. Res. 11, 1–7.
Rajaram, V., Nepolean, T., Senthilvel, S., Varshney, R. K., Vadez, V., Srivastava, R. K., et al. (2013). Pearl millet [Pennisetum glaucum (L.) R. Br.] consensus linkage map constructed using four RIL mapping populations and newly developed EST-SSRs. BMC Genomics 14:159. doi: 10.1186/1471-2164-14-159
Rajaram, V., Varshney, R. K., Vadez, V., Nepolean, T., Senthilvel, S., and Kholova, J. (2010). Development of EST resources in pearl millet and their use in development and mapping of EST-SSRs in four RIL populations. Plant Anim. Gen. 18, 9–13.
Ramya, A. R., Ahamed, M. L., Satyavathi, C. T., Rathore, A., Katiyar, P., Raj, A. G. B., et al. (2018). Towards Defining Heterotic Gene Pools in Pearl Millet [Pennisetum glaucum (L.) R. Br.]. Front. Plant Sci. 8:1934. doi: 10.3389/fpls.2017.01934
Rattunde, H. F., Singh, P., and Witcombe, J. R. (1989). Feasibility of mass selection in pearl millet. Crop Sci. 29, 1423–1427. doi: 10.2135/cropsci1989.0011183X002900060018x
Saïdou, A. A., Mariac, C., Luong, V., Pham, J. L., Bezançon, G., and Vigouroux, Y. (2009). Association studies identify natural variation at PHYC linked to flowering time and morphological variation in pearl millet. Genetics 182, 899–910. doi: 10.1534/genetics.109.102756
Sehgal, D., Rajaram, V., Vadez, V., Hash, C. T., and Yadav, R. S. (2012). Integration of gene based markers in pearl millet genetic map for identification of candidate genes underlying drought tolerance quantitative trait loci. BMC Plant Biol. 12:9. doi: 10.1186/1471-2229-12-9
Sehgal, D., Skot, L., Singh, R., Srivastava, R. K., Das, S. P., Taunk, J., et al. (2015). Exploring potential of pearl millet germplasm association panel for association mapping of drought tolerance traits. PLoS One 10:e0122165. doi: 10.1371/journal.pone.0122165
Senthilvel, S., Jayashree, B., Mahalakshmi, V., Kumar, P. S., Nakka, S., Nepolean, T., et al. (2008). Development and mapping of simple sequence repeat markers for pearl millet from data mining of expressed sequence tags. BMC Plant Biol. 8:119. doi: 10.1186/1471-2229-8-119
Senthilvel, S., Nepolean, T., Supriya, A., Rajaram, V., Kumar, S., Hash, C. T., et al. (2010). “Development of a molecular linkage map of pearl millet integrating DArT and SSR markers,” in Proc. Plant Animal Genome 18 Conference (San Diego), 9–13.
Serba, D. D., Muleta, K. T., St. Amand, P., Bernardo, A., Bai, G., Perumal, R., et al. (2019). Genetic diversity, population structure, and linkage disequilibrium of pearl millet. Plant Genome 12:180091. doi: 10.3835/plantgenome2018.11.0091
Serba, D. D., and Yadav, R. S. (2016). Genomic tools in pearl millet breeding for drought tolerance: status and prospects. Front. Plant Sci. 7:1724. doi: 10.3389/fpls.2016.01724
Serraj, R., Hash, C. T., Rizvi, S. M. H., Sharma, A., Yadav, R. S., and Bidinger, F. R. (2005). Recent advances in marker-assisted selection for drought tolerance in pearl millet. Plant Prod. Sci. 8, 334–337. doi: 10.1626/pps.8.334
Sharma, A. (2001). Marker-Assisted Improvement of Pearl Millet (Pennisetum glaucum) Downy Mildew Resistance in Elite Hybrid Parental line H 77/833-2. Doctoral dissertation. Hisar: CCS Haryana Agricultural University.
Sharma, P. C., Sehgal, D., Singh, D., Singh, G., and Yadav, R. S. (2011). A major terminal drought tolerance QTL of pearl millet is also associated with reduced salt uptake and enhanced growth under salt stress. Mol. Breed. 27, 207–222. doi: 10.1007/s11032-010-9423-3
Sharma, P. C., Singh, D., Sehgal, D., Singh, G., Hash, C. T., and Yadav, R. S. (2014). Further evidence that a terminal drought tolerance QTL of pearl millet is associated with reduced salt uptake. Environ. Exp. Bot. 102, 48–57. doi: 10.1016/j.envexpbot.2014.01.013
Shikha, M., Kanika, A., Rao, A. R., Mallikarjuna, M. G., Gupta, H. S., and Nepolean, T. (2017). Genomic selection for drought tolerance using genome-wide SNPs in maize. Front. Plant Sci. 8:550. doi: 10.3389/fpls.2017.00550
Shivhare, R., Asif, M. H., and Lata, C. (2020). Comparative transcriptome analysis reveals the genes and pathways involved in terminal drought tolerance in pearl millet. Plant Mol. Biol. 103, 639–652. doi: 10.1007/s11103-020-01015-w
Shivhare, R., and Lata, C. (2017). Exploration of genetic and genomic resources for abiotic and biotic stress tolerance in pearl millet. Front. Plant Sci. 7:2069. doi: 10.3389/fpls.2016.02069
Shivhare, R., and Lata, C. (2019). Assessment of pearl millet genotypes for drought stress tolerance at early and late seedling stages. Acta Physiol. Plant. 41:39. doi: 10.1007/s11738-019-2831-z
Siband, P. (1983). Essai d’analyse du fonctionnement du mil (Pennisetum typhoides) en zone Sahelienne. Agron. Trop. 38, 27–36.
Singh, P., Boote, K. J., Kadiyala, M., Nedumaran, S., Gupta, S. K., Srinivas, K., et al. (2017). An assessment of yield gains under climate change due to genetic modification of pearl millet. Sci. Total Environ. 60, 1226–1237. doi: 10.1016/j.scitotenv.2017.06.002
Singh, R. B., Mahenderakar, M. D., Jugran, A. K., Singh, R. K., and Srivastava, R. K. (2020). Assessing genetic diversity and population structure of sugarcane cultivars, progenitor species and genera using microsatellite (SSR) markers. Gene 753:144800. doi: 10.1016/j.gene.2020.144800
Sivasakthi, K., Marques, E., Kalungwana, N., Carrasquilla-Garcia, N., Chang, P. L., Bergmann, E. M., et al. (2019). Functional Dissection of the chickpea (Cicer arietinum L.) stay-green phenotype associated with molecular variation at an ortholog of Mendel’s I gene for cotyledon color: implications for crop production and carotenoid biofortification. Int. J. Mol. Sci. 20:5562. doi: 10.3390/ijms20225562
Sivasakthi, K., Thudi, M., Tharanya, M., Kale, S. M., Kholová, J., Halime, M. H., et al. (2018). Plant vigour QTLs co-map with an earlier reported QTL hotspot for drought tolerance while water saving QTLs map in other regions of the chickpea genome. BMC Plant Biol. 18:29. doi: 10.1186/s12870-018-1245-1
Srivastava, R. K., Katiyar, P., and Mahendrakar, M. D. (2015). “Improving pearl millet using genomics and molecular breeding tools,” in The 2nd International Conference on Bio-resource and Stress Management (ICBSM) (Hyderabad: PJTSAU), 142–146.
Srivastava, R. K., Singh, R. B., Srikanth, B., Satyavathi, C. T., Yadav, R., and Gupta, R. (2020a). Genome-wide association studies (GWAS) and genomic selection (GS) in pearl millet: advances and prospects. Front. Genet. 10:1389. doi: 10.3389/fgene.2019.01389
Srivastava, R. K., Bollam, S., Pujarula, V., Pusuluri, M., Singh, R. B., Potupureddi, G., et al. (2020b). Exploitation of heterosis in pearl millet: a review. Plants 9:807. doi: 10.3390/plants9070807
Srivastava, R. K., Vadez, V., Kholova, J., Lachagari, V. B. R., Mahendrakar, M. D., Katiyar, P., et al. (2017). “Fine mapping of the linkage group 2 drought tolerance QTL in pearl millet [Pennisetum glaucum (L.) R. Br.],” in Conference: InterDrought-V (India: ICRISAT).
Sun, M., Huang, D., Zhang, A., Khan, I., Yan, H., Wang, X., et al. (2020). Transcriptome analysis of heat stress and drought stress in pearl millet based on Pacbio full-length transcriptome sequencing. BMC Plant Biol. 20:323. doi: 10.1186/s12870-020-02530-0
Supriya, A., Senthilvel, S., Nepolean, T., Eshwar, K., Rajaram, V., Shaw, R., et al. (2011). Development of a molecular linkage map of pearl millet integrating DArT and SSR markers. Theor. Appl. Genet. 123, 239–250. doi: 10.1007/s00122-011-1580-1
Tara Satyavathi, C., Ambawat, S., Sehgal, D., Lata, C., Tiwari, S., Srivastava, R. K., et al. (2021). “Genomic Designing for Abiotic Stress Tolerance in Pearl Millet [Pennisetum glaucum (L.) R. Br.],” in Genomic Designing for Abiotic Stress Resistant Cereal Crops, ed. C. Kole (Cham: Springer), 223–253. doi: 10.1007/978-3-030-75875-2_6
Tharanya, M., Kholova, J., Sivasakthi, K., Seghal, D., Hash, C. T., Raj, B., et al. (2018). Quantitative trait loci (QTLs) for water use and crop production traits co-locate with major QTL for tolerance to water deficit in a fine-mapping population of pearl millet (Pennisetum glaucum L. R. Br.). Theor. Appl. Genet. 131, 1509–1529. doi: 10.1007/s00122-018-3094-6
Tharanya, M., Kholova, J., Sivasakthi, K., Thirunalasundari, T., and Vadez, V. (2017). “Pearl millet,” in Water-Conservation Traits to Increase Crop Yields in Water-Deficit Environments: Case Studies, ed. T. R. Sinclair (Springer: Berlin), 73–83.
Thompson, A. J., Andrews, J., Mulholland, B. J., McKee, J. M., Hilton, H. W., Horridge, J. S., et al. (2007). Overproduction of abscisic acid in tomato increases transpiration efficiency and root hydraulic conductivity and influences leaf expansion. Plant Physiol. 143, 1905–1917. doi: 10.1104/pp.106.093559
van Oosterom, E. J., Weltzien, E., Yadav, O. P., and Bidinger, F. R. (2006). Grain yield components of pearl millet under optimum conditions can be used to identify germplasm with adaptation to arid zones. Field Crops Res. 96, 407–421.
Vadez, V., Hash, T., and Kholova, J. (2012). II. 1.5 Phenotyping pearl millet for adaptation to drought. Front. Physiol. 3:386. doi: 10.3389/fphys.2012.00386
Vadez, V., Kholová, J., Hummel, G., Zhokhavets, U., Gupta, S. K., and Hash, C. T. (2015). LeasyScan: a novel concept combining 3D imaging and lysimetry for high-throughput phenotyping of traits controlling plant water budget. J. Exp. Bot. 66, 5581–5593. doi: 10.1093/jxb/erv251
Vadez, V., Kholová, J., Yadav, R. S., and Hash, C. T. (2013). Small temporal differences in water uptake among varieties of pearl millet (Pennisetum glaucum (L.) R Br) are critical for grain yield under terminal drought. Plant Soil 371, 447–462. doi: 10.1007/s11104-013-1706-0
Vadez, V., Krishnamurthy, L., Hash, C. T., Upadhyaya, H. D., and Borrell, A. K. (2011). Yield, Transpiration efficiency, and water use variations and their relationships in sorghum reference collection. Crop Pasture Sci. 62, 645–655. doi: 10.1071/CP11007
Vadez, V., and Ratnakumar, P. (2016). High transpiration efficiency increases pod yield under intermittent drought in dry and hot atmospheric conditions but less so under wetter and cooler conditions in groundnut (Arachis hypogaea (L.)). Field Crops Res. 193, 16–23. doi: 10.1016/j.fcr.2016.03.001
van Oosterom, E. J., Bidinger, F. R., and Weltzien, E. R. (2003). A yield architecture framework to explain adaptation of pearl millet to environmental stress. Field Crops Res. 80, 33–56. doi: 10.1016/S0378-4290(02)00153-3
Van Oosterom, E. J., Mahalakshmi, V., Arya, G. K., Dave, H. R., Gothwal, B. D., Joshi, A. K., et al. (1995). Effect of yield potential, drought escape and drought tolerance on yield of pearl millet (Pennisetum glaucum) in different stress environments. Indian J. Agric. Sci. 65, 629–635.
Varshney, R. K., Graner, A., and Sorrells, M. E. (2005). Genomics-assisted breeding for crop improvement. Trends Plant Sci. 10, 621–630. doi: 10.1016/j.tplants.2005.10.004
Varshney, R. K., Shi, C., Thudi, M., Mariac, C., Wallace, J., Qi, P., et al. (2017). Pearl millet genome sequence provides a resource to improve agronomic traits in arid environments. Nat. Biotech. 35, 969–976. doi: 10.1038/nbt.3943
Vengadessan, V., Rai, K. N., Kannan Bapu, J. R., Hash, C. T., Bhattacharjee, R., Senthilvel, S., et al. (2013). Construction of genetic linkage map and QTL analysis of sink-size traits in pearl millet (Pennisetum glaucum). Int. Sch. Res. Notices 2013:471632. doi: 10.5402/2013/471632
Wang, C., Guo, L., Li, Y., and Wang, Z. (2012). Systematic comparison of C3 and C4 plants based on metabolic network analysis. BMC Syst. Biol. 6:S9–S9. doi: 10.1186/1752-0509-6-S2-S9
Winkel, T., and Do, F. (1992). Caracteres morphologiques et physiologiques de resistance du mil [Pennisetum glaucum (L.) R. Br.] a la secheresse. Agron. Trop. 46, 339–350.
Winkel, T., Renno, J. F., and Payne, W. A. (1997). Effect of the timing of water deficit on growth, phenology and yield of pearl millet (Pennisetum glaucum (L) R Br) grown in Sahelian conditions. J. Exp. Bot. 48, 1001–1009. doi: 10.1093/jxb/48.5.1001
Yadav, O. P. (1994). Inheritance of threshing percentage in pearl millet. Euphytica 78, 77–80. doi: 10.1007/bf00021400
Yadav, O. P. (2004). CZP 9802- A new drought-tolerant cultivar of pearl millet. Indian Farming 54, 15–17.
Yadav, O. P. (2006). Heterosis in crosses between landraces and elite exotic populations of pearl millet (Pennisetum glaucum (L.) R. Br.) in arid zone environments. Indian J. Genet. Plant Breed. 66, 308–311.
Yadav, O. P. (2008). Performance of landraces exotic elite populations and their crosses in pearl millet (Pennisetum glaucum) in drought and non-drought conditions. Plant Breed. 127, 208–210. doi: 10.1111/j.1439-0523.2007.01467.x
Yadav, O. P. (2010). Drought response of pearl millet landrace-based populations and their crosses with elite composites. Field Crops Res. 118, 51–57. doi: 10.1016/j.fcr.2010.04.005
Yadav, O. P. (2014). Developing drought-resilient crops for improving productivity of drought-prone ecologies. Indian J. Genet. Plant Breed. 74, 548–552. doi: 10.5958/0975-6906.2014.00887.6
Yadav, O. P., and Bhatnagar, S. K. (2001). Evaluation of indices for identification of pearl millet cultivars adapted to stress and non-stress conditions. Field Crops Res. 70, 201–208. doi: 10.1016/S0378-4290(01)00138-1
Yadav, O. P., and Bidinger, F. R. (2007). Utilization, diversification and improvement of landraces for enhancing pearl millet productivity in arid environments. Ann. Arid Zone 46, 49–57.
Yadav, O. P., Bidinger, F. R., and Singh, D. V. (2009). Utility of pearl millet landraces in breeding dual-purpose hybrids for arid zone environments of India. Euphytica 166, 239–247. doi: 10.1007/s10681-008-9834-y
Yadav, O. P., and Manga, V. K. (1999). “Diversity in pearl millet landraces of arid regions of Rajasthan,” in Management of Arid Ecosystem, eds A. S. Faroda, N. L. Joshi, S. Kathju, and A. Kar (Jodhpur: Arid Zone Research Association of India & Scientific Publishers), 57–60.
Yadav, O. P., Mitchell, S. E., Fulton, T. M., and Kresovich, S. (2008). Transferring molecular markers from sorghum, rice and other cereals to pearl millet and identifying polymorphic markers. J. Semi Arid Trop. Agric. Res. 6, 1–4.
Yadav, O. P., Mitchell, S. E., Zamora, A., Fulton, T. M., and Kresovich, S. (2007). Development of new simple sequence repeat markers for pearl millet. J. Semi Arid Trop. Agric. Res. 3, 34–37.
Yadav, O. P., and Rai, K. N. (2011). Hybridization of Indian landraces and African elite composites of pearl millet results in biomass and stover yield improvement under arid zone conditions. Crop Sci. 51, 1980–1987. doi: 10.2135/cropsci2010.12.0731
Yadav, O. P., Rajpurohit, B. S., Kherwa, G. R., and Kumar, A. (2012a). Prospects of enhancing pearl millet (Pennisetum glaucum) productivity under drought environments of north-western India through hybrids. Indian J. Genet. Plant Breed. 72, 25–30.
Yadav, O. P., Rai, K. N., Bidinger, F. R., Gupta, S. K., Rajpurohit, B. S., and Bhatnagar, S. K. (2012b). Pearl millet (Pennisetum glaucum) restorer lines for breeding dual-purpose hybrids adapted to arid environments. Indian J. Agric. Sci. 82, 922–927.
Yadav, O. P., Rai, K. N., and Gupta, S. K. (2012c). “Pearl millet: genetic improvement for tolerance to abiotic stresses,” in Improving Crop Resistance to Abiotic Stress, eds N. Tuteja, S. S. GilL, and R. Tuteja (Weinheim: Wlley VCH Verlag GmbH & Co. KGaA), 261–288.
Yadav, O. P., Upadhyaya, H. D., Reddy, K. N., Jukanti, A. K., Pandey, S., and Tyagi, R. K. (2017). Genetic resources of pearl millet: status and utilization. Indian J. Plant Genet. Resour. 30, 31–47. doi: 10.5958/0976-1926.2017.00004.3
Yadav, O. P., and Weltzien, R. E. (2000). Differential response of pearl millet landrace-based populations and high yielding varieties in contrasting environments. Ann. Arid Zone 39, 39–45.
Yadav, O. P., Weltzien-Rattunde, E., and Bidinger, F. R. (2003). Genetic variation in drought response of landraces of pearl millet (Pennisetum glaucum (L.) R. Br.). Indian J. Genet. 63, 37–40.
Yadav, O. P., Weltzien-Rattunde, E., Bidinger, F. R., and Mahalakshmi, V. (2000). Heterosis in landrace-based topcross hybrids of pearl millet across arid environments. Euphytica 112, 285–295. doi: 10.1023/A:1003965025727
Yadav, R. S., Bidinger, F. R., Hash, C. T., Cavan, G. P., Serraj, R., and Howarth, C. J. (2005). Improving pearl millet drought tolerance. Int. Sorghum Millets Newsl. 42, 15–16.
Yadav, R. S., Hash, C. T., Bidinger, F. R., Cavan, G. P., and Howarth, C. L. (2002). Quantitative trait loci associated with traits determining grain and stover yield in pearl millet under terminal drought stress conditions. Theor. Appl. Genet. 104, 67–83. doi: 10.1007/s001220200008
Yadav, R. S., Hash, C. T., Bidinger, F. R., Devos, K. M., and Howarth, C. J. (2004). Genomic regions associated with grain yield and aspects of post-flowering drought tolerance in pearl millet across stress environments and tester background. Euphytica 136, 265–277. doi: 10.1023/B:EUPH.0000032711.34599.3a
Yadav, R. S., Sehgal, D., and Vadez, V. (2011). Using genetic mapping and genomics approaches in understanding and improving drought tolerance in pearl millet. J. Exp. Bot. 62, 397–408. doi: 10.1093/jxb/erq265
Keywords: drought stress, drought mechanism, drought tolerance, genetic resources, genomic resources
Citation: Srivastava RK, Yadav OP, Kaliamoorthy S, Gupta SK, Serba DD, Choudhary S, Govindaraj M, Kholová J, Murugesan T, Satyavathi CT, Gumma MK, Singh RB, Bollam S, Gupta R and Varshney RK (2022) Breeding Drought-Tolerant Pearl Millet Using Conventional and Genomic Approaches: Achievements and Prospects. Front. Plant Sci. 13:781524. doi: 10.3389/fpls.2022.781524
Received: 22 September 2021; Accepted: 11 February 2022;
Published: 07 April 2022.
Edited by:
Diaga Diouf, Cheikh Anta Diop University, SenegalReviewed by:
Kuldeep Kumar, Indian Institute of Pulses Research (ICAR), IndiaCopyright © 2022 Srivastava, Yadav, Kaliamoorthy, Gupta, Serba, Choudhary, Govindaraj, Kholová, Murugesan, Satyavathi, Gumma, Singh, Bollam, Gupta and Varshney. This is an open-access article distributed under the terms of the Creative Commons Attribution License (CC BY). The use, distribution or reproduction in other forums is permitted, provided the original author(s) and the copyright owner(s) are credited and that the original publication in this journal is cited, in accordance with accepted academic practice. No use, distribution or reproduction is permitted which does not comply with these terms.
*Correspondence: Rakesh K. Srivastava, ci5rLnNyaXZhc3RhdmFAY2dpYXIub3Jn; Rajeev K. Varshney, ci5rLnZhcnNobmV5QGNnaWFyLm9yZw==
Disclaimer: All claims expressed in this article are solely those of the authors and do not necessarily represent those of their affiliated organizations, or those of the publisher, the editors and the reviewers. Any product that may be evaluated in this article or claim that may be made by its manufacturer is not guaranteed or endorsed by the publisher.
Research integrity at Frontiers
Learn more about the work of our research integrity team to safeguard the quality of each article we publish.