- 1Department of Plant Sciences, University of Cambridge, Cambridge, United Kingdom
- 2Leibniz Centre for Agricultural Landscape Research (ZALF), Müncheberg, Germany
- 3Institute of Molecular Biology and Biotechnology (IMBB), The University of Lahore, Punjab, Pakistan
Phosphate deprivation compromises plant productivity and modulates immunity. DAMP signalling by extracellular ATP (eATP) could be compromised under phosphate deprivation by the lowered production of cytosolic ATP and the need to salvage eATP as a nutritional phosphate source. Phosphate-starved roots of Arabidopsis can still sense eATP, indicating robustness in receptor function. However, the resultant cytosolic free Ca2+ signature is impaired, indicating modulation of downstream components. This perspective on DAMP signalling by extracellular ATP (eATP) addresses the salvage of eATP under phosphate deprivation and its promotion of immunity, how Ca2+ signals are generated and how the Ca2+ signalling pathway could be overcome to allow beneficial fungal root colonization to fulfill phosphate demands. Safe passage for an endophytic fungus allowing root colonization could be achieved by its down-regulation of the Ca2+ channels that act downstream of the eATP receptors and by also preventing ROS accumulation, thus further impairing DAMP signalling.
Introduction
Phosphate (Pi) deprivation is readily experienced in the field without fertilizer input (Alewell et al., 2020) and leads to lower cellular and cytosolic Pi levels within minutes (Duff et al., 1989; Pratt et al., 2009). Deficiency triggers a shift to alternative metabolic pathways which consume less Pi (Duff et al., 1989; Plaxton and Tran, 2011; Pant et al., 2015) and phosphorylated metabolites decrease (Pant et al., 2015). Phospholipids are remodelled into sulpho- and glycolipids, restricted to the cytoplasmic leaflet of the plasma membrane (Andersson et al., 2005; Tjellström et al., 2010; Nakamura, 2013; Okazaki et al., 2013). This remodelling could be part of the restructuring of signalling systems. Indeed, Pi deprivation attenuates the cytosolic free Ca2+ ([Ca2+]cyt) signalling response to mechanical stress, salinity, and osmotic stress in Arabidopsis roots (Matthus et al., 2019a; Matthus et al., 2022).
It is now increasingly recognized that Pi availability and homeostasis are intricately linked with plant immunity signalling (Castrillo et al., 2017; Dindas et al., 2022; Tang et al., 2022; Val-Torregrosa et al., 2022). Under low Pi, plants initiate the Phosphate Starvation Response (PSR) driven by the MYB transcription factor Phosphate Starvation Response1 (PHR1) to modulate not only growth and metabolism but the composition of the plant’s microbiota (well beyond the interaction with mycorrhizal fungi) to favour those mineralizing poorly accessible Pi sources to promote Pi nutrition. Achieving this may involve modulating immunity, indeed PHR1 negatively regulates transcription of genes responding to the Pathogen Associated Molecular Pattern (PAMP) bacterial peptide flg22 (Castrillo et al., 2017; Isidra-Arellano et al., 2021). In roots of Arabidopsis thaliana (as a non-host for mycorrhizal fungi), part of the PSR is the production of a subset of anti-immunity RALF (Rapid Alkalinization Factor) peptides that are perceived by the plasma membrane Feronia receptor. This is then thought to disrupt perception of flg22 by the FLS2/BAK1 (BRASSINOSTEROID INSENSITIVE 1-ASSOCIATED RECEPTOR KINASE 1) receptor complex to lower immunity (Tang et al., 2022). However in Pi-starved Arabidopsis root hairs the abundance of the high affinity PHT1.4 transporter is increased in the PSR but PAMPs (including flg22) were found to act through BIK1 to inhibit PHT1.4-mediated Pi uptake (Dindas et al., 2022). Nevertheless, Pi-starved mutants lacking PHT1.4 were less susceptible than wild type to infection by a bacterial pathogen (Ralstonia solanacearum), placing this component as a negative regulator of immunity and consistent with PSR’s modulating defence (Dindas et al., 2022).
Wounding and the presence of microbes causes accumulation of extracellular ATP (eATP) by plants. Mechanical wounding of Arabidopsis roots (Weerasinghe et al., 2009; Dark et al., 2011) and leaves (Song et al., 2006; Myers Jr et al., 2022) increases eATP, consistent with breaches of the plasma membrane’s permitting efflux of cytosolic ATP. The effect is not limited to Arabidopsis; wounding cells of the macroalga Dasycladus vermicularis, roots of carrot (Daucus carota; Gastélum-Estrada et al., 2020) and leaves of kidney bean (Phaseolus vulgaris L) also causes eATP accumulation (Wang et al., 2019). eATP accumulation by Arabidopsis leaves can occur in response to flg22 and Pseudomonas syringae (Chen et al., 2017) but in those cases the mechanistic basis of eATP accumulation is unknown. For roots, eATP increases in barley (Hordeum vulgare) and Arabidopsis during colonization by the fungal endophyte Serendipita indica (Nizam et al., 2019). The significance of such eATP accumulation lies in eATP’s ability to signal wounding or microbial presence as a constitutive DAMP (Damage Associated Molecular Pattern; Choi et al., 2014; Tanaka and Heil, 2021). A constitutive DAMP is a molecule that is present before damage and becomes a signal on moving passively from its “normal” site as a consequence of damage (Tanaka and Heil, 2021); for ATP, this is moving from the cytosol to the extracellular face of the plasma membrane. eATP’s acting as a DAMP is conserved across kingdoms, working in animals and fungi as well as plants but signalling systems differ markedly (Medina-Castellanos et al., 2014; Medina-Castellanos et al., 2018; Verkhratsky, 2021). Studies on eATP signalling are usually conducted on plants grown under optimal nutrient conditions. Given the apparent need to conserve Pi, utilising a Pi-rich signalling molecule such as eATP potentially places plants suffering from Pi deprivation at risk of impaired signalling outcomes. However, in light of immunity modulation in the PSR, this could be a necessary and beneficial risk. After outlining the eATP signalling pathway in defence, this Perspective considers how Pi deprivation is currently known to affect it, how eATP as a nutritional Pi source may link with defence and argues that (although modulated) eATP signalling will remain a key line of defence for microbes to overcome under this abiotic stress.
eATP signalling intersects with multiple pathways
The eATP-regulated Arabidopsis transcriptome is enriched in immune- and wound-response genes (Jeter et al., 2004; Choi et al., 2014; Tripathi et al., 2018; Jewell et al., 2019; Jewell et al., 2022). The signalling pathway from eATP to wounding/immunity transcription runs through the plasma membrane legume-like lectin serine-threonine receptor kinase “DOes not Respond to Nucleotides1” (DORN1/P2K1) and also its co-receptor phosphorylation target P2K2, although whether all cell types deploy this co-receptor is unknown (Choi et al., 2014; Jewell et al., 2019; Pham et al., 2020). Wound-induced inhibition of plant growth is mediated by P2K1 (Shi et al., 2022) and this receptor is required for limiting infection by bacteria, oomycetes and fungi (Gouget et al., 2006; Bouwmeester et al., 2011; Bouwmeester et al., 2014; Balagué et al., 2017; Chen et al., 2017; Tripathi et al., 2018; Nizam et al., 2019; Kumar et al., 2020). Overexpression of P2K1 can confer resistance to insect and nematode attack (Jewell et al., 2022). Potential eATP receptors as P2K1 orthologues have been reported in Camelina sativa (Li et al., 2016) and banana (Musa acuminata; Shan et al., 2020) for example, but there are no reports on cereals. These contain large families of legume-like lectin serine-threonine receptor kinase genes for testing (72 in Oryza sativa (rice) and 84 in Triticum aestivum (bread wheat): Vaid et al., 2012; Shumayla et al., 2016).
In Arabidopsis Pi-replete roots, eATP causes a biphasic increase in [Ca2+]cyt as a second messenger with the first phase generated by the apex followed by a second, sub-apical phase (Figure 1A: Matthus et al., 2019a; Matthus et al., 2019b; Mohammad-Sidik et al., 2021; Matthus et al., 2022; Wang et al., 2022a). This response appears to have an absolute requirement for P2K1 but recently Matthus et al. (2022) reported a small but significant eATP-induced [Ca2+]cyt increase in roots that was independent of this receptor. In Arabidopsis root epidermis (Pi-replete), P2K1 and P2K2 cause an initial Ca2+ influx mediated by the plasma membrane Cyclic Nucleotide Gated Channel CNGC2 (Figure 1B: Wang et al., 2022a). Another CNGC, CNGC6, may also be involved in the root’s response (Duong et al., 2022). CNGC2 is also part of the eATP pathway in cotyledons and pollen grain (Sun et al., 2021; Wu et al., 2021), although its involvement in roots appears restricted to the epidermis (Wang et al., 2022a). CNGC2 may form a connection with PAMP-triggered immunity as it can also operate in flg22 signalling (Tian et al., 2019) and it also works in Jasmonic Acid signalling (Lu et al., 2016). CNGC2 may also form an intersect with abiotic stress signalling and development as it is involved in heat stress signalling (Finka et al., 2012; Katano et al., 2018), high light signalling (Fichman et al., 2021), response to auxin (Chakraborty et al., 2021) and floral transition (Chin et al., 2013). How the P2K1 and P2K2 eATP receptors promote opening of CNGC2 remains unknown; possibilities include phosphorylation or production of cyclic mononucleotides by cryptic cyclase domains (Sun et al., 2021). The resultant elevation of [Ca2+]cyt by CNGC2 or other Ca2+ channels (Wilkins et al., 2016; Jarratt-Barnham et al., 2021) may link to the transcriptional response through downstream elevation of nuclear Ca2+ (Krebs et al., 2012; Loro et al., 2012) and breakdown of the Calmodulin-binding Transcription Activator3, CAMTA3 (Jewell et al., 2019; Jiang et al., 2020).
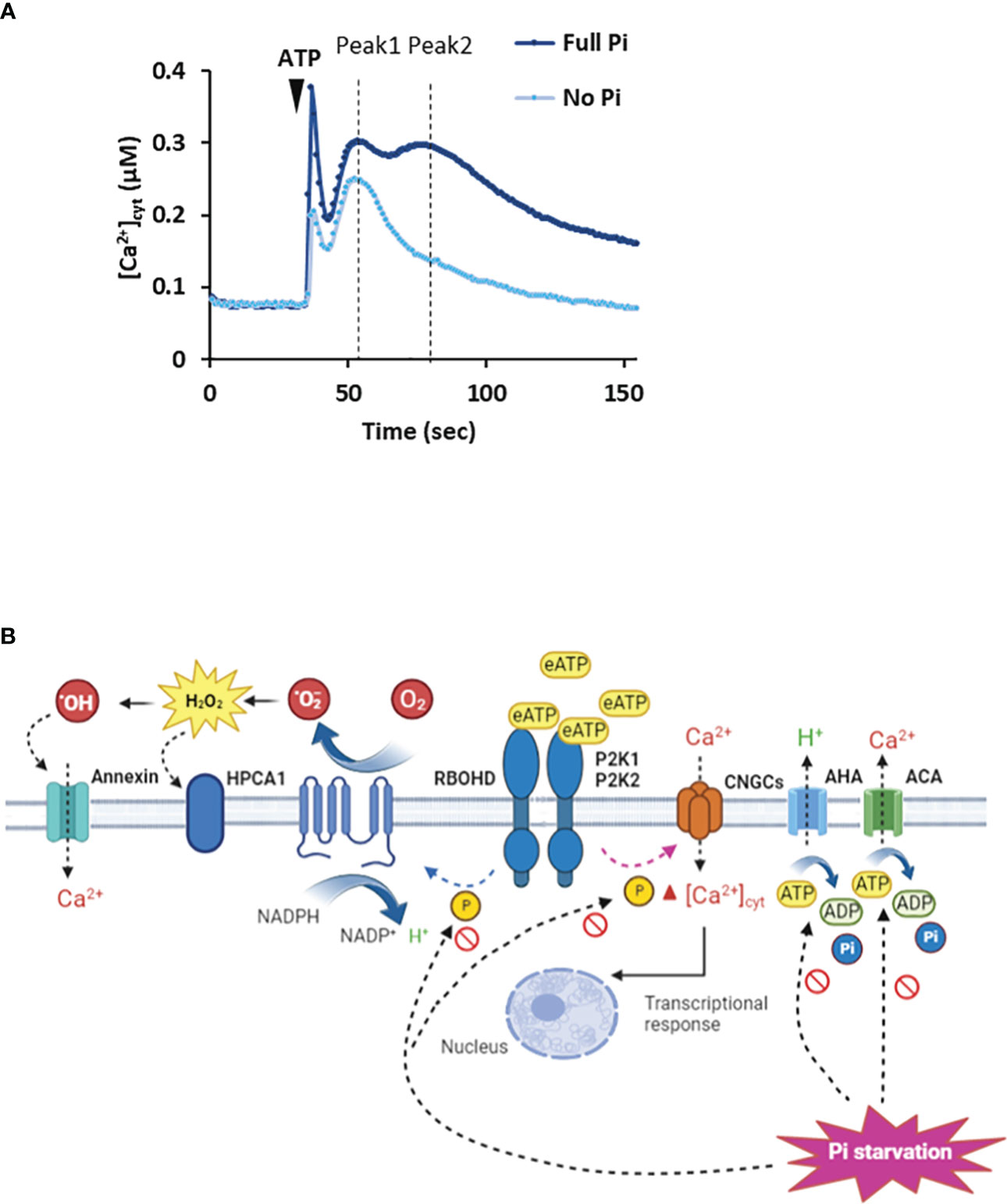
Figure 1 Extracellular ATP causes elevation of [Ca2+]cyt. (A) Arabidopsis roots or root tips (expressing cytosolic aequorin as a [Ca2+]cyt reporter) grown in full Pi medium respond to ATP addition with an initial [Ca2+]cyt increase caused by mechanical perturbation, followed by an ATP-induced biphasic increase (Peak 1, Peak 2). In Pi-starved roots, the magnitude of the Peak responses is lessened. Schema based on results of Matthus et al., 2019a; Matthus et al., 2019b; Mohammad-Sidik et al., 2021; Matthus et al., 2022; Wang et al., 2022a. (B) In Pi-replete Arabidopsis, extracellular ATP (eATP) is recognized by the plasma membrane P2K1 and P2K2 receptors. This can lead to opening of CNGC channels by an unknown mechanism to elevate [Ca2+]cyt (Sun et al., 2021; Wu et al., 2021; Duong et al., 2022; Wang et al., 2022a). In guard cells, P2K1 can activate RBOHD by phosphorylation (Chen et al., 2017) whilst in roots its target may be RBOHC (Demidchik et al., 2009). The resultant extracellular ROS could be sensed by the HPCA1 hydrogen peroxide receptor (Wu et al., 2020) although there is no evidence for this yet. Peroxide could enter the cytosol through aquaporins (not shown) or be converted to hydroxyl radicals to activate Annexin1. H+-ATPases (AHA) promote hyperpolarised membrane voltage (Haruta and Sussman, 2012) to facilitate Ca2+ channel opening whilst Ca2+-ATPase activity would help terminate the [Ca2+]cyt signal (Costa et al., 2017). Under Pi deprivation, cytosolic ATP limitation may impair AHA/Ca2+-ATPase activity and potentially the phosphorylation activity of the receptors. The involvement of P2K2 may be questioned (Matthus et al., 2022). The identities of the Ca2+ channels may change and the involvement of RBOHs has yet to be determined.
Pi deprivation influences the eATP-induced [Ca2+]cyt signatures
Arabidopsis roots deprived of Pi can still respond to eATP with a distinct [Ca2+]cyt increase or “signature” (Matthus et al., 2019a; Matthus et al., 2022). There is no substitution of P2K1 in Pi-deprived roots, it is still absolutely required for the [Ca2+]cyt response (Matthus et al., 2022), indicating a robust perception system that withstands perturbation. Indeed, expression of P2K1 does not respond significantly to Pi starvation whilst P2K1 abundance in roots can even increase (Lin et al., 2011; Lan et al., 2012; Zhang et al., 2020). However, the P2K1-independent component of the [Ca2+]cyt signature was lost on Pi deprivation (Matthus et al., 2022). It could be that this component was generated by P2K2 or unknown receptors, for which evidence is accumulating (Zhu et al., 2017; Matthus et al., 2019b; Zhu R. et al., 2020; Pham et al., 2020; Smith et al., 2021). Although Pi-deprived Arabidopsis roots can still respond to eATP, the spatio-temporal pattern of the [Ca2+]cyt increase is altered with a significantly lower first phase and the abolition of the second, sub-apical response (Figure 1A: Matthus et al., 2019a; Matthus et al., 2022). The downstream consequences of this change are unknown. The position where the sub-apical [Ca2+]cyt increase should occur corresponded with a region of increased cytosolic Reactive Oxygen Species (ROS), most likely hydrogen peroxide (Matthus et al., 2019a). This effect on the [Ca2+]cyt signal increased over days of Pi starvation and was linked to Fe availability (a normal response was restored by Fe deprivation; Matthus et al., 2019a). In plant signalling systems, ROS are held to amplify or propagate [Ca2+]cyt increase by modulating Ca2+ transporters (Demidchik and Shabala, 2018). In contrast, under Pi deprivation ROS ostensibly limits the [Ca2+]cyt response to eATP. Whether the impaired [Ca2+]cyt signal is the result of different complements of Ca2+ transporters (Shukla et al., 2021) as a consequence of Pi deprivation (possibly affecting the links with other pathways) and/or different regulatory mechanisms now needs to be determined. For the latter, it may be relevant that lowered cytosolic ATP (see section below) affects actin dynamics (Dai et al., 2022; Wang et al., 2022b) that could regulate plasma membrane Ca2+ channels (Qian and Xiang, 2019). It may also be relevant that lower cytosolic ATP could impair the activity of plasma membrane H+-ATPases, possibly impairing activity of voltage-dependent plasma membrane Ca2+ channels. This could explain why the Arabidopsis mutant lacking a major H+-ATPase isoform (AHA2) has a lower [Ca2+]cyt response to eATP (Haruta and Sussman, 2012).
eATP signalling under Pi deprivation – malnourished defence
Cellular ATP level drops sharply in response to Pi deprivation as shown in kidney bean roots, Catharanthus roseus and sycamore cell culture (Gniazdowska et al., 1998; Shimano and Ashihara, 2006; Gout et al., 2014). Although gradients of cytosolic Mg-ATP can be resolved at cellular level with imaging of the ATeam 1.03-nD/nA reporter (De Col et al., 2017), the effect of Pi deprivation remains untested. The drop in cellular ATP begs the questions of whether Pi-deprived tissues continue to maintain their basal eATP levels (with the possibility of too low a level triggering cell death; Chivasa et al., 2005) and whether wound/pathogen-induced eATP increases would be significantly lower. For Pi-replete Arabidopsis, wound-induced eATP estimates range from 35 nM to 45 µM (Song et al., 2006; Dark et al., 2011; Myers Jr et al., 2022). There appear to be no reports on the effect of Pi-deprivation in the literature although Tawaraya et al. (2014) reported that ADP was no longer present in the root exudates of Pi-deprived soybean roots.
Conservation of cytosolic ATP could involve restricting non-wounding ATP efflux pathways at the plasma membrane that are thought to include ABC transporters and anion channels (Thomas et al., 2000; Rieder and Neuhaus, 2011; Wu et al., 2011; Witte and Herde, 2020: Figure 2). Restricted growth caused by Pi deprivation could also limit cytosolic ATP release by exocytosis (Kim et al., 2006). However, as growing root hairs accumulate eATP at their apices (Kim et al., 2006), these levels might still be retained as root hair elongation increases as a potential mechanism to access soil Pi in the absence of mutualistic microbial partners. Indeed, lowering eATP can inhibit root hair elongation (Clark et al., 2010). Moreover, eATP may have a role to play in legume root hair deformation (curling) in response to nodulation factors. Curling is a re-orientation of elongative polar growth towards the nodulation factor (Esseling et al., 2003) and under Pi deprivation, significantly fewer Phaseolus vulgaris root hairs can curl, compromising the extent of the rhizobial symbiosis (Isidra-Arellano et al., 2018). The implication is that lowered Pi lowers root hair eATP and re-orientation is compromised. One effect of lowered eATP could be impaired ROS production at the root hair apex, which helps drive polar growth (Foreman et al., 2003; Cárdenas et al., 2008).
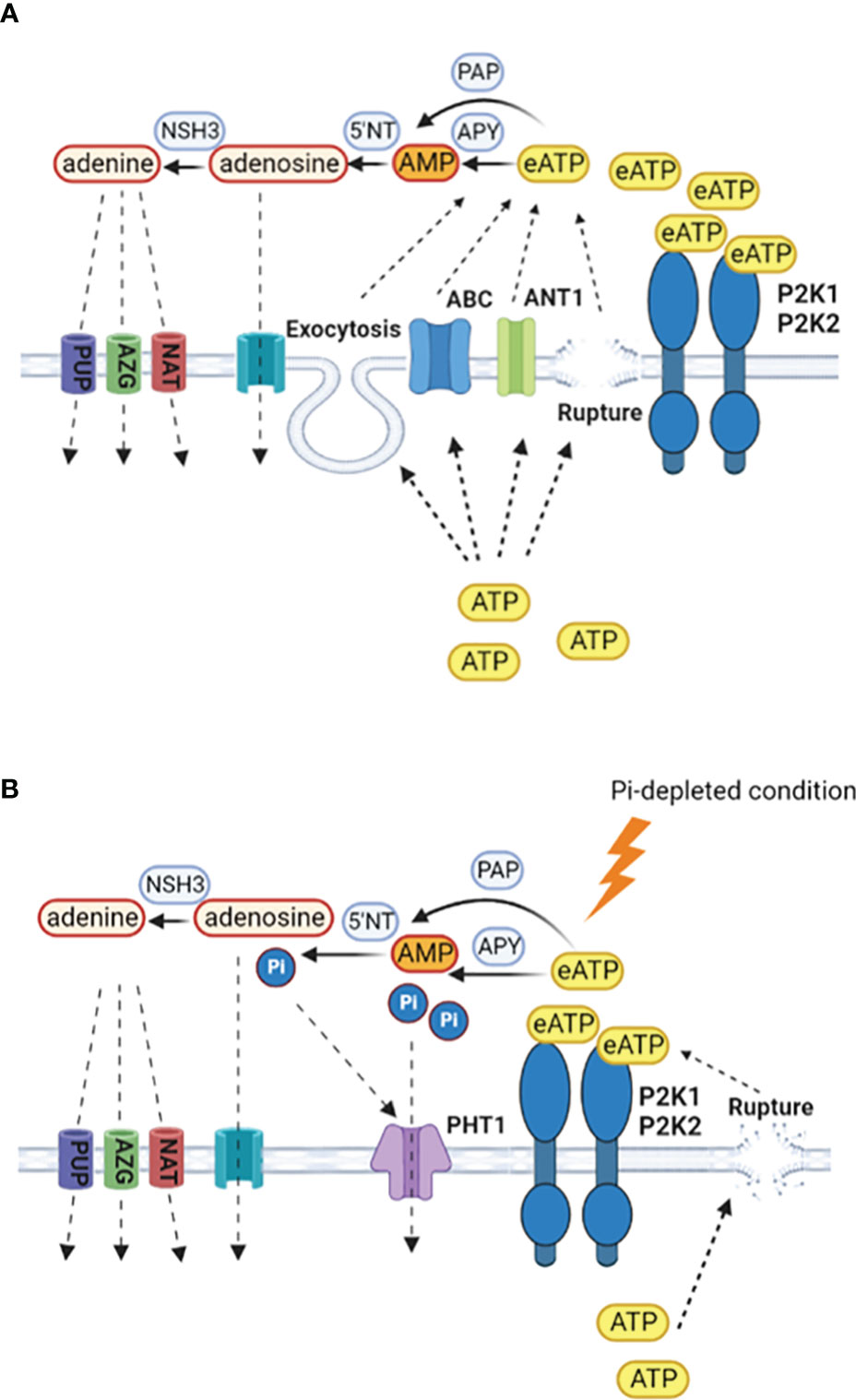
Figure 2 Production and scavenging of eATP. (A) In Pi-replete conditions, cytosolic ATP may be released to the extracellular space by wounding, exocytosis or specific transporters such as ANT1 (Thomas et al., 2000; Kim et al., 2006; Rieder and Neuhaus, 2011; Wu et al., 2011). Hydrolysis of eATP to terminate signalling may be by apyrases (APY), purple acid phosphatases (PAP), with subsequent breakdown by 5´nucleotidases and nucleoside hydrolases (Liang et al., 2010; Tran et al., 2010; Tian et al., 2012; Wang et al., 2014; Mehra et al., 2017; Kavka et al., 2021; Zhu S. et al., 2020; Clark et al., 2021). Retrieval of adenosine would be by equilibrative nucleoside transporters and for adenine by purine permeases (PUP), azaguanine resistant proteins (AZG) and nucleobase-ascorbate transporter family members (NAT) (Gillissen et al., 2000; Bernard et al., 2011; Witte and Herde, 2020). (B) Under Pi deprivation, there may be less cytosolic ATP to export and export systems could be limited, with wounding being the predominant route. P2K1 still appears competent but the involvement of P2K2 may be questioned (Matthus et al., 2022). Enzymes involved in eATP breakdown would become part of a Pi salvage system, with Pi uptake by PHT1 high affinity transporters that are induced by the PSR. Given the negative role of PHT1.4 in immunity (Dindas et al., 2022), the identities of the PHT1s may be an important control point in determining the resultant balance between nutrition and immunity.
Scavenging of eATP so that it can be utilised as a nutritional Pi source is evident in a wide range of plants including beech and poplar trees (Scheerer et al., 2019). Some extracellular Purple Acid Phosphatase (PAP) isoforms can scavenge eATP as a Pi source in Arabidopsis, Phaseolus vulgaris, poplar, rice and soybean, with production of some isoforms increasing upon Pi deprivation (Liang et al., 2010; Tran et al., 2010; Tian et al., 2012; Wang et al., 2014; Mehra et al., 2017; Kavka et al., 2021; Zhu S. et al., 2020; Figure 2). Apyrases hydrolyse ATP and are found in Golgi/ER, plasma membrane and apoplast (Summers et al., 2017; Clark et al., 2021). Apyrases could also scavenge eATP as a Pi source or limit its export, indeed there is an inverse relationship between their expression and eATP concentration around roots (Thomas et al., 1999; Lim et al., 2014; Deng et al., 2015). Studies suggest that regulation of ecto-apyrase may be critical to infection by Rhizobia and mycorrhizal fungi, such that their lowering of eATP promotes infection (Kalsi and Etzler, 2000; Govindarajulu et al., 2009; Roberts et al., 2013). It is held that the AMP produced by ecto-apyrase could be converted to adenosine then adenine by 5´nucleotidases and nucleoside hydrolases with uptake of those end products possibly by equilibrative nucleoside transporters (ENT) for adenosine and for adenine by the purine permeases, azaguanine resistant proteins and nucleobase-ascorbate transporter family members (Gillissen et al., 2000; Bernard et al., 2011; Witte and Herde, 2020; Figure 2). Efficient salvage of adenosine appears critical given that its accumulation compromises the resistance to Botrytis cinerea that is afforded by P2K1 (Daumann et al., 2015; Tripathi et al., 2018). Salvage of adenine should promote cytosolic ATP content (Dai et al., 2022).
As a non-mycorrhizal host, Arabidopsis roots allow colonization by fungal endophytes such as Colletotrichum tofieldiae and Serendipita indica to enhance Pi nutrition (Hiruma et al., 2016; Nizam et al., 2019; Frerigmann et al., 2021). Colonization by C. tofieldiae is controlled by the PSR response and the host’s production of tryptophan-derived indole glucosinolates (IG) as defence compounds keeps the extent of colonization in check (Hiruma et al., 2016; Frerigmann et al., 2021). Inability to synthesize IG (through loss of cyp79b2 cyp79b3 function) enables C. tofieldiae to behave as a pathogen (Hiruma et al., 2016). Pi-starvation can lower levels of IG in roots (but not shoots) consistent simplistically with the model of a lowering of plant defences (Frerigmann et al., 2021). Colonization increases levels of 4-methoxy-indole-3-methyl-glucosinolate which would require the activity of the P450 monooxygenases CYP83B1, CYP81F2, CYP81F3 and the Indole Glucosinolate O-Methyltransferase IGMT2 (Frerigmann et al., 2021). Recently eATP has been found to act through P2K1 (albeit in Pi-replete seedlings) to upregulate expression of the genes encoding those key enzymes (Jewell et al., 2022). This leads to the speculation that damage incurred by colonization could signal to effect the IG response, with the further possibility that ATP secreted by the fungus in this and other invasive scenarios could contribute to signalling. A first step would be to see if eATP modulates IG synthesis under Pi deprivation. The early phase of S. indica infection (albeit in Pi-replete roots) increases eATP and P2K1 helps limit colonization (Nizam et al., 2019). Over time the fungus secretes an eATP hydrolysing ecto-5´-nucleotidase (E5´NT) that can reduce eATP levels and promotes colonization, indicating that eATP signalling may ultimately need to be impaired (Nizam et al., 2019). Indeed, expressing the S. indica nucleotidase in Arabidopsis roots rendered them more susceptible to colonization by the pathogenic fungus C. incanum (Nizam et al., 2019). Modelling of Pi and sugar fluxes between Arabidopsis and S. indica suggests that if host ATP release were low, the fungal E5´NT could contribute to host Pi nutrition with no Pi cost to the fungus, only sucrose benefit. With high ATP release, E5´NT could contribute to Pi uptake of both host and fungus (Nizam et al., 2019). It seems in the fungus’ survival benefit to hydrolyze the eATP signal but as P2K1 is a high affinity receptor (dissociation constant 46 nM; Choi et al., 2014), eATP levels would have to be negligible to avoid triggering the pathway and there is evidence that P2K1 could still operate in [Ca2+]cyt signalling of Pi-starved roots (Matthus et al., 2022). Perhaps it is the loss of the P2K1-independent [Ca2+]cyt signal in Pi-starved roots that is critical to dampening the eATP defence pathway. The fungal endophyte could also modulate the eATP pathway and it is notable that although P2K1 acts to limit S. indica colonization (Nizam et al., 2019) this fungus does not cause the peroxide accumulation typical of eATP signalling (Camehl et al., 2011). Moreover, its cell wall extracts suppress expression of CNGC2 (Vadassery et al., 2009), a key component of eATP signalling (Wang et al., 2022a). Eat the signal and perturb the pathway.
Conclusions and prospects
Even under Pi-replete conditions plants must regulate eATP to render it an effective signal and avoid cell death. Under Pi deprivation, the balancing act may have to include the diminution of the endogenous cytosolic ATP supply and the salvage of eATP to bolster Pi nutrition. That eATP can still trigger a modified P2K1-dependent [Ca2+]cyt response in Pi- starved roots argues for a robust signalling system that is modulated to allow perhaps for beneficial colonisation. If “net” eATP were lower under Pi deprivation, even after wounding, then much depends on the receptors involved and their affinities, the eATP that could be produced by microbes and the ability of the microbes to degrade host or their own eATP. Whilst Arabidopsis remains the most well defined and tractable system, there is a clear need to resolve eATP signalling systems in crops and the impact of Pi deprivation.
Data availability statement
The original contributions presented in the study are included in the article. Further inquiries can be directed to the corresponding author.
Author contributions
All authors listed have made a substantial, direct, and intellectual contribution to the work and approved it for publication.
Funding
Funding was from the UKRI BBSRC (BB/J014540/1), the Henry Lester Trust, the Higher Education Commission of Pakistan and the University of Cambridge Trusts.
Acknowledgments
We thank the Administrative Staff of the Department of Plant Sciences and apologise for any omissions.
Conflict of interest
The authors declare that the research was conducted in the absence of any commercial or financial relationships that could be construed as a potential conflict of interest.
Publisher’s note
All claims expressed in this article are solely those of the authors and do not necessarily represent those of their affiliated organizations, or those of the publisher, the editors and the reviewers. Any product that may be evaluated in this article, or claim that may be made by its manufacturer, is not guaranteed or endorsed by the publisher.
References
Alewell, C., Ringeval, B., Ballabio, C., Robinson, D. A., Panagos, P., Borrelli, P. (2020). Global phosphorus shortage will be aggravated by soil erosion. Nat. Commun. 11. doi: 10.1038/s41467-020-18326-7
Andersson, M. X., Larsson, K. E., Tjellström, H., Liljenberg, C., Sandelius, ,. A. S. (2005). Phosphate-limited oat: The plasma membrane and the tonoplast as major targets for phospholipid-to-glycolipid replacement and stimulation of phospholipases in the plasma membrane. J. Biol. Chem. 280, 27578–27586. doi: 10.1074/jbc.M503273200
Balagué, C., Gouget, A., Bouchez, O., Souriac, C., Haget, N., Boutet-Mercey, S., et al. (2017). The Arabidopsis thaliana lectin receptor kinase LecRK-I . 9 is required for full resistance to Pseudomonas syringae and affects jasmonate signalling. Mol. Plant Pathol. 18, 937–948. doi: 10.1111/mpp.12457
Bernard, C., Traub, M., Kunz, H. H., Hach, S., Trentmann, O., Möhlmann, T. (2011). Equilibrative nucleoside transporter 1 (ENT1) is critical for pollen germination and vegetative growth in Arabidopsis. J. Exp. Bot. 62, 4627–4637. doi: 10.1093/jxb/err183
Bouwmeester, K., de Sain, M., Weide, R., Gouget, A., Klamer, S., Canut, H., et al. (2011). The lectin receptor kinase LecRK-I.9 is a novel Phytophthora resistance component and a potential host target for a RXLR effector. PloS Pathog. 7. doi: 10.1371/journal.ppat.1001327
Bouwmeester, K., Han, M., Blanco-Portales, R., Song, W., Weide, R., Guo, L.-Y., et al. (2014). The Arabidopsis lectin receptor kinase LecRK-I 9 enhances resistance to Phytophthora infestans in solanaceous plants. Plant Biotechnol. J. 12, 10–16. doi: 10.1111/pbi.12111
Camehl, I., Drzewiecki, C., Vadassery, J., Shahollari, B., Sherameti, I., Forzani, C., et al. (2011). The OXI1 kinase pathway mediates Piriformospora indica-induced growth promotion in Arabidopsis. PloS Pathog. 7. doi: 10.1371/journal.ppat.1002051
Cárdenas, L., Martínez, A., Sánchez, F., Quinto, C. (2008). Fast, transient and specific intracellular ROS changes in living root hair cells responding to nod factors (NFs). Plant J. 56, 802–813. doi: 10.1111/j.1365-313X.2008.03644.x
Castrillo, G., Teixeira, P. J. P. L., Paredes, S. H., Law, T. F., de Lorenzo, L., Feltcher, M. E., et al. (2017). Root microbiota drive direct integration of phosphate stress and immunity. Nature 543, 513–518. doi: 10.1038/nature21417
Chakraborty, S., Toyota, M., Moeder, W., Chin, K., Fortuna, A., Champigny, M., et al. (2021). CYCLIC NUCLEOTIDE-GATED ION CHANNEL 2 modulates auxin homeostasis and signaling. Plant Physiol. 187, 1690–1703. doi: 10.1093/plphys/kiab332
Chen, D., Cao, Y., Li, H., Kim, D., Ahsan, N., Thelen, J., et al. (2017). Extracellular ATP elicits DORN1-mediated RBOHD phosphorylation to regulate stomatal aperture. Nat. Commun. 8. doi: 10.1038/s41467-017-02340-3
Chin, K., DeFalco, T. A., Moeder, W., Yoshioka, K. (2013). The Arabidopsis cyclic nucleotide-gated ion channels AtCNGC2 and AtCNGC4 work in the same signaling pathway to regulate pathogen defense and floral transition. Plant Physiol. 163, 611–624. doi: 10.1104/pp.113.225680
Chivasa, S., Ndimba, B. K., Simon, W. J., Lindsey, K., Slabas, A. R. (2005). Extracellular ATP functions as an endogenous external metabolite regulating plant cell viability. Plant Cell 17, 3019–3034. doi: 10.1105/tpc.105.036806.osmotic
Choi, J., Tanaka, K., Cao, Y., Qi, Y., Qiu, J., Liang, Y., et al. (2014). Identification of a plant receptor for extracellular ATP. Science 343, 290–294. doi: 10.1126/science.343.6168.290
Clark, G., Brown, K. A., Tripathy, M. K., Roux, S. J. (2021). Recent advances clarifying the structure and function of plant apyrases (Nucleoside triphosphate diphosphohydrolases). Int. J. Mol. Sci. 22. doi: 10.3390/ijms22063283
Clark, G., Wu, M., Wat, N., Onyirimba, J., Pham, T., Herz, N. (2010). Both the stimulation and inhibition of root hair growth induced by extracellular nucleotides in Arabidopsis are mediated by nitric oxide and reactive oxygen species. Plant Mol. Biol. 74, 423–435. doi: 10.1007/s11103-010-9683-7
Costa, A., Luoni, L., Marrano, C. A., Hashimoto, K., Köster, P., Giacometti, S., et al. (2017). Ca2+-dependent phosphoregulation of the plasma membrane Ca2+-ATPase ACA8 modulates stimulus-induced calcium signatures. J. Exp. Bot. 68, 3215–3230. doi: 10.1093/jxb/erx162
Dai, L., Wang, B., Wang, T., Meyer, E. H., Kettel, V., Hoffmann, N., et al. (2022). The TOR complex controls ATP levels to regulate actin cytoskeleton dynamics in Arabidopsis. Proc. Natl. Acad. Sci. U. S. A. 119. doi: 10.1073/pnas.2122969119
Dark, A., Demidchik, V., Shabala, S., Davies, J. M. (2011). Release of extracellular purines from plant roots and effect on ion fluxes. Plant Signal. Behav. 6, 1855–1857. doi: 10.1093/jxb/ern242.5
Daumann, M., Fischer, M., Niopek-Witz, S., Girke, C., Möhlmann, T. (2015). Apoplastic nucleoside accumulation in Arabidopsis leads to reduced photosynthetic performance and increased susceptibility against Botrytis cinerea. Front. Plant Sci. 6. doi: 10.3389/fpls.2015.01158
De Col, V., Fuchs, P., Nietzel, T., Elsässer, M., Voon, C. P., Candeo, A., et al. (2017). ATP sensing in living plant cells reveals tissue gradients and stress dynamics of energy physiology. Elife. doi: 10.7554/eLife.26770
Demidchik, V., Shabala, S. (2018). Mechanisms of cytosolic calcium elevation in plants: The role of ion channels, calcium extrusion systems and NADPH oxidase-mediated “ROS-Ca2+ hub.” funct. Plant Biol. 45. doi: 10.1071/FP16420
Demidchik, V., Shang, Z., Shin, R., Thompson, E., Rubio, L., Laohavisit, A., et al. (2009). Plant extracellular ATP signalling by plasma membrane NADPH oxidase and Ca2+ channels. Plant J. 58 (6), 903–913. doi: 10.1111/j.1365-313X.2009.03830.x
Deng, S., Sun, J., Zhao, R., Ding, M., Zhang, Y., Sun, Y., et al. (2015). Populus euphratica APYRASE2 enhances cold tolerance by modulating vesicular trafficking and extracellular ATP in Arabidopsis plants. Plant Physiol. 169, 530–548. doi: 10.1104/pp.15.00581
Dindas, J., Defalco, T. A., Yu, G., Nussaume, L., Macho, A. P., Zipfel, C., et al. (2022). Direct inhibition of phosphate transport by immune signaling in Arabidopsis. Curr. Biol. 32, 488–495. doi: 10.1016/j.cub.2021.11.063
Duff, S. M., Moorhead, G. B., Lefebvre, D. D., Plaxton, W. C. (1989). Phosphate starvation inducible “Bypasses” of adenylate and phosphate dependent glycolytic enzymes in Brassica nigra suspension cells. Plant Physiol. 90, 1275–1278. doi: 10.1104/pp.90.4.1275
Duong, H. N., Cho, S.-H., Wang, L., Pham, A. Q., Davies, J. M., Stacey, G. (2022). Cyclic nucleotide gated ion channel 6 is involved in extracellular ATP signaling and plant immunity. Plant J. 109, 1386–1396. doi: 10.1111/tpj.15636
Esseling, J. J., Lhuissier, F. G. P., Emons, A. M. C. (2003). Nod factor-induced root hair curling: Continuous polar growth towards the point of nod factor application. Plant Physiol. 132, 1982–1988. doi: 10.1104/pp.103.021634
Fichman, Y., Myers, R. J., Grant, D. G., Mittler, R. (2021). Plasmodesmata-localized proteins and ROS orchestrate light-induced rapid systemic signaling in Arabidopsis. Sci. Signal 14. doi: 10.1126/scisignal.abf0322
Finka, A., Cuendet, A. F. H., Maathuis, F. J. M., Saidi, Y., Goloubinoff, P. (2012). Plasma membrane cyclic nucleotide gated calcium channels control land plant thermal sensing and acquired thermotolerance. Plant Cell 24, 3333–3348. doi: 10.1105/tpc.112.095844
Foreman, J., Demidchik, V., Bothwell, J. H. F., Mylona, P., Miedema, H., Torres, M. A., et al. (2003). Reactive oxygen species produced by NADPH oxidase regulate plant cell growth. Nature 422, 442–446. doi: 10.1038/nature01485
Frerigmann, H., Piotrowski, M., Lemke, R., Bednarek, P., Schulze-Lefert, P. (2021). A network of phosphate starvation and immune-related signaling and metabolic pathways controls the interaction between Arabidopsis thaliana and the beneficial fungus Colletotrichum tofieldiae. Mol. Plant-Microbe Interact. 34, 560–570. doi: 10.1094/MPMI-08-20-0233-R
Gastélum-Estrada, A., Hurtado-Romero, A., Santacruz, A., Cisneros-Zevallos, L., Jacobo-Velázquez, D. A. (2020). Sanitizing after fresh-cutting carrots reduces the wound-induced accumulation of phenolic antioxidants compared to sanitizing before fresh-cutting. J. Sci. Food Agric. 100, 4995–4998. doi: 10.1002/jsfa.10555
Gillissen, B., Bürkle, L., André, B., Kühn, C., Rentsch, D., Brandl, B., et al. (2000). A new family of high-affinity transporters for adenine, cytosine, and purine derivatives in Arabidopsis. Plant Cell 12, 291–300. doi: 10.1105/tpc.12.2.291
Gniazdowska, A., Mikulska, M., Rychter, A. M. (1998). Growth, nitrate uptake and respiration rate in bean roots under phosphate deficiency. Biol. Plant 41, 217–226. doi: 10.1023/A:1001862513105
Gouget, A., Senchou, V., Govers, F., Sanson, A., Barre, A., Rougé, P., et al. (2006). Lectin receptor kinases participate in protein-protein interactions to mediate plasma membrane-cell wall adhesions in Arabidopsis. Plant Physiol. 140, 81–90. doi: 10.1104/pp.105.066464
Gout, E., Rebeille, F., Douce, R., Bligny, R. (2014). Interplay of Mg2+, ADP, and ATP in the cytosol and mitochondria: Unravelling the role of Mg2+ in cell respiration. Proc. Natl. Acad. Sci. 111, E4560–E4567. doi: 10.1073/pnas.1406251111
Govindarajulu, M., Kim, S.-Y., Libault, M., Berg, R. H., Tanaka, K., Stacey, G., et al. (2009). GS52 ecto-apyrase plays a critical role during soybean nodulation. Plant Physiol. 149, 994–1004. doi: 10.1104/pp.108.128728
Haruta, M., Sussman, M. R. (2012). The effect of a genetically reduced plasma membrane protonmotive force on vegetative growth of Arabidopsis. Plant Physiol. 158, 1158–1171. doi: 10.1104/pp.111.189167
Hiruma, K., Gerlach, N., Sacristán, S., Nakano, R. T., Hacquard, S., Kracher, B., et al. (2016). Root endophyte Colletotrichum tofieldiae confers plant fitness benefits that are phosphate status dependent. Cell 165, 464–474. doi: 10.1016/j.cell.2016.02.028
Isidra-Arellano, M. C., Delaux, P. M., Valdés-López, O. (2021). The phosphate starvation response system: Its role in the regulation of plant–microbe interactions. Plant Cell Physiol. 62, 392–400. doi: 10.1093/pcp/pcab016
Isidra-Arellano, M. C., del Rocio Reyero-Saavedra, M., del Socorro Sánchez-Correa, M., Pingault, M. L., Sen, S., Joshi, T., et al. (2018). Phosphate deficiency negatively affects early steps of the symbiosis between common bean and rhizobia. Genes 9, 498. doi: 10.3390/genes9100498
Jarratt-Barnham, E., Wang, L., Ning, Y., Davies, J. M. (2021). The complex story of plant cyclic nucleotide-gated channels. Int. J. Mol. Sci. 22. doi: 10.3390/ijms22020874
Jeter, C. R., Tang, W., Henaff, E., Butterfield, T., Roux, S. J. (2004). Evidence of a novel cell signaling role for extracellular adenosine triphosphates and diphosphates in Arabidopsis. Plant Cell 16, 2652–2664. doi: 10.1105/tpc.104.023945.include
Jewell, J. B., Berim, A., Tripathi, D., Gleason, C., Olaya, C., Pappu, H. R., et al. (2022). Activation of indolic glucosinolate pathway by extracellular ATP in Arabidopsis. Plant Physiol. 27, 1574–1578. doi: 10.1093/plphys/kiac393
Jewell, J. B., Sowders, J. M., He, R., Willis, M. A., Gang, D. R., Tanaka, K. (2019). Extracellular ATP shapes a defense-related transcriptome both independently and along with other defense signaling pathways. Plant Physiol. 179, 1144–1158. doi: 10.1104/pp.18.01301
Jiang, X., Hoehenwarter, W., Scheel, D., Lee, J. (2020). Phosphorylation of the CAMTA3 transcription factor triggers its destabilization and nuclear export. Plant Physiol. 184, 1056–1071. doi: 10.1104/pp.20.00795
Kalsi, G., Etzler, M. E. (2000). Localization of a nod factor-binding protein in legume roots and factors influencing its distribution and expression. Plant Physiol. 124, 1039–1048. doi: 10.1104/pp.124.3.1039
Katano, K., Kataoka, R., Fujii, M., Suzuki, N. (2018). Differences between seedlings and flowers in anti-ROS based heat responses of Arabidopsis plants deficient in cyclic nucleotide gated channel 2. Plant Physiol. Biochem. 123, 288–296. doi: 10.1016/j.plaphy.2017.12.021
Kavka, M., Majcherczyk, A., Kües, U., Polle, A. (2021). Phylogeny, tissue-specific expression, and activities of root-secreted purple acid phosphatases for p uptake from ATP in p starved poplar. Plant Sci. 307. doi: 10.1016/j.plantsci.2021.110906
Kim, S.-Y., Sivaguru, M., Stacey, G. (2006). Extracellular ATP in plants. visualization, localization, and analysis of physiological significance in growth and signaling. Plant Physiol. 142, 984–992. doi: 10.1104/pp.106.085670
Krebs, M., Held, K., Binder, A., Hashimoto, K., Den Herder, G., Parniske, M., et al. (2012). FRET-based genetically encoded sensors allow high-resolution live cell imaging of Ca2+ dynamics. Plant J. 69, 181–192. doi: 10.1111/j.1365-313X.2011.04780.x
Kumar, S., Tripathi, D., Okubara, P. A., Tanaka, K. (2020). Purinoceptor P2K1/DORN1 enhances plant resistance against a soilborne fungal pathogen, Rhizoctonia solani. Front. Plant Sci. 11. doi: 10.3389/fpls.2020.572920
Lan, P., Li, W., Schmidt, W. (2012). Complementary proteome and transcriptome profiling in phosphate-deficient Arabidopsis roots reveals multiple levels of gene regulation. Mol. Cell. Proteomics 11, 1156–1166. doi: 10.1074/mcp.M112.020461
Liang, C., Tian, J., Lam, H. M., Lim, B. L., Yan, X., Liao, H. (2010). Biochemical and molecular characterization of PvPAP3, a novel purple acid phosphatase isolated from common bean enhancing extracellular ATP utilization. Plant Physiol. 152, 854–865. doi: 10.1104/pp.109.147918
Li, Z., Chakraborty, S., Xu, G. (2016). X-Ray crystallographic studies of the extracellular domain of the first plant ATP receptor, DORN1, and the orthologous protein from Camelina sativa. Struct. Biol. Commun. 72, 782–787. doi: 10.1107/S2053230X16014278
Lim, M. H., Wu, J., Yao, J., Gallardo, I. F., Dugger, J. W., Webb, L. J., et al. (2014). Apyrase suppression raises extracellular ATP levels and induces gene expression and cell wall changes characteristic of stress responses. Plant Physiol. 164, 2054–2067. doi: 10.1104/pp.113.233429
Lin, W.-D., Liao, Y.-Y., Yang, T. J. W., Pan, C.-Y., Buckhout, T. J., Schmidt, W. (2011). Coexpression-based clustering of Arabidopsis root genes predicts functional modules in early phosphate deficiency signaling. Plant Physiol. 155, 1383–1402. doi: 10.1104/pp.110.166520
Loro, G., Drago, I., Pozzan, T., Schiavo, F., Zottini, M., Costa, A. (2012). Targeting of cameleons to various subcellular compartments reveals a strict cytoplasmic/mitochondrial Ca2+ handling relationship in plant cells. Plant J. 71. doi: 10.1111/j.1365-313X.2012.04968.x
Lu, M., Zhang, Y., Tang, S., Pan, J., Yu, Y., Han, J., et al. (2016). AtCNGC2 is involved in jasmonic acid-induced calcium mobilization. J. Exp. Bot. 67, 809–819. doi: 10.1093/jxb/erv500
Matthus, E., Sun, J., Wang, L., Bhat, M. G., Mohammad-Sidik, A. B., Wilkins, K. A., et al. (2019b). DORN1/P2K1 and purino-calcium signalling in plants: Making waves with extracellular ATP. Ann. Bot. 124, 1227–1242. doi: 10.1093/aob/mcz135
Matthus, E., Wilkins, K. A., Mohammad-Sidik, A., Ning, Y., Davies, J. M. (2022). Spatial origin of the extracellular ATP-induced cytosolic calcium signature in Arabidopsis thaliana roots: wave formation and variation with phosphate nutrition. Plant Biol. 24, 863–873. doi: 10.1111/plb.13427
Matthus, E., Wilkins, K. A., Swarbreck, S. M., Doddrell, N. H., Doccula, F. G., Costa, A., et al. (2019a). Phosphate starvation alters abiotic-stress-induced cytosolic free calcium increases in roots. Plant Physiol. 179, 1754–1767. doi: 10.1104/pp.18.01469
Medina-Castellanos, E., Esquivel-Naranjo, E. U., Heil, M., Herrera-Estrella, A. (2014). Extracellular ATP activates MAPK and ROS signaling during injury response in the fungus Trichoderma atroviride. Front. Plant Sci. 5. doi: 10.3389/fpls.2014.00659
Medina-Castellanos, E., Villalobos-Escobedo, J. M., Riquelme, M., Read, N. D., Abreu-Goodger, C., Herrera-Estrella, A. (2018). Danger signals activate a putative innate immune system during regeneration in a filamentous fungus. PloS Genet. 14. doi: 10.1371/journal.pgen.1007390
Mehra, P., Pandey, B. K., Giri, J. (2017). Improvement in phosphate acquisition and utilization by a secretory purple acid phosphatase (OsPAP21b) in rice. Plant Biotechnol. J. 15, 1054–1067. doi: 10.1111/pbi.12699
Mohammad-Sidik, A., Sun, J., Shin, R., Song, Z., Ning, Y., Matthus, E., et al. (2021). Annexin 1 is a component of eATP-induced cytosolic calcium elevation in Arabidopsis thaliana roots. Int. J. Mol. Sci. 22. doi: 10.3390/ijms22020494
Myers, R. J., Fichman, Y., Stacey, G., Mittler, R. (2022). Extracellular ATP plays an important role in systemic wound response activation. Plant Physiol. 189, 1314–1325. doi: 10.1093/plphys/kiac148
Nakamura, Y. (2013). Phosphate starvation and membrane lipid remodeling in seed plants. Prog. Lipid Res. 52, 43–50. doi: 10.1016/j.plipres.2012.07.002
Nizam, S., Qiang, X., Wawra, S., Nostadt, R., Getzke, F., Schwanke, F., et al. (2019). Serendipita indica E5′NT modulates extracellular nucleotide levels in the plant apoplast and affects fungal colonization. EMBO Rep. 20. doi: 10.15252/embr.201847430
Okazaki, Y., Otsuki, H., Narisawa, T., Kobayashi, M., Sawai, S., Kamide, Y., et al. (2013). A new class of plant lipid is essential for protection against phosphorus depletion. Nat. Commun. 4. doi: 10.1038/ncomms2512
Pant, B.-D., Pant, P., Erban, A., Huhman, D., Kopka, J., Scheible, W.-R. (2015). Identification of primary and secondary metabolites with phosphorus status-dependent abundance in Arabidopsis, and of the transcription factor PHR1 as a major regulator of metabolic changes during phosphorus-limitation. Plant Cell Environ. 38, 172–187. doi: 10.1111/pce.12378
Pham, A. Q., Cho, S. H., Nguyen, C. T., Stacey, G. (2020). Arabidopsis lectin receptor kinase P2K2 is a second plant receptor for extracellular ATP and contributes to innate immunity. Plant Physiol. 183, 1364–1375. doi: 10.1104/pp.19.01265
Plaxton, W. C., Tran, H. T. (2011). Metabolic adaptations of phosphate-starved plants. Plant Physiol. 156, 1006–1015. doi: 10.1104/pp.111.175281
Pratt, J., Boisson, A.-M., Gout, E., Bligny, R., Douce, R., Aubert, S. (2009). Phosphate (Pi) starvation effect on the cytosolic pi concentration and pi exchanges across the tonoplast in plant cells: an in vivo 31P-nuclear magnetic resonance study using methylphosphonate as a pi analog. Plant Physiol. 151, 1646–1657. doi: 10.1104/pp.109.144626
Qian, D., Xiang, Y. (2019). Actin cytoskeleton as actor in upstream and downstream of calcium signaling in plant cells. Int. J. Mol. Sci. 20. doi: 10.3390/ijms20061403
Rieder, B., Neuhaus, H. E. (2011). Identification of an Arabidopsis plasma membrane–located ATP transporter important for anther development. Plant Cell 23, 1932–1944. doi: 10.1105/tpc.111.084574
Roberts, N. J., Morieri, G., Kalsi, G., Rose, A., Stiller, J., Edwards, A., et al. (2013). Rhizobial and mycorrhizal symbioses in Lotus japonicus require lectin nucleotide phosphohydrolase, which acts upstream of calcium signaling. Plant Physiol. 161, 556–567. doi: 10.1104/pp.112.206110
Scheerer, U., Trube, N., Netzer, F., Rennenberg, H., Herschbach, C. (2019). ATP as phosphorus and nitrogen source for nutrient uptake by Fagus sylvatica and Populus x canescens roots. Front. Plant Sci. 10. doi: 10.3389/fpls.2019.00378
Shan, Y., Huang, H., Lian, Q., Li, F., Zhang, J., Zhu, H., et al. (2020). Characterization and function of banana DORN1s during fruit ripening and cold storage. Postharvest Biol. Technol. 167. doi: 10.1016/j.postharvbio.2020.111236
Shimano, F., Ashihara, H. (2006). Effect of long-term phosphate starvation on the levels and metabolism of purine nucleotides in suspension-cultured Catharanthus roseus cells. Phytochemistry 67, 132–141. doi: 10.1016/j.phytochem.2005.10.013
Shi, Z., Wang, H., Zhang, Y., Jia, L., Pang, H., Feng, H., et al. (2022). The involvement of extracellular ATP in regulating the stunted growth of Arabidopsis plants by repeated wounding. BMC Plant Biol. 22. doi: 10.1186/s12870-022-03656-z
Shukla, D., Waigel, S., Rouchka, E. C., Sandhu, G., Trivedi, P. K., Sahi, S. V. (2021). Genome-wide expression analysis reveals contrasting regulation of phosphate starvation response (PSR) in root and shoot of Arabidopsis and its association with biotic stress. Environ. Exp. Bot. 188. doi: 10.1016/j.envexpbot.2021.104483
Shumayla, Sharma, S., Pandey, A. K., Kashmir Singh, K., Upadhyay, S. K. (2016). Molecular characterization and global expression analysis of lectin receptor kinases in bread wheat (Triticum aestivum). PloS One 11, e0153925. doi: 10.1371/journal.pone.0153925
Smith, S. J., Goodman, H., Kroon, J. T. M., Brown, A. P., Simon, W. J., Chivasa, S. (2021). Isolation of Arabidopsis extracellular ATP binding proteins by affinity proteomics and identification of PHOSPHOLIPASE c-LIKE 1 as an extracellular protein essential for fumonisin B1 toxicity. Plant J. 106, 1387–1400. doi: 10.1111/tpj.15243
Song, C. J., Steinebrunner, I., Wang, X., Stout, S. C., Roux, S. J. (2006). Extracellular ATP induces the accumulation of superoxide via NADPH oxidases in Arabidopsis. Plant Physiol. 140, 1222–1232. doi: 10.1104/pp.105.073072.et
Summers, E. L., Cumming, M. H., Oulavallickal, T., Roberts, N. J., Arcus, V. L. (2017). Structures and kinetics for plant nucleoside triphosphate diphosphohydrolases support a domain motion catalytic mechanism. Protein Sci. 26, 1627–1638. doi: 10.1002/pro.3199
Sun, J., Ning, Y., Wang, L., Wilkins, K. A., Davies, ,. J. M. (2021). Damage signaling by extracellular nucleotides: A role for cyclic nucleotides in elevating cytosolic free calcium? Front. Plant Sci. 12. doi: 10.3389/fpls.2021.788514
Tanaka, K., Heil, M. (2021). Damage-associated molecular patterns (DAMPs) in plant innate immunity: Applying the danger model and evolutionary perspectives. Annu. Rev. Phytopathol. 25, 53–75. doi: 10.1146/annurev-phyto-082718-100146
Tang, J., Wu, D., Li, X., Wang, L., Xu, L., Zhang, Y., et al. (2022). Plant immunity suppression via PHR1-RALF-FERONIA shapes the root microbiome to alleviate phosphate starvation. EMBO J. 41. doi: 10.15252/embj.2021109102
Tawaraya, K., Horie, R., Shinano, T., Wagatsuma, T., Saito, K., Oikawa, A. (2014). Metabolite profiling of soybean root exudates under phosphorus deficiency. Soil Sci. Plant Nutr. 60, 679–694. doi: 10.1080/00380768.2014.945390
Thomas, C., Rajagopal, A., Windsor, B., Dudler, R., Lloyd, A., Roux, S. J. (2000). A role for ectophosphatase in xenobiotic resistance. Plant Cell 12, 519–533. doi: 10.1105/tpc.12.4.519
Thomas, C., Sun, Y., Naus, K., Lloyd, A., Roux, S. (1999). Apyrase functions in plant phosphate nutrition and mobilizes phosphate from extracellular ATP. Plant Physiol. 119, 543–552. doi: 10.1104/pp.119.2.543
Tian, W., Hou, C., Ren, Z., Wang, C., Zhao, F., Dahlbeck, D., et al. (2019). A calmodulin-gated calcium channel links pathogen patterns to plant immunity. Nature 572, 131–135. doi: 10.1038/s41586-019-1413-y
Tian, J., Wang, C., Zhang, Q., He, X., Whelan, J., Shou, H. (2012). Overexpression of OsPAP10a, a root-associated acid phosphatase, increased extracellular organic phosphorus utilization in rice. J. Integr. Plant Biol. 54, 631–639. doi: 10.1111/j.1744-7909.2012.01143.x
Tjellström, H., Hellgren, L. I., Wieslander, A., Sandelius, A. S. (2010). Lipid asymmetry in plant plasma membranes: phosphate deficiency-induced phospholipid replacement is restricted to the cytosolic leaflet. FASEB J. 24, 1128–1138. doi: 10.1096/fj.09-139410
Tran, H. T., Qian, W., Hurley, B. A., She, Y. M., Wang, D., Plaxton, W. C. (2010). Biochemical and molecular characterization of AtPAP12 and AtPAP26: The predominant purple acid phosphatase isozymes secreted by phosphate-starved Arabidopsis thaliana. Plant Cell Environ. 33, 1789–1803. doi: 10.1111/j.1365-3040.2010.02184.x
Tripathi, D., Zhang, T., Koo, A. J., Stacey, G., Tanaka, K. (2018). Extracellular ATP acts on jasmonate signaling to reinforce plant defense. Plant Physiol. 176, 511–523. doi: 10.1104/pp.17.01477
Vadassery, J., Ranf, S., Drzewiecki, C., Mithöfer, A., Mazars, C., Scheel, D., et al. (2009). A cell wall extract from the endophytic fungus Piriformospora indica promotes growth of Arabidopsis seedlings and induces intracellular calcium elevation in roots. Plant J. 59, 193–206. doi: 10.1111/j.1365-313X.2009.03867.x
Vaid, N., Pandey, P. K., Tuteja, N. (2012). Genome-wide analysis of lectin receptor-like kinase family from Arabidopsis and rice. Plant Mol. Biol. 80, 365–388. doi: 10.1007/s11103-012-9952-8
Val-Torregrosa, B., Bundó, M., Martín-Cardoso, H., Bach-Pages, M., Chiou, T. J., Flors, V., et al. (2022). Phosphate-induced resistance to pathogen infection in Arabidopsis. Plant J. 110, 452–469. doi: 10.1111/tpj.15680
Verkhratsky, A. (2021). Early evolutionary history (from bacteria to hemichordata) of the omnipresent purinergic signalling: A tribute to Geoff burnstock inquisitive mind. Biochem. Pharmacol. doi: 10.1016/j.bcp.2020.114261
Wang, Q. W., Jia, L. Y., Shi, D. L., Wang, R. F., Lu, L. N., Xie, J. J., et al. (2019). Effects of extracellular ATP on local and systemic responses of bean (Phaseolus vulgaris L) leaves to wounding. Biosci. Biotechnol. Biochem. 83, 417–428. doi: 10.1080/09168451.2018.1547623
Wang, L., Lin, Z., Carli, J., Gladala-Kostarz, A., Davies, J. M., Franklin-Tong, V. E., et al. (2022b). ATP depletion plays a pivotal role in self-incompatibility, revealing a link between cellular energy status, cytosolic acidification and actin remodelling in pollen tubes. New Phytol. doi: 10.1111/nph.18350
Wang, L., Lu, S., Zhang, Y., Li, Z., Du, X., Liu, D. (2014). Comparative genetic analysis of Arabidopsis purple acid phosphatases AtPAP10, AtPAP12, and AtPAP26 provides new insights into their roles in plant adaptation to phosphate deprivation. J. Integr. Plant Biol. 56, 299–314. doi: 10.1111/jipb.12184
Wang, L., Ning, Y., Sun, J., Wilkins, K. A., Matthus, E., McNelly, R. E., et al. (2022a). Arabidopsis thaliana CYCLIC NUCLEOTIDE-GATED CHANNEL2 mediates extracellular ATP signal transduction in root epidermis. New Phytol. 234, 412–421. doi: 10.1111/nph.17987
Weerasinghe, R. R., Swanson, S. J., Okada, S. F., Garrett, M. B., Kim, S.-Y., Stacey, G., et al. (2009). Touch induces ATP release in Arabidopsis roots that is modulated by the heterotrimeric G-protein complex. FEBS Lett. 2521–2526. doi: 10.1016/j.febslet.2009.07.007
Wilkins, K., Matthus, E., Swarbreck, S., Davies, J. (2016). Calcium-mediated abiotic stress signaling in roots. Front. Plant Sci. 7(245). doi: 10.3389/fpls.2016.01296
Witte, C. P., Herde, M. (2020). Nucleotide metabolism in plants. Plant Physiol. 182, 63–78. doi: 10.1104/pp.19.00955
Wu, F., Chi, Y., Jiang, Z., Xu, Y., Xie, L., Huang, F., et al. (2020). Hydrogen peroxide sensor HPCA1 is an LRR receptor kinase in Arabidopsis. Nature 578, 577–581. doi: 10.1038/s41586-020-2032-3
Wu, S. J., Siu, K. C., Wu, ,. J. Y. (2011). Involvement of anion channels in mediating elicitor-induced ATP efflux in Salvia miltiorrhiza hairy roots. J. Plant Physiol. 168, 128–132. doi: 10.1016/j.jplph.2010.07.015
Wu, Y., Yin, H., Liu, X., Xu, J., Qin, B., Feng, K., et al. (2021). P2K1 receptor, heterotrimeric gα protein and CNGC2/4 are involved in extracellular ATP-promoted ion influx in the pollen of Arabidopsis thaliana. Plants 10. doi: 10.3390/plants10081743
Zhang, H., Zhang, F., Yu, Y., Li, F., Jia, J., Liu, B., et al. (2020). A comprehensive online database for exploring 20,000 punlic Arabidopsis RNA-seq libraries. Mol. Plant 13, 1231–1233. doi: 10.1016/i.molp.2020.08.001
Zhu, S., Chen, M., Liang, C., Xue, Y., Lin, S., Tian, J. (2020). Characterization of purple acid phosphatase family and functional analysis of GmPAP7a/7b involved in extracellular ATP utilization in soybean. Front. Plant Sci. 11. doi: 10.3389/fpls.2020.00661
Zhu, R., Dong, X., Hao, W., Gao, W., Zhang, W., Xia, S., et al. (2017). Heterotrimeric G protein-regulated Ca2+ influx and PIN2 asymmetric distribution are involved in Arabidopsis thaliana roots’ avoidance response to extracellular ATP. Front. Plant Sci. 8. doi: 10.3389/fpls.2017.01522
Keywords: phosphate deprivation, ATP and damage signalling, calcium, DAMP, immunity, phosphate, root
Citation: Matthus E, Ning Y, Shafiq F and Davies JM (2023) Phosphate-deprivation and damage signalling by extracellular ATP. Front. Plant Sci. 13:1098146. doi: 10.3389/fpls.2022.1098146
Received: 14 November 2022; Accepted: 19 December 2022;
Published: 12 January 2023.
Edited by:
Kiwamu Tanaka, Washington State University, United StatesReviewed by:
Oswaldo Valdes-Lopez, National Autonomous University of Mexico, MexicoYusuke Saijo, Nara Institute of Science and Technology (NAIST), Japan
Stan Roux, The University of Texas at Austin, United States
Copyright © 2023 Matthus, Ning, Shafiq and Davies. This is an open-access article distributed under the terms of the Creative Commons Attribution License (CC BY). The use, distribution or reproduction in other forums is permitted, provided the original author(s) and the copyright owner(s) are credited and that the original publication in this journal is cited, in accordance with accepted academic practice. No use, distribution or reproduction is permitted which does not comply with these terms.
*Correspondence: Julia M. Davies, am1kMzJAY2FtLmFjLnVr