- 1Crop Science Centre, Department of Plant Sciences, University of Cambridge, Cambridge, United Kingdom
- 2Department of Plant Biology, University of California, Davis, CA, United States
Parasitic plants are notorious for causing serious agricultural losses in many countries. Specialized intrusive organs, haustoria, confer on parasitic plants the ability to acquire water and nutrients from their host plants. Investigating the mechanism involved in haustorium development not only reveals the fascinating mystery of how autotrophic plants evolved parasitism but also provides the foundation for developing more effective methods to control the agricultural damage caused by parasitic plants. Cuscuta species, also known as dodders, are one of the most well-known and widely spread stem holoparasitic plants. Although progress has been made recently in understanding the evolution and development of haustoria in root parasitic plants, more and more studies indicate that the behaviors between root and stem haustorium formation are distinct, and the mechanisms involved in the formation of these organs remain largely unknown. Unlike most endoparasites and root holoparasitic plants, which have high host-specificity and self- or kin-recognition to avoid forming haustoria on themselves or closely related species, auto-parasitism and hyper-parasitism are commonly observed among Cuscuta species. In this review, we summarize the current understanding of haustorium development in dodders and the unique characteristics of their parasitizing behaviors. We also outline the advantages of using Cuscuta species as model organisms for haustorium development in stem holoparasitic plants, the current unknown mysteries and limitations in the Cuscuta system, and potential future research directions to overcome these challenges.
1. Introduction
Plants have often been defined as autotrophic eukaryotic photosynthetic organisms. Plants have chlorophyll, which enables them to convert inorganic carbons into carbohydrates utilizing light energy. However, parasitic plants are exceptions to this definition. They evolved to form a specialized organ, the haustorium, which allows parasitic plants to obtain water and nutrients from their host plants (Yoshida et al., 2016; Kokla and Melnyk, 2018). Depending on the haustorium attachment position on their host, parasitic plants are generally categorized as stem or root parasites. Based on their host dependency, parasitic plants are classified as hemiparasites or holoparasites. Holoparasitic plants have mostly lost their photosynthetic ability and need to rely entirely on their hosts to provide water and nutrients. Therefore, haustorium formation has been considered an essential element for plant parasitism (Yoshida et al., 2016; Kokla and Melnyk, 2018). Investigating the mechanism involved in haustorium development reveals how autotrophic plants evolved to acquire heterotrophic lifestyles. Our evolutionary developmental knowledge of haustorium formation also provides the foundation for developing more effective methods to control the agricultural damage caused by parasitic plants.
Cuscuta species (dodders) are the most well-known and widely spread stem holoparasitic plants. The Cuscuta genus comprises about 200 species and is the only genus that evolved parasitism in the Convolvulaceae (Yuncker, 1932). Cuscuta plants have degenerated roots and leaves and have stems that can coil around their host in a counterclockwise manner. Depending on the species and the growth conditions, Cuscuta stems are orange-yellow, yellow, or greenish-yellow because they only have a meagre amount of chlorophyll. Several previous studies on genomes of Cuscuta species indicate that Cuscuta species have lost some genes needed for efficient photosynthetic activity (McNeal et al., 2007; Braukmann et al., 2013; Sun et al., 2018; Vogel et al., 2018), which can be considered evidence for transitioning from hemiparasites to holoparasites. Therefore, obtaining water and nutrients from their host is the top priority for their survival. Unlike root parasitic plants that mostly depend on haustorium-inducing factors (HIFs) (Yoshida et al., 2016), the Cuscuta haustorium induction is also regulated by environmental signals such as light signals (Furuhashi et al., 2011). These unique lifestyles and morphological characteristics make Cuscuta species a good system to study how autotrophic plants evolved parasitism by attaching onto above-ground organs of their host plants.
Aside from the opportunities they present for investigating evo-devo mysteries, Cuscuta plants are the focus of intensive research since they cause massive agricultural losses. Several Cuscuta species are listed in multiple countries’ noxious weed lists (Holm et al., 1997) because they parasitize a wide range of important vegetable and fruit crops, and ornamental plants (Lanini and Kogan, 2005). Comparing with Striga species that mostly target monocot hosts, the host plants for Cuscuta species are primarily eudicots, but a few monocot crops can also reportedly be attacked by Cuscuta species (Lanini and Kogan, 2005). Cuscuta species are parasites on 25 crops in at least 55 countries (Holm et al., 1997; Lanini and Kogan, 2005). If left uncontrolled, Cuscuta growth will decrease host nutrient status and lead to reduced stand, canopy, biomass and fruit weight (Musselman, 1987; Lanini and Kogan, 2005). Yield reductions of 50–72% in tomatoes and 70–90% in carrots have been reported (Lanini and Kogan, 2005; Mishra et al., 2006). In temperate climates, yield losses up to 80–100% have been reported in cranberry in the US due to Cuscuta (Devlin and Deubert, 1980). In California alone, over 12,000 ha. are affected by Cuscuta (Lanini and Kogan, 2005). Even when crop rotation is practised, Cuscuta is hard to eradicate due to long-term seed viability. Therefore, a better understanding of the mechanisms involved in Cuscuta haustorium development is required to build more effective control strategies to stop the agricultural damages brought on by Cuscuta. During the past decade, progress has been made in understanding the interaction between Cuscuta species and their hosts, especially on the mechanism involved in resistance responses against Cuscuta species (Kaiser et al., 2015; Jhu and Sinha, 2022), and the signal exchange and horizontal gene transfer between Cuscuta plants and their hosts (Kim and Westwood, 2015; Wu, 2018; Yang et al., 2019; Lin et al., 2022). These aspects have been reviewed recently (Kaiser et al., 2015; Kim and Westwood, 2015; Wu, 2018; Jhu and Sinha, 2022). On the other hand, although review articles cover some specific aspects of Cuscuta haustorium development and parasitism behaviours (Yoshida et al., 2016; Shimizu and Aoki, 2019), several recent discoveries in the field have not been systematically reviewed yet.
This review summarises our current knowledge of the mechanism involved in the four stages of Cuscuta haustorium development, including the initiation, adhesion, penetration, and vascular connection. We discuss the unique characteristics of the parasitizing behaviours in Cuscuta species compared with other well-studied root parasitic plants. These unique features are compelling reasons to use Cuscuta species as model organisms for studying haustorium development in stem holoparasitic plants. We outline the advantages, unknown mysteries, and current limitations of the Cuscuta system. We also propose potential models and directions that might overcome these challenges.
2. Before haustorium formation: Finding their hosts
Cuscuta seeds are about 1 mm in size and cannot store sufficient nutrients to support seedling growth for longer than one week (Figures 1A–D). Therefore, finding a suitable host and forming successful haustorial attachments within a few days post-germination are important for seedling survival. Previous research indicates that Cuscuta seedlings can detect the volatiles released by their hosts and use these chemical cues to locate their preferred hosts (Runyon et al., 2006). In addition to airborne chemical signals, previous studies also indicate that the high far-red light relative to red light plays a vital role in controlling Cuscuta parasitism and growth directions (Furuhashi et al., 1995; Orr et al., 1996; Tada et al., 1996). This signal might help Cuscuta find green and healthy plants as their hosts (Orr et al., 1996). Blue light has been reported to be essential for the twining behavior of Cuscuta stems (Furuhashi et al., 1995; Furuhashi et al., 2021), which is tightly associated with the following haustorium induction phase.
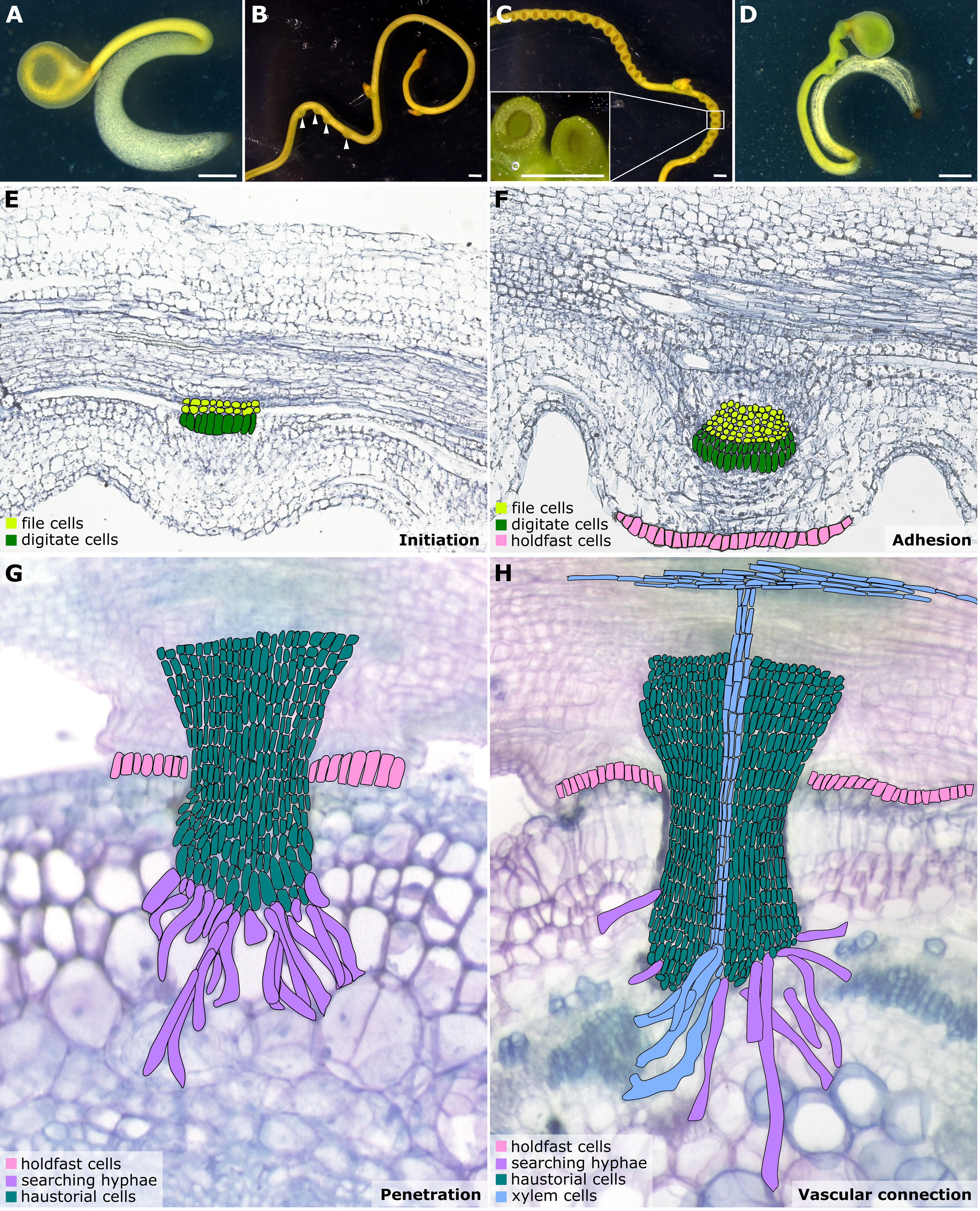
Figure 1 Illustrations of Cuscuta developmental stages and haustorium organogenesis. (A–D) Cuscuta campestris seedlings and strands with prehaustoria and haustoria at different developmental stages. (A) A Cuscuta campestris seedling. (B) A Cuscuta campestris strand with prehaustoria. White arrowheads indicate prehaustoria. (C) Haustoria with adhesive disks on a Cuscuta campestris strand. Adhesive disks are enlarged in the subfigure. (D) A Cuscuta campestris seedling autoparasitizes itself. (A–D) Scale bar = 1 mm. (E–H) Four major haustorium developmental phases in Cuscuta species. (E) Initiation phase: The prehaustorium structure appears because the disc-like meristem emerges in the inner cortex region of the Cuscuta stem. The prehaustorium primordia consist of file cells and digitate cells. (F) Adhesion phase: The epidermal cells of Cuscuta stems divide and differentiate into holdfast cells, which comprise the adhesive disk to secure the adhesion of haustorial attachments. (G) Penetration phase: The cortex-originated inner haustorial cells penetrate through the epidermis and cortex of their host tissues. At the tip of the haustorium, the inner haustorial cells elongate and differentiate into searching hyphae, which start invading through the apoplastic region of host cortex tissues and searching for host vasculature. (H) Vascular connection phase: Establishing a successful vascular connection is the final stage of haustorium organogenesis. If the searching hyphae reach host xylem cells or phloem cells, they obtain xylem or phloem identity and differentiate into xylem- or phloem-conductive elements, respectively. Part of this figure (B, C) is modified from images in a previously published paper Jhu et al., 2021 with new information added.
3. Cuscuta haustorium organogenesis
Haustorium organogenesis in Cuscuta species has been well-reviewed (Yoshida et al., 2016; Kokla and Melnyk, 2018; Shimizu and Aoki, 2019). However, the developmental stages are reported differently among references and diverse names are used to refer to the same stage, leading to some confusion. Here, we aim to summarise the Cuscuta haustorium organogenesis process and standardize the vocabulary used. Based on cell types and morphological characteristics, we classified haustorium organogenesis in Cuscuta species into four development stages: the initiation phase, adhesion phase, penetration phase, and vascular connection phase (Figures 1E–H).
3.1. Initiation phase (prehaustorium)
Once Cuscuta plants find their hosts and successfully coil around them, the next step is the start of haustorium initiation and formation of the prehaustorium, which is the immature haustorial structure seen prior to penetration of the host tissues (Yoshida et al., 2016). A group of cortical cells begin to accumulate starch-containing amyloplasts and enlarged nuclei. These cells are identified as the initial cells, which will then dedifferentiate and develop into haustorial meristem cells. The disc-like meristem that emerges in the inner cortex of the Cuscuta stem is the prehaustorium primordia, consisting of two types of cells: file and digitate cells (Figure 1E) (Lee, 2007). This primordium is also known as the “endophyte primordium” because it develops into an “endophyte.” An endophyte refers to the inner haustorial structure that grows into the host tissues during the penetration phase (Kuijt, 1977; Lee, 2007).
Based on previous studies, the two primary triggers for Cuscuta haustorium initiation are the far-red light signal and mechanical stimulation (Tada et al., 1996; Furuhashi et al., 2011) (Figure 2). Upon receiving these light signals and physical contacts, the prehaustorium structure starts to develop. Cuscuta species probably employ phytochromes to sense the change in red light and far-red light ratio and adopt phototropism signaling transduction to control the initiation of haustoria. In Arabidopsis, phytochromes are transformed from their inactive form (Pr) to their active form (Pfr) in high red-light environments. Pfr then translocates from the cytoplasm into the nucleus, where Pfr interacts with phytochrome interaction factors (PIFs). As a result of their interaction, PIFs are phosphorylated and then degraded. PIFs are transcription factors that regulate the downstream gene expression involved in skotomorphogenesis and photomorphogenesis in Arabidopsis. Cuscuta species likely co-opt similar signaling pathways and use phytochromes to control the genes involved in hormone transport or biosynthesis (Figure 2) (Furuhashi et al., 1997; Furuhashi et al., 2011; Furuhashi et al., 2021; Pan et al., 2022). A previous study indicates that strong far-red light or low red to far-red ratios (low red light and high far-red light mixture condition) promoted Cuscuta stem coiling and haustorium formation (Furuhashi et al., 1997; Haidar and Orr, 1999). The results of in vivo quantification of the percentage of phytochrome status also showed that a maximum number of prehaustoria were produced when a low percentage of phytochrome is in Pfr form (Haidar and Orr, 1999). This evidence supports the model that phytochromes are in the inactive (Pr) state under intense far-red light circumstances, which prevents phytochromes from entering the nucleus. PIFs are thus unrestricted from regulation and activate the genes, including those involved in auxin and cytokinin biosynthesis or transport, needed for haustorium initiation (Furuhashi et al., 2021; Pan et al., 2022) (Figure 2).
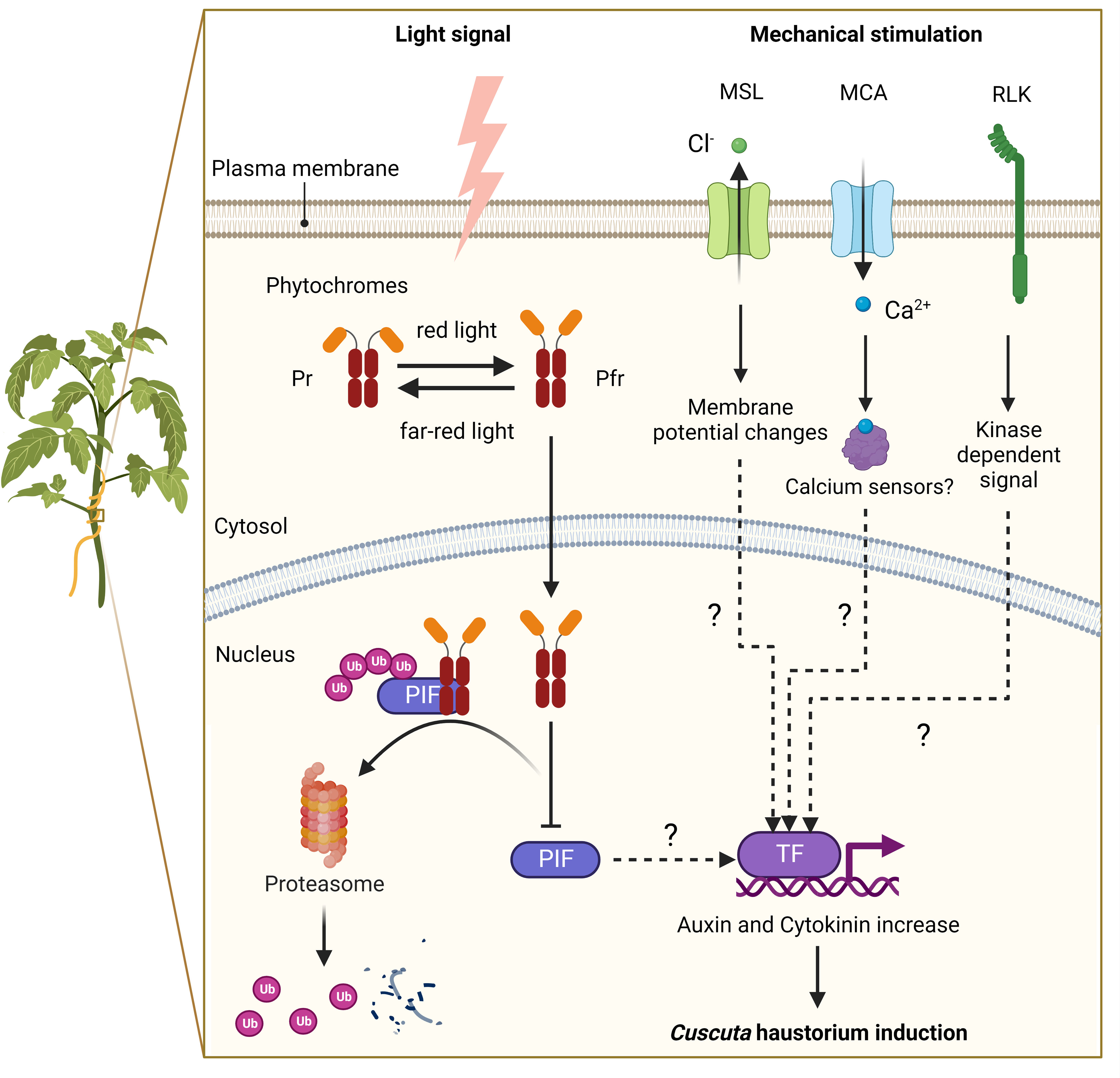
Figure 2 A putative model of signalling pathways for haustorium induction in Cuscuta species. Far-red light signal and mechanical stimulation are known to be the two major factors in inducing Cuscuta haustorium development. Cuscuta species likely have adopted far-red light signaling transduction and use phytochromes to regulate haustorium initiation. In high red-light conditions, phytochromes are converted from inactive form (Pr) to active form (Pfr), which will translocate from the cytosol into the nucleus and interact with phytochrome interacting factors (PIFs). PIFs are transcription factors, which are likely to regulate the downstream genes involved in hormone biosynthesis or transport. Interacting with Pfr phytochromes leads to PIFs phosphorylation and subsequent degradation. In high far-red light conditions, phytochromes are in the Pr inactive form and cannot enter the nucleus. Therefore, PIFs are released from repression and activate genes involved in haustorium initiation. Mechanosensing is another required element for Cuscuta haustorium development. A physical contact signal with hosts might activate mechanosensory proteins such as ion channels and receptor-like kinases. Mechanosensory ion channels elicit cytosolic Ca2+-dependent signaling and regulate downstream gene expression via unknown mechanisms. Receptor-like kinases trigger protein kinase cascades and then influence downstream gene transcription, which can lead to hormone status changes and haustorium induction.
Another component needed for forming the Cuscuta haustorium is physical contact with its host (Tada et al., 1996; Furuhashi et al., 2011). Several herbaceous and woody plant species have been described that can perceive mechanical cues from their environment and respond physiologically to change their growth or morphology by a process known as thigmomorphogenesis (Börnke and Rocksch, 2018). During the past decade, progress has been made in investigating the underlying molecular mechanisms of mechanoperception and thigmomorphogenesis in several plant species (Monshausen and Gilroy, 2009; Hamilton et al., 2015; Börnke and Rocksch, 2018; Mano and Hasebe, 2021), but how these thigmomorphogenesis signaling pathways are involved in Cuscuta haustorium organogenesis remains largely unknown. Here, we propose a hypothetical model based on our current understanding of non-parasitic plant models (Figure 2). Ion channels and receptor-like kinases are examples of mechanosensory proteins (Monshausen and Gilroy, 2009; Hamilton et al., 2015; Börnke and Rocksch, 2018) that may be activated by a physical contact signal with hosts. Plasma membrane-localized mechanosensitive calcium channels, like Mid1-complementing activity (MCA), trigger Ca2+ influx, cytosolic Ca2+-dependent signaling, and activate the expression of downstream genes possibly through Ca2+ sensors, such as calmodulin or calcium-dependent protein kinases (Nakagawa et al., 2007; Kurusu et al., 2013; Börnke and Rocksch, 2018; Mano and Hasebe, 2021). Another potential candidate is the mechanosensitive channel of small conductance (MscS) -like (MSL) family proteins, which are reported to be stretch-activated anion channels for Cl− and cause alteration in the membrane potential (Haswell et al., 2008; Maksaev and Haswell, 2012; Mano and Hasebe, 2021). Receptor-like kinases (RLKs) might also play a role in triggering protein kinase cascades and altering Ca2+ signaling (Humphrey et al., 2007; Shih et al., 2014; Börnke and Rocksch, 2018), which in turn may affect downstream gene transcription, change hormone status and induce haustorium formation (Figure 2).
3.2. Adhesion phase (attached haustorium)
In the adhesion phase, prehaustoria continue to grow toward the host and form adhesive disks (also known as holdfasts or upper haustorium). The Cuscuta haustorial epidermal cells in contact with the host proliferate anticlinally, elongate, and differentiate into secretory holdfast cells. These holdfast cells can secrete adhesive glue containing de-esterified pectins to secure the haustorium on their host’s surface and seal the gap between themselves and their host (Figure 1F) (Vaughn, 2002).
3.3. Penetration phase (invading haustorium)
Once a haustorium securely attaches to its host, the haustorium development enters the penetration phase (also known as intrusive phase), and the file and digitate cells in endophyte primordium continue to divide and form inner haustorial cells. These cortex-originated inner haustorial cells then penetrate through the epidermis and cortex of their host tissues. At the tip of the penetrating haustorium, the inner haustorial cells elongate and differentiate into searching hyphae (Vaughn, 2003). Searching hyphae continue invading through the apoplastic region of host cortex tissues and searching for host vasculature via tip growth (Figure 1G).
3.4. Vascular connection phase (mature haustorium)
The final stage of developing a functional haustorium is establishing a successful vascular connection between host and parasite. The searching hyphae continue tip growth to seek host vascular tissues. Once the searching hyphae reach the host vascular cells, they form interspecies plasmodesmata connections at the tip of the searching hyphae. These searching hyphae then convert their cell identity depending on the type of cells they contact. For instance, the searching hyphae that make contact with host xylem cells will obtain xylem identity and become xylic hyphae (Vaughn, 2006). The xylic hyphae differentiate into xylem conductive elements and form xylem bridges connecting the xylem system between the host and the parasite (Figure 1H). On the other hand, the searching hyphae that make contact with host phloem sieve elements will obtain phloem identity and become phloic hyphae (also known as absorbing hyphae) (Vaughn, 2006). The phloic hyphae differentiate into phloem-conductive elements with finger-like protrusions connecting the phloem system between the host and the parasite. The mechanisms involved in the transition of cell identity are currently unknown. However, based on the discovery of mRNA, small RNA, and small peptides exchanged between host and parasite via haustorial connection (Kim et al., 2014; Kim and Westwood, 2015; Shahid et al., 2018), we propose a potential hypothesis that these exchanged signals likely function as signals to facilitate cell identity conversion, which will be of interest for future investigation.
4. Cuscuta as a model for haustorium development in stem holoparasitic plants
Compared with our recent advances in understanding root parasitic plant haustorium development, our knowledge of haustorium development and parasitism behaviours on stem parasitic plants is relatively limited. According to an increasing number of research reports, the required signals for inducing haustorium formation are different, the parasitism behaviours between root and stem haustorium formation are distinct, and the mechanisms behind these phenomena are still not completely understood. Therefore, good model organisms representing stem holoparasitic plants will help investigate how parasitic plants evolved to have different strategies to effectively attach to various above-ground organs of their host. The Cuscuta genus is one of the most well-studied stem parasitic plants and here we summarized why they are the popular choices for scientists in this field.
4.1. Advantage 1: Distinct mechanisms involved in haustorium induction
A stem parasitic plant-specific model system is required because the mechanisms involved in haustorium induction differ between root and stem parasitic plants. For example, many studies indicate that detecting quinones or phenolics from the hosts, known as haustorium-inducing factors (HIFs), is a critical factor in inducing haustorium development for root parasitic plants, like those seen in the Orobanchaceae (Chang and Lynn, 1986; Goyet et al., 2019). On the other hand, stem parasites use tactile stimuli and light signals for haustorium induction (Tada et al., 1996; Furuhashi et al., 2011). These different factors required for triggering haustorium formation show the distinct strategies needed to adapt to underground and aboveground parasitism. For underground parasitism, physical contact and light signals would not be effective searching mechanisms for host root systems surrounded by soil. Consequently, the ability to detect host-specific HIFs would be the primary criteria for root parasitic plant haustorium induction. Therefore, using Cuscuta species for studying stem parasitic plant haustorium organogenesis could help us understand the unique mechanisms deployed in aboveground parasitism.
4.2. Advantage 2: Special parasitizing behaviors: Autoparasitism, hyperparasitism, cross-organ parasitism
Besides serving as a sound system for studying the distinctive mechanisms involved in haustorium initiation, Cuscuta species also perform some unique parasitizing behaviours that are currently underinvestigated, including hyperparasitism (Wilson and Calvin, 2017), autoparasitism (Krasylenko et al., 2021), and cross-organ parasitism (Jhu and Sinha, 2022). These unique parasitizing behaviours among multiple parasitic plants have been previously reviewed (Krasylenko et al., 2021; Jhu and Sinha, 2022). Therefore, here, we focus on providing clear definitions with concise discussion and then propose hypotheses to understand why Cuscuta species evolved these unique parasitizing behaviours.
Hyperparasitism indicates the phenomenon of a parasitic plant parasitizing another parasitic plant. For stem parasites that attach to the aerial part of their host, this phenomenon is also known as epiparasitism because they grow “on top of” another parasite (Wilson and Calvin, 2017). Several previous studies have reported Cuscuta hyperparasitism on other parasitic plants. Hyperparasitism can happen between species or within the same species (Hawksworth, 1996). When a parasitic plant parasitizes the same parasitic plant species, this is known as autoparasitism. One specific type of autoparasitism is when the parasitic plants form functional haustoria on themselves, which is also known as self-parasitism (Fineran, 1965). In Cuscuta species, both autoparasitism and self-parasitism are commonly observed (Krasylenko et al., 2021). This is a distinctive parasitism strategy compared with several facultative or obligate root parasitic plants, which have self- and kin-recognition mechanisms to avoid forming haustoria on themselves or their similar relatives.
Deploying hyperparasitism and autoparasitism strategies might be the evolutionary consequence of adapting to aboveground parasitism. One hypothesis proposed by McLuckie is that self-parasitic haustoria in Cassytha or Cuscuta species might facilitate water conduction and long-distance transport (McLuckie, 1924). In addition, based on the observation that Cuscuta species also often form attachments to non-biological materials (Bernal-Galeano et al., 2022), we propose that the formation of these non-conductive haustoria or self-parasitic haustoria might both serve as physical support for Cuscuta to spread over a wider area and reach longer distances, which increases the possibility of finding new hosts and is therefore, beneficial for parasite survival.
Cross-organ parasitism refers to the phenomenon of a parasitic plant forming nonconventional haustorial attachments with other host organs (Jhu and Sinha, 2022). For example, C. campestris is known as a parasitic stem plant but has been reported to be able to form haustoria on tomato seedling roots (Jhu and Sinha, 2022). The reason why Cuscuta species evolved to have the ability to parasitize different organs of their host is still a mystery. However, the mechanisms of haustorium induction and host-specificity might contribute to these characteristics. Previous studies indicate that parasitic plants that can form self-parasitic haustoria, like Cuscuta, Cassytha and some hemiparasitic Orobanchaceae, can form haustoria without depending on detecting host-specific HIFs and are also more likely to show hyperparasitism (Krasylenko et al., 2021). These parasites usually also have a wider host range. To parasitize different host species, which might have different anatomical structures, having a higher degree of haustorium plasticity and HIF-independent haustorium induction might be criteria to adapt to different host structures and form successful haustorial connections with them. Therefore, this higher degree of haustorium plasticity might also confer on Cuscuta the ability to form haustoria on different organs of their hosts.
4.3. Advantage 3: Whole genome and abundant transcriptome data are available
Another advantage of using Cuscuta species as a model organism is the availability of whole genome information and different types of transcriptome data. The genomes of C. campestris and C. australis were both published in 2018 (Sun et al., 2018; Vogel et al., 2018). The Cuscuta transcriptome profiles in different developmental stages and tissue types are readily available (Ranjan et al., 2014; Jhu et al., 2021; Jhu et al., 2022; Bawin et al., 2022). Cuscuta mobile mRNAs and miRNAs that can transfer into the host plants are also reported (Kim et al., 2014; Shahid et al., 2018). These resources will facilitate research on Cuscuta development and the interaction between hosts and Cuscuta plants.
4.4. Advantage 4: Easy propagation and in vitro haustorium system
Another key criterion that makes an organism a good model organism is the ability to propagate or reproduce easily in vast numbers. Cuscuta species can quickly propagate through vines and produce many fruits and seeds, which can remain viable for more than ten years (Lanini and Kogan, 2005; Goldwasser et al., 2012; Masanga et al., 2022). These characteristics make them excellent model organisms. In addition, as mentioned in previous sections, many studies have shown that far-red light signals and physical contacts can trigger Cuscuta haustorium formation (Furuhashi et al., 1995; Tada et al., 1996; Furuhashi et al., 1997; Haidar and Orr, 1999). Therefore, several in vitro methods of growing Cuscuta or inducing haustorium formation without hosts have also been developed simultaneously (Kaga et al., 2020; Jhu et al., 2021; Bernal-Galeano et al., 2022). These methods allow us to investigate the function of genes in haustorium organogenesis more effectively and remove the influence or variations created by host conditions.
4.5. Major limitations: Lack of efficient transformation systems to obtain stable Cuscuta transgenic lines
To study the function of genes, generating mutants or transgenic plants with specific gene knockouts or gene overexpression is one critical approach. Unfortunately, the current major limitation of using Cuscuta species as a model is lack of efficient transformation methods to obtain stable transgenic lines. Several methods for producing successful transformation events have been published in different Cuscuta species, including Cuscuta trifolii, Cuscuta reflexa, and Cuscuta europaea (Borsics et al., 2002; Švubová and Blehová, 2013; Lachner et al., 2020). However, regenerating the transformed cells into calli and whole plants is still an unsolved issue. Developing a transformation system to generate stable transgenic Cuscuta plants will be a significant breakthrough technology.
At the same time, scientists have been developing different tools and methods for functional gene studies to bypass and overcome the current transformation obstacles. Based on previous studies, Cuscuta haustoria not only transport water and nutrients but also can transport small RNAs, messenger RNAs and small peptides (Kim et al., 2014; Kim and Westwood, 2015; Shahid et al., 2018; Wu, 2018; Johnson and Axtell, 2019). Therefore, host-induced gene silencing (HIGS) is a commonly used method to knock-down specific gene expressions in Cuscuta by attaching them to transgenic hosts that carry RNA interference (RNAi) constructs targeting Cuscuta genes (Albert et al., 2006; Alakonya et al., 2012; Jhu et al., 2021; Jhu et al., 2022). This method has been used widely in the Cuscuta research field to conduct functional analysis on genes of interest.
5. Conclusions
This review summarises the four major developmental stages of haustorium organogenesis in Cuscuta species. We organize the current understanding of haustorium induction by light signals and physical contacts and propose a potential pathway for haustorium formation. The detailed mechanisms of phytochrome signalling, hormone regulation, and thigmomorphogenesis in Cuscuta haustorium development are still elusive and will be of interest for future research. We also discuss the four significant advantages of using Cuscuta species as model organisms for haustorium development research, including the unique haustorium induction mechanisms, parasitizing behaviours, abundant genetic resources, and easy propagation. The current major limitation is absence of efficient transformation systems to obtain stable Cuscuta transgenic lines. However, host induces gene silencing has been widely used to overcome this limitation. With several reports on successful transformation events published recently from different research groups, we are optimistic that stable Cuscuta transformation systems might be established in the near future.
Author contributions
M-YJ wrote the initial draft and prepared the figures. M-YJ and NRS both revised and approved the final manuscript. All authors contributed to the article and approved the submitted version.
Funding
M-YJ was supported by Taiwan Government GSSA Scholarship, Loomis Robert S. and Lois Ann Graduate Fellowship, Katherine Esau Summer Graduate Fellowship, Elsie Taylor Stocking Memorial Fellowship, Yen Chuang Taiwan Fellowship, and the UCD Graduate Research Award. NRS was funded by USDA-NIFA (2013-02345) and funding from the California Tomato Research Institute, Inc.
Acknowledgments
We thank Eli Marable’s feedback on the early draft of this manuscript, and Anindya Kundu and Victor Hugo Moura De Souza for their valuable discussion.
Conflict of interest
The authors declare that the research was conducted in the absence of any commercial or financial relationships that could be construed as a potential conflict of interest.
Publisher’s note
All claims expressed in this article are solely those of the authors and do not necessarily represent those of their affiliated organizations, or those of the publisher, the editors and the reviewers. Any product that may be evaluated in this article, or claim that may be made by its manufacturer, is not guaranteed or endorsed by the publisher.
References
Alakonya, A., Kumar, R., Koenig, D., Kimura, S., Townsley, B., Runo, S., et al. (2012). Interspecific RNA interference of SHOOT MERISTEMLESS-like disrupts cuscuta pentagona plant parasitism. Plant Cell 24, 3153–3166. doi: 10.1105/tpc.112.099994
Albert, M., Belastegui-Macadam, X., Kaldenhoff, R. (2006). An attack of the plant parasite cuscuta reflexa induces the expression of attAGP, an attachment protein of the host tomato. Plant J. 48, 548–556. doi: 10.1111/j.1365-313X.2006.02897.x
Bawin, T., Bruckmüller, J., Olsen, S., Krause, K. (2022). A host-free transcriptome for haustoriogenesis in cuscuta campestris: Signature gene expression identifies markers of successive development stages. Physiol. Plant 174(2). doi: 10.1111/ppl.13628
Bernal-Galeano, V., Beard, K., Westwood, J. H. (2022). An artificial host system enables the obligate parasite cuscuta campestris to grow and reproduce in vitro. Plant Physiol. 189, 687–702. doi: 10.1093/plphys/kiac106
Börnke, F., Rocksch, T. (2018). Thigmomorphogenesis – control of plant growth by mechanical stimulation. Sci. Hortic. 234, 344–353. doi: 10.1016/j.scienta.2018.02.059
Borsics, T., Mihálka, V., Oreifig, A. S., Bárány, I., Lados, M., Nagy, I., et al. (2002). Methods for genetic transformation of the parasitic weed dodder (Cuscuta trifolii bab. et gibs) and for PCR-based detection of early transformation events. Plant Sci. 162, 193–199. doi: 10.1016/S0168-9452(01)00536-2
Braukmann, T., Kuzmina, M., Stefanović, S. (2013). Plastid genome evolution across the genus cuscuta (Convolvulaceae): two clades within subgenus grammica exhibit extensive gene loss. J. Exp. Bot. 64, 977–989. doi: 10.1093/jxb/ers391
Chang, M., Lynn, D. G. (1986). The haustorium and the chemistry of host recognition in parasitic angiosperms. J. Chem. Ecol. 12, 561–579. doi: 10.1007/BF01020572
Devlin, R. M., Deubert, K. H. (1980). Control of swamp dodder on cranberry bogs with butralin. Proc. Northeastern Weed Sci. Society 1980. 34, 399–405.
Fineran, B. A. (1965). Studies on the root parasitism of exocarpus bidwillii hook. f. VI. haustorial attachment and the phenomenon of self-parasitism. Phytomorphology 15, 387–399.
Furuhashi, T., Furuhashi, K., Weckwerth, W. (2011). The parasitic mechanism of the holostemparasitic plant cuscuta. J. Plant Interact. 6, 207–219. doi: 10.1080/17429145.2010.541945
Furuhashi, K., Iwase, K., Furuhashi, T. (2021). Role of light and plant hormones in stem parasitic plant (Cuscuta and cassytha) twining and haustoria induction. Photochem. Photobiol. 97, 1054–1062. doi: 10.1111/php.13441
Furuhashi, K., Kanno, M., Morita, T. (1995). Photocontrol of parasitism in a parasitic flowering plant, cuscuta japonica chois, cultured in vitro. Plant Cell Physiol. 36, 533–536. doi: 10.1093/oxfordjournals.pcp.a078790
Furuhashi, K., Tada, Y., Okamoto, K., Sugai, M., Kubota, M., Watanabe, M. (1997). Phytochrome participation in induction of haustoria in cuscuta japonica, a holoparasitic flowering plant. Plant Cell Physiol. 38, 935–940. doi: 10.1093/oxfordjournals.pcp.a029254
Goldwasser, Y., Sazo, M. R. M., Lanini, W. T. (2012). Control of field dodder (Cuscuta campestris) parasitizing tomato with ALS-inhibiting herbicides. Weed Technol. 26, 740–746. doi: 10.1614/WT-D-11-00173.1
Goyet, V., Wada, S., Cui, S., Wakatake, T., Shirasu, K., Montiel, G., et al. (2019). Haustorium inducing factors for parasitic orobanchaceae. Front. Plant Sci. 10. doi: 10.3389/fpls.2019.01056
Haidar, M. A., Orr, G. L. (1999). The response of cuscuta planiflora seedlings to red and far-red, blue light and end-of-day irradiations. Ann. Appl. Biol. 134, 117–120. doi: 10.1111/j.1744-7348.1999.tb05242.x
Hamilton, E. S., Schlegel, A. M., Haswell, E. S. (2015). United in diversity: Mechanosensitive ion channels in plants. Annu. Rev. Plant Biol. 66, 113–137. doi: 10.1146/annurev-arplant-043014-114700
Haswell, E. S., Peyronnet, R., Barbier-Brygoo, H., Meyerowitz, E. M., Frachisse, J. M. (2008). Two MscS homologs provide mechanosensitive channel activities in the arabidopsis root. Curr. Biol. 18, 730–734. doi: 10.1016/j.cub.2008.04.039
Hawksworth, F. G. (1996). Dwarf mistletoes: biology, pathology, and systematics (Forest Service: US Department of Agriculture).
Holm, L. R., Doll, J., Holm, E., Pancho, J., Herberger, J. P. (1997). World weeds: natural histories and distribution. John Wiley & Sons.
Humphrey, T., Bonetta, D. T., Goring, D. R. (2007). Sentinels at the wall: Cell wall receptors and sensors. New Phytol. 176, 7–21. doi: 10.1111/j.1469-8137.2007.02192.x
Jhu, M. Y., Farhi, M., Wang, L., Zumstein, K., Sinha, N. R. (2022). Investigating host and parasitic plant interaction by tissue-specific gene analyses on tomato and cuscuta campestris interface at three haustorial developmental stages. Front. Plant Sci. 12. doi: 10.3389/fpls.2021.764843
Jhu, M. Y., Ichihashi, Y., Farhi, M., Wong, C., Sinha, N. R. (2021). LATERAL ORGAN BOUNDARIES DOMAIN 25 functions as a key regulator of haustorium development in dodders. Plant Physiol. 186, 2093–2110. doi: 10.1093/plphys/kiab231
Jhu, M.-Y., Sinha, N. R. (2022). Annual review of plant biology parasitic plants: An overview of mechanisms by which plants perceive and respond to parasites. doi: 10.1146/annurev-arplant-102820
Johnson, N. R., Axtell, M. J. (2019). Small RNA warfare: exploring origins and function of trans-species microRNAs from the parasitic plant cuscuta. Curr. Opin. Plant Biol. 50, 76–81. doi: 10.1016/j.pbi.2019.03.014
Kaga, Y., Yokoyama, R., Sano, R., Ohtani, M., Demura, T., Kuroha, T., et al. (2020). Interspecific signaling between the parasitic plant and the host plants regulate xylem vessel cell differentiation in haustoria of cuscuta campestris. Front. Plant Sci. 11. doi: 10.3389/fpls.2020.00193
Kaiser, B., Vogg, G., Fürst, U. B., Albert, M. (2015). Parasitic plants of the genus cuscuta and their interaction with susceptible and resistant host plants. Front. Plant Sci. 6. doi: 10.3389/fpls.2015.00045
Kim, G., LeBlanc, M. L., Wafula, E. K., dePamphilis, C. W., Westwood, J. H. (2014). Genomic-scale exchange of mRNA between a parasitic plant and its hosts. Sci. (1979) 345, 808–811. doi: 10.1126/science.1253122
Kim, G., Westwood, J. H. (2015). Macromolecule exchange in cuscuta-host plant interactions. Curr. Opin. Plant Biol. 26, 20–25. doi: 10.1016/j.pbi.2015.05.012
Kokla, A., Melnyk, C. W. (2018). Developing a thief: Haustoria formation in parasitic plants. Dev. Biol. 442, 53–59. doi: 10.1016/j.ydbio.2018.06.013
Krasylenko, Y., Těšitel, J., Ceccantini, G., Oliveira-da-Silva, M., Dvořák, V., Steele, D., et al. (2021). Parasites on parasites: hyper-, epi-, and autoparasitism among flowering plants. Am. J. Bot. 108, 8–21. doi: 10.1002/ajb2.1590
Kuijt, J. (1977). Haustoria of phanerogamic parasites. Annu. Rev. Phytopathol. 15, 91–118. doi: 10.1146/annurev.py.15.090177.000515
Kurusu, T., Kuchitsu, K., Nakano, M., Nakayama, Y., Iida, H. (2013). Plant mechanosensing and Ca2+ transport. Trends Plant Sci. 18, 227–233. doi: 10.1016/j.tplants.2012.12.002
Lachner, L. A. M., Galstyan, L., Krause, K. (2020). A highly efficient protocol for transforming cuscuta reflexa based on artificially induced infection sites. Plant Direct 4 (8), e00254. doi: 10.1002/pld3.254
Lanini, W. T., Kogan, M. (2005). Biology and management of cuscuta in crops. Int. J. Agric. Natural Resour. 32, 127–141. doi: 10.7764/rcia.v32i3.317
Lee, K. B. (2007). Structure and development of the upper haustorium in the parasitic flowering plant cuscuta japonica (Convolvulaceae). Am. J. Bot. 94, 737–745. doi: 10.3732/ajb.94.5.737
Lin, Q., Banerjee, A., Stefanović, S. (2022). Mitochondrial phylogenomics of cuscuta (Convolvulaceae) reveals a potentially functional horizontal gene transfer from the host. Genome Biol. Evol. 14, evac091. doi: 10.1093/gbe/evac091
Maksaev, G., Haswell, E. S. (2012). MscS-Like10 is a stretch-activated ion channel from arabidopsis thaliana with a preference for anions. Proc. Natl. Acad. Sci. U.S.A. 109, 19015–19020. doi: 10.1073/pnas.1213931109
Mano, H., Hasebe, M. (2021). Rapid movements in plants. J. Plant Res. 134, 3–17. doi: 10.1007/s10265-020-01243-7
Masanga, J., Oduor, R., Alakonya, A., Ngugi, M., Ojola, P., Bellis, E. S., et al. (2022). Comparative phylogeographic analysis of cuscuta campestris and cuscuta reflexa in Kenya: Implications for management of highly invasive vines. Plants People Planet 4, 182–193. doi: 10.1002/ppp3.10236
McLuckie, J. (1924). Studies in parasitism. i. a contribution to the physiology of the genus cassytha, part 1. in. Proc. Linn. Soc. New South Wales, 55–78.
McNeal, J. R., Arumugunathan, K., Kuehl, J., Boore, J. L., dePamphilis, C. W. (2007). Systematics and plastid genome evolution of the cryptically photosynthetic parasitic plant genus Cuscuta(Convolvulaceae). BMC Biol. 5, 55. doi: 10.1186/1741-7007-5-55
Mishra, J. S., Moorthy, B. T. S., Bhan, M. (2006). Relative tolerance of linseed (Linum usitatissimum) varieties to dodder (Cuscuta campestris) infestation. Indian Journal of Agricultural Sciences 76, 380–382.
Monshausen, G. B., Gilroy, S. (2009). Feeling green: mechanosensing in plants. Trends Cell Biol. 19, 228–235. doi: 10.1016/j.tcb.2009.02.005
Nakagawa, Y., Katagiri, T., Shinozaki, K., Qi, Z., Tatsumi, H., Furuichi, T., et al. (2007). Arabidopsis plasma membrane protein crucial for Ca2+ influx and touch sensing in roots. Proc. Natl. Acad. Sci. 104, 3639–3644. doi: 10.1073/pnas.0607703104
Orr, G. L., Haidar, M. A., Orr, D. A. (1996). Smallseed dodder (Cuscuta planiflora) phototropism toward far- red when in white light. Weed Sci. 44, 233–240. doi: 10.1017/s0043174500093838
Pan, H., Li, Y., Chen, L., Li, J. (2022). Molecular processes of dodder haustorium formation on host plant under low Red/Far red (R/FR) irradiation. Int. J. Mol. Sci. 23 (14), 7528. doi: 10.3390/ijms23147528
Ranjan, A., Ichihashi, Y., Farhi, M., Zumstein, K., Townsley, B., David-Schwartz, R., et al. (2014). De novo assembly and characterization of the transcriptome of the parasitic weed dodder identifies genes associated with plant parasitism. Plant Physiol. 166, 1186–1199. doi: 10.1104/pp.113.234864
Runyon, J. B., Mescher, M. C., de Moraes, C. M. (2006). Volatile chemical cues guide host location and host selection by parasitic plants. Sci. (1979) 313, 1964–1967. doi: 10.1126/science.1131371
Shahid, S., Kim, G., Johnson, N. R., Wafula, E., Wang, F., Coruh, C., et al. (2018). MicroRNAs from the parasitic plant cuscuta campestris target host messenger RNAs. Nature 553, 82–85. doi: 10.1038/nature25027
Shih, H. W., Miller, N. D., Dai, C., Spalding, E. P., Monshausen, G. B. (2014). The receptor-like kinase FERONIA is required for mechanical signal transduction in arabidopsis seedlings. Curr. Biol. 24, 1887–1892. doi: 10.1016/j.cub.2014.06.064
Shimizu, K., Aoki, K. (2019). Development of parasitic organs of a stem holoparasitic plant in genus cuscuta. Front. Plant Sci. 10. doi: 10.3389/fpls.2019.01435
Sun, G., Xu, Y., Liu, H., Sun, T., Zhang, J., Hettenhausen, C., et al. (2018). Large-Scale gene losses underlie the genome evolution of parasitic plant cuscuta australis. Nat. Commun. 9 (9), 1–8. doi: 10.1038/s41467-018-04721-8
Švubová, R., Blehová, A. (2013). Stable transformation and actin visualization in callus cultures of dodder (Cuscuta europaea). Biol. (Poland) 68, 633–640. doi: 10.2478/s11756-013-0188-0
Tada, Y., Sugai, M., Furuhashi, K. (1996). Haustoria of cuscuta japonica, a holoparasitic flowering plant, are induced by the cooperative effects of far-red light and tactile stimuli. Plant Cell Physiol. 37, 1049–1053. doi: 10.1093/oxfordjournals.pcp.a029052
Vaughn, K. C. (2002). Attachment of the parasitic weed dodder to the host. Protoplasma 219, 227–237. doi: 10.1007/s007090200024
Vaughn, K. C. (2003). Dodder hyphae invade the host: A structural and immunocytochemical characterization. Protoplasma 220, 189–200. doi: 10.1007/s00709-002-0038-3
Vaughn, K. C. (2006). Conversion of the searching hyphae of dodder into xylic and phloic hyphae: A cytochemical and immunocytochemical investigation. Int. J. Plant Sci. 167, 1099–1114. doi: 10.1086/507872
Vogel, A., Schwacke, R., Denton, A. K., Usadel, B., Hollmann, J., Fischer, K., et al. (2018). Footprints of parasitism in the genome of the parasitic flowering plant cuscuta campestris. Nat. Commun. 9 (1), 1–11. doi: 10.1038/s41467-018-04344-z
Wilson, C. A., Calvin, C. L. (2017). Metadata provide insights on patterns of epiparasitism in mistletoes (Santalales), an overlooked topic in forest biology. Botany 95, 259–269. doi: 10.1139/cjb-2016-0264
Wu, J. (2018). miRNAs as a secret weapon in the battlefield of haustoria, the interface between parasites and host plants. Mol. Plant 11, 354–356. doi: 10.1016/j.molp.2018.02.004
Yang, Z., Wafula, E. K., Kim, G., Shahid, S., McNeal, J. R., Ralph, P. E., et al. (2019). Convergent horizontal gene transfer and cross-talk of mobile nucleic acids in parasitic plants. Nat. Plants 5, 991–1001. doi: 10.1038/s41477-019-0458-0
Yoshida, S., Cui, S., Ichihashi, Y., Shirasu, K. (2016). The haustorium, a specialized invasive organ in parasitic plants. Annu. Rev. Plant Biol. 67, 643–667. doi: 10.1146/annurev-arplant-043015-111702
Yuncker, T. G. (1932). The genus cuscuta. Memoirs Torrey Botanical Club 18, 109–331. Available at: https://www.biodiversitylibrary.org/part/250866.
Keywords: Cuscuta, parasitic plants, haustorium, model organisms, parasitism, holoparasite, organ development, organogenesis
Citation: Jhu M-Y and Sinha NR (2022) Cuscuta species: Model organisms for haustorium development in stem holoparasitic plants. Front. Plant Sci. 13:1086384. doi: 10.3389/fpls.2022.1086384
Received: 01 November 2022; Accepted: 28 November 2022;
Published: 12 December 2022.
Edited by:
Simon Scofield, Cardiff University, United KingdomReviewed by:
Malay Das, Presidency University, IndiaCopyright © 2022 Jhu and Sinha. This is an open-access article distributed under the terms of the Creative Commons Attribution License (CC BY). The use, distribution or reproduction in other forums is permitted, provided the original author(s) and the copyright owner(s) are credited and that the original publication in this journal is cited, in accordance with accepted academic practice. No use, distribution or reproduction is permitted which does not comply with these terms.
*Correspondence: Min-Yao Jhu, myj23@cam.ac.uk; Neelima R. Sinha, nrsinha@ucdavis.edu