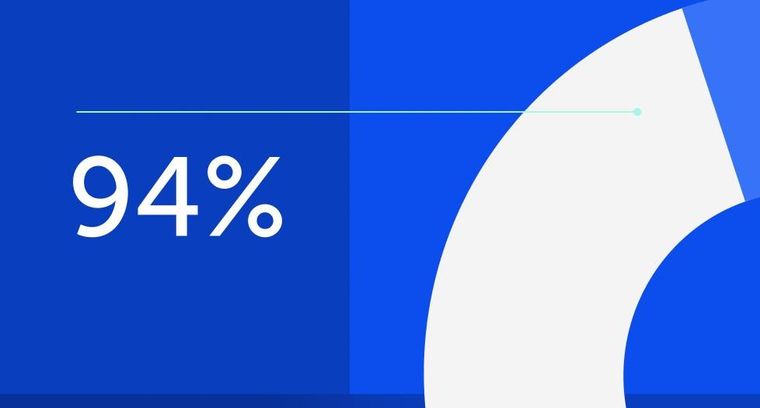
94% of researchers rate our articles as excellent or good
Learn more about the work of our research integrity team to safeguard the quality of each article we publish.
Find out more
ORIGINAL RESEARCH article
Front. Plant Sci., 14 December 2022
Sec. Plant Pathogen Interactions
Volume 13 - 2022 | https://doi.org/10.3389/fpls.2022.1080416
This article is part of the Research TopicThrough Thick and Thin - The Army of Secondary Metabolites in Plant-Fungi InteractionsView all 8 articles
Arbuscular mycorrhizal fungi (AMF) are considered biofertilizers for sustainable agriculture due to their ability to facilitate plant uptake of important mineral elements, such as nitrogen (N). However, plant mycorrhiza-dependent N uptake and interplant transfer may be highly context-dependent, and whether it is affected by aboveground herbivory remains largely unknown. Here, we used 15N labeling and tracking to examine the effect of aboveground insect herbivory by Spodoptera frugiperda on mycorrhiza-dependent N uptake in maize (Zea mays L.). To minimize consumption differences and 15N loss due to insect chewing, insect herbivory was simulated by mechanical wounding and oral secretion of S. frugiperda larvae. Inoculation with Rhizophagus irregularis (Rir) significantly improved maize growth, and N/P uptake. The 15N labeling experiment showed that maize plants absorbed N from soils via the extraradical mycelium of mycorrhizal fungi and from neighboring plants transferred by common mycorrhizal networks (CMNs). Simulated aboveground leaf herbivory did not affect mycorrhiza-mediated N acquisition from soil. However, CMN-mediated N transfer from neighboring plants was blocked by leaf simulated herbivory. Our findings suggest that aboveground herbivory inhibits CMN-mediated N transfer between plants but does not affect N acquisition from soil solutions via extraradical mycorrhizal mycelium.
Nitrogen (N) is one of the most important nutrients that limit plant growth and crop yield (Crawford and Glass, 1998). Beneficial microbes, such as mycorrhizal fungi, rhizobial bacteria, and plant growth promoting bacteria (PGPR), play a key role in the acquisition of plant nutrients in natural ecosystems (Etesami and Adl, 2020; Jaiswal et al., 2021; Ma et al., 2021). Arbuscular mycorrhizal fungi (AMF) belonging to the subphylum Glomeromycotina can establish mutually beneficial relationships with most land plants (including the most important crops) to form arbuscular mycorrhizae (Choi et al., 2018), in which AMF supply plants with multiple nutrients including N, and in return gain photosynthetic products from plants in the form of sugars (Ge et al., 2008; Boldt et al., 2011; An et al., 2019) and lipids (Jiang et al., 2017; Wang et al., 2017). The inorganic nutrients obtained through AMF promote plant growth and development (Smith et al., 2003; Smith et al., 2004; Yang et al., 2012; Wipf et al., 2019). Studies have shown that 1/3 of plant root N can be supplied by symbiotic AMF in the form of amino acids (Govindarajulu et al., 2005). Although the role of N in mycorrhizal symbiosis is not as clear as that of P, it has been demonstrated that AMF are able to absorb (Frey and Schüepp, 1993), (Tobar et al., 1994), and organic N (such as amino acids) (Cliquet et al., 1997). In addition, transporter genes involved in mycorrhizal N uptake have also been identified in many important crops, such as GmAMT3;1, GmAMT4;4, GmAMT4;1 and GmAMT1;4 in soybean (Glycine max L.) (Kobae et al., 2010), OsAMT3;1 in rice (Oryza sativa L.) (Pérez-Tienda et al., 2011), SbAMT3;1 in sorghum (Sorghum bicolor L.) (Koegel et al., 2013), and LeAMT4 and LeAMT5 in tomato (Solanum lycopersicum L.) (Ruzicka et al., 2012).
For the purpose of exploring nutrients, extraradical mycorrhizal mycelia (ERM) expand and grow, and by means of infection of neighboring plants and hyphal fusion, common mycorrhizal networks (CMNs) are formed to connect multiple plants, strengthening the connections, communications and especially the transport and exchange of nutrients among plants (Whiteside et al., 2019). Typically, CMNs play a crucial role in the distribution of nutrients from a shared resource pool among different plants. Plant-to-plant N transfer mediated by CMNs has important implications for improving yields in agricultural and forest systems (He et al., 2003; Hodge and Storer, 2015). In many cases, N is transferred from N-fixing or N-rich mycorrhizal plants to N-poor or non-N-fixing mycorrhizal plants through CMNs, for example, from soybean to maize (Zea mays L.) (Wang et al., 2016), from alfalfa (Medicago sativa L.) to maize (Zhang et al., 2020), and from faba bean (Vicia faba L.) to wheat (Triticum turgidum L.) (Wahbi et al., 2016) in intercropping systems. However, some studies have also shown that CMN-mediated N transfer from non-N-fixing to N-fixing mycorrhizal plants also occurs, and that such transfer is bidirectional (Johansen and Jensen, 1996; He et al., 2004). Therefore, CMN-mediated interplant N transfer may be widespread, and plant-derived N transfer can be viewed as a complement to plant mycorrhiza-dependent N uptake.
The improvement of plant mineral nutrition by ERM or CMNs has been widely recognized (Wipf et al., 2019), however, in natural environments, mycorrhizal plants often interact with other biotic or abiotic factors (Almario et al., 2022). Therefore, a comprehensive understanding of how mycorrhizal plants interact with other biotic or abiotic factors is particularly important for understanding the improvement of crop mineral nutrition by ERM or CMNs. Aboveground herbivory is one of the main factors limiting agricultural production (Oerke, 2006). Herbivory usually impairs carbon (C) fixation in plants by directly depleting plant photosynthetic tissue or indirectly inhibiting photosynthesis (Nabity et al., 2013), and this may affect nutrient exchange and transfer between plants and AMF. Currently, researchers are interested in how aboveground herbivory affects nutrient exchange between plants and AMF. It has been reported that the aboveground herbivory by the generalist insect Helicoverpa punctigera inhibits plant mycorrhiza-dependent P uptake (Frew, 2021); Charters et al. revealed that host plants transferred less C to their symbiotic fungal partners upon aphid attack, whereas the P supply from AMF to host plants was unaffected (Charters et al., 2020). This report demonstrated that nutrient exchange between AMF and host plants can be quantified in the presence of exogenous sap-sucking herbivores (Merckx and Gomes, 2020). In addition, AMF colonization and AMF-mediated plant growth responses are not affected by aphid feeding or by N or P uptake (Charters et al., 2022). The different effects of aboveground herbivory on mycorrhizae-mediated mineral nutrient uptake may be related to herbivorous types, AMF or plant species. Therefore, mycorrhiza-dependent mineral nutrient uptake may have a high context dependency (Pozo et al., 2015).
Maize is an important economic crop as well as a model plant for studying mycorrhizal symbiosis. Insect herbivores, especially invasive fall-armyworm (Spodoptera frugiperda) in Africa and Asia, are likely to be a major constraint on maize production in these regions (Xiao et al., 2020; Paredes-Sánchez et al., 2021; Prasanna et al., 2022). In this study, we systematically evaluated N acquisition from soil by ERM and N transfer from neighboring plants via CMNs. Aboveground herbivory did not affect mycorrhiza-dependent N acquisition from soil but inhibited CMN-mediated N interplant transfer in maize plants.
The mycorrhizal colonization rate in maize roots was measured five weeks post Rhizophagus irregularis (Rir) inoculation as illustrated in Experiment I (Figure 1A). The mycorrhizal symbiosis relationship was well developed, with colonization rate over 60% in maize roots (Figure 1B), and the typical structures of vesicles, hyphae and arbuscules were observed by staining the mycorrhizal roots (Figure 1C), showing successful mycorrhizal colonization in maize plants. Furthermore, mycorrhizal inoculation on maize plants led to increases in plant height by 11.9% (P< 0.001, Figure 1D), leaf area by 17.1% (P< 0.001, Figure 1E), and dry weight by 25.3% (P< 0.001, Figure 1F). Compared to non-mycorrhizal maize plants, P content in arbuscular mycorrhizal maize plants was significantly increased (Figure 1G), and the expression of mycorrhizal inducible phosphate transporter gene ZmPht1;6 (a mycorrhizal symbiosis marker gene in maize (Yu et al., 2018)) was increased by 54.5% (P< 0.001, Figure 1H). In addition to P, N accumulation was also enhanced by 32.5% in mycorrhizal symbiosis plants (P< 0.001, Figure 1I), and the expression of three N transporter genes (including ZmAMT3;1, a recently reported mycorrhiza induced N transporter gene) was upregulated during mycorrhizal symbiosis (Figures 1J-L).
Figure 1 Mycorrhizal symbiosis promotes the growth and nutrient uptake of maize plants. Maize plants were inoculated with Rhizophagus irregularis (Rir) and cultivated in self-designed boxes (A). Five weeks post-Rir inoculation, maize plant roots were harvested for the measurement of mycorrhizal root colonization rate (B) and mycorrhizal observation by wheat germ agglutinin (WGA) fluorescence staining (C) (bar scale: 25 μm). Plant height (D), leaf area (E), and dry weight (F) were measured, followed by the analysis of phosphorus content (G), ZmPht1;6 (a mycorrhizal symbiosis marker gene in maize) expression (H), nitrogen content (I) and N transporter gene expressions (J–L). For analysis of root colonization, n = 6; plant height and leaf area, n = 20; dry weight, n = 10; nitrogen content, n = 5; phosphorus content, n = 8; expression of ZmPht1;6 and N transporter genes, n = 3. Data are means ± SE. Asterisks at the top of the bars indicate statistical significance (Student’s t-test, *P < 0.05; ***P < 0.001; ns, no significance). NM, non-mycorrhizal maize plants; AM, arbuscular mycorrhizal maize plants.
The exchange of nutrients between host plants and mycorrhizal fungi is considered the main benefit for the two symbiotic partners (Wipf et al., 2019). Therefore, we determined whether maize plants acquire N through mycorrhizal pathways similar to other plants described in previous studies (Frey and Schüepp, 1993; Tobar et al., 1994). As illustrated in Experiment II (Figure 2A), the PVC core was rotated daily to cut the extraradical mycelium (ERM) to block the 15N transfer from the PVC core to plant roots. Rotation treatment did not affect root colonization compared to nontreated (Intact) maize plants (Figure 2B). 15N accumulation in the shoots of maize plants with ERM into a PVC core (Intact) was significantly higher than that in the plants that did not obtain N from PVC core (Rotated) (Figure 2C; S1A), reaching a net 15N accumulation of 1.01 mg/per plant shoot. A consistent result was also observed in maize roots, in that the rotation treatment decreased 15N accumulation compared to Intact treatment (Figure 2D; S1B). These results further confirmed that maize plants acquired N through the mycorrhizal pathway via ERM from the soil habitat.
Figure 2 Mycorrhiza-mediated nitrogen uptake from soil by maize plants. Maize plants were inoculated with the mycorrhizal fungus Rhizophagus irregularis (Rir) and cultivated in the boxes with a PVC core in each box (A). Five weeks post Rir inoculation, the PVC core was injected with (15NH4)2SO4 solution, and was then either rotated daily (for the purpose of damaging the mycelium) or non-rotated (intact for maintaining mycelial contact to labeling 15N). Plants were harvested to measure the root colonization rate (B) and the accumulation of shoot 15N (C) and root 15N (D) ten days after labeling N injection. For the analysis of root colonization, n = 6; 15N accumulation, n = 4. Data are the means ± SE of biological replicates. Asterisks indicate significant differences (Student’s t-test, *P < 0.05; ***P < 0.001; ns, no significance).
Aboveground herbivorous insects are generally considered to be important external biological sinks for plant carbon and may influence symbiotic relationships and nutrient transfer between plants and AMF by directly competing with AMF for plant carbon resources (Charters et al., 2020). To investigate the effect of aboveground herbivory on maize uptake of N from soil via ERM, we used simulated herbivory (Wounding + Oral secretion, W+OS, Figure S2, Figure 3A) to exclude 15N loss due to insect consumption and the differences in S. frugiperda feed intake. Our experimental results showed that rotation treatment had no effect on root colonization rate (Figure 3B), while W+OS treatment slightly decreased root colonization (12.1% decrease; P = 0.014; Figure 3B). However, the W+OS treatment did not affect the ammonia uptake of maize plants by the mycorrhizal pathway via ERM. There was no significant difference in receiving 15N from Rir between the control and W+OS treatment in both shoots (Figure 3C, S3A) and roots (Figure 3D, S3B). Meanwhile, the expression of the mycorrhizal-induced N transporter gene ZmAMT3;1 was upregulated during symbiosis (AM), but it was not affected by simulated herbivory (Figure 3E, P = 0.735), implying that herbivory has no obvious influence on mycorrhiza dependent N uptake. Together, these results together indicated that N uptake by the mycorrhizal pathway from soil in maize is independent of insect herbivory.
Figure 3 Mycorrhiza-mediated nitrogen uptake from soil by maize plants is independent of aboveground herbivory. Maize plants were inoculated with Rir and were cultivated in self-designed boxes, with a PVC core in each box as illustrated in experiment III. Five weeks post Rir inoculation, the PVC core was injected with (15NH4)2SO4 solution, and was then either rotated daily (for the purpose of severing the mycelium) or nontreated (Intact) for 10 days, during which W+OS treatment was introduced (A). Plants were then harvested to measure the root colonization rate (B), shoot 15N (C), root 15N (D), and ZmAMT3;1 (a mycorrhizal inducible nitrogen transporter gene) expression (E). The 15N in Intact-’W+OS’ minus the 15N in Rotated-’W+OS’ (to counteract the effect of root acquisition of 15N) was present as a ‘Control’, and 15N in Intact+’W+OS’ minus the 15N in Rotated+’W+OS’ (to counteract the effect of herbivory on root acquisition of 15N) was present as ‘W+OS’ in (C) and (D). NM, non-mycorrhizal roots; AM, arbuscular mycorrhizal roots. For the analysis of root colonization, n = 6; 15N accumulation, n = 4; ZmAMT3;1 expression, n = 3. Data are the means ± SE of biological replicates. Asterisks indicate significant differences (Student’s t-test, *P < 0.05; ns, no significance).
To examine CMN-mediated N transfer between maize plants, we designed a mesocosm, as illustrated in experiment IV (Figures S4A, B), to investigate whether maize plants obtain N from the 15N-labeled plants by CMNs (Figure 4A). The colonization rates in maize roots were high (Figures 4B, E), suggesting that the CMNs between two the maize plants were well established and that Rir infection was not affected by CMN damage or herbivory treatment. In 15N-labeled plants, the content of 15N was higher in the shoot than in the roots, since 15N was injected into plant shoots (Figures 4F, G; S5D, E). In addition, no statistical difference was detected among the different treatments in labeled plant shoots (Figure 4F; S5D), suggesting that CMN damage and simulated herbivory (W+OS) had no effect on 15N transport from the shoot to the roots in labeled maize plants. However, the CMN Intact treatment resulted in increased 15N accumulation in unlabeled plant roots (P< 0.001, Figure 4D; S5C) and shoots (P< 0.001, Figure 4C; S5B) compared to the CMN-damaged treatment. These results demonstrated that 15N injected in labeled maize shoots could flow to the roots and thus transfer to unlabeled maize plants by CMNs. This further confirmed that the maize plant was able to receive N from its neighboring plant by CMNs as with other plant species described in other previous studies (He et al., 2003; Wang et al., 2016). Interestingly, the same results were observed in W+OS treatment in that the 15N accumulation were also reduced both in unlabeled plant roots (P< 0.001, Figure 4D) and shoots (P< 0.001, Figure 4C), suggesting that the exportation of 15N via intact CMNs was restrained due to aboveground W+OS treatment. In addition, the reduced 15N accumulation in unlabeled plants caused by W+OS treatment was abolished when the CMNs were damaged. These unlabeled plants with damaged CMNs showed almost the same amount of 15N in roots and shoot (Figures 4C, D; S5B, C). Additionally, the reduction of 15N accumulation in unlabeled plants caused by CMN-damage treatment was eliminated by W+OS treatment. These results indicate that aboveground herbivory could block CMN-mediated N transfer from the labeled plant to the unlabeled plant. It is possible that 15N could be exudated from the roots of labeled maize plants and therefore, diffuse in soil. Thus, maize plants were each cultivated in a single pot and either 15N labeled ((15NH4)2SO4 injected in shoot) or nontreated. The soils of the two treatments were then used to detect the exudation of 15N from maize in the soil. δ15N of labeled maize soil was not significantly different from unlabeled controls (natural 15N abundance) (Figure S6), implying that there was no 15N or only an extremely small amount of 15N (derived from (15NH4)2SO4) that exuded from the roots of the labeled maize plant during the experimental period. These results suggest that the increased 15N accumulation in Intact CMN unlabeled maize plants was obtained through CMNs rather than an extended ERM or root apparatus. Therefore, we confirmed that the CMN-mediated 15N transfer between maize plants and the CMN-mediated 15N transfer was inhibited by aboveground herbivory.
Figure 4 Common mycorrhizal network-mediated N transfer between maize plants was affected by herbivory. Maize plants were inoculated with Rir and cultivated in self-designed mesocosms as demonstrated in experiment IV (A), RC, root compartment; CMNs, common mycorrhizal networks; ERM, extraradical mycorrhizal mycelia. The CMNs established between the two maize plants were either damaged daily or intact. Five weeks after Rir inoculation the mycorrhizal colonization rate in the roots was measured (B, E). Thereafter, 10 days after 15N labeling and W+OS treatment, 15N was detected in unlabeled plants (C, D) and 15N-labeled plants (F, G). Data are the means ± SE of the three (or six) biological replicates. Asterisks indicate significant differences (Student’s t-test, *P < 0.05; ***P < 0.001; ns, no significance).
Mycorrhizal symbiosis ubiquitously exists in terrestrial ecosystems (Smith and Smith, 2011; Hazard and Johnson, 2018). The formation and regulation of the symbiotic relationship depend on nutrient reciprocity (Ferlian et al., 2018). The bidirectional exchange of “carbon-mineral nutrients” has been extensively studied (Wipf et al., 2019). Consistent with many previous studies, our results showed that mycorrhizal colonization significantly promoted the biomass accumulation and uptake of mineral nutrients such as N in maize plants (Figure 1). N is a crucial nutrient element for plant growth and development in agricultural and natural ecosystems (Crawford and Glass, 1998; Kennedy et al., 2015). The existence of arbuscular mycorrhiza-dependent N uptake pathways in plants has been reported (Govindarajulu et al., 2005; Wang et al., 2020). Therefore, the management of mycorrhiza-dependent N uptake pathways has been considered a feasible strategy for developing sustainable agriculture (Thirkell et al., 2017; Verzeaux et al., 2017), especially for intensive agriculture (Rillig et al., 2019). Our results showed that, except for the root apparatus, mycorrhizal maize plants could also absorb from soil solution via ERM (Figure 2).
Meanwhile, our experiments confirmed that mycorrhizal maize could acquire N from neighboring plants through CMNs (Figure 4). Notably, most of the N delivered by CMNs stayed in the mycorrhizal roots (Figure S5C), while only a small amount of labeled 15N in shoots was detected (Figure S5B), implying that CMNs transferred N from labeled plants might have not been well absorbed and assimilated by maize plants. This may be related to the short time span of our experiments or the need for plant-derived N to complete the complex transformation in the fungus. Nevertheless, the increased accumulation of 15N in the shoots of mycorrhizal maize plants suggests that mycorrhizal maize plants could obtain and utilize N from the CMNs connected to neighboring plants. Two recent studies demonstrated that the root-specific and arbuscular mycorrhizal inducible gene, ZmAMT3;1, in maize is a high-affinity ammonium transporter that is essential for transfer to plants via the peri-arbuscular membrane, and it contributes about 70% to mycorrhiza-dependent uptake of N (Moulin, 2022.; Hui et al., 2022). Therefore, a large proportion of labeled 15 might be transferred by ZmAMT3;1 in the mycorrhizal maize roots and thus transported to the shoots. Together, these results suggests that multiple mycorrhiza-dependent N uptake pathways exist in maize plants, by either ERM or CMNs (Figure 5A), to improve the utilization of N from different sources.
Figure 5 Diagram of pathways for N uptake in maize plants. (A) Pathway for maize plant N uptake under no stress. (B) Diagram of the pathway for maize plant N uptake under herbivory. Myc-N, N uptake by mycorrhizal pathway from soil; root-N, N uptake by root from soil; transfer-N, N obtained from the CMN-connected neighboring plant; , N transferred from a CMN-connected neighboring plant was blocked.
Plants interact with a variety of other organisms simultaneously in the natural environment, including AMF and aboveground herbivorous insects, and these interactions often affect plant nutrient uptake and utilization (Frew and Price, 2019; Charters et al., 2020). Therefore, the extent to which plants benefit from AMF colonization largely depends on environmental conditions (Pozo et al., 2015). Chewing insects, such as S. frugiperda, consume a large proportion of plant biomass (carbon source) (Giri et al., 2006; Nabity et al., 2009). In addition, insect herbivory can influence the carbon source intensity of plants by inducing JA defense signaling and/or altering net photosynthetic rates (Kerchev et al., 2012; Nabity et al., 2013). According to the carbon limitation hypothesis, aboveground herbivory might lead to reduced carbon transfer from host plants to fungi, thereby impairing mycorrhizal colonization (Gehring and Whitham, 1994). In this study, aboveground herbivory slightly inhibited mycorrhizal colonization in maize plants (Figure 3B). Other studies have shown that aboveground herbivory can either increase, decrease or have no effect on mycorrhizal colonization (Charters et al., 2020; Frew, 2021; Charters et al., 2022). The well-established symbiotic relationship underlays plant mycorrhiza-dependent mineral nutrient uptake, so that reduced colonization caused by aboveground herbivory might, to some extent, affect N uptake by mycorrhizal maize from soil solutions via ERM. However, the results of 15N tracer experiments showed that this fraction of N uptake from soil solution by ERM was not affected by aboveground herbivory (Figures 3C, D, S7). These results are similar to the conclusion by Charters et al. that aboveground aphid infestation does not affect plant mycorrhiza-dependent P uptake (Charters et al., 2020; Charters et al., 2022) but differs from that of Frew (Frew and Price, 2019; Frew, 2021). The variability of these results highlights that host plant–AMF symbiosis and nutrient transfer might be influenced by a range of specific environmental factors, such as host and fungal species, the degree of stress or soil nutrient status (Barto and Rillig, 2010). In addition, the expression of the maize ZmAMT3;1 gene, which is responsible for transport in the mycorrhizal pathway, was not affected by aboveground herbivory neither (Figure 4B).
Numerous studies have demonstrated that N transfer between plants can occur directly through ERM in CMNs (He et al., 2009). Such CMN-mediated N transfer between plants might have practical implications for plant growth, especially in N-deficient ecosystems (Sprent, 2005). Therefore, understanding the CMN-mediated N dynamics and N distribution in agroecosystems is important for exploring crop N utilization. However, it remains unclear whether biotic stress affects CMN-mediated interplant N transfer. Surprisingly, our results showed that aboveground herbivory blocked the CMN-mediated transfer of 15N from the labeled neighboring plants (Figure 4). Similarly, N accumulation promoted by CMNs was also inhibited by aboveground herbivory (Figure S8). This implies that aboveground herbivory not only affects the CMN-dependent N uptake of host maize, but also regulates maize–plant interactions through CMNs. At present, the molecular mechanism by which aboveground herbivory affects CMN-mediated mineral nutrients transfer is still unknown. However, several studies have shown that CMN-mediate interplant defense–signaling transfer. As Song et al. reported that CMNs could mediate disease defense signaling between tomato plants (Song et al., 2010), CMNs have served as a “pipeline” for defense signaling transfer in various mycorrhizal plants, including Vicia faba L (Babikova et al., 2013; Babikova et al., 2013; Cabral et al., 2019)., citrus (Poncirus trifoliata L.) (Zhang et al., 2019), and potato (Solanum tuberosum L.) (Alaux et al., 2020). A recent study on tobacco (Nicotiana attenuata) showed that the ERMs of CMNs expanded the scale of plant defenses and might act as a channel for the transmission of defense signals and terpenoids that help neighboring plants build better defense responses (Song et al., 2019). These findings highlight the complexity of studying the ecological functions of CMNs.
Resource relocation is an important strategy for plant tolerance to insect herbivory (Züst and Agrawal, 2017). Reallocation of key nutrients such as N is vital for plant tolerance to insect herbivory. Upon aboveground herbivory plants may shut down the exchange from neighboring plants via CMNs to enhance plant tolerance to insect herbivory although the detailed mechanism is still unknown. Therefore, we speculate that when mycorrhizal maize plants are at risk of herbivory, they use widespread underground CMNs to transmit ‘danger signals’ to neighboring plants, and the ‘warned’ neighboring plants limit CMN-mediated interplant N transfer for better survival under the coming herbivory (Figure 5B).
N is a key nutrient element in both natural and agricultural ecosystems. Mycorrhiza-dependent N acquisition and CMN-mediated N transfer between neighboring plants have important ecological implications. Although the molecular basis for how nutrient transfer and stress signaling occur is lacking, it is clear that CMN-mediated distribution and transmission of such nutrients, chemicals, and defense signaling has broad implications for ecosystems, pest management, and sustainable agriculture (Bücking et al., 2016; Oelmüller, 2019).
Maize seeds (Zhengdan 958, purchased from Shandong Luyan Agricultural Seed Co., Ltd., China) were sterilized by 2% NaClO for 10 min and then placed in a seedling substrate (German Dahan peat soil, type: 413, particle size: 0-6 mm) for germination. After 7 d, consistent healthy seedlings were selected for transplantation for subsequent experiments. The maize plants were cultivated in an artificial intelligence greenhouse (14 h/10 h, light/dark) with a day and night temperature regime of 30°C (16 h) and 25°C (8 h). The relative humidity of the greenhouse was 70%. The maize plants were properly watered with low-phosphorus Hoagland nutrient solution.
The original strain of Rhizophagus irregularis (Rir, BGC GD01C) was obtained from the Institute of Plant Nutrition and Resources, Beijing Academy of Agriculture and Forestry. Maize plants grown in sands were used for reproduction of Rir stain. For mycorrhizal inoculation, the inocula (the mixture of sands, spores, mycelia and root segments) were mixed with high-temperature sterilized sands in a ratio of 1:6, and then were put into plastic pots in which the maize plants were planted.
The original insect population of Spodoptera frugiperda used in the experiments was obtained from the laboratory of Professor Lin Jintian, Zhongkai University of Agriculture and Engineering, and was raised and bred for more than 10 generations on artificial diets without exposure to any pesticides. The adults were raised in 10% honey water. The rearing chamber was kept under constant environmental conditions (25 ± 2°C, 60% RH, L:D = 16:8 h).
A box was separated into two equal-sized compartments by two layers of 30–35 μm nylon mesh (which allowed passage of AMF mycelia but not roots) with an interval of 1 cm (Figure 1A). Maize plants grown in the left compartment were either inoculated with Rir (AM) or with high-temperature sterilized Rir (NM; 121°C for 1 h twice). For AM treatment, the mycelium could extend into the right compartment to absorb the substances via the mycorrhizal pathway. Five weeks post inoculation, maize plants were harvested for the analysis of dry weight and nutrient content.
At the time of planting (all maize plants were inoculated with Rir), PVC cores, each with a stainless-steel tube in the center (made according to Charters et al. (Charters et al., 2020)), were inserted into the pot (15 cm × Φ13 cm), with one PVC core per pot (Figure 2A). Five weeks post-planting, the pot was filled with AMF ERM, and then a dose of 15N (2.2 mg) was introduced into the central tubes in an aqueous solution. The 15N solution was added every two days for all PVC cores. Half of the PVC cores were rotated every day to sever the connection of the ERM inside and outside the PVC core and set as the ‘Rotated’ treatment; the other half of the PVC cores were not rotated and set as the ‘Intact’ treatment. Ten days later, maize plants were harvested to determine 15N uptake.
The design of this experiment is shown in Figure 3A, and the maize leaves were treated with simulated herbivory. Maize plant cultivation, 15N labeling, and PVC core rotation treatment were conducted as described in experiment II. To minimize consumption differences and 15N losses due to insect chewing, insect herbivory was simulated by mechanical wounding + oral secretions (W+OS) of S. frugiperda larvae. Oral secretions were collected as described in Figure S2. 15N injection and PVC core rotation were carried out every two days, five times in total) (Figure 3A). Thus, four treatment groups in this part of experiment, included: Rotated-’W+OS’, Intact-’W+OS’, Rotated+’W+OS’, and Intact+’W+OS’. Among the four treatment groups, Rotated-’W+OS’ and Rotated+’W+OS’ were used to exclude experimental errors caused by direct absorption of 15N by plant roots due to soil solution flow or soil capillarity. Therefore, the value of 15N in Intact-’W+OS’minus the value of 15N in Rotated-’W+OS’ was present as the ‘control’ in the final result, and the value of 15N in Intact+’W+OS’ minus the value of 15N in Rotated+’W+OS’ was present as ‘W+OS’ in the final result. Ten days post-treatment, the plants were harvested for biomass and 15N content analyses.
The mesocosm design for this experiment was based on the classical compartmental partition method (Song et al., 2010). The mesocosm design schematic diagram is shown in Figure S4A. The mesocosm frame was made of a box (length: width: height = 30: 18: 15 cm), and the box was separated into two equal-sized compartments by two layers of 30–35 μm nylon mesh (which excluded root contact between plants but allowed the passage of ERM) with an interval of 1 cm. The narrow interval space between the two layers of nylon mesh was called the mycelia compartment (MC). Both the left and right compartments were planted with maize plants, and the compartment with plant roots was named the root compartment (RC). Maize plants grown in the left RC were inoculated with Rir, while maize plants grown in the right RC were non-inoculated. The mycelium of the mycorrhizal maize roots in the left RC could pass through the nylon mesh and thus reinfect the non-inoculated maize roots to form CMNs. The purpose of setting double-layer nylon mesh was mainly to increase the distance of the root-free zone between two maize plant roots, which could prevent the direct transfer of nutrients, water or root exudates between the two root compartments. Five weeks post Rir inoculation, the CMNs between two maize plants were well established (by detecting the mycorrhizal rate of maize roots in the right RC). 15N labeling was conducted every two days by directly injecting the 15N solution into the stem of the maize plant in the right RC (labeled plant). For CMN damage treatment, a steel ruler was inserted into the air gap between two-layer nylon mesh to cut the mycelium back and forth every day. Insect herbivory proceeded on unlabeled plants was simulated by mechanical wounding and oral secretions (W+OS) of S. frugiperda larvae (Figure S2B). Ten days later, the plants were harvested to measure for dry weight and analyze the 15N content.
Oral secretions (OS) were collected from the 5th instar S. frugiperda larvae fed on maize seedlings (Figure S2A). The larval mouth was touched with a 0.1-10 µL pipette tip to stimulate the larvae to spit out oral secretions (Figure S2B). The oral secretions of the larvae were quickly pipetted and collected in pre-chilled 1.5 mL EP tubes (Figure S2C) and centrifuged at 12,000 rpm for 10 min at 4°C to remove food debris. The oral secretions were finally diluted 5-fold with ddH2O before use (Figure S2D). For wounding and OS-elicitation treatment (W+OS), maize seedlings with the same growth phenotype were selected for the W+OS treatment. A barbed roller dipped with the OS was used to pierce each leaf of the maize plants to imitate the herbivorous insect (Figure S2E). The number of piercing holes was determined according to the size of the leaf, generally 50–100 holes/leaf (Figure S2F). The treatment was repeated every three days, three times during the test period, and the samples were collected after 10 days of treatment.
Ammonium sulfate-15N2, ((15NH4)2SO4; CAS: 43086-58-4; abundance: 99 atom %, chemical purity: ≥ 98.5%; Shanghai Stable Isotope Engineering Technology Research Center) was used for 15N labeling. 15N labeling in maize plants was performed after the plants had grown to the V6 or 6-leaf stage to the V10 or 10-leaf stage. In the experiment, maize plants were labeled with 15N by the stem injection method. (15NH4)2SO4 (0.118 g) was dissolved in 10 mL ddH2O to prepare 15N labeling solution. Using a micro-syringe, the 15N labeling solution was injected into the main stem of maize plants. Each plant received 10 µL 15N labeling solution every two days, continuously five times. For the unlabeled plant in the same apparatus, an equal amount of unlabeled nitrogen was injected.
The stable isotopes of 15N were analyzed and determined using a trace gas pre-concentration system-stable isotope ratio mass spectrometer (UK, model: Isoprime100). The determination process was completed at the Forest Ecology-Stable Isotope Research Center, School of Forestry, Fujian Agriculture and Forestry University. 15N abundance and accumulation were as calculated as follows:
Maize plant shoots and roots of experiment I−IV were separately harvested. After washing, the roots were dried on a paper towel, and one half were immediately frozen in liquid nitrogen RNA extraction. The rest of roots and the shoots were oven-dried to constant weight at 60°C. Totally twelve plants were harvested in each treatment group. For the determination of the mycorrhizal infection rate from two independent root samples were mixed into one biological replicate resulting in six biological replicates. For the measurements of 15N accumulation, four independent plant samples were combined into one biological sample resulting in three biological replicates.
The staining method was slightly modified from Song et al. (Song et al., 2019). The fresh maize root segments were placed in a centrifuge tube containing 10% KOH and heated at 96°C for 5 min. The roots were washed with sterile water three times to remove the remaining KOH and placed in a centrifuge tube containing 2% HCl at 96°C for 5 min. After washing several times with 0.2 M PBS (pH = 7.4), the roots were re-immersed in PBS and placed at room temperature for 3 h. The roots were then transferred to PBS containing 5 µg/mL WGA-488 (wheat germ agglutinin-Alexa fluor 488 conjugate, Invitrogen, W11261) and stained overnight at 4°C. The stained root segments were washed several times with PBS and then placed in 0.2 M PBS containing 10 µg/mL PI for 1 h at room temperature, followed by washing with PBS. Stained root segments were observed under a Nikon Eclipse Ti2 inverted fluorescence microscope (Shanghai Nikon Instruments Co., Ltd., China), with an excitation wavelength of 488 nm and an emission wavelength of 507 nm, and photographed with a monochrome microscope camera (Nikon DS-Ri2). The mycorrhizal colonization rate was calculated as described by Biermann et al. (Biermann and Linderman, 1981).
Forty days post Rir inoculation, the mycorrhizal mycelial network was established. The plant vertical distance from the stem base of the plant to the highest point in the natural state of the plant was measured as the natural height of maize plants. The fresh plants were harvested, and then placed into an oven with 60°C constant temperature to constant weight.
The phosphorus content was determined using Bao’s method (Bao, 2000), with slight modifications. Maize plants were harvested and cut into small pieces (0-2 mm) with scissors, and then were ground into powder. The powder (0.100 g) was placed into a digestion tube (Denmark, FOSS), and 5 mL of HNO3 were added to digest the sample at room temperature for 12 h, followed by further digestion at 180°C for 30 min on the digestion furnace. The digested solution was made up to 50 mL, shaken, mixed, filtered, and centrifuged at 4000 rpm for 5 min; 10 μL of the supernatant was added to the molybdenum antimony anti-staining agent and incubated at 42°C for 20 min. The absorbance was detected at OD820nm.
The Kjeldahl method, with slight modifications, was used to determine the nitrogen content (Bao, 2000). For H2SO4-H2O2 digestion, 0.100 g of the plant powder sample was placed in a digestive tube (provided with the FOSS Kjeldahl nitrogen analyzer), and 2 g catalyst (Cu2SO4: KCl=9:1, m/m) and 5 mL of concentrated sulfuric acid were added. The sample was then digested at 400°C for about 60 min. During digestion, drops of 300 g/L H2O2 were added (after cooling the digestion tube) until the digestion liquid became colorless or clear. The total nitrogen content in the digested plant samples was determined with a FOSS automatic Kjeldahl nitrogen analyzer (Denmark, model: Kjeltec 8400). The detailed procedure was performed according to the instrument manual for the operation process.
Procedures used for RNA extraction and reverse transcription of plant samples were carried out as previously described (Lin et al., 2019), with slight modifications. Total RNA was extracted from ~0.1 g flash-frozen, powdered root samples using the Eastep® Super Total RNA Extraction kit (Promega Biotech Co., Ltd., China) according to the manufacturer’s instructions. Total RNA was treated with RNase-Free DNaseI (TIANGEN Biotech Co., Ltd., China), and 1 μg of total RNA was pipetted for cDNA synthesis using the GoScript Reverse Transcription System (Promega Biotech Co., Ltd., China). Real-time PCR was performed using the MonAmp ChemoHS qPCR Mix (High Rox) Kit (Monad Biotech Co., Ltd., China). Reaction conditions for thermal cycling were 95°C for 5 min, followed by 40 cycles of 95°C for 10 s, 55–65°C for 10 s, and 72°C for 30 s. Fluorescence data were collected during the cycle at 72°C. The gene expression level was normalized using the maize housekeeping gene GAPDH and the 2-ΔΔCT method. The gene-specific primers used in this research are listed in Table S1. Biological triplicates with technical duplicates were performed.
Data were processed and plotted using Microsoft Excel 2013 and GraphPad Prism 9 software, and significance was tested using SPSS 19. All experiments were conducted using a completely randomized experimental design. Data were checked for normality (P > 0.05) using the Shapiro-Wilk normality test and Levene’s test for homogeneity of variance (P > 0.05) prior to all statistical analyses. On the premise of satisfying the assumption of normality and homogeneity of variance, a Student’s t-test or analysis of variance (ANOVA) (Tukey’s post hoc test, P< 0.05) were used to compare the differences between two or more treatments. When the assumptions of normality and variance homogeneity were not met, the Mann-Whitney U test or the Kruskal–Wallis test were used to analyze the data of two or more groups, and then Fisher’s least significant difference (LSD) and the Holm correction adjusted for P values were used to compare significant differences.
The original contributions presented in the study are included in the article/Supplementary Material. Further inquiries can be directed to the corresponding authors.
YL, YS, DC and RZ conceived and designed the experiments, and wrote the manuscript. CH, YL, LT, YZ, YD, MY and QL carried out the experiments. YS, CH., YL. and RZ analyzed the data and contributed to the discussion and revision. All authors contributed to the article and approved the submitted version.
This research was funded by the National Natural Science Foundation of China (31971833, U2005208) and Natural Science Foundation of Fujian Province (2019J01375, 2021J02024).
We thank Prof. Jintian Lin of Zhongkai University of Agriculture and Engineering (China) for kindly providing us with the original insect source of Spodoptera frugiperda.
The authors declare that the research was conducted in the absence of any commercial or financial relationships that could be construed as a potential conflict of interest.
All claims expressed in this article are solely those of the authors and do not necessarily represent those of their affiliated organizations, or those of the publisher, the editors and the reviewers. Any product that may be evaluated in this article, or claim that may be made by its manufacturer, is not guaranteed or endorsed by the publisher.
The Supplementary Material for this article can be found online at: https://www.frontiersin.org/articles/10.3389/fpls.2022.1080416/full#supplementary-material
Alaux, P.-L., Naveau, F., Declerck, S., Cranenbrouck, S. (2020). Common mycorrhizal network induced JA/ET genes expression in healthy potato plants connected to potato plants infected by phytophthora infestans. Front. Plant Sci. 11. doi: 10.3389/fpls.2020.00602
Almario, J., Fabiańska, I., Saridis, G., Bucher, M. (2022). Unearthing the plant–microbe quid pro quo in root associations with beneficial fungi. New Phytol. 234 (6), 1967–1976. doi: 10.1111/nph.18061
An, J., Zeng, T., Ji, C., de Graaf, S., Zheng, Z., Xiao, T. T., et al. (2019). A medicago truncatula SWEET transporter implicated in arbuscule maintenance during arbuscular mycorrhizal symbiosis. New Phytol. 224 (1), 396–408. doi: 10.1111/nph.15975
Babikova, Z., Gilbert, L., Bruce, T. J., Birkett, M., Caulfield, J. C., Woodcock, C., et al. (2013). Underground signals carried through common mycelial networks warn neighbouring plants of aphid attack. Ecol. Lett. 16 (7), 835–843. doi: 10.1111/ele.12115
Babikova, Z., Johnson, D., Bruce, T., Pickett, J., Gilbert, L. (2013). How rapid is aphid-induced signal transfer between plants via common mycelial networks? Commun. Integr. Biol. 6 (6), 835–843. doi: 10.4161/cib.25904
Barto, E. K., Rillig, M. C. (2010). Does herbivory really suppress mycorrhiza? a meta-analysis. J. Ecol. 98 (4), 745–753. doi: 10.1111/j.1365-2745.2010.01658.x
Biermann, B., Linderman, R. (1981). Quantifying vesicular-arbuscular mycorrhizae: a proposed method towards standardization. New Phytol. 87 (1), 63–67. doi: 10.1111/j.1469-8137.1981.tb01690.x
Boldt, K., Pörs, Y., Haupt, B., Bitterlich, M., Kühn, C., Grimm, B., et al. (2011). Photochemical processes, carbon assimilation and RNA accumulation of sucrose transporter genes in tomato arbuscular mycorrhiza. J. Plant Physiol. 168 (11), 1256–1263. doi: 10.1016/j.jplph.2011.01.026
Bücking, H., Mensah, J. A., Fellbaum, C. R. (2016). Common mycorrhizal networks and their effect on the bargaining power of the fungal partner in the arbuscular mycorrhizal symbiosis. Commun. Integr. Biol. 9 (1), e1107684. doi: 10.1080/19420889.2015.1107684
Cabral, C., Wollenweber, B., António, C., Ravnskov, S. (2019). Activity in the arbuscular mycorrhizal hyphosphere warning neighbouring plants. Front. Plant Sci. 10. doi: 10.3389/fpls.2019.00511
Charters, M. D., Durant, E. K., Sait, S. M., Field, K. J. (2022). Impacts of aphid herbivory on mycorrhizal growth responses across three cultivars of wheat. Plants. People. Planet 4 (6), 655–666. doi: 10.1002/ppp3.10302
Charters, M. D., Sait, S. M., Field, K. J. (2020). Aphid herbivory drives asymmetry in carbon for nutrient exchange between plants and an arbuscular mycorrhizal fungus. Curr. Biol. 30 (10), 1801–1808. e5. doi: 10.1016/j.cub.2020.02.087
Choi, J., Summers, W., Paszkowski, U. (2018). Mechanisms underlying establishment of arbuscular mycorrhizal symbioses. Annu. Rev. Phytopathol. 56, 135–160. doi: 10.1146/annurev-phyto-080516-035521
Cliquet, J. B., Murray, P. J., Boucaud, J. (1997). Effect of the arbuscular mycorrhizal fungus glomus fasciculatum on the uptake of amino nitrogen by lolium perenne. New Phytol. 137 (2), 345–349. doi: 10.1046/j.1469-8137.1997.00810.x
Crawford, N. M., Glass, A. D. (1998). Molecular and physiological aspects of nitrate uptake in plants. Trends Plant Sci. 3 (10), 389–395. doi: 10.1016/S1360-1385(98)01311-9
Etesami, H., Adl, S. M. (2020). Plant growth-promoting rhizobacteria (PGPR) and their action mechanisms in availability of nutrients to plants. In: Kumar, M., Kumar, V., Prasad, R. editors, Phyto-Microbiome. Stress Regul. Environmental and Microbial Biotechnology. (Singapore: Springer). 147–203. doi: 10.1007/978-981-15-2576-6_9
Ferlian, O., Biere, A., Bonfante, P., Buscot, F., Eisenhauer, N., Fernandez, I., et al. (2018). Growing research networks on mycorrhizae for mutual benefits. Trends Plant Sci. 23 (11), 975–984. doi: 10.1016/j.tplants.2018.08.008
Frew, A. (2021). Aboveground herbivory suppresses the arbuscular mycorrhizal symbiosis, reducing plant phosphorus uptake. Appl. Soil Ecol. 168, 104133. doi: 10.1016/j.apsoil.2021.104133
Frew, A., Price, J. N. (2019). Mycorrhizal-mediated plant–herbivore interactions in a high CO2 world. Funct. Ecol. 33 (8), 1376–1385. doi: 10.1111/1365-2435.13347
Frey, B., Schüepp, H. (1993). Acquisition of nitrogen by external hyphae of arbuscular mycorrhizal fungi associated with zea mays l. New Phytol. 124 (2), 221–230. doi: 10.1111/j.1469-8137.1993.tb03811.x
Gehring, C. A., Whitham, T. G. (1994). Interactions between aboveground herbivores and the mycorrhizal mutualists of plants. Trends Ecol. Evol. 9 (7), 251–255. doi: 10.1016/0169-5347(94)90290-9
Ge, L., Sun, S., Chen, A., Kapulnik, Y., Xu, G. (2008). Tomato sugar transporter genes associated with mycorrhiza and phosphate. Plant Growth Regul. 55 (2), 115–123. doi: 10.1007/s10725-008-9266-7
Giri, A. P., Wunsche, H., Mitra, S., Zavala, J. A., Muck, A., Svatosš, A., et al. (2006). Molecular interactions between the specialist herbivore manduca sexta (Lepidoptera, sphingidae) and its natural host Nicotiana attenuata. VII. changes in the plant's proteome. Plant Physiol. 142 (4), 1621–1641. doi: 10.1104/pp.106.088781
Govindarajulu, M., Pfeffer, P. E., Jin, H., Abubaker, J., Douds, D. D., Allen, J. W., et al. (2005). Nitrogen transfer in the arbuscular mycorrhizal symbiosis. Nature 435 (7043), 819–823. doi: 10.1038/nature03610
Hazard, C., Johnson, D. (2018). Does genotypic and species diversity of mycorrhizal plants and fungi affect ecosystem function? New Phytol. 220 (4), 1122–1128. doi: 10.1111/nph.15010
He, X.-H., Critchley, C., Bledsoe, C. (2003). Nitrogen transfer within and between plants through common mycorrhizal networks (CMNs). Crit. Rev. Plant Sci. 22 (6), 531–567. doi: 10.1080/713608315
He, X., Critchley, C., Ng, H., Bledsoe, C., Reciprocal, N. (2004). (15 or 15) transfer between nonN2-fixing eucalyptus maculata and N2-fixing Casuarina cunninghamiana linked by the ectomycorrhizal fungus Pisolithus sp. New Phytol. 163 (3), 629–640. doi: 10.1111/j.1469-8137.2004.01137.x
He, X., Xu, M., Qiu, G. Y., Zhou, J. (2009). Use of 15N stable isotope to quantify nitrogen transfer between mycorrhizal plants. J. Plant Ecol. 2 (3), 107–118. doi: 10.1038/s41598-021-90436-8
Hodge, A., Storer, K. (2015). Arbuscular mycorrhiza and nitrogen: Implications for individual plants through to ecosystems. Plant Soil 386 (1), 1–19. doi: 10.1007/s11104-014-2162-1
Hui, J., An, X., Li, Z., Neuhäuser, B., Ludewig, U., Wu, X., et al. (2022). The mycorrhiza-specific ammonium transporter ZmAMT3; 1 mediates mycorrhiza-dependent nitrogen uptake in maize roots. Plant Cell 34 (10), 4066–4087. doi: 10.1093/plcell/koac225
Jaiswal, S. K., Mohammed, M., Ibny, F. Y., Dakora, F. D. (2021). Rhizobia as a source of plant growth-promoting molecules: Potential applications and possible operational mechanisms. Front. Sustain. Food Syst. 4. doi: 10.3389/fsufs.2020.619676
Jiang, Y., Wang, W., Xie, Q., Liu, N., Liu, L., Wang, D., et al. (2017). Plants transfer lipids to sustain colonization by mutualistic mycorrhizal and parasitic fungi. Science 356 (6343), 1172–1175. doi: 10.1126/science.aam9970
Johansen, A., Jensen, E. (1996). Transfer of n and p from intact or decomposing roots of pea to barley interconnected by an arbuscular mycorrhizal fungus. Soil Biol. Biochem. 28 (1), 73–81. doi: 10.1016/0038-0717(95)00117-4
Kennedy, P., Walker, J., Bogar, L., Horton, T. (2015). Interspecific mycorrhizal networks and non-networking hosts: Exploring the ecology of the host genus Alnus. (Springer Netherlands Dordrecht), 227–254. Available at: https://www.springer.com/gp/book/9789401773942.
Kerchev, P. I., Fenton, B., Foyer, C. H., Hancock, R. D. (2012). Plant responses to insect herbivory: interactions between photosynthesis, reactive oxygen species and hormonal signalling pathways. Plant. Cell Environ. 35 (2), 441–453. doi: 10.1111/j.1365-3040.2011.02399.x
Kobae, Y., Tamura, Y., Takai, S., Banba, M., Hata, S. (2010). Localized expression of arbuscular mycorrhiza-inducible ammonium transporters in soybean. Plant Cell Physiol. 51 (9), 1411–1415. doi: 10.1093/pcp/pcq099
Koegel, S., Ait Lahmidi, N., Arnould, C., Chatagnier, O., Walder, F., Ineichen, K., et al. (2013). The family of ammonium transporters (AMT) in s orghum bicolor: Two AMT members are induced locally, but not systemically in roots colonized by arbuscular mycorrhizal fungi. New Phytol. 198 (3), 853–865. doi: 10.1111/nph.12199
Lin, Y., Sun, Z., Li, Z., Xue, R., Cui, W., Sun, S., et al. (2019). Deficiency in silicon transporter Lsi1 compromises inducibility of anti-herbivore defense in rice plants. Front. Plant Sci. 10. doi: 10.3389/fpls.2019.00652
Ma, X., Geng, Q., Zhang, H., Bian, C., Chen, H. Y., Jiang, D., et al. (2021). Global negative effects of nutrient enrichment on arbuscular mycorrhizal fungi, plant diversity and ecosystem multifunctionality. New Phytol. 229 (5), 2957–2969. doi: 10.1111/nph.17077
Merckx, V. S., Gomes, S. I. (2020). Symbiosis: Herbivory alters mycorrhizal nutrient exchange. Curr. Biol. 30 (10), R437–R439. doi: 10.1016/j.cub.2020.04.016
Moulin, S. (2022). Get connected to the fungal network for improved transfer of nitrogen: the role of ZmAMT3; 1 in ammonium transport in maize-arbuscular mycorrhizal symbiosis. Plant Cell 34, 3509–3511. doi: 10.1093/plcell/koac221
Nabity, P. D., Zavala, J. A., DeLucia, E. H. (2009). Indirect suppression of photosynthesis on individual leaves by arthropod herbivory. Ann. Bot. 103 (4), 655–663. doi: 10.1093/aob/mcn127
Nabity, P. D., Zavala, J. A., DeLucia, E. H. (2013). Herbivore induction of jasmonic acid and chemical defences reduce photosynthesis in nicotiana attenuata. J. Exp. Bot. 64 (2), 685–694. doi: 10.1093/jxb/ers364
Oelmüller, R. (2019). Interplant communication via hyphal networks. Plant Physiol. Rep. 24 (4), 463–473. doi: 10.1111/nph.13115
Oerke, E.-C. (2006). Crop losses to pests. J. Agric. Sci. 144 (1), 31–43. doi: 10.1017/S0021859605005708
Paredes-Sánchez, F. A., Rivera, G., Bocanegra-García, V., Martínez-Padrón, H. Y., Berrones-Morales, M., Niño-García, N., et al. (2021). Advances in control strategies against spodoptera frugiperda. A review. Molecules 26 (18), 5587. doi: 10.3390/molecules26185587
Pérez-Tienda, J., Testillano, P. S., Balestrini, R., Fiorilli, V., Azcón-Aguilar, C., Ferrol, N. (2011). GintAMT2, a new member of the ammonium transporter family in the arbuscular mycorrhizal fungus glomus intraradices. Fungal Genet. Biol. 48 (11), 1044–1055. doi: 10.1016/j.fgb.2011.08.003
Pozo, M. J., López-Ráez, J. A., Azcón-Aguilar, C., García-Garrido, J. M. (2015). Phytohormones as integrators of environmental signals in the regulation of mycorrhizal symbioses. New Phytol. 205 (4), 1431–1436. doi: 10.1111/nph.13252
Prasanna, B. M., Bruce, A., Beyene, Y., Makumbi, D., Gowda, M., Asim, M., et al. (2022). Host plant resistance for fall armyworm management in maize: Relevance, status and prospects in Africa and Asia. Theor. Appl. Genet. 135, 1–20. doi: 10.1007/s00122-022-04073-4
Rillig, M. C., Aguilar-Trigueros, C. A., Camenzind, T., Cavagnaro, T. R., Degrune, F., Hohmann, P., et al. (2019). Why farmers should manage the arbuscular mycorrhizal symbiosis. New Phytol. 222 (3), 1171–1175. doi: 10.1111/nph.15602
Ruzicka, D. R., Hausmann, N. T., Barrios-Masias, F. H., Jackson, L. E., Schachtman, D. P. (2012). Transcriptomic and metabolic responses of mycorrhizal roots to nitrogen patches under field conditions. Plant Soil 350 (1), 145–162. doi: 10.1007/s11104-011-0890-z
Smith, S. E., Smith, F. A. (2011). Roles of arbuscular mycorrhizas in plant nutrition and growth: New paradigms from cellular to ecosystem scales. Annu. Rev. Plant Biol. 62, 227–250. doi: 10.1146/annurev-arplant-042110-103846
Smith, S. E., Smith, F. A., Jakobsen, I. (2003). Mycorrhizal fungi can dominate phosphate supply to plants irrespective of growth responses. Plant Physiol. 133 (1), 16–20. doi: 10.1104/pp.103.024380
Smith, S. E., Smith, F. A., Jakobsen, I. (2004). Functional diversity in arbuscular mycorrhizal (AM) symbioses: the contribution of the mycorrhizal p uptake pathway is not correlated with mycorrhizal responses in growth or total p uptake. New Phytol. 162 (2), 511–524. doi: 10.1111/j.1469-8137.2004.01039.x
Song, Y., Wang, M., Zeng, R., Groten, K., Baldwin, I. T. (2019). Priming and filtering of antiherbivore defences among nicotiana attenuata plants connected by mycorrhizal networks. Plant. Cell Environ. 42 (11), 2945–2961. doi: 10.1111/pce.13626
Song, Y. Y., Zeng, R. S., Xu, J. F., Li, J., Shen, X., Yihdego, W. G. (2010). Interplant communication of tomato plants through underground common mycorrhizal networks. PloS One 5 (10), e13324. doi: 10.1371/journal.pone.0013324
Sprent, J. (2005). West African Legumes: the role of nodulation and nitrogen fixation. New Phytol. 168 (1), 326–330. doi: 10.1111/j.1469-8137.2005.01499.x
Thirkell, T. J., Charters, M. D., Elliott, A. J., Sait, S. M., Field, K. J. (2017). Are mycorrhizal fungi our sustainable saviours? Considerations for achieving food security. J. Ecol. 105 (4), 921–929. doi: 10.1111/1365-2745.12788
Tobar, R., Azcón, R., Barea, J. (1994). Improved nitrogen uptake and transport from 15N-labelled nitrate by external hyphae of arbuscular mycorrhiza under water-stressed conditions. New Phytol. 126 (1), 119–122. doi: 10.1111/j.1469-8137.1994.tb07536.x
Verzeaux, J., Hirel, B., Dubois, F., Lea, P. J., Tétu, T. (2017). Agricultural practices to improve nitrogen use efficiency through the use of arbuscular mycorrhizae: Basic and agronomic aspects. Plant Sci. 264, 48–56. doi: 10.1016/j.plantsci.2017.08.004
Wahbi, S., Maghraoui, T., Hafidi, M., Sanguin, H., Oufdou, K., Prin, Y., et al. (2016). Enhanced transfer of biologically fixed n from faba bean to intercropped wheat through mycorrhizal symbiosis. Appl. Soil Ecol. 107, 91–98. doi: 10.1016/j.apsoil.2016.05.008
Wang, S., Chen, A., Xie, K., Yang, X., Luo, Z., Chen, J., et al. (2020). Functional analysis of the OsNPF4.5 nitrate transporter reveals a conserved mycorrhizal pathway of nitrogen acquisition in plants. Proc. Natl. Acad. Sci. United. States America 117 (28), 16649–16659. doi: 10.1073/pnas.2000926117
Wang, G., Sheng, L., Zhao, D., Sheng, J., Wang, X., Liao, H. (2016). Allocation of nitrogen and carbon is regulated by nodulation and mycorrhizal networks in soybean/maize intercropping system. Front. Plant Sci. 7. doi: 10.3389/fpls.2016.01901
Wang, W., Shi, J., Xie, Q., Jiang, Y., Yu, N., Wang, E. (2017). Nutrient exchange and regulation in arbuscular mycorrhizal symbiosis. Mol. Plant 10 (9), 1147–1158. doi: 10.1016/j.molp.2017.07.012
Whiteside, M. D., Werner, G. D., Caldas, V. E., van’t Padje, A., Dupin, S. E., Elbers, B., et al. (2019). Mycorrhizal fungi respond to resource inequality by moving phosphorus from rich to poor patches across networks. Curr. Biol. 29 (12), 2043–2050. e8. doi: 10.1016/j.cub.2019.04.061
Wipf, D., Krajinski, F., van Tuinen, D., Recorbet, G., Courty, P. E. (2019). Trading on the arbuscular mycorrhiza market: From arbuscules to common mycorrhizal networks. New Phytol. 223 (3), 1127–1142. doi: 10.1111/nph.15775
Xiao, H., Ye, X., Xu, H., Mei, Y., Yang, Y., Chen, X., et al. (2020). The genetic adaptations of fall armyworm spodoptera frugiperda facilitated its rapid global dispersal and invasion. Mol. Ecol. Resour. 20 (4), 1050–1068. doi: 10.1111/1755-0998.13182
Yang, S.-Y., Grønlund, M., Jakobsen, I., Grotemeyer, M. S., Rentsch, D., Miyao, A., et al. (2012). Nonredundant regulation of rice arbuscular mycorrhizal symbiosis by two members of the PHOSPHATE TRANSPORTER1 gene family. Plant Cell 24 (10), 4236–4251. doi: 10.1105/tpc.112.104901
Yu, P., Wang, C., Baldauf, J. A., Tai, H., Gutjahr, C., Hochholdinger, F., et al. (2018). Root type and soil phosphate determine the taxonomic landscape of colonizing fungi and the transcriptome of field-grown maize roots. New Phytol. 217 (3), 1240–1253. doi: 10.1111/nph.14893
Zhang, H., Wang, X., Gao, Y., Sun, B. (2020). Short-term n transfer from alfalfa to maize is dependent more on arbuscular mycorrhizal fungi than root exudates in n deficient soil. Plant Soil 446 (1), 23–41. doi: 10.1007/s11104-019-04333-1
Zhang, Y. C., Zou, Y. N., Liu, L. P., Wu, Q. S. (2019). Common mycorrhizal networks activate salicylic acid defense responses of trifoliate orange (Poncirus trifoliata). J. Integr. Plant Biol. 61 (10), 1099–1111. doi: 10.1111/jipb.12743
Keywords: mycorrhiza, nitrogen, Zea mays, common mycorrhizal networks, insect herbivory
Citation: He C, Lin Y, Zhang Y, Tong L, Ding Y, Yao M, Liu Q, Zeng R, Chen D and Song Y (2022) Aboveground herbivory does not affect mycorrhiza-dependent nitrogen acquisition from soil but inhibits mycorrhizal network-mediated nitrogen interplant transfer in maize. Front. Plant Sci. 13:1080416. doi: 10.3389/fpls.2022.1080416
Received: 26 October 2022; Accepted: 29 November 2022;
Published: 14 December 2022.
Edited by:
Qian-Hua Shen, Institute of Genetics and Developmental Biology, Chinese Academy of Sciences (CAS), ChinaReviewed by:
Suhua Li, Agricultural Genomics Institute at Shenzhen, Chinese Academy of Agricultural Sciences (CAAS), ChinaCopyright © 2022 He, Lin, Zhang, Tong, Ding, Yao, Liu, Zeng, Chen and Song. This is an open-access article distributed under the terms of the Creative Commons Attribution License (CC BY). The use, distribution or reproduction in other forums is permitted, provided the original author(s) and the copyright owner(s) are credited and that the original publication in this journal is cited, in accordance with accepted academic practice. No use, distribution or reproduction is permitted which does not comply with these terms.
*Correspondence: Yuanyuan Song, eXl1YW5zb25nQDE2My5jb20=; Dongmei Chen, ZG9uZ21laWZqQDE2My5jb20=
Disclaimer: All claims expressed in this article are solely those of the authors and do not necessarily represent those of their affiliated organizations, or those of the publisher, the editors and the reviewers. Any product that may be evaluated in this article or claim that may be made by its manufacturer is not guaranteed or endorsed by the publisher.
Research integrity at Frontiers
Learn more about the work of our research integrity team to safeguard the quality of each article we publish.