- 1Department of Plant and Environmental Sciences, University of Copenhagen, Frederiksberg, Denmark
- 2Department of Accelerated Taxonomy, Royal Botanic Gardens, Kew, Richmond, Surrey, United Kingdom
- 3School of Natural and Environmental Sciences, Newcastle University, Newcastle Upon Tyne, United Kingdom
- 4School of Life Sciences, University of Essex, Colchester, United Kingdom
- 5Institute of Plant Genetics and Biotechnology, Slovak Academy of Sciences, Plant Science and Biodiversity Center, Nitra, Slovakia
Succulence is an adaptation to low water availability characterised by the presence of water-storage tissues that alleviate water stress under low water availability. The succulent syndrome has evolved convergently in over 80 plant families and is associated with anatomical, physiological and biochemical traits. Despite the alleged importance of cell wall traits in drought responses, their significance in the succulent syndrome has long been overlooked. Here, by analyzing published pressure–volume curves, we show that elastic adjustment, whereby plants change cell wall elasticity, is uniquely beneficial to succulents for avoiding turgor loss. In addition, we used comprehensive microarray polymer profiling (CoMPP) to assess the biochemical composition of cell walls in leaves. Across phylogenetically diverse species, we uncover several differences in cell wall biochemistry between succulent and non-succulent leaves, pointing to the existence of a ‘succulent glycome’. We also highlight the glycomic diversity among succulent plants, with some glycomic features being restricted to certain succulent lineages. In conclusion, we suggest that cell wall biomechanics and biochemistry should be considered among the characteristic traits that make up the succulent syndrome.
Introduction
Climate change-induced aridity is expected to increase across much of the globe in the future (Sheffield and Wood, 2008; Jiao et al., 2021). Consequently, it has become imperative that we understand the ways in which plants cope with drought (Choat et al., 2018; Trueba et al., 2019). Recently, plant scientists have begun to pay renewed attention to the drought adaptations found in succulent plants (Heyduk et al., 2016; Males, 2017; Fradera-Soler et al., 2021; Leverett et al., 2021). Succulence is defined by the presence of water stores, in the leaf, stem and/or roots, which can be mobilized when a plant is dehydrated (Ogburn and Edwards, 2010). Typically, succulent tissues (i.e. the tissues responsible for water storage) arise due to the development of enlarged cells, either in the photosynthetic tissue (chlorenchyma), in a specialized achlorophyllous water-storage tissue (hydrenchyma), or a combination of the two (Eggli and Nyffeler, 2009; Borland et al., 2018; Heyduk, 2021; Leverett et al., 2022). If water stored in large cells can be mobilized during drought, succulent plants can dehydrate whilst maintaining water potentials (Ψ) at safe, stable levels. By buffering plant Ψ, succulence prevents a number of detrimental processes from occurring, such as the closing of stomata, the buckling of cells and the formation of emboli in the xylem (Brodribb et al., 2016; Vollenweider et al., 2016; Zhang et al., 2016; Henry et al., 2019). The benefits conferred by succulence have resulted in the succulent syndrome being found in plants across the globe, following adaptive radiations into the world’s arid and semi-arid ecosystems (Arakaki et al., 2011).
The adaptive benefits of succulence have recently drawn the attention of synthetic biologists, who have begun to recognize the potential this adaptation could have for food security and bioenergy in a drying world under climate change scenarios (Borland et al., 2009; Grace, 2019). Both modelling and field trials have assessed the value of growing succulent Agave and Opuntia in dry marginal and underused lands (Owen and Griffiths, 2014; Davis et al., 2017; Hartzell et al., 2021; Neupane et al., 2021). Furthermore, progress has been made to synthetically produce succulence in non-succulent species. The introduction of an exogenous transcription factor gene into Arabidopsis thaliana led to increased tissue succulence and higher water-use efficiency (Lim et al., 2018; Lim et al., 2020). These findings strongly suggest that bioengineering succulence has the potential to enhance drought resistance in crops. Whilst some work has been done to understand the genetic programs controlling the development of succulence (Heyduk, 2021), a great deal more research is needed if we are to fully utilize this adaptation in agricultural settings. In addition, we must appreciate every important trait that makes up the succulent syndrome. Beyond the genetic control of cell size, succulent species often exhibit a number of other co-adaptive traits, such as 3D vascular patterning, crassulacean acid metabolism (CAM) and waxy cuticles (Griffiths and Males, 2017). Cell walls have recently been postulated as an often-overlooked key component of the succulent syndrome (Ahl et al., 2019; Fradera-Soler et al., 2022), yet the precise mechanistic relevance of cell walls in succulent tissues remains largely speculative. In the present study, we analyse cell wall biomechanics and biochemistry in diverse succulent species and propose that these traits should be considered among the characteristic components of the succulent syndrome.
Cell wall biomechanics in succulents
All plant cells are encased in a lattice-like structure, the cell wall (Popper et al., 2011). Primary, extensible cell walls are complex and dynamic systems composed largely of polysaccharides, polyphenols and certain types of glycoproteins (Carpita et al., 2015). When plant cells are hydrated, an osmotic gradient exists across the plasma membrane which results in water moving into the protoplasm (Beadle et al., 1993). This intake of water causes the plasma membrane to push against the cell wall, generating a positive pressure called turgor (P). The bulk modulus of cell wall elasticity (ε) relates to P according to the equation:
where relative water content (RWC) is the percentage of total water present in a tissue. Higher values of ε indicate greater cell wall rigidity and thus more resistance for the plasma membrane to push against, with changes in RWC resulting in large changes in P. Conversely, when ε is low and cell walls are highly elastic, changes to RWC have a lower impact on P, because cell walls can stretch and provide less resistance.
For succulent plants, ε has the potential to affect the point at which turgor is lost. As plant tissues dehydrate, Ψ falls, which results in a linear drop in P (Beadle et al., 1993). Eventually, Ψ falls to a point where P = 0, meaning there has been a total loss of turgor. When this turgor loss point (TLPΨ) has been reached, leaves will typically wilt and cells will begin to experience damage (Trueba et al., 2019). Consequently, it is beneficial for plants to avoid reaching their TLPΨ (Kunert et al., 2021). Bartlett et al. (2012) found that the TLPΨ can be estimated by:
where πO is the osmotic potential of fully hydrated tissues (a more negative πO corresponds to a higher concentration of osmotically active solutes). Modifying ε or πO are named elastic and osmotic adjustment, respectively, and can be used to alter the TLPΨ in order to allow cells to maintain turgor at more negative water potentials. Lower ε could result in cell walls capable of changing shape and folding as the protoplasm within shrinks (Ahl et al., 2019; Fradera-Soler et al., 2022). This would prevent the catastrophic disruption of the membrane-wall continuum and other forms of irreversible damage due to mechanical stress which occur when the TLPΨ is reached. However, studies of non-succulent species have found that ε is generally so high that changes to this trait are inconsequential for the TLPΨ (Bartlett et al., 2012). Put differently, in non-succulent species, cell walls are quite rigid, which means that even substantial changes to their elastic properties will not affect their TLPΨ. This can be visualized by considering Figure 1A. If πO is held constant and ε is allowed to vary, the TLPΨ can be simulated using Equation 2. This simulation forms a curve, and in non-succulent tissues the true value of ε intersects at the flat portion of the curve. Consequently, the phenotypic space inhabited by non-succulent species is one where changes to ε have no effect on the TLPΨ.
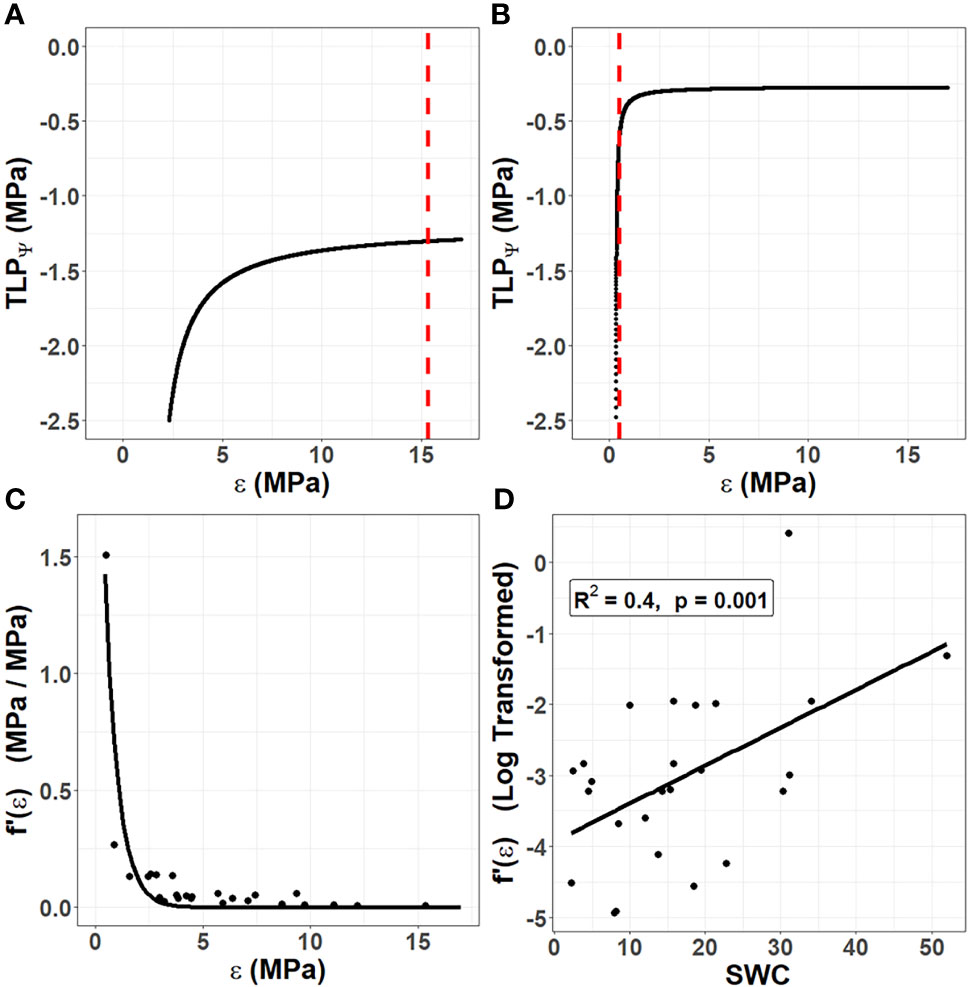
Figure 1 The unique role of cell wall biomechanics in succulent species. Using data from 25 species in the Caryophyllales published by Ogburn and Edwards (2012), the turgor loss point (TLPΨ) was simulated according to Equation 2 by holding the osmotic potential at full hydration (πO) constant for each species and varying the bulk modulus of elasticity (ε). (A) In non-succulent species, such as Calandrinia colchaguensis, the true value of ε (dashed line) intersects at the flat portion of the curve. Hence, changes to ε have little to no effect on the TLPΨ. (B) In some succulent species, such as Grahamia bracteata, the true value of ε intersects at the curved potion of the line, meaning changes to ε affect the TLPΨ. A quantitative estimate of the extent to which changing ε affects the TLPΨ was generated by finding the derivative of the curve at the point where the dashed line intersects [f’(ε)]. (C) Among the 25 species, lower values of ε result in exponentially higher values of f’(ε). An exponential curve still fit these data well when the species with the highest f’(ε) value was removed (data not shown). (D) Among the 25 species, saturated water content (SWC) correlates with the f’(ε), after this value has been log transformed.
The primary cell walls in succulent tissues are generally very thin and elastic (Goldstein et al., 1991; Ogburn and Edwards, 2010). Thus, the true value of ε for succulent species more often falls on the curved portion of the line (Figure 1B). This means that for many succulent tissues, changes to cell wall biomechanics through elastic adjustment would have a much more substantial effect on the TLPΨ than in non-succulent plants. We sought to quantify this effect of ε on TLPΨ by repeating the simulation in Figures 1A, B for several species. Ogburn and Edwards (2012) studied the relationship between parameters derived from pressure–volume curves and measures of succulence in 25 species in the Caryophyllales, an angiosperm order comprising many succulent-rich groups with a broad range of tissue succulence. Using their published data, πO was held constant for each of the 25 species and ε was allowed to vary in order to simulate the TLPΨ according to Equation 2. Then, for each species, we found the derivative of the curve, at the true value of ε (i.e. where the dashed line intersects the curve). This derivative, f’(ε), is a quantitative estimate of the extent to which changing ε affects the TLPΨ. As ε values become very low in highly succulent species, f’(ε) becomes exponentially higher (Figure 1C). Finally, we explored the relationship between f’(ε) and saturated water content (SWC), as the latter has been shown to be a powerful metric to quantify succulence in the Caryophyllales (Ogburn and Edwards, 2012). Log-transformed estimates of f’(ε) correlated significantly with SWC, using a linear regression model (Figure 1D).
Together, our data show that unlike non-succulent species, succulent plants occupy a phenotypic space in which increases in cell wall elasticity during drought (i.e. elastic adjustment) can result in substantial decreases in TLPΨ. Furthermore, once a succulent species moves into this phenotypic space, decreasing ε has an exponential effect on f’(ε), so that alterations to cell wall biomechanics become an increasingly efficient means of controlling the TLPΨ. This agrees with the recently observed drought-induced modifications of pectic polysaccharides in hydrenchyma cell walls of Aloe (Ahl et al., 2019), which are believed to be a form of elastic adjustment that allows them to fold as cells shrink during dehydration.
Cell wall biochemistry in succulents
One way to assess the biochemical composition of cell walls is to investigate the extracellular glycome, which encompasses the entirety of extracellular carbohydrates in a tissue, organ or plant, and the majority of which corresponds to the cell wall. Characterizing glycomic profiles across different plant species can indicate which cell wall components have been favored under different environmental conditions. Whilst the glycomes of some economically important succulent taxa, such as Agave, Aloe and Opuntia, have recently been analyzed (Ginestra et al., 2009; Li et al., 2014; Ahl et al., 2018; Jones et al., 2020), little has been done to compare the cell wall composition of other distantly related succulent species. Hence, we sought to test the hypothesis that the extracellular glycome of phylogenetically diverse succulent species will exhibit some differences from those of non-succulents, so that a common ‘succulent glycome’ emerges. To this end, we sampled leaf material from 10 species with succulent leaves and 10 with non-succulent leaves, representing diverse lineages within the angiosperms (Figure 2A and Table S1). Using the succulence index (SI) from Ogburn and Edwards (2010) as a proxy for the degree of succulence (see Suppl. Methods), these two groups differed significantly (p < 0.01) (Figure 2B and Table S2). We used comprehensive microarray polymer profiling (CoMPP) to estimate and compare the relative polysaccharide contents of leaves from these species (see Suppl. Methods) (Moller et al., 2007; Ahl et al., 2018). We used whole leaves for comparability across species, assuming that mesophyll tissues would dominate the results. In the current study we used three extraction steps: water (targeting soluble unbound or loosely bound polysaccharides), CDTA (targeting primarily pectins) and NaOH (targeting primarily hemicelluloses). CoMPP relies on antibody-based molecular probes, so we used 49 monoclonal antibodies (mAbs) to target the majority of known cell wall polymer motifs (Moller et al., 2007; Rydahl et al., 2018) (Tables S3–S5). No representatives of commelinid monocots were included, given that their type-II cell wall biochemistry is particularly distinct from that of the rest of angiosperms (Carpita et al., 2015). CoMPP results in heatmap format can be found in Suppl. Data.
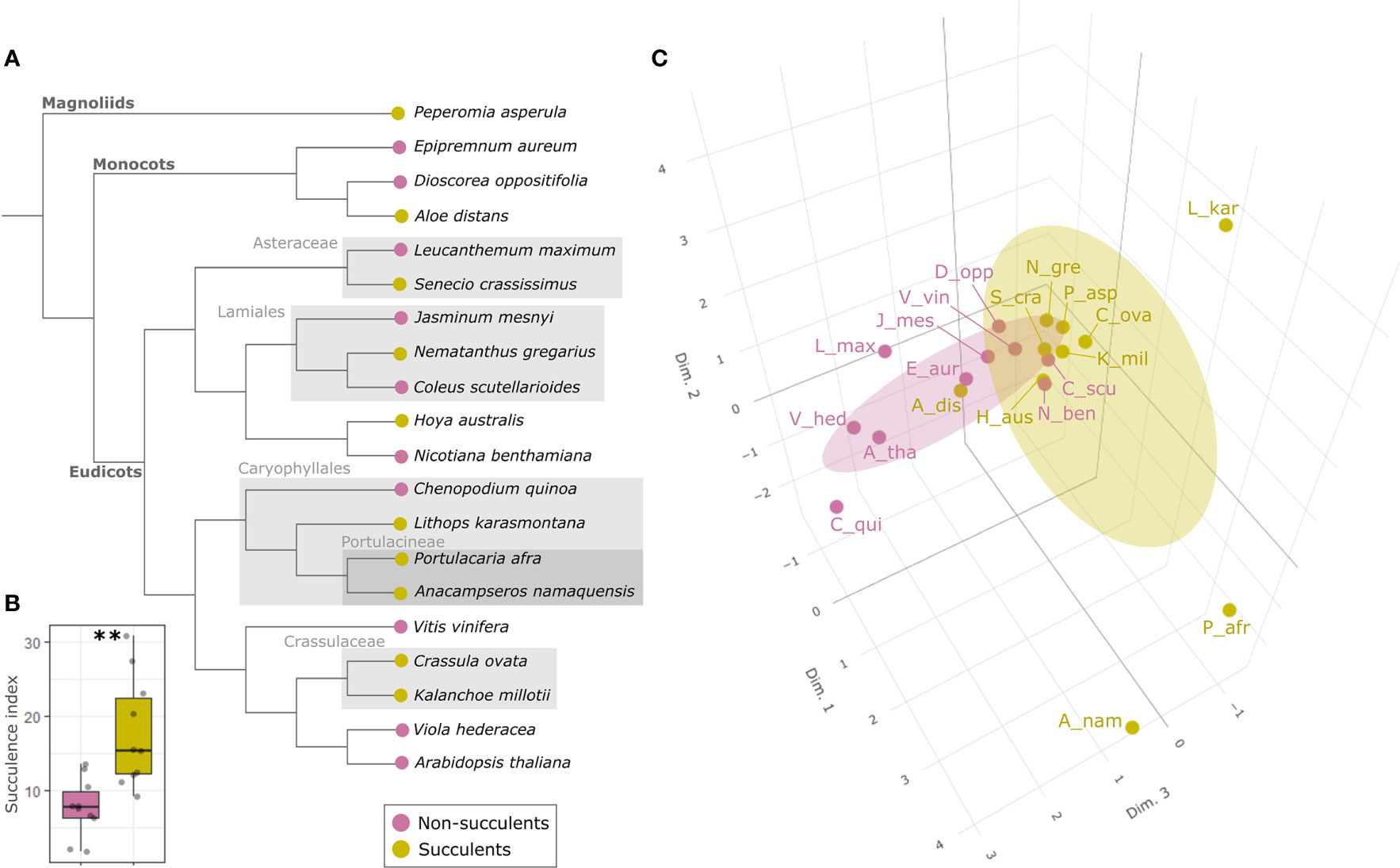
Figure 2 The succulent glycome: succulents and non-succulents occupy different phenotypic spaces in terms of glycomic profiles. (A) Cladogram representing the phylogenetic relationship (according to Baker et al., 2022) among the 20 species included in this study; some major clades are indicated in grey for clarity. (B) Boxplot of succulence index (SI) values for all the species; the two groups differ significantly (**p < 0.01) according to Welch’s t-test. (C) 3D score plot of the first three MFA dimensions (17%, 12.1% and 10.8% of total variance respectively) of glycomic data from 10 leaf succulents and 10 non-succulents (see Table S1 for abbreviations), with concentration ellipsoids for each group. Succulents and non-succulents occupy distinct phenotypic spaces, particularly along dimension 3.
CoMPP results were analyzed using multiple factor analysis (MFA) (see Suppl. Methods), which indicated that succulent species occupy a distinct phenotypic space different from non-succulent species (Figure 2C). Of particular note is MFA dimension 3, along which succulents and non-succulents differed significantly (p < 0.01) and was driven mostly by glycoprotein- and pectin-targeting mAbs (Figure S1). Three succulent species were “pulling” along dimension 1 and fell far from the main cluster, but even when omitting these three outliers from the MFA, the results still showed a significant difference between succulents and non-succulents (Figure S2). We observed a higher signal for rhamnogalacturonan I (RG-I) backbones in succulents compared to non-succulents (Figure 3). RG-I and its side chains (i.e. arabinans, galactans and/or arabinogalactans) have been linked to increased cell wall elasticity (Harholt et al., 2010; Carroll et al., 2022) and have been postulated as cell wall plasticizers, which is a crucial feature for cells undergoing structural wall changes during dehydration and rehydration (Moore et al., 2013). Furthermore, we observed a higher signal for homogalacturonans (HGs) with a high degree of methyl-esterification (DM) in succulents (Figure 3), which may indicate highly elastic cell walls. In contrast, non-succulents had a higher signal for low-DM HGs (Figure 3), which may indicate stiffer cell walls. According to the textbook model, low-DM HGs can cross-link in the presence of Ca2+ and stiffen the cell wall, whereas a high DM prevents the formation of these cross-links and renders the wall more elastic (Willats et al., 2001; Cosgrove, 2005). However, several factors (e.g. pH, Ca2+ availability, different enzymatic activities) can influence the outcome, so caution should be taken when using DM as a proxy for cell wall mechanics (Palin and Geitmann, 2012; Bidhendi and Geitmann, 2016; Chebli and Geitmann, 2017; Hocq et al., 2017). Together, MFA of the CoMPP results suggests that fundamental differences exist between the cell wall composition of diverse succulent and non-succulent species. The three outlying succulent species (Anacampseros namaquensis, Lithops karasmontana and Portulacaria afra) belong to the core Caryophyllales, and two of them (A. namaquensis and P. afra) to suborder Portulacineae. These species showed remarkably high signal for RG-I and its side chains and for glucuronoxylans (Figure 3), which most likely reflects the presence of highly hydrophilic apoplastic mucilage in succulents in the Caryophyllales, particularly those in the Portulacineae (Figure 4) (Cárdenas et al., 1997; Vignon and Gey, 1998; Hernandes-Lopes et al., 2016; Cole, 2022).
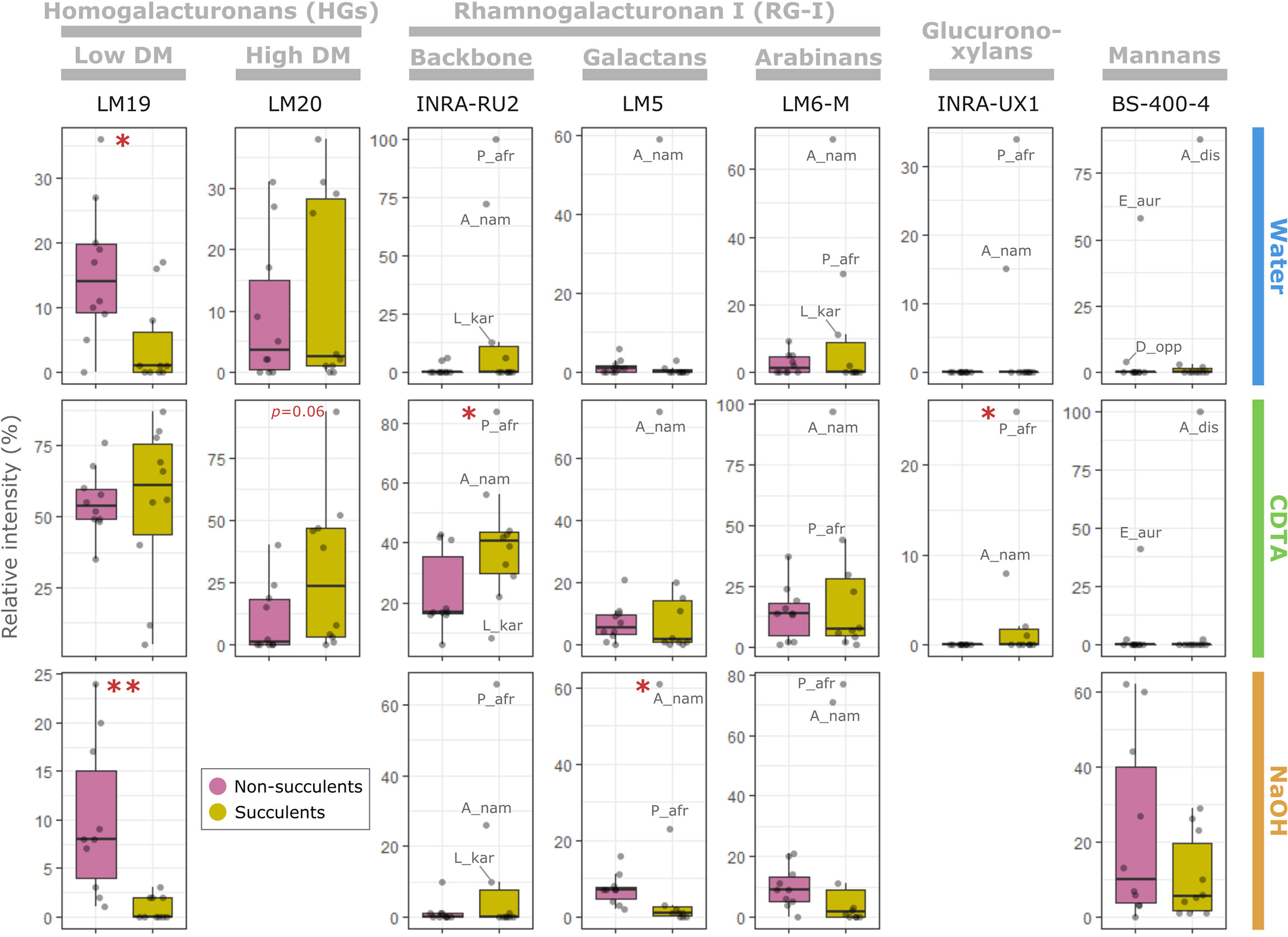
Figure 3 The succulent glycome: main glycomic differences between succulents and non-succulents. Selection of antibodies depicting the main glycomic differences between succulents and non-succulents for pectins (homogalacturonans and rhamnogalacturonan I), glucuronoxylans and mannans (see Suppl. Data for raw data). Each column corresponds to a specific antibody and rows represent the three fractions (i.e. CoMPP extraction steps); the y-axes represent relative intensity of signal within a specific fraction. Some outlying species have been labelled (see Table S1 for abbreviations). Significant differences between the two groups, assessed using either Welch’s t-test (if both are normally distributed) or Wilcoxon test, are indicated by asterisks (*p ≤ 0.05; **p ≤ 0.01).
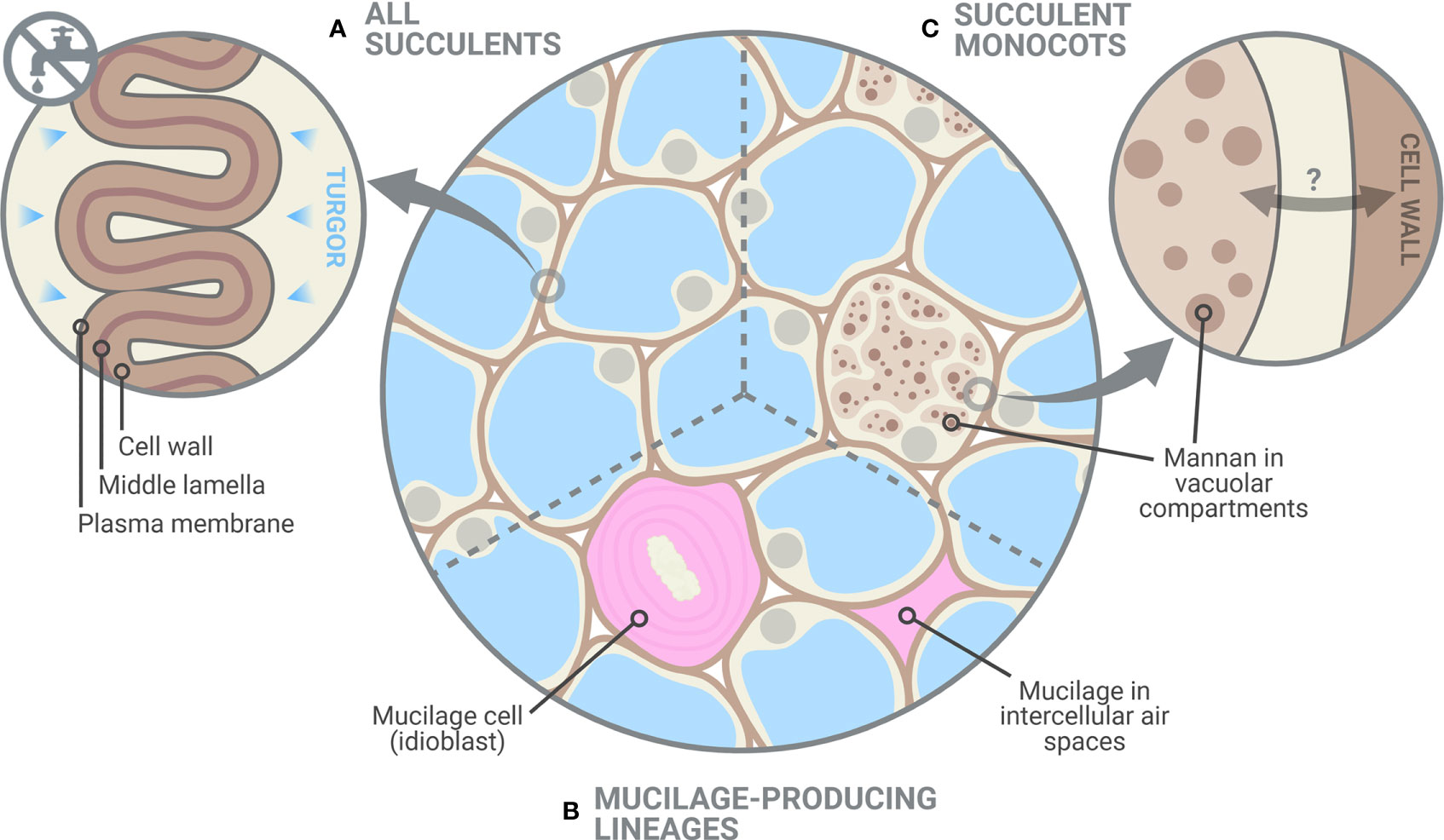
Figure 4 Glycomic diversity among succulent plants. (A) Succulent tissues have thin and highly elastic cell walls and, as shown in this study, elastic adjustment through cell wall remodelling likely plays a crucial role in preventing turgor loss during dehydration. Despite the clear differences between succulents and non-succulents, we also noted considerable glycomic diversity among succulents. (B) Mucilage-producing succulent lineages, mostly those in the Caryophyllales and particularly in the Portulacineae, accumulate pectin-rich mucilage in the periplasmic space of mucilage cells and/or in intercellular spaces, which boosts their water-storage capacity (Mauseth, 2005; Ogburn and Edwards, 2009). (C) Storage mannans can be found in vegetative tissues of many monocot lineages, often stored within vacuolar compartments. In succulent monocots, mobilization of these mannans may be part of the drought response, as seen in Aloe (Ahl et al., 2019), in which cell wall-associated mannan is remobilized into the protoplasm. However, the dynamics between cell-wall associated and vacuolar mannans in monocots remain largely unexplored. Created with BioRender.com.
In addition to MFA, we used a random forest (RF) algorithm to determine whether glycomic profiles can be used to predict if a species is succulent or non-succulent (see Suppl. Methods). Based on CoMPP data alone, the RF algorithm was able to classify species in their respective categories with 90% accuracy (Suppl. Data). The variable importance plot from the RF algorithm identified several cell wall components driving this classification (Figure S3), namely arabinogalactan proteins (AGPs), xylans, low-DM HGs and RG-I (incl. arabinan and galactan side chains). Regarding HGs and RG-I, these results agree with the differences between succulents and non-succulents mentioned above. We also observed drastically lower levels of xylans in the succulents studied, compared to the non-succulent species (Suppl. Data). However, xylans are often found in lignified support tissues (Zhong et al., 2013), and small-stature succulent species such as the ones we studied generally lack these tissues, relying primarily on turgor for support (Niklas, 1992; Gibson, 1996; Bobich and North, 2009). Thus, such differences may not hold for larger succulents. An interesting observation concerns AGPs, a notoriously complex group of cell wall glycoproteins with many suggested functions (Seifert and Roberts, 2007; Silva et al., 2020). LM14 and MAC207, two mAbs that recognize the same or structurally related AGP epitopes (Marzec et al., 2015; Yan et al., 2015), did not yield any signal among succulents despite being present in most non-succulent species tested (Suppl. Data). In contrast, other AGP-targeting mAbs (e.g. JIM13) showed comparable levels between the two groups, likely reflecting the diversity of AGPs and their numerous alleged functions. For instance, periplasmic AGPs have been postulated as stabilizers of the membrane-cell wall continuum and may also act as cell wall plasticizers when they are released from their membrane anchors (Gens et al., 2000; Knox, 2006; Lamport et al., 2006; Liu et al., 2015). The striking differences in signal intensity of the AGP-targeting mAbs we used warrant further exploration into the specific epitopes that they recognize and their functions.
The mobilization of soluble mannans has been suggested as a general drought response among succulents, based on studies of succulent leaves of Aloe and succulent-like storage organs of orchids and monocot geophytes (Ranwala and Miller, 2008; Wang et al., 2008; Chua et al., 2013; Ahl et al., 2019). However, our CoMPP data showed no clear difference between the mannans of succulents and non-succulents (Figure 3). Instead, two species exhibited remarkably high signal for loosely bound soluble mannans, Aloe distans (leaf succulent) and Epipremnum aureum (non-succulent), with Dioscorea oppositifolia (non-succulent) also showing above-average levels. These three species are the only non-commelinid monocots included in this study. Among angiosperms, the presence of storage mannans in vegetative tissues is believed to be restricted to monocots, with mannans being stored in granular or highly hydrated mucilaginous form within vacuolar cell compartments (Meier and Reid, 1982; He et al., 2017). Soluble mannans may therefore be uniquely important to monocots, being repurposed for drought response in succulent monocots (e.g. Ahl et al., 2019; Figure 4), and not a component of a more general succulent glycome.
Conclusions and future directions
Cell wall biomechanics and biochemistry of succulent leaves exhibit distinct differences from non-succulent species. In non-succulent species, highly rigid cell walls prevent elastic adjustment from having a physiologically meaningful impact on the TLPΨ (Bartlett et al., 2012). However, many succulent species have highly elastic cell walls, and our modelling indicates that even slight increases in cell wall elasticity (i.e. decreases in ε) in these species can have a large exponential effect on the TLPΨ. Therefore, succulent plants use elastic adjustment advantageously during dehydration to acclimate to declining Ψ. In addition to biomechanical differences, our glycomic data show several similarities across phylogenetically diverse succulent species, namely a higher degree of HG methyl-esterification and a greater abundance of RG-I. These biochemical differences likely contribute to the high elasticity in the cell walls of succulent organs, which in turn facilitates the folding process during dehydration (Fradera-Soler et al., 2022). Interestingly, some glycomic features seem to be restricted to certain succulent lineages, pointing to some glycomic diversity among succulent plants: succulent monocots may have co-opted soluble mannans for drought response, whereas succulents in the Caryophyllales contain pectin-rich apoplastic mucilage which boosts their water-storage capacity. Together, our data demonstrate that succulent plants occupy a unique phenotypic space regarding both cell wall biomechanics and biochemistry. We suggest that cell wall traits should be regarded as one of the core components of the adaptations that make up the succulent syndrome.
Looking forward, it will be valuable to explore cell wall biology among closely related succulent taxa and considering cell wall trait heterogeneity within succulent organs. Cell wall thickness and elasticity are known to differ between hydrenchyma and chlorenchyma in some succulent organs (Goldstein et al., 1991; Nobel, 2006; Leverett et al., 2022), but further examination of cell wall biomechanics and biochemistry is needed to fully understand how these traits aid in whole-plant survival during drought. Ultimately, further research is needed into the dynamic nature of cell walls in succulent plants and to determine whether cell wall traits are indeed regulated during drought. Besides high-throughput methods based on immune-profiling such as CoMPP, our understanding of cell wall composition, structure and assembly in succulents can also be advanced using visualization with fluorescent probes (Rydahl et al., 2018; Bidhendi et al., 2020), high-resolution microscopy techniques (Zhao et al., 2019; DeVree et al., 2021), and nuclear magnetic resonance (NMR) (Zhao et al., 2020).
Data availability statement
The original contributions presented in the study are included in the article/Supplementary Material. Further inquiries can be directed to the corresponding authors.
Author contributions
MF-S and AL conceived the study and wrote the manuscript. AL designed and conducted modelling and data analysis regarding cell wall biomechanics. MF-S designed and conducted experiments and data analysis regarding cell wall biochemistry. JM, BJ, AB and OG contributed with supervision, review and editing of the manuscript. All authors read and approved the final manuscript.
Funding
This research was partially funded by Newcastle University’s R. B. Cook Scholarship. JM is supported by a grant from the Slovak Academy of Sciences (project number IM-2021-23). This project has received funding from the European Union’s Horizon 2020 research and innovation programme under the Marie Skłodowska-Curie grant agreement No 801199.
Acknowledgments
The authors would like to thank Matthew Ogburn and Erika Edwards for the data that was used in the biomechanical modelling (see Ogburn and Edwards, 2012). We would also like to thank Theodor E. Bolsterli, Morten L. Stephensen, Ouda Khammy, Davide Visintainer and Luu Trinh (PLEN, University of Copenhagen) and the Kakteen-Haage nursery (Erfurt, Germany) for providing plant material. A special thanks to Sylwia Głazowska and Jeanett Hansen (PLEN, University of Copenhagen) for support during laboratory work, and to Karen S. Nissen for helpful discussion regarding statistical analyses.
Conflict of interest
The authors declare that the research was conducted in the absence of any commercial or financial relationships that could be construed as a potential conflict of interest.
Publisher’s note
All claims expressed in this article are solely those of the authors and do not necessarily represent those of their affiliated organizations, or those of the publisher, the editors and the reviewers. Any product that may be evaluated in this article, or claim that may be made by its manufacturer, is not guaranteed or endorsed by the publisher.
Supplementary material
The Supplementary Material for this article can be found online at: https://www.frontiersin.org/articles/10.3389/fpls.2022.1043429/full#supplementary-material
Abbreviations
Physiological parameters: ε, Bulk modulus of cell wall elasticity; P, Turgor pressure; RWC, Relative water content; TLPΨ, Turgor loss point, i.e. water potential at which turgor is lost; Ψ, Water potential; πO, Osmotic potential of tissue at full hydration. Cell wall polymers: AGP, Arabinogalactan protein; HG, Homogalacturonan; RG-I, Rhamnogalacturonan I; DM, Degree of methyl-esterification; DP, Degree of polymerization.
References
Ahl, L. I., Grace, O. M., Pedersen, H. L., Willats, W. G. T., Jørgensen, B., Rønsted, N. (2018). Analyses of Aloe polysaccharides using carbohydrate microarray profiling. J. AOAC Int. 101, 1720–1728. doi: 10.5740/jaoacint.18-0120
Ahl, L. I., Mravec, J., Jørgensen, B., Rudall, P. J., Rønsted, N., Grace, O. M. (2019). Dynamics of intracellular mannan and cell wall folding in the drought responses of succulent Aloe species. Plant Cell Environ. 42, 2458–2471. doi: 10.1111/pce.13560
Arakaki, M., Christin, P.-A., Nyffeler, R., Lendel, A., Eggli, U., Ogburn, R. M., et al. (2011). Contemporaneous and recent radiations of the world’s major succulent plant lineages. PNAS 108, 8379–8384. doi: 10.1073/pnas.1100628108
Baker, W. J., Bailey, P., Barber, V., Barker, A., Bellot, S., Bishop, D., et al. (2022). A comprehensive phylogenomic platform for exploring the angiosperm tree of life. Syst. Biol. 71, 301–319. doi: 10.1093/sysbio/syab035
Bartlett, M. K., Scoffoni, C., Sack, L. (2012). The determinants of leaf turgor loss point and prediction of drought tolerance of species and biomes: A global meta-analysis. Ecol. Lett. 15, 393–405. doi: 10.1111/j.1461-0248.2012.01751.x
Beadle, C. L., Ludlow, M. M., Honeysett, J. L. (1993). “Water relations,” in Photosynthesis and production in a changing environment: A field and laboratory manual. Eds. Hall, D. O., Scurlock, J. M. O., Bolhàr-Nordenkampf, H. R., Leegood, R. C., Long, S. P. (Dordrecht: Springer Netherlands), 113–128. doi: 10.1007/978-94-011-1566-7_8
Bidhendi, A. J., Chebli, Y., Geitmann, A. (2020). Fluorescence visualization of cellulose and pectin in the primary plant cell wall. J. Microsc. 278, 164–181. doi: 10.1111/jmi.12895
Bidhendi, A. J., Geitmann, A. (2016). Relating the mechanics of the primary plant cell wall to morphogenesis. J. Exp. Bot. 67, 449–461. doi: 10.1093/jxb/erv535
Bobich, E. G., North, G. B. (2009). “Structural implications of succulence: architecture, anatomy, and mechanics of photosynthetic stem succulents, pachycauls, and leaf succulents,” in Perspectives in biophysical plant ecophysiology: A tribute to park s. Nobel. Eds. de la Barrera, E., Smith, W. K. (México: UNAM), 3–37.
Borland, A. M., Griffiths, H., Hartwell, J., Smith, J. A. C. (2009). Exploiting the potential of plants with crassulacean acid metabolism for bioenergy production on marginal lands. J. Exp. Bot. 60, 2879–2896. doi: 10.1093/jxb/erp118
Borland, A. M., Leverett, A., Hurtado-Castano, N., Hu, R., Yang, X. (2018). “Functional anatomical traits of the photosynthetic organs of plants with crassulacean acid metabolism,” in The leaf: A platform for performing photosynthesis. Eds. Adams, W. W., III, Terashima, I. (Cham: Springer International Publishing), 281–305. doi: 10.1007/978-3-319-93594-2_10
Brodribb, T. J., Skelton, R. P., McAdam, S. A. M., Bienaimé, D., Lucani, C. J., Marmottant, P. (2016). Visual quantification of embolism reveals leaf vulnerability to hydraulic failure. New Phytol. 209, 1403–1409. doi: 10.1111/nph.13846
Cárdenas, A., Higuera-Ciapara, I., Goycoolea, F. M. (1997). Rheology and aggregation of cactus (Opuntia ficus-indica) mucilage in solution. J. Prof. Assoc. Cactus Dev. 2, 152–159. doi: 10.56890/jpacd.v2i.181
Carpita, N. C., Ralph, J., McCann, M. C. (2015). “The cell wall,” in Biochemistry and molecular biology of plants. Eds. Buchanan, B. B., Gruissem, W., Jones, R. L. (Chichester: John Wiley & Sons), 45–110.
Carroll, S., Amsbury, S., Durney, C. H., Smith, R. S., Morris, R. J., Gray, J. E., et al. (2022). Altering arabinans increases Arabidopsis guard cell flexibility and stomatal opening. Curr. Biol. 32, 3170–3179. doi: 10.1016/j.cub.2022.05.042
Chebli, Y., Geitmann, A. (2017). Cellular growth in plants requires regulation of cell wall biochemistry. Curr. Opin. Cell Biol. 44, 28–35. doi: 10.1016/j.ceb.2017.01.002
Choat, B., Brodribb, T. J., Brodersen, C. R., Duursma, R. A., López, R., Medlyn, B. E. (2018). Triggers of tree mortality under drought. Nature 558, 531–539. doi: 10.1038/s41586-018-0240-x
Chua, M., Hocking, T. J., Chan, K., Baldwin, T. C. (2013). Temporal and spatial regulation of glucomannan deposition and mobilization in corms of Amorphophallus konjac (Araceae). Am. J. Bot. 100, 337–345. doi: 10.3732/ajb.1200547
Cosgrove, D. J. (2005). Growth of the plant cell wall. Nat. Rev. Mol. Cell Biol. 6, 850–861. doi: 10.1038/nrm1746
Davis, S. C., Kuzmick, E. R., Niechayev, N., Hunsaker, D. J. (2017). Productivity and water use efficiency of Agave americana in the first field trial as bioenergy feedstock on arid lands. GCB Bioenergy 9, 314–325. doi: 10.1111/gcbb.12324
DeVree, B. T., Steiner, L. M., Głazowska, S., Ruhnow, F., Herburger, K., Persson, S., et al. (2021). Current and future advances in fluorescence-based visualization of plant cell wall components and cell wall biosynthetic machineries. Biotechnol. Biofuels 14, 1–26. doi: 10.1186/s13068-021-01922-0
Eggli, U., Nyffeler, R. (2009). Living under temporarily arid conditions – succulence as an adaptive strategy. Bradleya 2009, 13–36. doi: 10.25223/brad.n27.2009.a10
Fradera-Soler, M., Grace, O. M., Jørgensen, B., Mravec, J. (2022). Elastic and collapsible: Current understanding of cell walls in succulent plants. J. Exp. Bot. 73, 2290–2307. doi: 10.1093/jxb/erac054
Fradera-Soler, M., Rudall, P. J., Prychid, C. J., Grace, O. M. (2021). Evolutionary success in arid habitats: Morpho-anatomy of succulent leaves of Crassula species from southern Africa. J. Arid. Environ. 185, 104319. doi: 10.1016/j.jaridenv.2020.104319
Gens, J. S., Fujiki, M., Pickard, B. G. (2000). Arabinogalactan protein and wall-associated kinase in a plasmalemmal reticulum with specialized vertices. Protoplasma 212, 115–134. doi: 10.1007/BF01279353
Gibson, A. C. (1996). Structure-function relations of warm desert plants (Berlin, Heidelberg: Springer-Verlag).
Ginestra, G., Parker, M. L., Bennett, R. N., Robertson, J., Mandalari, G., Narbad, A., et al. (2009). Anatomical, chemical, and biochemical characterization of cladodes from prickly pear [Opuntia ficus-indica (L.) mill.]. J. Agric. Food Chem. 57, 10323–10330. doi: 10.1021/jf9022096
Goldstein, G., Andrade, J. L., Nobel, P. S. (1991). Differences in water relations parameters for the chlorenchyma and the parenchyma of Opuntia ficus-indica under wet versus dry conditions. Funct. Plant Biol. 18, 95–107. doi: 10.1071/pp9910095
Grace, O. M. (2019). Succulent plant diversity as natural capital. Plants People Planet. 1, 336–345. doi: 10.1002/ppp3.25
Griffiths, H., Males, J. (2017). Succulent plants. Curr. Biol. 27, R890–R896. doi: 10.1016/j.cub.2017.03.021
Harholt, J., Suttangkakul, A., Scheller, H. V. (2010). Biosynthesis of pectin. Plant Physiol. 153, 384–395. doi: 10.1104/pp.110.156588
Hartzell, S., Bartlett, M. S., Inglese, P., Consoli, S., Yin, J., Porporato, A. (2021). Modelling nonlinear dynamics of crassulacean acid metabolism productivity and water use for global predictions. Plant Cell Environ. 44, 34–48. doi: 10.1111/pce.13918
Henry, C., John, G. P., Pan, R., Bartlett, M. K., Fletcher, L. R., Scoffoni, C., et al. (2019). A stomatal safety-efficiency trade-off constrains responses to leaf dehydration. Nat. Commun. 10, 3398. doi: 10.1038/s41467-019-11006-1
Hernandes-Lopes, J., Oliveira-Neto, M. A., Melo-de-Pinna, G. F. A. (2016). Different ways to build succulent leaves in portulacineae (Caryophyllales). Int. J. Plant Sci. 177, 198–208. doi: 10.1086/684178
He, C., Wu, K., Zhang, J., Liu, X., Zeng, S., Yu, Z., et al. (2017). Cytochemical localization of polysaccharides in Dendrobium officinale and the involvement of DoCSLA6 in the synthesis of mannan polysaccharides. Front. Plant Sci. 8. doi: 10.3389/fpls.2017.00173
Heyduk, K. (2021). The genetic control of succulent leaf development. Curr. Opin. Plant Biol. 59, 101978. doi: 10.1016/j.pbi.2020.11.003
Heyduk, K., McKain, M. R., Lalani, F., Leebens-Mack, J. (2016). Evolution of a CAM anatomy predates the origins of crassulacean acid metabolism in the agavoideae (Asparagaceae). Mol. Phylogenet. Evol. 105, 102–113. doi: 10.1016/j.ympev.2016.08.018
Hocq, L., Pelloux, J., Lefebvre, V. (2017). Connecting homogalacturonan-type pectin remodeling to acid growth. Trends Plant Sci. 22, 20–29. doi: 10.1016/j.tplants.2016.10.009
Jiao, W., Wang, L., Smith, W. K., Chang, Q., Wang, H., D’Odorico, P. (2021). Observed increasing water constraint on vegetation growth over the last three decades. Nat. Commun. 12, 3777. doi: 10.1038/s41467-021-24016-9
Jones, A. M., Zhou, Y., Held, M. A., Davis, S. C. (2020). Tissue composition of Agave americana l. yields greater carbohydrates from enzymatic hydrolysis than advanced bioenergy crops. Front. Plant Sci. 11. doi: 10.3389/fpls.2020.00654
Knox, J. P. (2006). Up against the wall: arabinogalactan-protein dynamics at cell surfaces. New Phytol. 169, 443–445. doi: 10.1111/j.1469-8137.2006.01640.x
Kunert, N., Zailaa, J., Herrmann, V., Muller-Landau, H. C., Wright, S. J., Pérez, R., et al. (2021). Leaf turgor loss point shapes local and regional distributions of evergreen but not deciduous tropical trees. New Phytol. 230, 485–496. doi: 10.1111/nph.17187
Lamport, D. T. A., Kieliszewski, M. J., Showalter, A. M. (2006). Salt stress upregulates periplasmic arabinogalactan proteins: using salt stress to analyse AGP function. New Phytol. 169, 479–492. doi: 10.1111/j.1469-8137.2005.01591.x
Leverett, A., Castaño, N. H., Ferguson, K., Winter, K., Borland, A. M. (2021). Crassulacean acid metabolism (CAM) supersedes the turgor loss point (TLP) as an important adaptation across a precipitation gradient, in the genus Clusia. Funct. Plant Biol. 48, 703–716. doi: 10.1071/FP20268
Leverett, A., Hartzell, S., Winter, K., Garcia, M., Aranda, J., Virgo, A., et al. (2022). Dissecting succulence: Crassulacean acid metabolism and hydraulic capacitance are independent adaptations in clusia leaves. bioRxiv. doi: 10.1101/2022.03.30.486278
Lim, S. D., Mayer, J. A., Yim, W. C., Cushman, J. C. (2020). Plant tissue succulence engineering improves water-use efficiency, water-deficit stress attenuation and salinity tolerance in Arabidopsis. Plant J. 103, 1049–1072. doi: 10.1111/tpj.14783
Lim, S. D., Yim, W. C., Liu, D., Hu, R., Yang, X., Cushman, J. C. (2018). A Vitis vinifera basic helix–loop–helix transcription factor enhances plant cell size, vegetative biomass and reproductive yield. Plant Biotechnol. J. 16, 1595–1615. doi: 10.1111/pbi.12898
Li, H., Pattathil, S., Foston, M. B., Ding, S.-Y., Kumar, R., Gao, X., et al. (2014). Agave proves to be a low recalcitrant lignocellulosic feedstock for biofuels production on semi-arid lands. Biotechnol. Biofuels 7, 50. doi: 10.1186/1754-6834-7-50
Liu, Z., Persson, S., Sánchez-Rodríguez, C. (2015). At The border: the plasma membrane–cell wall continuum. J. Exp. Bot. 66, 1553–1563. doi: 10.1093/jxb/erv019
Marzec, M., Szarejko, I., Melzer, M. (2015). Arabinogalactan proteins are involved in root hair development in barley. J. Exp. Bot. 66, 1245–1257. doi: 10.1093/jxb/eru475
Mauseth, J. D. (2005). Anatomical features, other than wood, in subfamily opuntioideae (Cactaceae). Haseltonia 2005 (11), 113–125. doi: 10.2985/1070-0048(2005)11[113:AFOTWI]2.0.CO;2
Meier, H., Reid, J. S. G. (1982). “Reserve polysaccharides other than starch in higher plants,” in Plant carbohydrates I. Eds. Loewus, F. A., Tanner, W. (Berlin, Heidelberg: Springer), 418–471. doi: 10.1007/978-3-642-68275-9_11
Moller, I., Sørensen, I., Bernal, A. J., Blaukopf, C., Lee, K., Øbro, J., et al. (2007). High-throughput mapping of cell-wall polymers within and between plants using novel microarrays. Plant J. 50, 1118–1128. doi: 10.1111/j.1365-313X.2007.03114.x
Moore, J. P., Nguema-Ona, E. E., Vicré-Gibouin, M., Sørensen, I., Willats, W. G. T., Driouich, A., et al. (2013). Arabinose-rich polymers as an evolutionary strategy to plasticize resurrection plant cell walls against desiccation. Planta 237, 739–754. doi: 10.1007/s00425-012-1785-9
Neupane, D., Mayer, J. A., Niechayev, N. A., Bishop, C. D., Cushman, J. C. (2021). Five-year field trial of the biomass productivity and water input response of cactus pear (Opuntia spp.) as a bioenergy feedstock for arid lands. GCB Bioenergy 13, 719–741. doi: 10.1111/gcbb.12805
Niklas, K. J. (1992). Plant biomechanics: An engineering approach to plant form and function (Chicago: University of Chicago Press).
Nobel, P. S. (2006). Parenchyma–chlorenchyma water movement during drought for the hemiepiphytic cactus Hylocereus undatus. Ann. Bot. 97, 469–474. doi: 10.1093/aob/mcj054
Ogburn, R. M., Edwards, E. J. (2009). Anatomical variation in cactaceae and relatives: trait lability and evolutionary innovation. Am. J. Bot. 96, 391–408. doi: 10.3732/ajb.0800142
Ogburn, R. M., Edwards, E. J. (2010). “The ecological water-use strategies of succulent plants,” in Advances in botanical research. Eds. Kader, J.-C., Delseny, M. (Burlington, MA: Academic Press), 179–225. doi: 10.1016/B978-0-12-380868-4.00004-1
Ogburn, R. M., Edwards, E. J. (2012). Quantifying succulence: a rapid, physiologically meaningful metric of plant water storage. Plant Cell Environ. 35, 1533–1542. doi: 10.1111/j.1365-3040.2012.02503.x
Owen, N. A., Griffiths, H. (2014). Marginal land bioethanol yield potential of four crassulacean acid metabolism candidates (Agave fourcroydes, Agave salmiana, Agave tequilana and Opuntia ficus-indica) in Australia. GCB Bioenergy 6, 687–703. doi: 10.1111/gcbb.12094
Palin, R., Geitmann, A. (2012). The role of pectin in plant morphogenesis. Biosystems 109, 397–402. doi: 10.1016/j.biosystems.2012.04.006
Popper, Z. A., Michel, G., Hervé, C., Domozych, D. S., Willats, W. G. T., Tuohy, M. G., et al. (2011). Evolution and diversity of plant cell walls: from algae to flowering plants. Annu. Rev. Plant Biol. 62, 567–590. doi: 10.1146/annurev-arplant-042110-103809
Ranwala, A. P., Miller, W. B. (2008). Analysis of nonstructural carbohydrates in storage organs of 30 ornamental geophytes by high-performance anion-exchange chromatography with pulsed amperometric detection. New Phytol. 180, 421–433. doi: 10.1111/j.1469-8137.2008.02585.x
Rydahl, M. G., Hansen, A. R., Kračun, S. K., Mravec, J. (2018). Report on the current inventory of the toolbox for plant cell wall analysis: proteinaceous and small molecular probes. Front. Plant Sci. 9. doi: 10.3389/fpls.2018.00581
Seifert, G. J., Roberts, K. (2007). The biology of arabinogalactan proteins. Annu. Rev. Plant Biol. 58, 137–161. doi: 10.1146/annurev.arplant.58.032806.103801
Sheffield, J., Wood, E. F. (2008). Global trends and variability in soil moisture and drought characteristics 1950–2000, from observation-driven simulations of the terrestrial hydrologic cycle. J. Clim. 21, 432–458. doi: 10.1175/2007JCLI1822.1
Silva, J., Ferraz, R., Dupree, P., Showalter, A. M., Coimbra, S. (2020). Three decades of advances in arabinogalactan-protein biosynthesis. Front. Plant Sci. 11. doi: 10.3389/fpls.2020.610377
Trueba, S., Pan, R., Scoffoni, C., John, G. P., Davis, S. D., Sack, L. (2019). Thresholds for leaf damage due to dehydration: declines of hydraulic function, stomatal conductance and cellular integrity precede those for photochemistry. New Phytol. 223, 134–149. doi: 10.1111/nph.15779
Vignon, M. R., Gey, C. (1998). Isolation, 1H and 13C NMR studies of (4-O-methyl-D-glucurono)-D-xylans from luffa fruit fibres, jute bast fibres and mucilage of quince tree seeds. Carbohydr. Res. 307, 107–111. doi: 10.1016/S0008-6215(98)00002-0
Vollenweider, P., Menard, T., Arend, M., Kuster, T. M., Günthardt-Goerg, M. S. (2016). Structural changes associated with drought stress symptoms in foliage of central European oaks. Trees 30, 883–900. doi: 10.1007/s00468-015-1329-6
Wang, C.-Y., Chiou, C.-Y., Wang, H.-L., Krishnamurthy, R., Venkatagiri, S., Tan, J., et al. (2008). Carbohydrate mobilization and gene regulatory profile in the pseudobulb of Oncidium orchid during the flowering process. Planta 227, 1063–1077. doi: 10.1007/s00425-007-0681-1
Willats, W. G. T., McCartney, L., Mackie, W., Knox, J. P. (2001). Pectin: cell biology and prospects for functional analysis. Plant Mol. Biol. 47, 9–27. doi: 10.1023/A:1010662911148
Yan, Y., Takáč, T., Li, X., Chen, H., Wang, Y., Xu, E., et al. (2015). Variable content and distribution of arabinogalactan proteins in banana (Musa spp.) under low temperature stress. Front. Plant Sci. 6. doi: 10.3389/fpls.2015.00353
Zhang, Y.-J., Rockwell, F. E., Graham, A. C., Alexander, T., Holbrook, N. M. (2016). Reversible leaf xylem collapse: A potential “circuit breaker” against cavitation. Plant Physiol. 172, 2261–2274. doi: 10.1104/pp.16.01191
Zhao, W., Fernando, L. D., Kirui, A., Deligey, F., Wang, T. (2020). Solid-state NMR of plant and fungal cell walls: a critical review. Solid State Nucl. Magn. Reson. 107, 101660. doi: 10.1016/j.ssnmr.2020.101660
Zhao, Y., Man, Y., Wen, J., Guo, Y., Lin, J. (2019). Advances in imaging plant cell walls. Trends Plant Sci. 24, 867–878. doi: 10.1016/j.tplants.2019.05.009
Keywords: succulence, plant diversity, cell walls, cell wall elasticity, CoMPP, glycomics, turgor
Citation: Fradera-Soler M, Leverett A, Mravec J, Jørgensen B, Borland AM and Grace OM (2022) Are cell wall traits a component of the succulent syndrome? Front. Plant Sci. 13:1043429. doi: 10.3389/fpls.2022.1043429
Received: 13 September 2022; Accepted: 31 October 2022;
Published: 25 November 2022.
Edited by:
Howard Scott Neufeld, Appalachian State University, United StatesReviewed by:
Kim Johnson, La Trobe University, AustraliaHeather Joesting, Georgia Southern University, United States
Copyright © 2022 Fradera-Soler, Leverett, Mravec, Jørgensen, Borland and Grace. This is an open-access article distributed under the terms of the Creative Commons Attribution License (CC BY). The use, distribution or reproduction in other forums is permitted, provided the original author(s) and the copyright owner(s) are credited and that the original publication in this journal is cited, in accordance with accepted academic practice. No use, distribution or reproduction is permitted which does not comply with these terms.
*Correspondence: Marc Fradera-Soler, bWZzQHBsZW4ua3UuZGs=; Olwen M. Grace, by5ncmFjZUBrZXcub3Jn
†These authors have contributed equally to this work and share first authorship