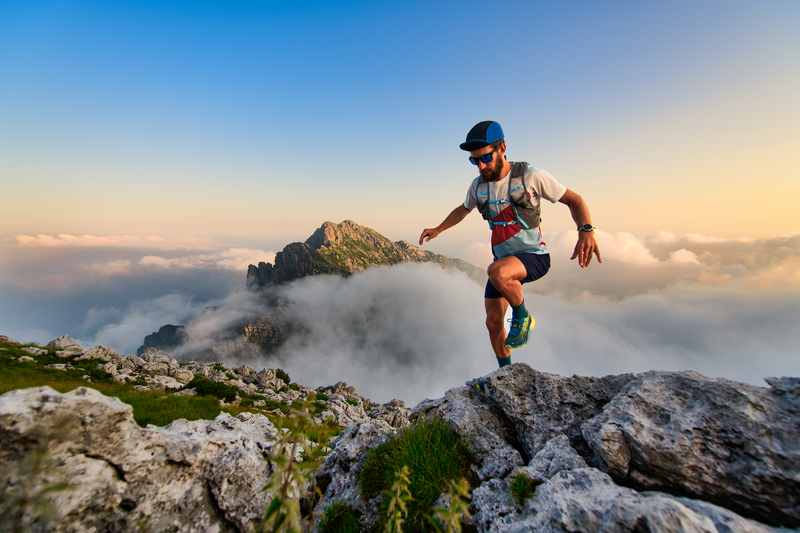
95% of researchers rate our articles as excellent or good
Learn more about the work of our research integrity team to safeguard the quality of each article we publish.
Find out more
ORIGINAL RESEARCH article
Front. Plant Sci. , 05 December 2022
Sec. Plant Physiology
Volume 13 - 2022 | https://doi.org/10.3389/fpls.2022.1033953
Tropical grasslands are very important to global carbon and water cycles. C4 plants have increased heat tolerance and a CO2 concentrating mechanism that often reduces responses to elevated concentrations of CO2 ([CO2]). Despite the importance of tropical grasslands, there is a scarcity of studies that elucidate how managed tropical grasslands will be affected by elevated [CO2] and warming. In our study, we used a combination of a temperature-free air-controlled enhancement (T-FACE) and a free-air carbon dioxide enrichment (FACE) systems to increase canopy temperature and [CO2] under field conditions, respectively. We warmed a field-grown pasture dominated by the C4 tropical forage grass Megathyrsus maximus by 2°C above ambient under two levels of [CO2] (ambient (aC) and elevated (eC - 600 ppm) to investigate how these two factors isolated or combined regulate water relations through stomatal regulation, and how this combination affects PSII functioning, biochemistry, forage nutritive value, and digestibility. We demonstrated that the effects of warming negated the effects of eC in plant transpiration, water potential, proline content, and soil moisture conservation, resulting in warming canceling the eCO2-induced improvement in these parameters. Furthermore, there were additive effects between eC and warming for chlorophyll fluorescence parameters and aboveground nutritive value. Warming sharply intensified the eCO2-induced decrease in crude protein content and increases in forage fibrous fraction and lignin, resulting in a smaller forage digestibility under a warmer CO2-enriched atmosphere. Our results highlight the importance of multifactorial studies when investigating global change impacts on managed ecosystems and the potential consequences for the global carbon cycle like amplification in methane emissions by ruminants and feeding a positive climate feedback system.
Atmospheric CO2 concentration ([CO2]) and temperature are critical components of global carbon and water cycles. Anthropogenic greenhouse emissions are expected to increase atmospheric [CO2] to 600 ppm by 2050 with a simultaneous warming of the troposphere of +2°C above pre-industrial values (IPCC, 2021). This unequivocal influence of anthropic activity on climate is already causing significative disturbances on natural (Brienen et al., 2015) and managed ecosystems (Jägermeyr et al., 2021; Spera et al., 2020). The impacts of human-induced climate change will presumably be more intense in tropical and subtropical developing countries (Chapagain et al., 2020) where agriculture and livestock are the main economic activities. In tropical and subtropical countries, meat and milk production greatly depends on productivity and nutritive value of rainfed grasslands that will likely be affected by climate change (Habermann et al., 2021). Therefore, understanding how the combination of increased levels of [CO2] and temperature will impact tropical ecosystems is paramount.
In general, elevated [CO2] (eC) and elevated temperature (eT) have contrasting effects on plant transpiration and water relations (Ainsworth and Long, 2021). On the one hand, eC often reduces stomatal conductance (gs) and transpiration rates (E) by a combination of short-term mechanisms (stomatal closure) and long-term changes (decreased stomatal density) (Xu et al., 2016). As a consequence, evapotranspiration is reduced improving plant water status and conserving soil moisture (Leakey et al., 2006; Markelz et al., 2011). Moreover, eC improves PSII performance, and photosynthetic rates (Habermann et al., 2019a), and alters the metabolism of proline (Shabbaj et al., 2022) and antioxidant enzymes (Pritchard et al., 2000). On the other hand, eT increases the permeability of K+ to guard cells and promotes H+ efflux from guard cells, leading to water influx and stomata opening (Ilan et al., 1995). As a consequence, evapotranspiration increases reducing soil moisture (Xu et al., 2013). The final effects of the combination of eC and eT will depend on a balance of the antagonistic forces over plants at the canopy and leaf level and may be affected by other biotic and abiotic factors. Despite the importance of multifactorial studies to investigate the combined effects of eC and eT on the performance of pastures, there is a paucity of leaf or canopy level studies in the literature, limiting our understanding of global change effects on pastures.
In the context of livestock, any impact of global changes on the chemical composition of leaves may change the potential acquisition of energy by animals, possibly impacting the global food production chain and food security (Craine et al., 2010). Ruminants obtain energy from plant material especially from sugars, proteins and other easily digestible compounds, while leaf fibrous fraction and lignin are anti-quality components that prevent forage from being degraded, decreasing the digestibility of food (Waghorn and Clark, 2004). Some studies (Habermann et al., 2019a) and meta-analyses (Lee et al., 2017; Moyo and Nsahlai, 2021) have indicated that future atmospheric conditions will decrease forage nutritive value by elevating lignin and fibrous fraction levels, while decreasing forage crude protein content, even under well-watered conditions (Habermann et al., 2019a). Moreover, elevated [CO2] is also expected to decrease forage nutritive value by decreasing protein levels and increasing fibrous fraction and lignin (Augustine et al., 2018). Therefore, future eC and eT conditions may synergize to produce pastures with reduced nutritive value and digestibility, impacting cattle weight gain, milk production, and the methane emissions by ruminants.
Evaluating plant performance under predicted global changing conditions has some methodological challenges that arrive from physical barriers used to control atmospheric conditions (Miglietta et al., 1999; Kimball, 2005). Some of these challenges are related to the artificial atmospheric conditions inside growth chambers or open top chambers such as modified radiation intensity and wind speed, and magnified vapor pressure deficit (Leadley et al., 1997; Uprety et al., 2006), whilst other challenges are related to limited plant growth, such as limited root development in pots or plant size in growth chambers. All these limitations may lead to different plant behaviors between controlled and field conditions (Macháčová, 2010). Here, we used a combination of a Temperature Free-Air Controlled Enhancement system (T-FACE) and a mini free-air CO2 enrichment system (mini-FACE) to warm the canopy and to increase atmospheric [CO2], respectively, in open field conditions. Until this moment, the majority of FACE and T-FACE field facilities are located in temperate regions, creating a knowledge gap in tropical and subtropical regions.
Megathyrsus maximus cv. Mombaça (C4) (popularly known as Guinea grass) is a perennial bunchgrass native to Africa and widely cultivated in Brazil for animal feeding (Hitchcock and Chase, 1910), covering around 8 million hectares of Brazilian territory. This cultivar is adapted to fertile soils (Embrapa, 2014) and has total dry matter productivity of 33 t/ha/year (Embrapa, 2012). Here, we warmed a field-grown pasture of M. maximus cv. Mombaça under ambient and elevated [CO2] using a T-FACE and FACE system to investigate how elevated [CO2] and warming interact to regulate water relations of a managed field-grown pasture of M. maximus, and how this interaction will affect PSII functioning, biochemistry, forage nutritive value, and digestibility. We hypothesized that warming will counteract the beneficial effects of elevated [CO2] on plant water relations while amplifying the negative effects of eC on forage nutritive value.
The experiment was conducted under field conditions at Trop-T-FACE facility located at the University of São Paulo at Ribeirão Preto, São Paulo State, Brazil (21° 9’42.56” S, 47° 50’ 47.12” W) from September/2015 to December/2015. The climate in this region is classified as B2rB’4a’ (moist meso-thermal with small water deficiency) (Thornthwaite, 1948) and the soil is Rhodic Ferralsol with clay texture (IUSS, 2015). Two months before planting, we conducted a soil chemical analysis using soil samples (20 cm depth) from all 16 plots to correct any nutritional deficiency or pH differences between plots. To raise soil pH to 5.5 we used calcined limestone (48% CaO and 16% MgO) and to correct soil nutritional deficiency between plots we conducted a differential soil fertilization for each plot according to Raij et al. (1997).
Seeds of Megathyrsus maximus (Syn. Panicum maximum) cv. Mombaça were sowed on 10 × 10 m plots and seedlings were irrigated until pasture establishment by a sprinkler irrigation system. Irrigation automatically watered plants in the early morning (6 am) to maintain soil water content (SWC) around 0.45 m3 m-3. After pasture establishment (which occurred approximately 2 months after sowing), plants reached 90 cm height and were clipped at 30 cm above the ground to simulate grazing. After clipping, treatments were immediately applied during 30 days of vegetative regrowth. In Brazil, rainfed pastures represent the most common feed source for ruminants. Therefore, during the experimental period, no additional irrigation was applied and plants were rainfed. We used an automatic climate station WS-PH1 connected to a data logger DL2e (Delta-T Devices, Cambridge, UK) to monitor and store the meteorological data. We monitored the SWC and soil temperature (Tsoil) hourly during the entire experiment using theta Probe ML2X and ST2 sensors, respectively, connected to a DL2e datalogger (Delta-T Devices Ltd., Burwell, Cambridge, UK) located in the center of each plot at 10-cm depth. During the growing season, a high frequency of rainfall was observed, totalizing 224 mm of total precipitation. The average total solar radiation was 0.33 kW m-2 with maximum values of 1.1 kW m-2 at the midday period. The average relative air humidity was 87% with minimum values of 38%. Average air temperature, maximum air temperature, and minimum air temperature were 25°C, 35°C, and 16°C, respectively.
In this experiment, we tested the effects of two levels of canopy temperature: ambient (aT) and elevated (eT, +2°C above ambient) under two atmospheric CO2 concentration levels: ambient CO2 concentration (aC, plots with ambient [CO2]) and elevated CO2 concentration (eC, 200 ppm above ambient CO2 concentration). We combined both factors with two levels each in four treatments (aCaT, eCaT, aCeT, and eCeT) in a randomized blocks design. The experiment was conducted with four replicates (16 plots, n = 4 for each treatment).
The plant canopy was warmed by a T-FACE system (temperature-free air-controlled enhancement system) 24 hours per day during the entire experiment as described by Kimball (2005). In each warmed plot, six 750 W infrared heaters, model FTE-750-240 Salamander ceramic infrared heating element (Mor Electric Heating, Comstock Park, MI, USA) located at 0.8 m above the canopy in a hexagonal arrangement were responsible for warming the plants. We installed each heater on an aluminum reflector Salamander ALEX (Mor Electric Heating, Comstock Park, MI, USA). We also installed six reflectors in each non-warmed plot with no infrared heater (dummy heaters) to simulate the shading (nearly negligible) between warmed and non-warmed plots (around 1% of shading). To control the canopy temperature between plots, T-FACE system used a proportional-integrative-derivative (PID) control system installed in a datalogger model CR1000 with AM2 5 T multi- plexors (Campbell Scientific, Logan, UT, USA). Canopy temperature was constantly monitored by infrared radiometers model SI-1H1-L20 (Apogee Instruments, Logan, UT, USA) located in each plot. In this study, we used the 2°C above ambient canopy temperature set-point during the entire experiment and 24 h per day.
We used a mini free-air CO2 enrichment system (mini-FACE) (Miglietta et al., 1999) to increase the atmospheric [CO2] concentration by 200 ppm above ambient [CO2] under field conditions. In each eC plot, one PVC ring of 2 m diameter punctured with micro holes fumigated pure CO2 within the plant canopy. ‘Dummy’ rings were installed in each aC plot. Each ring was placed within the 10 × 10 m plots to minimize the edge effect. To monitor the atmospheric [CO2] concentration in each plot, we installed one GMT222 CO2 transmitter sensor (Vaisala, Helsinki, Finland) at canopy height in the central region of each plot. Using a proportional integration device algorithm (PID) and [CO2] data of aC and eC plots, FACE central unit regulated the amount of [CO2] needed in eC plots to increase atmospheric [CO2] by 200 ppm above ambient [CO2]. Wind speed was measured by an anemometer located in the central region of the experimental site 3 m above the ground. The liquid pure CO2 fumigated in each plot was stored within a 12-ton cryogenic tank with a vaporizer unit. The 200 ppm above ambient [CO2] target was maintained from sunrise to sunset.
We measured leaf gas exchange in the central region of 4 fully expanded leaves per plot, between 9 and 11 am, 22 days after the treatments started (DATS). Measurements were conducted with a LCProSD+ advanced photosynthesis measurement system (ADC BioScientific, UK). Leaves were kept in the chamber until the variables remained stable. During measurements we used constant conditions of radiation (1740 μmol m-2 s-1), temperature of 30°C (aT plots) or 32°C (eT plots), and [CO2] of 400 μmol mol-1 (aC plots) or 600 μmol mol-1 (eC plots). We measured the net photosynthesis rate (A), stomatal conductance (gs), and transpiration rate (E).
Xylem water potential (Ψw) was measured using a Scholander chamber model 3005 (Soil moisture Equipment Corp., Santa Barbara, CA, USA) (Scholander et al., 1965) at 16, 23, and 25 DATS at the midday period. At 27 DATS, a diurnal course of Ψw was conducted every 4 hours from 5 am to 5 pm. For Ψw measurements, 3 fully expanded leaves were detached from each plot from different plants and placed within sealed plastic bags containing a wet filter paper. Then, samples were taken to Scholander chamber where measurements were performed (this process took approximately 3 minutes). Leaf relative water content (RWC) was measured at 16, 23, and 25 DATS at the midday period using leaf discs as described by Smart and Bingham (1974). For RWC measurements, 3 fully expanded leaves were detached from each plot from different plants and placed within sealed plastic bags containing a wet filter paper. Ten leaf discs were detached from the central region of each leaf and immediately weight to measure the fresh weight (FW). Then, samples were submerged in distilled water for 24 h at 4°C in the dark. Samples were dried to remove water from the leaf surfaces and weight to obtain the turgid weight (TW). Finally, all plant material was dried at 60°C until constant dry weight in a fan-forced oven to determine the dry weight (DW). RWC was calculated according to the equation: RWC = FW-DW/TW- DW × 100.
Three fully expanded leaves were detached from each plot from different plants and immediately placed on liquid nitrogen. Samples were kept at -20°C until analysis. Leaf proline content (Pro) was measured according to Bates et al. (1973) at 16, 23, and 25 DATS at the midday period. At 27 DATS, a diurnal course of Pro was conducted every 4 hours from 5 am to 5 pm. In summary, 200 mg of fresh leaves were macerated in liquid nitrogen and homogenized in 10 mL of sulphosalicylic acid (3%). This solution was filtered and 2 mL of filtered solution was placed in glass tubes. In each tube, we added 2 mL of acid ninhydrin (1.25 g of ninhydrin, 30 mL of glacial acetic acid, and 20 mL of phosphoric acid 6M). The tubes were maintained in a stove during 1 hour at 100°C and immediately put on ice to stop the reaction. Then, 4 mL of toluene was added to each tube and the solution was mixed in a vortex for 20 seconds. From the two-phase system formed in each tube, the upper layer (chromophore) was used for the measurements at 520 nm.
We collected 3 fully expanded leaves per plot at midday period from different plants at the end of the experimental period to measure the activity of antioxidant enzymes and the level of lipid peroxidation. Leaves were immediately placed in liquid nitrogen and maintained at -20°C until analysis. We used the method described by Azevedo et al. (1998) to obtain the crude extracts. In summary, 1 g of fresh leaves was macerated with liquid nitrogen and homogenized with 10 mL of potassium phosphate buffer (100 mM, pH 9.0) containing EDTA (1 mM), DTT (3 mM) and PVPP (2%, m/v). Crude protein content was measured according to Bradford (1976) at 595 nm. We measured the activity of four enzymes: catalase (CAT, EC 1.11.1.6) using the spectrophotometric method described by Azevedo et al. (1998), in which the results were expressed as μmol min-1 mg-1 protein using the molar extinction coefficient 40.0 M-1 cm-1. Ascorbate peroxidase (APX, EC 1.11.1.11) was determined using the Nakano and Asada (1981) method. APX activity was expressed in μmol min-1 mg-1 protein. We used the molar extinction coefficient 3051.4 M-1 cm-1, determined for our experimental conditions. Superoxide dismutase (SOD, EC 1.15.1.1) was measured as suggested in Giannopolitis and Ries (1977). In this study, a SOD unit was defined as the amount of enzyme that inhibits NBT photoreduction by 50% (Beauchamp and Fridovich, 1971). The activity of non-specific peroxidases (POD, EC 1.11.1.7) according to Zeraik et al. (2008) using guaiacol as substrate. The results were expressed in μmol min-1 mg-1 of protein, using the coefficient of molar extinction 26.6 mM-1 cm-1 (Chance and Maehly, 1955) in our calculations. We measured the level of lipid peroxidation of lead tissues determining the level of malondialdehyde (MDA) according to the thiobarbituric acid (TBA) method as described by Heath and Packer (1968).
On the last day of the experimental period, we collected 3 fully expanded leaves per plot at the midday period (between 12 am and 1 pm) and immediately put the samples on liquid nitrogen. Samples were maintained at -20°C until analysis. We used a method adapted from Lichtenthaler and Wellburn (1983). Here, 100 mg of fresh leaves were macerated in 5 mL of 80% acetone solution and centrifuged to precipitate the biggest particles. Supernatant absorbance was measured with a spectrophotometer at 480, 645 and 663 nm. We determined chlorophyll a, chlorophyll b, and carotenoid content and calculated the total chlorophyll content (a + b) and chlorophyll/carotenoid.
We measured chlorophyll fluorescence parameters from 3 fully expanded leaves per plot between 9 am and 11 am on the last day of the experiment using an imaging-PAM M-series chlorophyll fluorescence system (MINI-version model, Heinz Walz GmbH, Germany). Leaves were detached, maintained in water and dark-acclimated for 30 minutes. We measured dark fluorescence yield (Fo) using a low frequency of pulse-modulated measuring light (0.5 μmol m−2 s−1, 100 μs, 1 Hz) and maximum fluorescence yield (Fm) using a saturation pulse (2700 μmol m−2 s−1, 0.8 s, 10 Hz). Then, leaves were submitted to increasing steps of photosynthetic photon flux density (PPFD) levels (from 0 to 784 μmol photons m−2 s−1). We measured the effective PS II quantum yield during illumination Y(II), the quantum yield of non-regulated energy dissipation Y(NO), the quantum yield of regulated energy dissipation Y(NPQ) and maximum electron transport rate (ETRmax). Parameters were calculated as follows: Y(II) = (Fm’ − F)/Fm’, Y(NO) = 1/(NPQ + 1 + qL(Fm/Fo − 1), Y(NPQ) = 1 - Y(II) - 1/(NPQ+1+qL(Fm/Fo-1)), NPQ = (Fm − Fm’)/Fm’ and ETR = 0.5 × PSII yield × PAR × 0.84 equivalents m−2 s−1. Results were analyzed at 784 μmol photons m−2 s−1.
At the end of the experimental period, we harvested the aboveground material 30 cm aboveground. Plant material was oven dried at 70°C until constant weight, grounded in an electric mill (Willey type), and used to measure forage nutritive value parameters. Neutral detergent fiber (NDF) and acid detergent fiber (ADF) were determined according to the method described by Mertens (2002) using fiber determination equipment (TE-149, Tecnal). We measured nitrogen content (N) using a nitrogen distiller (TE-0363, Tecnal) and calculated the crude protein content (CP) using the equation CP = N × 6.25 (AOAC, 1990). Using the forage ADF fraction, samples were submitted to acid hydrolysis with sulfuric acid 72% as described in Silva and Queiroz (2002) to determine lignin content. In-vitro dry matter digestibility (IVDMD) was measured according to Tilley and Terry (1963) that was adjusted to artificial rumen by ANKON® using the “Daisy incubator” device from Ankon Technology (in vitro true digestibility).
Data were checked for outliers and normality. We used a two-way ANOVA with two factors (with two levels each) to evaluate the isolated effects of [CO2] and temperature and the interaction between factors. Comparisons between two average values were conducted using a student t-test. Regressions analysis was used to investigate the relations between parameters. Statistical analysis was conducted using Past and GraphPad Prism 9 software.
The effects of treatments on the microclimate conditions are shown in Figure 1 and more details can be found on Supplementary Figure 1. We observed that SWC under eCaT was higher than under aCaT during the entire experimental period, resulting in the distribution of SWC values observed in Figure 1A. However, this water conservation effect was completely offset by warming (Figure 1A). Tsoil was higher under eT plots regardless [CO2] level (Figure 1B). On average, Tcanopy at eCaT treatment was warmer than under aCaT, especially during the warmest period of the day. In warmed plots, T-FACE system maintained Tcanopy 2°C above ambient, especially during the night period (Supplementary Figure 1). However, during the day, variations among the set-point were observed, leading to an average warming of approximately 1.5°C above ambient (Figure 1C). This behavior is expected in open-field warming systems due to the higher evapotranspiration and wind speed, especially during the mid-day period (Kimball, 2005). Average [CO2] on aCaT, eCaT, aCeT, and eCeT plots were 406 ± 45, 402 ± 46, 611 ± 49, and 581 ± 43 ppm, respectively (Figure 1D). [CO2] was 404 and 596 ppm under aC and eC plots, respectively (Figure 1D).
Figure 1 Difference (Δ) in the microclimate of each treatment compared to ambient conditions (aCaT). (A) SWC = soil water content (m3 m-3), (B) Tsoil = soil temperature (°C). (C) Tcanopy = canopy temperature (°C). (D) [CO2] = atmospheric CO2 concentration. Bars show the average difference between treatments and aCaT, whilst each point shows the dispersion around the average value. Points were obtained each hour for SWC and Tsoil and every 15 minutes for Tcanopy throughout the entire experiment. For [CO2], each point represents a 5-minute average value along six days of the experimental period during the diurnal period (6 am to 6 pm - fumigation period). Treatments: aC (ambient CO2 concentration), eC (elevated CO2 concentration – 600 ppm), aT (ambient temperature), and eT (elevated temperature - 2°C above ambient temperature).
Figure 2 Linear regressions between leaf gas exchange parameters measured in fully expanded leaves of M. maximus at different levels of [CO2] and temperature. A = net photosynthesis rate. gs = stomatal conductance. E = transpiration rate. R2 and p-values are shown for each regression line according to the color of each treatment. (A) Equations: aC = Y = 16,40*X + 30,19, eC = Y = 44,53*X + 31,92. (B) Equations: aCaT = Y = 5,437*X + 1,843, eCaT = Y = 5,100*X + 2,334, aCeT = Y = 3,237*X + 3,837, and eCeT = Y = 5,845*X + 2,763. Regressions were performed using the raw data from Habermann et al. (2019). Treatments: aC (ambient CO2 concentration), eC (elevated CO2 concentration – 600 ppm), aT (ambient temperature), and eT (elevated temperature - 2°C above ambient temperature).
In general, eC improved photosynthesis regardless of temperature level (Figure 1, Supplementary Table 1), while eC and eT showed antagonistic effects on plant transpiration and water relations (Figures 1-3). Since ANOVA analysis revealed no effects of temperature on photosynthesis and stomatal aperture, we investigated the association between A and gs under both levels of [CO2]. As expected, higher values of A associated with smaller gs were observed under eC (Figure 1A). However, we observed antagonistic effects between eC and eT for E. Therefore, associations between gs and E were made for each treatment and eT completely mitigated the CO2-induced reduction of E (Figure 1B).
Figure 3 Water status and leaf proline content of fully expanded leaves of M. maximus during the experimental period in different levels of [CO2] and temperature (left column) and average values (right column). Ψw = water potential. RWC = relative water content. Pro = leaf proline content. DOE = day of the experiment. Treatments: aC (ambient CO2 concentration), eC (elevated CO2 concentration – 600 ppm), aT (ambient temperature), and eT (elevated temperature - 2°C above ambient temperature). ANOVA results are shown for diurnal averages: C: [CO2] effect, T: temperature effect, C×T: interaction between C and T. *P < 0.05, **P < 0.01, ***P < 0.001, ns, non-significant.
At 16 DATS, Ψw increased (smaller negative values) under eC regardless of temperature level by approximately 21% (Figure 3A). At 23 DATS, we observed antagonistic effects between CO2 and warming, leading to a Ψw similar to aCaT treatment. At 25 DATS, eC regardless of temperature level increased Ψw by 13% (Figure 3A). Therefore, the diurnal average Ψw was higher under eC regardless of temperature level (Figure 3B). However, no differences in RWC were observed during the experiment (Figure 3C) or in the diurnal average value (Figure 3D) as a result of CO2 enrichment or warming. PRO content was also not modified during the experiment (Figure 3E) or in the diurnal average value (Figure 3F).
Additional measurements conducted during an entire diurnal course revealed that the pattern of response observed during the experimental period was similar to those observed in the diurnal course (Figure 4). In the diurnal course conducted at 27 DATS, we observed that Ψw increased by 38% (p=0.015) at 1 pm under eC regardless of the temperature level (Figure 4A). During the entire diurnal course, we observed that Ψw remained higher (smaller negative values) under eC, resulting in a daily average Ψw 23% higher (P<0.05) than under aC regardless of the temperature level (Figure 4B). However, at 9 am and 5 pm Ψw decreased (P<0.05) due to isolated effects of eT in 45% and 44%, respectively, regardless CO2 level (Figure 4A), resulting in a daily average Ψw 25% smaller (higher negative values) (Figure 4B). Under eCeT, the antagonistic effects of CO2 and warming resulted in a diurnal average Ψw not different when compared to aCaT conditions (Figure 4B). During the diurnal course, Pro increased under eT regardless CO2 level by approximately 50% at 5 am (P<0.05) (Figure 4C), but with no significant results in Pro diurnal average (Figure 4D). On the other hand, eC regardless of temperature level decreased (P<0.05) Pro diurnal average by 19% (Figure 4D).
Figure 4 Diurnal course of water potential and leaf proline content measured in fully expanded leaves of M. maximus in different levels of [CO2] and temperature during the diurnal period (left column) and diurnal average values (right column). Ψw = water potential. Pro = leaf proline content. Treatments: aC (ambient CO2 concentration), eC (elevated CO2 concentration – 600 ppm), aT (ambient temperature), and eT (elevated temperature - 2°C above ambient temperature). ANOVA results are shown for diurnal averages: C: [CO2] effect, T: temperature effect, C×T: interaction between C and T. *P < 0.05, **P < 0.01, ***P < 0.001, ns, non-significant.
We observed that ETR increased by additive effects of eC and eT resulting in an ETR approximately 60% higher under eCeT when compared to aCaT (Table 1). Additive effects between eC and eT were also observed for Y(II). In addition, qP and qL both increased under eC regardless of temperature level. Y(NPQ) and Y(NO) were not affected by [CO2] or temperature levels. CAT, POD, and SOD activities and MDA content were also not changed as a result of treatments (Table 1). However, APX activity decreased under eT regardless [CO2] level. A few interactions between eC and eT were observed for photosynthetic pigments, but no significant difference was observed between eCeT and aCaT average values (Table 1).
Table 1 Chlorophyll fluorescence parameters, enzymatic activity, malondialdehyde levels, and photosynthetic pigments content measured on the last day of the experimental period in fully expanded leaves of M. maximus developed at two levels of [CO2] and temperature.
Significative additive effects between eC and eT were observed for forage nutritive value parameters (Figure 5). Forage fiber fraction increased due to eC and eT effects, resulting in NDF and ADF values 14% and 13.4% higher under eCeT when compared to aCaT, respectively (student t-test comparison) (Figures 5A, B). CP decreased more under combined conditions (eCeT), than under isolated effects, resulting in 21.2% less CP available in the forage material under eCeT compared to aCaT (Figure 5C). Lignin content increased by warming regardless [CO2] level by approximately 21% when compared to aT conditions (Figure 5D). As result of higher forage fiber fraction, lignin, and less CP, IVDMD decreased by 4.7%, 8.2%, and 11% under eCaT, aCeT, and eCeT, respectively, when compared to aCaT (additive effect) (Figure 5E).
Figure 5 Forage nutritional value of the aboveground dry mass of M. maximus measured in different levels of [CO2] and temperature. NDF , neutral detergent fiber. ADF , acid detergent fiber. CP , crude protein. IVDMD , in-vitro dry matter digestibility. Treatments: aC (ambient CO2 concentration), eC (elevated CO2 concentration – 600 ppm), aT (ambient temperature), and eT (elevated temperature - 2°C above ambient temperature). ANOVA results are shown for each parameter: C, [CO2] effect; T, temperature effect; C×T, interaction between C and T. *P < 0.05, **P < 0.01, ***P < 0.001, ns, non-significant.
We observed linear relations between forage nutritive value parameters and IVDMD (Figure 6). IVDMD decreased as ADF, NDF, and lignin content increased (Figures 6A–C), whilst a higher IVDMD was observed as CP increased (Figure 6D).
Figure 6 Linear regressions between for age nutritive value parameters and in-vitro dry matter digestibility (IVDMD) of M. maximus above ground dry mass measured at different levels of [CO2] and temperature. (A) NDF, neutral detergent fiber. (B) ADF, acid detergent fiber. (C) Lignin. (D) CP, crude protein. R2 and p-values are shown for each regression line. Treatments: aC (ambient CO2 concentration), eC (elevated CO2 concentration – 600 ppm), aT (ambient temperature), and aT (elevated temperature - 2°C above ambient temperature).
It is widely reported that eC greatly impacts the physiology of C3 plants. However, the impacts of eC on the growth and physiology of C4 plants are less understood (Bowes, 1993). Until recently, it was supposed that C4 photosynthesis would not be affected by eC conditions, because intercellular [CO2] (Ci) is saturated or near saturated values under aC. And there was weak evidence to support the opposite. However, several recent studies have demonstrated that photosynthesis rates of C4 plants are also increased in high [CO2] (Ainsworth and Long, 2021). Here, we demonstrated that enhanced photosynthesis under eC was associated with improved PSII performance (higher Y(II), qP, qL, and ETR) (Figure 2A; Table 1), suggesting that the extra carbon fixed under eC increased the efficiency of photosystems, corroborating the findings of Habermann et al. (2019b). Y(II) is the proportion of absorbed light that is being used in PSII photochemistry and used to calculate the electron transport rate through the PSII, whilst qL and qP estimate the fraction of open PSII centres (Walz, 2019). In this study, qP and qL increased due to the isolated effect of eC, while Y(II) and ETR increased due to additive effects of eC and eT, leading to an enhanced PSII performance under eCeT treatment (Table 1). Therefore, our results suggest that the PSII activity of M. maximus can acclimate to a warmer, CO2-enriched environment.
This improved photosynthetic performance under eC was accompanied by reduced gs and E values (Figure 2B). Smaller gs and E are widely reported to occur in other FACE studies, with no evidence indicating that this gs decrease acclimates with time (Ainsworth and Rogers, 2007; Ainsworth and Long, 2021). Smaller gs may occur due to short-term mechanisms such as stomatal closure (Ainsworth and Rogers, 2007) or long-term changes, such as decreases in stomatal density (Lin et al., 2001). The regulatory role of [CO2] in the differentiation process of the epidermic cells is well-recognized in the literature (Gray et al., 2000; Doheny-Adams et al., 2012; Engineer et al., 2014; Xu et al., 2016). For example, in a different study from this same experiment, Habermann et al. (2019b) showed that plants that developed under eC regardless of the temperature level had reduced stomatal density and stomatal index in both leaf surfaces.
One of the consequences of smaller gs and E is the modification of energy flux between canopy and environment. In this study, Tcanopy of plants under eCaT was slightly higher when compared to aCaT treatment (Figure 1C), especially during the hottest hours of the day (between 11 am and 4 pm). This CO2-induced increase in crop temperature is a well-documented response associated with the smaller gs and E under eC, which decrease the cooling capacity of the canopy (Negi et al., 2014; Šigut et al., 2015). This [CO2] effect on Tcanopy can approximate leaf temperature to the optimum temperature of photosynthesis or even exceed it, leading to some deleterious impacts on plant photosynthesis (Warren et al., 2011). However, in this study, there was no additive effect of [CO2] on Tcanopy of plants grown under eCeT (Figure 1C). Interestingly, this additive effect has been found by our research team repeated times in different experiments conducted with the forage legume Stylosanthes capitata (Fabaceae, C3) under the combination of eCeT (Habermann et al., 2019a) and under eT combined with reduced soil moisture for S. capitata (Habermann et al., 2021) and M. maximus (Habermann et al., 2019c), but not here. To our knowledge this has not been described in the literature and would be crucial information to understand water use, heat stress and to improve management and the selection of future germplasm adapted different climate conditions. We speculate that this response can be associated with a higher evaporative demand under eT plots, cancelling the smaller E under eC (Figure 1B). This can also be associated with plant genotype being highly adapted to heat conditions and thus being able to function at a warmer Tcanopy downregulating gs.
This differential stomatal response to eC and eT promoted significant impacts on the plant water status of M. maximus. Separating the direct effects of elevated temperature from some level of water stress induced by higher vapor pressure deficit (VPD) of the atmosphere is highly daunting (Xu et al., 2021). As expected, eC improved plant water status (Figures 3, 4) presumably due to the reduced gs and E (Wullschleger et al., 2002), resulting in a smaller transpiration flux and water absorption from the soil (Nelson et al., 2004). Therefore, soil moisture under eCaT plots remained higher during the experimental period (Figure 1A). It has been reported that the conservation of soil moisture under eC can ameliorate the negative impacts of moderate water shortage periods (Nelson et al., 2004). However, in this study, this water conservation effect was completely offset by eT under eCeT treatment (Figure 1A). eT also promoted an antagonistic effect in plant water status presumably due to the intensification of leaf transpiratory rates. Therefore, our data suggest that a slight increase in temperature can mitigate the beneficial effect of eC on soil moisture conservation and plant water status.
The antagonistic effect between eT and eC was also observed for leaf proline content (Figures 3E, F, 4C, D). The accumulation of protective compounds such as proline is often found to increase under heat stress (Haudecoeur et al., 2009; Szabados and Savoure, 2010). Here, eT increased leaf proline content, while eC decreased it, leading to contrary effects under combined conditions. Proline can act as reactive oxygen species (ROS) scavenger, protecting the structure of membranes and proteins, being essential in osmotic potential adjustment (Verbruggen and Hermans, 2008). In this study, we observed that MDA and chlorophyll contents were not modified by any treatment, and antioxidant enzymatic activity was mostly unaffected, except by a decrease in APX activity under eT (Table 1), suggesting a smaller investment in this antioxidant pathway defense system. Thus, the conditions of [CO2] and temperature tested in this study did not impose stressful conditions on plants.
Besides the impacts of eC and eT on the physiology and biochemistry of M. maximus, we investigated the impacts of both environmental factors on plant traits that are related to animal feeding (Figures 5, 6). In pastures, aboveground productivity is one of the most important traits that determine milk and meat production. According to the study conducted by Carvalho et al. (2020) in the same experiment, eC alone did not increase belowground or aboveground biomass of M. maximus (Supplementary Figure 2), suggesting that surplus carbon assimilated under eC was partitioned to other structures or physiological processes, such as synthesis of secondary metabolites, respiratory metabolism, or the production of fibers. Interestingly, eT was the main responsible for increasing aboveground productivity at the final harvest (Carvalho et al., 2020) (Supplementary Figure 2). This higher biomass accumulation under eT was presumably related to higher photosystem efficiency (Table 1), greater root growth (Carvalho et al., 2020), sugar partitioning (Habermann et al., 2019c), and salicylic acid (SA) biosynthesis (Wedow et al., 2019). In addition, higher biomass productivity under eT is expect to increase the demand for more N, Ca, and S of M. maximus plants (Carvalho et al., 2020). However, this eT-induced improvement in aboveground biomass is only observed when soil moisture is not a limiting factor (Viciedo et al., 2019).
Another important response observed in our study is the decrease of aboveground nutritive value and digestibility of M. maximus pasture (Figures 5, 6). Here, the aboveground fibrous fraction (NDF content) increased by eC and eT effects, leading to an additive increase under eCeT, whilst ADF increased due to isolated eT effect. ADF and NDF are anti-quality components of forages that reduces the digestibility of plant material and the amount of energy that animals obtain from forages (Waghorn and Clark, 2004). Moreover, forages with high fiber content increase the satiety level of animals, reducing animal feeding (Allen, 1996; Dias et al., 2021). The additive pattern of response promoted by eC and eT was also observed for lignin synthesis. Our results indicate that part of the additional carbon assimilated under eC was presumably being partitioned to the production of fibers. This is the first study that demonstrated that higher levels of [CO2] also contribute to reducing forage quality and digestibility of M. maximus and that eC and eT synergize to amplify this negative effect on forage traits.
Lignification process is highly dependent on temperature (Nahar et al., 2015). For instance, forages from warmer regions such as tropical zones are poorer in quality when compared to forages from temperate zones, since elevated temperature is reported as an important factor in increasing the lignification level of plant tissues (Ball et al., 2001; Lee, 2018). Lignin limits the digestion of plant material because it represents a physical barrier against the digestive enzymes from microorganisms in the rumen (Dumont et al., 2015). This eT-induced decrease of M. maximus forage quality was previously reported by Habermann et al. (2019c); Habermann et al. (2022) under well-watered and rainfed conditions. Evidence indicates that the increase of leaf fibrous fraction and lignin contents of M. maximus are not correlated with the proportion of any leaf tissue, suggesting that leaf chemical composition, not leaf tissue proportion, was the main factor reducing forage quality (Habermann et al., 2022). This hypothesis was also corroborated by Wedow et al. (2019), where authors showed that M. maximus plants developed under elevated temperature (2°C) had a higher concentration of synaptic acid and tocopherol, two intermediates of the lignin synthesis pathway, suggesting that lignin composition is being modified by eT.
Forages rich in proteins and minerals are essential to sustain cattle performance, health, and productivity (Moyo and Nsahlai, 2021). In this study, we observed an additive decrease of CP due to eC and eT, dropping protein levels from approximately 20% under aCaT to 15% under eCeT. Protein is one of the most important nutrients that animals obtain from plant material. Forages with low protein content cannot sustain the metabolism of microorganisms responsible for the digestion of plant material, resulting in a smaller ruminant’s intake and forage digestibility (Bach et al., 2005). Crude protein content also declines with rising temperatures and fiber content, as observed here (Figures 5, 6). This reduced quality of forages under future conditions of temperature and CO2 is a matter of concern since other abiotic conditions such as increased tropospheric ozone concentration (Hayes et al., 2016) and reduced soil moisture (Habermann et al., 2019c; Habermann et al., 2021) also can promote a reduction in forage quality, suggesting that when all those factors are combined more intense impacts on forages may be expected. However, there is a need for multifactorial studies that combine all those factors to test this hypothesis.
Our results, based on one grazing growth cycle, showed that high atmospheric [CO2] and warming have antagonistic effects on M. maximus plant water relations. In summary, we observed that plants fixed more carbon using fewer water resources under eC conditions, conserving more water in the soil and plant tissues. However, this water conservation effect was offset by warming. Plant metabolism was not harmed by eC or eT conditions, instead, PSII photochemistry improved due to the additive effects of both factors. Both high atmospheric [CO2] and warming synergized to increase fibrous fraction and lignin and decrease the digestibility and crude protein of this common tropical forage grass, with potential consequences for animal feeding and the global carbon cycle like an amplification in methane emissions by ruminants, driving positive carbon-climate feedback (Knapp et al., 2014; Wollenberg et al., 2016). We highlight the need for more multifactorial and long-term field studies to better elucidate how tropical grasses will be affected by future global change conditions.
The original contributions presented in the study are included in the article/Supplementary Material. Further inquiries can be directed to the corresponding author.
EH and ED wrote the manuscript. EH, CM, ED, and JC collected data in the field and processed the data. CM and KP conceived and designed the experiments. All authors contributed to the revision of the manuscript. All authors contributed to the article and approved the submitted version.
This work was supported by the Sao Paulo Research Foundation, FAPESP Thematic Project (Grant 08/58075-8) to CM. FAPESP provided graduate studentships to EH (Grant 14/26821-3; 16/09742-8) and ED (Grant 14/00317-7). CNPq provided a scholarship to DC (Grant 385485/2015-8). CM received financial support from CNPq (Process 446357/2015-4). CM is a research fellow from CNPq (Grant 302628/2019-3).
We thank Bruce Kimball from the USDA and Franco Miglietta from IBIMET, Italy. The authors thank Wolf Seeds from Ribeirão Preto, São Paulo State, Brazil, for providing seeds of M. maximus. We thank graduated and undergraduate students that contributed in the field work. We thank the Goiano Federal Institute for the support provided for bromatological analysis.
The authors declare that the research was conducted in the absence of any commercial or financial relationships that could be construed as a potential conflict of interest.
The reviewer AG declared a shared affiliation with the authors EH, ED, DC, CM to the handling editor at the time of the review.
All claims expressed in this article are solely those of the authors and do not necessarily represent those of their affiliated organizations, or those of the publisher, the editors and the reviewers. Any product that may be evaluated in this article, or claim that may be made by its manufacturer, is not guaranteed or endorsed by the publisher.
The Supplementary Material for this article can be found online at: https://www.frontiersin.org/articles/10.3389/fpls.2022.1033953/full#supplementary-material
Ainsworth, E. A., Long, S. P. (2021). 30 years of free-air carbon dioxide enrichment (FACE): What have we learned about future crop productivity and its potential for adaptation? Global Chang. Biol. 27 (1), 27–49.
Ainsworth, E. A., Rogers, A. (2007). The response of photosynthesis and stomatal conductance to rising [CO2]: mechanisms and environmental interactions. Plant Cell Environ. 30, 258–270. doi: 10.1111/j.1365-3040.2007.01641.x
Allen, M. S. (1996). Physical constraints on voluntary intake of forages by ruminants. J. Anim. Sci. 74, 3063. doi: 10.2527/1996.74123063x
AOAC (1990). Official methods of analysis. 15th edition (Washington DC: Association of Official Analytical Chemist).
Augustine, D. J., Blumenthal, D. M., Springer, T. L., LeCain, D. R., Gunter, S. A., Derner, J. D. (2018). Elevated CO2 induces substantial and persistent declines in forage quality irrespective of warming in mixedgrass prairie. Ecol. Appl. 28, 721–735. doi: 10.1002/eap.1680
Azevedo, R. A., Alas, R. M., Smith, R. J., Lea, P. J. (1998). Response of antioxidant enzymes to transfer from elevated carbon dioxide to air and ozone fumigation, in the leaves and roots of wild-type and a catalase- deficient mutant of barley. Physiol. Plant 104, 280–292. doi: 10.1034/j.1399-3054.1998.1040217.x
Bach, A., Calsamiglia, S., Stern, M. D. (2005). Nitrogen metabolism in the rumen. Int. J. Dairy Sci. 88, 9–21. doi: 10.3168/jds.S0022-0302(05)73133-7
Ball, D. M., Collins, M., Lacefield, G. D., Martin, N. P., Mertens, D. A., Olson, K. E., et al. (2001). Understanding forage quality (Park Ridge, Illinois: American Farm Bureau Federation Publication 1–01).
Bates, L. S., Waldren, R. P., Teare, I. D. (1973). Rapid determination of free proline for water-stress studies. Plant Soil 39, 205–207. doi: 10.1007/BF00018060
Beauchamp, C. O., Fridovich, I. (1971). Superoxide dismutase. improved assays and an assay applicable to acrylamide gel. Anal. Biochem. 44, 276–287. doi: 10.1016/0003-2697(71)90370-8
Bowes, G. (1993). Facing the inevitable: plants and increasing atmospheric CO2. Annu. Rev. Plant Physiol. 44, 309–332. doi: 10.1146/annurev.pp.44.060193.001521
Bradford, M. M. (1976). A rapid and sensitive method for the quantitation of microgram quantities of protein utilizing the principle of protein-dye binding. Anal. Biochem. 72, 248–254. doi: 10.1006/abio.1976.9999
Brienen, R., Phillips, O., Feldpausch, T., Gloor, E., Baker, T. R., Lloyd, J., et al. (2015). Long-term decline of the Amazon carbon sink. Nature 519, 344–348. doi: 10.1038/nature14283
Carvalho, J. M., Barreto, R. F., Prado, R., Habermann, E., Branco, R. B. F., Martinez, C. A. (2020). Elevated CO2 and warming change the nutrient status and use efficiency of Panicum maximum jacq. PLos One 15, e0223937. doi: 10.1371/journal.pone.0223937
Chance, B., Maehly, A. C. (1955). Assay of catalase and peroxidases. Methods Enzymol. 11, 764–775. doi: 10.1016/S0076-6879(55)02300-8
Chapagain, D., Baarsch, F., Schaeffer, M., D'haen, S. (2020). Climate change adaptation costs in developing countries: insights from existing estimates. Clim. Dev. 12, 934–942. doi: 10.1080/17565529.2020.1711698
Craine, J. M., Elmore, A. J., Olson, K. C., Tolleson, D. (2010). Climate change and cattle nutritional stress. Glob. Change Biol. 16, 2901–2911. doi: 10.1111/j.1365-2486.2009.02060.x
Dias, M. B. C., Costa, K. A. P., Severiano, E. C., Bilego, U., Lourival, V., Souza, W. F., et al. (2021). Cattle performance with Brachiaria and Panicum maximum forages in an integrated crop-livestock system. Afr. J. Range Forage Sci. 39, 230–243. doi: 10.2989/10220119.2021.1901311
Doheny-Adams, T., Hunt, L., Franks, P. J., Beerling, D. J., Gray, J. E. (2012). Genetic manipulation of stomatal density influences stomatal size, plant growth and tolerance to restricted water supply across a growth carbon dioxide gradient. Philos. Trans. R Soc. Lond. B Biol. Sci. 367, 547–555. doi: 10.1098/rstb.2011.0272
Dumont, B., Andueza, D., Niderkorn, V., Luscher, A., Porqueddu, C., Picon-Cochard, C. (2015). A meta-analysis of climate change effects on forage quality in grasslands: specificities of mountain and Mediterranean areas. Grass For. Sci. 70, 239–254. doi: 10.1111/gfs.12169
Embrapa (2012). “Capim mombaça,” in Embrapa gado de corte, v. 1, n. 1 (Belém, Brazil: Embrapa Amazônia Oriental), 2.
Embrapa (2014). Relatório de avaliação dos impactos das tecnologias geradas pela embrapa gado de corte - capim mombaça (Campo Grande, Brazil: Embrapa Gado de Corte), 1–25. 2014.
Engineer, C. B., Ghassemian, M., Anderson, J. C., Peck, S. C., Hu, H., Schroeder, J. I. (2014). Carbonic anhydrases, EPF2 and a novel protease mediate CO2 control of stomatal development. Nature 513, 246–250. doi: 10.1038/nature13452
Giannopolitis, C. N., Ries, S. K. (1977). Superoxide dismutase I occurrence in higher plants. Plant Physiol. 59, 309–314. doi: 10.1104/pp.59.2.309
Gray, J. E., Holroyd, G. H., van der Lee, F. M., Bahrami, A. R., Sijmons, P. C., Woodward, F. I., et al. (2000). The HIC signalling pathway links CO2 perception to stomatal development. Nature 408, 713–716. doi: 10.1038/35047071
Habermann, E., Contin, D. R., Afonso, L. F., Barosela, J. R., Costa, K. A. P., Viciedo, D. O., et al. (2022). Future warming will change the chemical composition and leaf blade structure of tropical C3 and C4 forage species depending on soil moisture levels. Sci. Total Environ. 821, 153342. doi: 10.1016/j.scitotenv.2022.153342
Habermann, E., Dias de Oliveira, E. A., Contin, D. R., Delvecchio, G., Viciedo, D., Moraes, M. A., et al. (2019c). Warming and water deficit impact leaf photosynthesis and decrease forage quality and digestibility of a C4 tropical grass. Physiol. Plant 165, 383–402. doi: 10.1111/ppl.12891
Habermann, E., Dias de Oliveira, E. A., Contin, D. R., San Martin, J. A. B., Curtarelli, L., Gonzalez-Meler, M. A., et al. (2019a). Stomatal development and conductance of a tropical forage legume are regulated by elevated [CO2] under moderate warming. Front. Plant Sci. 10. doi: 10.3389/fpls.2019.00609
Habermann, E., Dias de Oliveira, E. A., Delvecchio, G., Belisário, R., Barreto, R. F., Viciedo, D. O., et al. (2021). How does leaf physiological acclimation impact forage production and quality of a warmed managed pasture of stylosanthes capitata under different conditions of soil water availability? Sci. Total Environ. 759, 143505. doi: 10.1016/j.scitotenv.2020.143505
Habermann, E., San Martin, J. A. B., Contin, D. R., Bossan, V. P., Barboza, A., Braga, M. R., et al. (2019b). Increasing atmospheric CO2 and canopy temperature induces anatomical and physiological changes in leaves of the C4 forage species Panicum maximum. PLos One 14, 1–25. doi: 10.1371/journal.pone.0212506
Haudecoeur, E., Planamente, S., Cirou, A., Tannières, M., Shelp, B. J., Morera, S., et al. (2009). Proline antagonizes GABA-induced quenching of quorum-sensing in Agrobacterium tumefaciens. PNAS 106, 14587–14592. doi: 10.1073/pnas.080800510
Hayes, F., Mills, G., Jones, L., Abbott, J., Ashmore, M., Barnes, J., et al. (2016). Consistent ozone-induced decreases in pasture forage quality across several grassland types and consequences for UK lamb production. Sci. Total Environ. 543, 336–346. doi: 10.1016/j.scitotenv.2015.10.128
Heath, R. L., Packer, L. (1968). Photoperoxidation in isolated chloroplasts. i. kinetics and stoichiometry of fatty acid peroxidation. Arch. Biochem. Biophys. 125, 189–198. doi: 10.1016/0003-9861(68)90654-1
Hitchcock, A. S., Chase, A. (1910). “The north American species of panicum,” in Contributions from the united states national herbarium, vol. 15 (Washington, USA: Smithsonian Institute, United States National Museum).
Ilan, N., Moran, N., Schwartz, A. (1995). The role of potassium channels in the temperature control of stomatal aperture. Plant Physiol. 108, 1161–1170. doi: 10.1104/pp.108.3.1161
IPCC (2021). “Summary for policymakers,” in Climate change 2021: The physical science basis. contribution of working group I to the sixth assessment report of the intergovernmental panel on climate change. Ed. Masson-Delmotte, V. (Cambridge, United Kingdom and New York: Cambridge University Press).
IUSS (2015). World reference base for soil resources 2014, update 2015 international soil classification system for naming soils and creating legends for soil maps (Rome: FAO). World Soil Resources Reports No. 106.
Jägermeyr, J., Müller, C., Ruane, A. C., Elliott, J., Balkovic, J., Castillo, O., et al. (2021). Climate impacts on global agriculture emerge earlier in new generation of climate and crop models. Nat. Food 2, 873–885. doi: 10.1038/s43016-021-00400-y
Kimball, B. A. (2005). Theory and performance of an infrared heater for ecosystem warming. Glob. Change Biol. 11, 2041–2056. doi: 10.1111/j.1365-2486.2005.1028.x
Knapp, J. R., Laur, G. L., Vadas, P. A., Weiss, W. P., Tricarico, J. M. (2014). Invited review: Enteric methane in dairy cattle production: quantifying the opportunities and impact of reducing emissions. J. Dairy Sci. 97, 3231–3261. doi: 10.3168/jds.2013-7234
Leadley, P. W., Niklaus, P., Stocker, R., Körner, C. (1997). Screen-aided CO2 control (SACC): middle ground between FACE and open-top chambers. Acta Ecologica. 18, 207–219. doi: 10.1016/S1146-609X(97)80007-0
Leakey, A. D. B., Uribelarrea, M., Ainsworth, E. A., Naidu, S. L., Rogers, A., Ort, D. R., et al. (2006). Photosynthesis, productivity, and yield of maize are not affected by open-air elevation of CO2 concentration in the absence of drought. Plant Physiol. 140, 779–790. doi: 10.1104/pp.105.073957
Lee, M. A. (2018). A global comparison of the nutritive values of forage plants grown in contrasting environments. J. Plant Res. 131, 641–654. doi: 10.1007/s10265-018-1024-y
Lee, M. A., Davis, A. P., Chagunda, M. G. G., Manning, P. (2017). Forage quality declines with rising temperatures, with implications for livestock production and methane emissions. Biogeosciences 14, 1403–1417. doi: 10.5194/bg-14-1403-2017
Lichtenthaler, H. K., Wellburn, A. R. (1983). Determinations of total carotenoids and chlorophylls a and b of leaf extracts in different solvents. Biochem. Soc Trans. 11, 591–592. doi: 10.1042/bst0110591
Lin, J., Jach, M. E., Ceulemans, R. (2001). Stomatal density and needle anatomy of scots pine (Pinus sylvestris) are affected by elevated CO2. New Phytol. 150, 665–674. doi: 10.1046/j.1469-8137.2001.00124.x
Macháčová, K. (2010). Open top chamber and free air CO2 enrichment - approaches to investigate tree responses to elevated CO2. iForest 3, 102–105. doi: 10.3832/ifor0544-003
Markelz, R. J. C., Strellner, R. S., Leakey, A. D. B. (2011). Impairment of C4 photosynthesis by drought is exacerbated by limiting nitrogen and ameliorated by elevated [CO2] in maize. J. Exp. Bot. 62, 3235–3246. doi: 10.1093/jxb/err056
Mertens, D. R. (2002). Gravimetric determination of amylase-treated neutral detergent fiber in feeds with refluxing in beaker or crucibles: collaborative study. J. AOAC Int. 85, 1217–1240.
Miglietta, F., Hoosbeek, M. R., Foot, J., Gigon, F., Hassinen, A., Heijmans, M., et al. (1999). Spatial and temporal performance of the miniFACE (Free air CO2 enrichment) system on bog ecosystems in northern and central Europe. Environ. Monit. Assess. 66, 107–127. doi: 10.1023/A:1026495830251
Moyo, M., Nsahlai, I. (2021). Consequences of increases in ambient temperature and effect of climate type on digestibility of forages by ruminants: A meta-analysis in relation to global warming. Animals 11, 172. doi: 10.3390/ani11010172
Nahar, K., Hasanuzzaman, M., Ahamed, K. U., Hakeem, K. R., Ozturk, M., Fujita, M. (2015). “Plant responses and tolerance to high temperature stress: role of exogenous phytoprotectants,” in Crop production and global environmental issues. Ed. Hakeem, K. (Cham: Springer), 385–435.
Nakano, Y., Asada, K. (1981). Hydrogen peroxide is scavenged by ascorbate specific peroxidase in spinach chloroplasts. Plant Cell Physiol. 22, 867–880.
Negi, J., Hashimoto-Sugimoto, M., Kusumi, K., Iba, K. (2014). New approaches to the biology of stomatal guard cells. Plant Cell Physiol. 55, 241–250. doi: 10.1093/pcp/pct145
Nelson, J. A., Morgan, J. A., LeCain, D. R., Mosier, A. R., Milchunas, D. G., Parton, B. A. (2004). Elevated CO2 increases soil moisture and enhances plant water relations in a long-term field study in semi-arid shortgrass steppe of Colorado. Plant Soil 259, 169–179. doi: 10.1023/B:PLSO.0000020957.83641.62
Pritchard, S. G., Ju, Z., van Santen, E., Qiu, J., Weaver, D. B., Prior, S. A., et al. (2000). The influence of elevated CO2 on the activities of antioxidative enzymes in two soybean genotypes. Funct. Plant Biol. 27, 1061–1068. doi: 10.1071/PP99206
Raij, B., Cantarella, H., Quaggio, J., Furlani, A. (1997). Recomendações de adubação e calagem para o estado de são paulo. 2 ed (Campinas: Instituto Agronômico de Campinas (IAC).
Šigut, L., Holišovaí, P., Klem, K., Šprtovaí, M., Calfapietra, C., Marek, M. V., et al. (2015). Does long-term cultivation of saplings under elevated CO2 concentration influence their photosynthetic response to temperature? Ann. Bot. 116, 929–939. doi: 10.1093/aob/mcv043
Scholander, P. F., Hammel, H. T., Bradstreet, E. D., Hemmingsen, E. A. (1965). Sap pressure in plants: negative hydrostatic pressure can be measured in plants. Science 149, 920. doi: 10.1126/science.149.3687.920
Shabbaj, I. I., Madany, M. M. Y., Balkhyour, M. A., Tammar, A., AbdElgawad, H. (2022). CO2 enrichment differentially upregulated sugar, proline, and polyamine metabolism in young and old leaves of wheat and sorghum to mitigate indium oxide nanoparticles toxicity. Front. Plant Sci. 13. doi: 10.3389/fpls.2022.843771
Silva, D. J., Queiroz, A. C. (2002). Análise de alimentos: métodos químicos e biológicos. 1 ed (Viçosa: Editora UFV).
Smart, R. E., Bingham, G. E. (1974). Rapid estimates of relative water content. Plant Physiol. 53, 258–260. doi: 10.1104/pp.53.2.258
Spera, S. A., Winter, J. M., Partridge, T. F. (2020). Brazilian Maize yields negatively affected by climate after land clearing. Nat. Sustain. 3, 845–852. doi: 10.1038/s41893-020-0560-3
Szabados, L., Savoure, A. (2010). Proline: a multifunctional amino acid. Trends Plant Sci. v. 15 p, 89–97. doi: 10.1016/j.tplants.2009.11.009
Thornthwaite, C. W. (1948). An approach toward a rational classification of climate. Geogr. Rev. 38, 55–94. doi: 10.2307/210739
Tilley, J. M. A., Terry, R. A. (1963). A two-stage technique for the in vitro digestion of forage crops. Grass Forage Sci. 18, 104–111. doi: 10.1111/j.1365-2494.1963.tb00335.x
Uprety, D. C., Garg, S. C., Bisht, B. S., Maini, H. K., Dwivedi, N., Paswan, G., et al. (2006). Carbon dioxide enrichment technologies for crop response studies. J. Sci. Ind. Res. 65, 859–866.
Verbruggen, N., Hermans, C. (2008). Proline accumulation in plants: A review. Amino Acids 35, 753–759. doi: 10.1007/s00726-008-0061-6
Viciedo, D. O., Prado, R. M., Martinez, C. A., Habermann, E., Piccolo, M. C. (2019). Short-term warming and water stress affect Panicum maximum jacq. stoichiometric homeostasis and biomass production. Sci. Total Environ. 681 1, 267–274. doi: 10.1016/j.scitotenv.2019.05.108
Waghorn, G., Clark, D. (2004). Feeding value of pastures for ruminants. N. Z. Vet. J. 52, 332–341. doi: 10.1080/00480169.2004.36449
Walz (2019). “Imaging-PAM m-series chlorophyll fluorescence system,” in Instrument description and information for users (Effeltrich, Germany: Heinz Walz GmbH).
Warren, J. M., Norby, R. J., Wullschleger, S. D., Oren, R. (2011). Elevated CO2 enhances leaf senescence during extreme drought in a temperate forest. Tree Physiol. 31, 117–130. doi: 10.1093/treephys/tpr002
Wedow, J. M., Yendrek, C. R., Mello, T. R., Creste, S., Martinez, C. A., Ainsworth, E. A. (2019). Metabolite and transcript profiling of Guinea grass (Panicum maximum jacq) response to elevated [CO2] and temperature. Metabolomics 15, 51. doi: 10.1007/s11306-019-1511-8
Wollenberg, E., Richards, M., Smith, P., Havlík, P., Obersteiner, M., Tubiello, F. N., et al. (2016). Reducing emissions from agriculture to meet the 2 °C target. Glob. Change Biol. 22, 3859–3864. doi: 10.1111/gcb.13340
Wullschleger, S. D., Tschaplinski, T. J., Norby, R. J. (2002). Plant water relations at elevated CO2 — implications for water-limited environments. Plant Cell Environ. 25, 319–331. doi: 10.1046/j.1365-3040.2002.00796.x
Xu, M., Hu, J., Zhang, T., Wang, H., Zhu, X., Wang, J., et al. (2021). Specific responses of canopy conductance to environmental factors in a coniferous plantation in subtropical China ecol. Indic. 131, 108168. doi: 10.1016/j.ecolind.2021.108168
Xu, Z., Jiang, Y., Jia, B., Zhou, G. (2016). Elevated-CO2 response of stomata and its dependence on environmental factors. Front. Plant Sci. 7. doi: 10.3389/fpls.2016.00657
Xu, W., Yuan, W., Dong, W., Xia, J., Liu, D., Chen, Y. (2013). A meta-analysis of the response of soil moisture to experimental warming environ. Res. Lett. 8, 044027. doi: 10.1088/1748-9326/8/4/044027
Keywords: climate change, field conditions, grassland, soil moisture conservation, tropical ecosystem
Citation: Habermann E, Dias de Oliveira EA, Contin DR, Costa JVCP, Costa KAdP and Martinez CA (2022) Warming offsets the benefits of elevated CO2 in water relations while amplifies elevated CO2-induced reduction in forage nutritional value in the C4 grass Megathyrsus maximus. Front. Plant Sci. 13:1033953. doi: 10.3389/fpls.2022.1033953
Received: 01 September 2022; Accepted: 07 November 2022;
Published: 05 December 2022.
Edited by:
Jairo A. Palta, Commonwealth Scientific and Industrial Research Organisation (CSIRO), AustraliaReviewed by:
Adriana Grandis, University of São Paulo, BrazilCopyright © 2022 Habermann, Dias de Oliveira, Contin, Costa, Costa and Martinez. This is an open-access article distributed under the terms of the Creative Commons Attribution License (CC BY). The use, distribution or reproduction in other forums is permitted, provided the original author(s) and the copyright owner(s) are credited and that the original publication in this journal is cited, in accordance with accepted academic practice. No use, distribution or reproduction is permitted which does not comply with these terms.
*Correspondence: Carlos Alberto Martinez, Y2FybG9zYW1oQGZmY2xycC51c3AuYnI=
†ORCID: Carlos Alberto Martinez, orcid.org/0000-0002-0246-9481
Disclaimer: All claims expressed in this article are solely those of the authors and do not necessarily represent those of their affiliated organizations, or those of the publisher, the editors and the reviewers. Any product that may be evaluated in this article or claim that may be made by its manufacturer is not guaranteed or endorsed by the publisher.
Research integrity at Frontiers
Learn more about the work of our research integrity team to safeguard the quality of each article we publish.