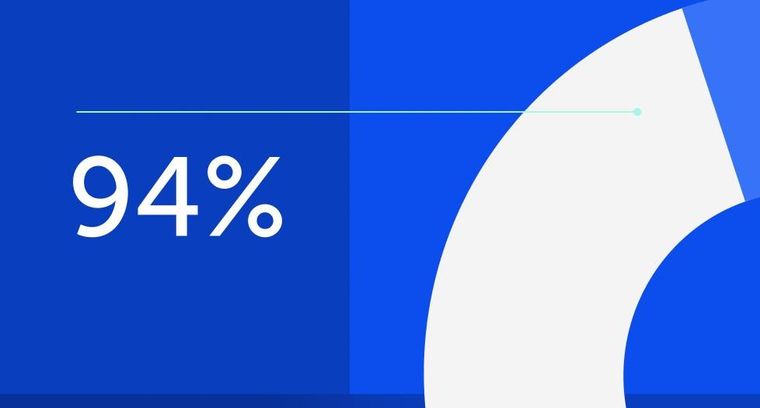
94% of researchers rate our articles as excellent or good
Learn more about the work of our research integrity team to safeguard the quality of each article we publish.
Find out more
REVIEW article
Front. Plant Sci., 29 November 2022
Sec. Crop and Product Physiology
Volume 13 - 2022 | https://doi.org/10.3389/fpls.2022.1032820
This article is part of the Research TopicImproving crop nutritional security for sustainable agriculture in the era of climate changeView all 12 articles
Rapid climate change caused by human activity is threatening global crop production and food security worldwide. In particular, the emergence of new infectious plant pathogens and the geographical expansion of plant disease incidence result in serious yield losses of major crops annually. Since climate change has accelerated recently and is expected to worsen in the future, we have reached an inflection point where comprehensive preparations to cope with the upcoming crisis can no longer be delayed. Development of new plant breeding technologies including site-directed nucleases offers the opportunity to mitigate the effects of the changing climate. Therefore, understanding the effects of climate change on plant innate immunity and identification of elite genes conferring disease resistance are crucial for the engineering of new crop cultivars and plant improvement strategies. Here, we summarize and discuss the effects of major environmental factors such as temperature, humidity, and carbon dioxide concentration on plant immunity systems. This review provides a strategy for securing crop-based nutrition against severe pathogen attacks in the era of climate change.
Climate change is a major factor in determining where humans can live on the planet under tolerable and safe conditions (Timmermann et al., 2022). Global warming due to environmental destruction and excessive burning of fossil fuels is creating adverse conditions for the continued survival of many plant and animal species and the wellness of the human population (Román-Palacios and Wiens, 2020). The crops that have made human settlement possible since the dawn of agriculture by providing a stable source of dietary calories are now suffering from the effects of climate change (Challinor et al., 2014; Rising and Devineni, 2020). Biotic stress factors such as pathogens and insect pests reduce crop yield and quality in agricultural settings (Savary et al., 2019; Savary and Willocquet, 2020). Indeed, damage to major crop yields is estimated to reach up to 40% globally (Oerke, 2006; Savary et al., 2012). In warmer and wetter environments more amenable to pathogen growth and spread, the damage they cause can be even more devastating (Velasquez et al., 2018). For example, bacterial blight caused by Xanthomonas oryzae pv. oryzae (Xoo) can decrease yield in rice (Oryza sativa) by up to 80% (Srinivasan and Gnanamanickam, 2005). Wheat blast caused by the fungus Magnaporthe oryzae Triticum can infect wheat (Triticum aestivum) and completely eradicate fields (Islam et al., 2020), as can banded leaf and sheath blight caused by Rhizoctonia solani in maize (Zea mays) (Haque et al., 2022). Moreover, the emergence of new pathogenic strains and the expansion of their effective damage zones due to climate change are two of the most serious threats to crop production and food security (Chaloner et al., 2021). Therefore, efficient strategies are urgently needed to reduce the impact of pathogens on crop growth and yield.
According to the disease triangle model, three factors are required for disease development: a susceptible host, a virulent pathogen, and a favorable environment (Scholthof, 2007). Of these, only plant-based strategies are available to affect one side of the triangle with current technologies. Indeed, the development of new crop cultivars conferring innate immunity will be essential for conservation of food resources. Plant breeding has traditionally been performed through laborious and time-consuming genetic crosses to introduce superior alleles into a given background (Lusser et al., 2012). However, biotechnological innovations now offer eight new plant breeding technologies (NPBTs): site-directed nucleases (SDNs), oligonucleotide-directed mutagenesis, cisgenesis and intragenesis, RNA-dependent DNA methylation, grafting, reverse breeding, Agrobacterium-mediated infiltration, and synthetic genomics (Lusser et al., 2011). Among them, SDNs are the most widely used NPBT for a broad range of crops. In particular, development of the clustered regularly interspaced short palindromic repeats (CRISPR)/CRISPR-associated nuclease 9 (Cas9) system has ushered in a new era of crop improvement (Son and Park, 2022). Therefore, understanding the molecular mechanisms and identifying novel genes conferring desired traits are essential for their targeting by NPBTs in plant breeding.
Plants have evolved varied stress responses and defense mechanisms to overcome adverse environmental conditions, about which we have gained a wealth of knowledge thanks to the efforts of countless scientists. Nevertheless, how climate change affects the molecular mechanisms related to plant immunity against pathogens is largely unknown. Luckily, this knowledge gap is beginning to be filled. In this review, we give an overview and discuss the negative effects of temperature, humidity, and carbon dioxide (CO2) concentration on plant defense mechanisms to better understand how to design mitigation strategies.
Plants employ two important immune systems known as pathogen-associated molecular pattern (PAMP)-triggered immunity (PTI) and effector-triggered immunity (ETI) to perceive and respond to pathogen attacks (Thomma et al., 2011). PTI is activated mainly by plasma membrane–localized extracellular pattern recognition receptors (PRRs) that can recognize conserved PAMPs (Monaghan and Zipfel, 2012). For example, recognition of the 22–amino acid region of bacterial flagellin (flg22) by the leucine-rich repeat receptor kinase (LRR-RK) FLAGELLIN SENSING 2 (FLS2) at the plasma membrane leads to formation of a heteromer between FLS2 and BRASSINOSTEROID INSENSITIVE-ASSOCIATED KINASE 1 (BAK1), a member of the LRR receptor-like kinase (LRR-RLK) and also known as SOMATIC EMBRYOGENESIS RECEPTOR-LIKE KINASE 3 (SERK3) (Chinchilla et al., 2007). The FLS2/BAK1 complex phosphorylates the receptor-like cytoplasmic kinase BOTRYTIS-INDUCED KINASE 1 (BIK1) and mitogen-activated protein kinase (MAPK) cascade to activate the downstream signaling pathway, resulting in expression of PTI-related genes (Wang et al., 2020b). Similarly, perception of a highly conserved epitope of bacterial translation elongation factor Tu (EF-Tu) by the LRR-RK EF-Tu RECEPTOR (EFR) also results in PTI activation through heteromerization with BAK1 and phosphorylation of BIK1 (Lal et al., 2018). Moreover, the recognition of plant-derived damage-associated molecular patterns (DAMPs) and phytocytokines by LRR-RKs/RLKs is important for PTI (Hou et al., 2021; Tanaka and Heil, 2021). PTI acts as a basal defense mechanism against various types of pathogens through defense responses that include the induction of defense gene expression, reactive oxygen species (ROS) production, callose deposition, and accumulation of antimicrobial secondary metabolites (Naveed et al., 2020).
ETI is triggered following the recognition by intracellular receptor resistance (R) proteins of specific pathogen effectors that can neutralize the plant immune system in the cytoplasm (Chisholm et al., 2006; Jones and Dangl, 2006). ETI activates a prolonged and robust resistance response and rapid localized programmed cell death known as the hypersensitive response (HR) (Coll et al., 2011). Most R proteins are nucleotide-binding leucine-rich repeat proteins (NLRs) that can be classified into three groups based on their N terminus domain: Toll/interleukin-1 receptor (TIR), coiled-coil (CC), and RESISTANCE TO POWDERY MILDEW 8 (RPW8)-type CC (CCR) domain (Monteiro and Nishimura, 2018). The ETI signal triggered by TIR-NLRs (TNLs) relies on the three acyl hydrolases ENHANCED DISEASE SUSCEPTIBILITY 1 (EDS1), PHYTOALEXIN DEFICIENT 4 (PAD4), and SENESCENCE-ASSOCIATED GENE 101 (SAG101) (Wiermer et al., 2005). EDS1 interacts directly with PAD4 or SAG101 to form exclusive heterodimers, each with distinct functions in immunity (Wagner et al., 2013; Lapin et al., 2020). It was recently revealed that helper CCR-NLRs such as ACTIVATED DISEASE RESISTANCE 1 (ADR1) and N REQUIREMENT GENE 1 (NRG1) are required for the activation of the EDS1 complex and TNL defense signaling (i.e., EDS1–PAD4–ADR1 and EDS1–SAG101–NRG1) (Pruitt et al., 2021; Sun et al., 2021). The EDS1 pathway is involved not only in ETI but also in basal immunity and promotes salicylic acid (SA) biosynthesis and signaling (Cui et al., 2017). Therefore, EDS1 signaling plays a critical role in SA-dependent and -independent resistance. For CC-NLRs (CNLs), the plasma membrane-localized integrin-like protein NON-RACE SPECIFIC DISEASE RESISTANCE 1 (NDR1) appears to function downstream of CNLs, although several do not require NDR1 to activate ETI (van Wersch et al., 2020). Since NDR1 acts upstream of SA biosynthesis and signaling, it is also involved in SA-dependent resistance (Shapiro and Zhang, 2001).
Another plant immune response is referred to as quantitative disease resistance (QDR), which is characterized by a continuous distribution of resistance phenotypes—from highly sensitive to highly resistant—within a population (Poland et al., 2009). QDR is typically partial resistance conferred by multiple small-effect loci, while qualitative disease resistance, also referred as ETI, is complete resistance conferred by a single large-effect gene (French et al., 2016). Since multiple genes are involved in QDR, it is important in the context of the evolutionary pressure imposed by pathogens and confers broad-spectrum resistance to a wide range of pathogens including biotrophic and necrotrophic pathogens (Anderson et al., 2010; French et al., 2016). Most loci identified as quantitative trait loci for QDR are associated with the biosynthesis of the cell wall and defense compounds, thus extending beyond simple pathogen perception (Corwin and Kliebenstein, 2017).
Phytohormones participate in and control PTI and ETI. In particular, the three phytohormones SA, jasmonic acid (JA), and ethylene (ET) play critical roles in plant immunity. SA contributes significantly to innate immunity against biotrophic pathogens by evoking local and systemic resistance, whereas JA/ET play critical roles in plant resistance to necrotrophic pathogens (Glazebrook, 2005; Li et al., 2019). The SA and JA/ET defense signals can be antagonistic or synergistic (Tsuda and Katagiri, 2010). Abscisic acid (ABA) is also important for innate immunity. ABA interacts with various phytohormones during defense responses (Lee and Luan, 2012; Pieterse et al., 2012). For example, ABA suppresses SA-dependent immunity, leading to greater susceptibility against various pathogens (Berens et al., 2019). However, ABA can also increase plant disease resistance due to closure of stomata which constitutes one of the main entry routes for pathogens (Ton Mauch‐Mani, 2004; Melotto et al., 2006; Flors et al., 2008). In response to the stimulus, ABA is primarily biosynthesized in vascular tissues and accumulates in guard cells through ABA transporters (e.g., ATP-binding cassette transporter G [ABCG]) (Merilo et al., 2015). In guard cells, ABA binds to its cognate receptor from the pyrabactin resistance 1/pyrabactin resistance 1-like/regulatory components of ABA receptors (PYR/PYL/RCAR) family, leading to the inactivation of type 2C protein phosphatases (PP2Cs). The alleviation of PP2C-mediated repression of SUCROSE NON-FERMENTING 1 (SNF1)-related protein kinase 2s (SnRK2s) results in activation of the downstream ABA signaling cascade (Hsu et al., 2021). For example, the PP2Cs ABA INSENSITIVE 1 (ABI1) and ABI2 inactivate OPEN STOMATA 1 (OST1), also known as SnRK2.6, thus preventing the phosphorylation of SLOW ANION CHANNEL 1 (SLAC1), which releases anions for stomatal closure. However, perception of flg22 by PRRs increases ABA levels in guard cells to inactivate ABIs, and it results in rapid stomatal closure through the activation of the OST1/SnRK2.6–SLAC1 module (Guzel Deger et al., 2015). Therefore, ABA promotes stomatal closure and prevents pathogen entry into the host plant.
ROS signaling is also important for plant immunity. ROS are highly oxidative agents, but they also act as signaling molecules that regulate biotic stress responses (e.g., systemic acquired resistance [SAR] and cell death) (Waszczak et al., 2018). ROS are generated via metabolic and stress signaling pathways. Metabolic ROS are produced in several intracellular compartments (e.g., chloroplast, mitochondria, peroxisomes, and apoplast) during photosynthesis and photorespiration, while signaling ROS are produced mainly by plant NADPH oxidases, mostly from members of the plasma membrane–localized respiratory burst oxidase homolog (RBOH) family (Kangasjärvi et al., 2012; Chapman et al., 2019). Pathogen recognition is accompanied by ROS production through both the metabolic and stress signaling pathways. Recognition of PAMPs by PRRs induces an initial oxidative burst that activates plant basal defenses within the infected cells; effector perception by R proteins then promotes a second oxidative burst that results in HR (Nanda et al., 2010; Torres, 2010). Therefore, ROS play a key role linking pathogen perception and plant defense responses.
However, these various plant defense systems may be adversely affected significantly by climate change, as discussed below.
Environmental factors influence not only pathogenicity but also plant disease resistance (Elad and Pertot, 2014). Temperature is perhaps the most studied climate factor modulating plant–pathogen interactions. Higher average temperatures brought upon by climate change can increase the pathogenicity of phytopathogens by raising their virulence, active geographical regions, fitness, reproduction period/rate, and epidemic risks (Agrios, 2005; Deutsch et al., 2008; Caffarra et al., 2012; Vaumourin and Laine, 2018). Temperature is also one of the most important environmental factors that shapes plant immunity against bacteria, fungi, viruses, and insects (Garrett et al., 2006). Since different host–pathogen interactions behave differently over different temperature ranges, higher temperatures will sometimes work in favor of plant immunity. In many cases though, higher temperature will benefit the pathogen to the detriment of the host (Desaint et al., 2021).
In Arabidopsis (Arabidopsis thaliana), higher temperature increases early PTI signaling (through BIK1 and MAPKs) and decreases the occupancy of nucleosomes containing the histone variant H2A.Z, which modulates the plant transcriptome in response to changes in temperature (Kumar and Wigge, 2010; Cheng et al., 2013). Moderately high temperatures (23°C–32°C) will therefore activate PTI-dependent gene expression at the expense of ETI (Cheng et al., 2013). Cysteine-rich receptor-like kinases (CRKs) are one of the largest RLK subfamilies that recognizes pathogens and activates downstream signaling cascades. Recently, Wang et al. identified a CRK from wheat cultivar ‘XY 6’ conferring high-temperature seedling-plant resistance (Wang et al., 2021). The expression level of this gene, TaCRK10, was induced significantly by infection with the fungal pathogen Puccinia striiformis f. sp. tritici causing strip rust at high temperature. TaCRK10 was shown to directly phosphorylate histone H2A in wheat (TaH2A.1) and activate the SA signaling pathway, resulting in enhanced high-temperature seedling-plant resistance to P. striiformis f. sp. tritici (Wang et al., 2021). However, several studies have also indicated that PTI can be compromised at high temperature upon inhibition of flg22- and SA-induced defense responses (Rasmussen et al., 2013; Huot et al., 2017; Janda et al., 2019). Therefore, further studies are needed to understand the effect of temperature on PTI in detail.
Unlike PTI, much work has shown that high temperature decreases immunity evoked by ETI and QDR; this topic was well covered by a previous review (Desaint et al., 2021). Therefore, we focus here on recent important discoveries that illustrate how plant defense mechanisms are affected by high temperature.
Disruptions of NLR- and SA-mediated defense signaling by high temperature are thought to be the main reason behind diminished plant innate immunity against pathogens under these conditions. In Arabidopsis, the photoreceptor phytochrome B (phyB) also acts as a thermosensor, whereby far-red light and high temperatures lead to its inactivation (Jung et al., 2016; Legris et al., 2016). DE-ETIOLATED 1 (DET1) and CONSTITUTIVELY PHOTOMORPHOGENIC 1 (COP1), which are two key negative regulators of photomorphogenesis, promote the transcription of PHYTOCHROME INTERACTION FACTOR 4 (PIF4), which encodes a basic-helix-loop-helix (bHLH) transcription factor acting as a positive regulator of growth and negative regulator of immunity (Gangappa et al., 2017; Gangappa and Kumar, 2018). phyB inhibits COP1 and PIF4 to modulate the trade-off between growth and defense. However, inactivation of phyB by high temperature results in the activation of the DET1/COP1–PIF4 module. As a result, PIF4 represses the expression of SUPPRESSOR OF NPR1-1, CONSTITUTIVE 1 (SNC1), which encodes a TNL initiating ETI through the EDS1-PAD4 signaling pathway at high temperature (Gangappa et al., 2017). Since SNC1 and EDS1 play a critical role in plant defense responses such as SA biosynthesis (Zhang et al., 2003; García et al., 2010), the inhibition of SNC1 expression at high temperature also significantly hinders SA-dependent resistance. Moreover, the SUMO E3 ligase SIZ1 (SAP and MIZ1 DOMAIN-CONTAINING LIGASE1) not only inhibits SNC1-dependent immune response but also enhances COP1 function at elevated ambient temperature (Hammoudi et al., 2018). Therefore, the activation of negative regulators (e.g., PIF4 and SIZ1) of SNC1 lead to impaired ETI and SA-dependent immunity. Recently, the transcription factor bHLH059 was identified as a temperature-responsive regulator for SA-dependent immunity acting independently of PIF4 (Bruessow et al., 2021). Relative bHLH059 transcript level increased at 22°C compared to 16°C in Arabidopsis ecotype Columbia (Col-0). Total SA contents and resistance to Pseudomonas syringae pv. tomato (Pst) DC3000 decreased at 22°C relative to 16°C in Col-0, but remained similar in the bhlh59 mutant regardless of ambient temperature. Moreover, bHLH059 has the potential to be a negative regulator involved in a defense hub associated with multiple NLRs (Mukhtar et al., 2011), hinting at a new mechanism for the temperature-mediated vulnerability of plant immune responses that should be explored in more detail.
SA is major defense phytohormone involved in PTI, ETI, and SAR; importantly, SA-dependent immunity is repressed by high temperature (Velásquez et al., 2018; Zhang and Li, 2019; Castroverde and Dina, 2021), whereas JA/ET defense signaling are enhanced under elevated temperature (Havko et al., 2020; Huang et al., 2021a). Therefore, any susceptibility to temperature in the context of plant disease resistance is mainly associated with SA signaling. SA is synthesized through the isochorismate synthase (ICS) and phenylalanine ammonia-lyase (PAL) pathways in plants (Lefevere et al., 2020). Especially, pathogen-induced SA production takes place in chloroplasts, from which it is exported to the cytoplasm via the SA transporter EDS5 (Serrano et al., 2013). SA activates NONEXPRESSOR OF PATHOGENESIS-RELATED GENES 1 (NPR1), the master regulator of SA signaling in the cytosol, resulting in the nuclear translocation of NPR1 to induce the expression of pathogenesis-related (PR) genes conferring disease resistance and SAR (Backer et al., 2019). Moreover, although ETI activates SA signaling, SA and NPR1 repress ETI-induced cell death via the formation of SA-induced NPR1 condensates to promote the degradation of proteins (e.g., NLRs, EDS1, WRKY54, and WRKY70) involved in HR (Zavaliev et al., 2020).
Huot et al. showed that inhibition of ICS1, which is also called SALICYLIC ACID-INDUCTION DEFICIENT 2 (SID2), under high-temperature conditions raised the susceptibility of Arabidopsis to Pst DC3000 due to the loss of SA biosynthesis and SA defense signaling (Huot et al., 2017). Furthermore, Arabidopsis disease resistance to Pst DC3000 increased at low temperature due to greater SA signaling that can itself be repressed by JA/ET defense signals (Li et al., 2020). However, the molecular mechanisms determining the temperature sensitivity of the SA defense signaling pathway were unknown.
Recently, two groups demonstrated different mechanisms by which the SA-mediated immune system is modulated under high temperature (Figure 1). Kim et al. showed that the expression of SA response genes is decreased under elevated temperature in various dicot (e.g., Arabidopsis, rapeseed [Brassica napus], tobacco [Nicotiana tabacum], and tomato [Solanum lycopersicum]) and monocot (rice) plants, with the downregulation of CALMODULIN BINDING PROTEIN 60g (CBP60g) being key for the temperature vulnerability of SA defense signaling in Arabidopsis (Kim et al., 2022). GUANYLATE BINDING PROTEIN-LIKE GTPase 3 (GBPL3) binds to the promoter region of genes involved in the plant immune system and recruits the Mediator complex and RNA polymerase II to form GBPL defense–activated condensates (GDACs) (Huang et al., 2021b). The recruitment of GBPL3 and the formation of the GDAC at the CBP60g and SYSTEMIC ACQUIRED RESISTANCE DEFICIENT 1 (SARD1) loci, which have partially redundant functions, were necessary for their transcription, and these were attenuated by heat stress (Kim et al., 2022). Therefore, the expression of various genes (e.g., ICS1, EDS1, and PAD4) that would normally induce TNL-mediated ETI and SA biosynthesis downstream of CBP60g and SARD1 was suppressed under elevated temperature. However, and surprisingly, optimized CBP60g expression was sufficient to restore SA accumulation and plant immune responses at high temperature without growth or developmental penalty (Kim et al., 2022). Another group unraveled the molecular mechanism explaining the temperature vulnerability of CNLs and SA defense signaling in Arabidopsis (Samaradivakara et al., 2022). RESISTANCE TO P. SYRINGAE PV. MACULICOLA 1 (RPM1) and RESISTANCE TO P. SYRINGAE 2 (RPS2) encode two CNLs that recognize type III bacterial effectors indirectly through RPM1-INTERACTING PROTEIN 4 (RIN4) (Mackey et al., 2002; Mackey et al., 2003). P. syringae bacterial effectors such as AvrRpm1 and AvrB activate RPM1-mediated ETI through hyperphosphorylation of RIN4, while AvrRpt2 activates RPS2-mediated ETI via the degradation of RIN4 (Axtell and Staskawicz, 2003; Zhao et al., 2021). Plasma membrane–localized NDR1 interacts with RIN4 and is required for the activation of RPS2-based ETI in response to AvrRpt2 (Belkhadir et al., 2004; Coppinger et al., 2004; Day et al., 2006). Samaradivakara et al. showed that overexpression of NDR1 rescues the transcript levels of RPS2 and SA-associated genes including those of ICS1 and CBP60g, which are repressed by high temperature, thus resulting in enhanced resistance to Pst DC3000 by maintaining ETI and SA defense signaling under elevated temperature (29°C) (Samaradivakara et al., 2022). In wheat, CNLs such as TaRPM1 and TaRPS2 also positively regulate disease resistance to P. striiformis f. sp. tritici at high temperature through the SA signaling pathway (Wang et al., 2020a; Hu et al., 2021a).
Figure 1 Molecular mechanisms demonstrating the negative effect of high temperature on SA-dependent immunity and ETI. In Arabidopsis, the induction of CALMODULIN BINDING PROTEIN 60g (CBP60g) and NON-RACE SPECIFIC DISEASE RESISTANCE 1 (NDR1) is necessary for innate immunity against Pst DC3000. However, under high temperature, the formation of guanylate binding protein-like GTPase (GBPL) defense-activated condensate (GDAC), consisting of GBPL3, Mediator, and RNA polymerase II, at the CBP60g loci (Kim et al., 2022) and the expression of NDR1 which can increase the transcript levels of RESISTANCE TO P. SYRINGAE 2 (RPS2) and SA-associated genes (Samaradivakara et al., 2022) are repressed significantly, resulting in temperature vulnerability of SA-dependent immunity and ETI.
A recent study revealed that the phytohormone cytokinin (CK) also plays an important role in plant immunity at high temperatures (Yang et al., 2022). The trade-off between growth and defense modulated by CK can result in opposite effects on plant–pathogen interactions (Choi et al., 2011). Exogenous and endogenous CK both enhance plant resistance against biotrophic pathogens through SA-dependent and -independent immune responses, therefore exerting a potentiation (or priming) defense response activated upon pathogen attack (Conrath et al., 2015; Albrecht and Argueso, 2017). Although CK displays a synergistic effect with SA, increased SA levels can inhibit CK signaling via a negative feedback (Argueso et al., 2012). In addition, high concentrations of CK enhance disease resistance against biotrophic oomycetes in Arabidopsis, while low concentrations raise susceptibility (Argueso et al., 2012). CK can also increase susceptibility to pathogens not only by inhibiting the plant immune system (i.e., PTI and ROS) but also by establishing source-sink relationships (Albrecht and Argueso, 2017; McIntyre et al., 2021). In pepper (Capsicum annuum), Yang et al. showed that infection with Ralstonia solanacearum, a hemibiotrophic pathogen causing bacterial wilt, activates SA signaling at an early stage and JA signaling at a later stage in roots at ambient temperature, but these responses are both impaired at high temperature (Yang et al., 2022). Instead, isopentenyltransferase (IPT) genes, including CaIPT5, encoding a critical enzyme in cytokinin biosynthesis, were upregulated by R. solanacearum infection under high temperature. Surprisingly, exogenous treatment with trans-zeatin (tZ), the bioactive CK, significantly enhanced disease resistance to R. solanacearum in pepper, tomato, and tobacco (Nicotiana benthamiana) under high temperature, while SA and JA did not (Yang et al., 2022). Moreover, the authors suggested that CK triggers chromatin remodeling, resulting in the upregulation of genes encoding glutathione S‐transferase (e.g., CaPRP1 and CaMgst3) and downregulation of genes involved in SA and JA signaling (e.g., CaSTH2 and CaDEF1 (Yang et al., 2022).
Recently, the molecular mechanisms by which high temperature affects the calcium ion (Ca2+)–mediated immune system have also been reported. Ca2+ is an important second messenger modulating various signaling pathways, including the plant immune response (Yang and Poovaiah, 2003). Biotic/abiotic stresses increase Ca2+ levels in plant cells; Ca2+ then binds to calcium-binding proteins (CBPs) and Ca2+ sensors (e.g., calmodulin [CaM], calmodulin-like proteins [CMLs], calcineurin B-like proteins [CBLs], and calcium-dependent protein kinases CDPKs]) (Bose et al., 2011). The Ca2+/CBP complex activates Ca2+ signaling by regulating the activity of signaling components such as kinases and transcription factors (Iqbal et al., 2020; Junho et al., 2020; Ma et al., 2020). Arabidopsis SIGNAL RESPONSIVE 1 (AtSR1), also known as CALMODULIN-BINDING TRANSCRIPTION ACTIVATOR 3 (CAMTA3), plays a central role in Ca2+ signaling–mediated immunity (Yuan et al., 2021a). AtSR1 acts as a negative regulator of the plant immune response by decreasing the expression of genes involved in ETI and/or SA signaling (e.g., EDS1, NDR1, CBP60g, SARD1, and NPR1) directly or indirectly (Du et al., 2009; Nie et al., 2012; Sun et al., 2020; Yuan et al., 2021b). Recently, Yuan and Poovaiah showed that the Ca2+ influx induced by Pst DC3000 is blocked in Arabidopsis at high temperature (30°C) compared to ambient temperature (18°C). In addition, the susceptibility to Pst DC3000 was reduced in the atsr1 mutant plant compared to the wild type at both 18°C and 30°C (Yuan and Poovaiah, 2022). Moreover, the authors suggested that AtSR1 increases plant vulnerability to temperature by acting on stomatal and apoplastic immunity in an SA-dependent manner. In pepper, the expression of the WRKY transcription factor gene CaWRKY40 is induced by Ralstonia solanacearum infection, high temperature, and major defense phytohormones (e.g., SA, JA, and ET), and CaWRKY40 enhances both R. solanacearum resistance and heat tolerance (DANG et al., 2013). CaWRKY40 forms positive feedback loops with CaWRKY6, BASIC LEUCINE ZIPPER 63 (CabZIP63), and CaCDPK15, all positive regulators of resistance against R. solanacearum and/or heat stress tolerance (Cai et al., 2015; Shen et al., 2016a; Shen et al., 2016b). Recently, two signaling components controlled by CaWRKY40 were identified as positive and negative regulators of R. solanacearum resistance, respectively. CaCBL1 contributes to disease resistance against R. solanacearum at high temperature and participates in the positive feedback loop with CaWRKY40 (Shen et al., 2020). However, pepper MILDEW-RESISTANCE LOCUS O5 (CaMLO5) has the opposite function in plant immunity and heat resistance (Yang et al., 2020). CaWRKY40 induces the expression of CaMLO5 at high temperature, while CaWRKY40 represses it after R. solanacearum inoculation. CaMLO5 increases tolerance to heat stress but reduces the plant immune response against R. solanacearum. Moreover, the NAM/ATAF/CUC (NAC) transcription factor CaNAC2c was recently identified as being involved in temperature-responsive immunity (Cai et al., 2021). Expression of CaNAC2c was induced by both high temperature and R. solanacearum inoculation, resulting in positive effects on both thermotolerance and resistance against R. solanacearum but negative effects on pepper growth. CaNAC2c modulated the thermotolerance/immunity trade-off through differential and context-specific interactions with HEAT SHOCK PROTEIN 70 (CaHSP70) and CaNAC029. However, CaNAC2c/CaNAC029-mediated R. solanacearum resistance was impaired by ABA at high temperature, suggesting that the observed thermotolerance/immunity trade-off might be modulated by an antagonistic interaction between ABA and JA signaling (Cai et al., 2021).
Along with temperature, humidity is an influential environmental factor during plant–pathogen interactions. In general, high humidity conditions (e.g., rainfall, high atmospheric humidity, and high soil moisture) are favorable for plant infections not only by phyllosphere pathogens but also by rhizosphere pathogens. Indeed, high humidity increases the incidence of bacterial disease and the potential threat to yield in various crops (Xin et al., 2016). In fact, humidity can be more important than temperature in predicting fungal disease outbreaks (Romero et al., 2021). Since air can maintain more water vapor at high temperature, climate change is frequently accompanied by high humidity. Therefore, understanding the effect of humidity on plant immune mechanisms will be important for ensuring food security.
By far, the main target of humidity affecting plant immunity is associated with stomatal control. Stomata consist of two guard cells that play a central role in modulating water transpiration and gas exchange between the plant and the atmosphere to balance the needs of photosynthesis while minimizing drought stress. Therefore, stomatal movements are tightly regulated in response to various environmental stimuli (e.g., humidity and CO2) (Driesen et al., 2020). However, stomata also offer convenient portals through which pathogens can penetrate inner leaf tissues. To mitigate this threat, plants have developed sophisticated signaling networks conferring so-called stomatal immunity (Arnaud and Hwang, 2015; Murata et al., 2015). Guard cells recognize various PAMPs, resulting in PAMP-triggered stomatal closure through the activation of downstream signaling components (Figure 2A). However, according to a coevolutionary model between plants and their pathogens known as the zigzag model, some adapted pathogens have developed phytotoxins (e.g., coronatine and syringolin A) and effectors (e.g., avirulence protein B [AvrB], hrp-dependent outer protein F2 [HopF2], HopM1, HopX1, and HopZ1) to overcome stomatal immunity and use open stomata as their entry point into the leaf apoplast space (Melotto et al., 2017). Recently, Lie et al. also revealed that Xanthomonas oryzae pv. oryzicola (Xoc) secretes the bacterial effector AvrRxo1 to impair stomatal immunity by inducing the degradation of rice PYRIDOXAL PHOSPHATE SYNTHASE 1 (OsPDX1) involved in ABA biosynthesis (Liu et al., 2022a). Mechanisms of immunity by stomatal closure and their relationship with humidity have been covered in previous reviews (Melotto et al., 2017; Aung et al., 2018). Notably, after pathogens invade internal plant tissues, stomatal closure can support conditions of apoplast hydration auspicious for pathogen colonization. Therefore, we focus here on the most recent mechanisms regulating stomatal conductance after pathogen entry.
Figure 2 Stomatal immunity restricting pathogen entry or water soaking. (A) Pattern recognition receptors (PRRs)-triggered stomatal immunity. Recognition of pathogen-associated molecular patterns (PAMPs) by PRRs in guard cells promotes stomatal closure to prevent pathogen entry through activation of various signaling pathways such as ABA, SA, ROS, and Ca2+ (Arnaud and Hwang, 2015; Murata et al., 2015). (B) Stomatal immunity preventing water soaking. After pathogens invade internal plant tissues, stomatal closure can confer apoplast hydration inducing pathogen colonization. To prevent it, the secreted peptides SMALL PHYTOCYTOKINES REGULATING DEFENSE AND WATER LOSS (SCREWs) and the cognate receptor kinase PLANT SCREW UNRESPONSIVE RECEPTOR (NUT) are induced in Arabidopsis. Recognition of SCREWs by NUT increases the activity of the protein phosphatases type 2C (PP2Cs) such as ABA INSENSITIVE 1 (ABI1) and ABI2, and it results in stomatal reopening through inhibition of OST1/SnRK2.6-SLAC1 module (Liu et al., 2022b).
Since water is essential for the survival of pathogens as well as plants, pathogens have to work hard to obtain water when inside their host plants (Beattie, 2016). Water soaking is a common disease symptom visible as leaf spots caused by virulent bacterial pathogens (Davis et al., 1991; Reimers and Leach, 1991). Bacterial pathogens (e.g., Pst DC3000) induce water soaking to establish a favorable colonization milieu by using their effectors (e.g., WtsE, AvrHah1, HopM1, and AvrE1) (Ham et al., 2006; Schornack et al., 2008; Xin et al., 2016). For instance, Xin et al. identified two effectors (HopM1 and AvrE1) that induce water soaking in Arabidopsis and demonstrated the molecular mechanism by which HopM1 promotes apoplast hydration for bacterial proliferation (Xin et al., 2016). Arabidopsis HopM1 INTERACTOR 7 (AtMIN7), which is an ADP ribosylation factor–guanine nucleotide exchange factor (ARF-GEF) localized to the trans-Golgi-network/early endosome and involved in vesicle trafficking, is identified as a binding partner of HopM1 during a yeast two-hybrid (Y2H) screen and confirmed by pull-down assay (Nomura et al., 2006). AtMIN7 contributes to PTI and ETI, and the Pst DC3000 effector HopM1 induces its degradation through the host 26S proteasome to suppress plant innate immunity (Nomura et al., 2011). Since AtMIN7 also plays a critical role in limiting fluid loss from plant cells, HopM1-mediated AtMIN7 degradation results in apoplast hydration and provides the favorable water condition needed for Pst DC3000 colonization; notably, high ambient humidity is required for water soaking (Beattie, 2016; Xin et al., 2016). Moreover, HopM1 and AvrE1 increase the expression of ABA-associated genes through transcriptome reprogramming and by raising ABA contents in guard cells (Roussin-Léveillée et al., 2022). The guard cell–specific ABA transporter ABCG40 is necessary for HopM1-mediated water soaking (Roussin-Léveillée et al., 2022), while AvrE1 activates ABA signaling through the inhibition of type one protein phosphatases (TOPPs), thereby suppressing SnRK2s (Hu et al., 2022). Therefore, Pst DC3000 utilizes HopM1 and AvrE1 to activate ABA signaling, inducing stomatal closure for water soaking after having invaded the plant inner space.
To prevent water soaking, plants promote stomatal reopening to establish a drier apoplast environment in pathogen-infected cells (Figure 2B). In rice, the osaba1 mutant provided genetic evidence that increased stomatal conductance can enhance disease resistance to Xoo (Zhang et al., 2019). OsWRKY114 negatively regulated stomatal closure and conferred innate immunity against Xoo by repressing ABA signaling (Son et al., 2022; Song et al., 2022). Finally, in Arabidopsis, Lie et al. elucidated the molecular mechanism of stomatal immunity by which stomata reopen following effector-triggered stomatal closure (Liu et al., 2022b). They identified a class of small peptides, named the SMALL PHYTOCYTOKINES REGULATING DEFENSE AND WATER LOSS (SCREWs), and their receptor, the PLANT SCREW UNRESPONSIVE RECEPTOR (NUT), a member of the LRR-RK family. Flg22 treatment increases the expression of SCREWs and NUT, and recognition of SCREWs by NUT promotes the heterodimerization of NUT with BAK1. The NUT/BAK1 complex phosphorylates and enhances the phosphatase activity of ABI1 and ABI2, thus inhibiting the OST1/SnRK2.6-SLAC1 module whose activity promotes stomatal closure. As a result, plants can increase stomatal conductance to prevent water soaking through apoplast dehydration.
Since the industrial revolution in the second half of the 18th century, the concentration of atmospheric CO2 has begun to increase at an alarming rate. The Mauna Loa Observatory forecasts that the 2022 annual average CO2 concentration will be 418.3 ± 0.5 parts per million (ppm). This trend is expected to continue and reach 730–1000 ppm by the end of the 21st century (Alley et al., 2007). Elevated CO2 levels can increase the yield of C3 plants by enhancing photosynthesis, but will not benefit C4 plants (Long et al., 2006). High CO2 levels will also affect plant–pathogen interactions. However, the effects of CO2 concentrations on plant defense mechanisms depend on specific plant–pathogen interactions and are complex (Noctor and Mhamdi, 2017). Moreover, the detailed underlying molecular mechanisms are not yet well known. Therefore, we provide below an overview of the best-documented effects of high CO2 on plant defense mechanisms related to stomata and photorespiration.
Like humidity, atmospheric CO2 concentrations control stomatal immunity. CO2 promotes stomatal closure through complex signaling networks (Zhang et al., 2018). First, atmospheric CO2 enters guard cells via the PLASMA MEMBRANE INTRINSIC PROTEIN (PIP) aquaporins, followed by the conversion of CO2 to bicarbonate (HCO3 −) by beta carbonic anhydrases (βCAs) to activate downstream signaling events. Indeed, several studies have shown that the ubiquitous βCA enzymes are involved in the plant defense response. In Arabidopsis, genetic evidence demonstrated that βCA1 and βCA4 contribute to CO2-induced stomatal closure by converting CO2 into HCO3 − (Hu et al., 2010). The CA activity of βCA1 is required for a full defense response against avirulent Pst DC3000 carrying the effector AvrB (Wang et al., 2009). In addition, the quintuple mutant βca1 βca2 βca3 βca4 βca6 exhibited a partial reduction in SA sensitivity (Medina-Puche et al., 2017). However, Zhou et al. showed that, despite impaired stomatal closure preventing pathogen entry, PTI-mediated SA-dependent immunity against virulent P. syringae was enhanced in the βca1 βca4 double mutant (Zhou et al., 2020). Furthermore, they revealed that the PRR-mediated downregulation of βCA1 and βCA4 expression was attenuated by high CO2. These results suggest that CO2 concentration and βCAs regulate plant immunity positively or negatively as a function of compatible and incompatible interactions with the incoming pathogen. In tobacco (N. tabacum), βCA SA-BINDING PROTEIN 2 (SABP2) exhibits lipase activity and confers SA-dependent immunity against tomato mosaic virus (Kumar and Klessig, 2003). Similarly, SABP3 has antioxidant activity and confers HR triggered by Pto-mediated recognition of the effector AvrPto (Slaymaker et al., 2002). In addition, silencing of SABP3 increases susceptibility to Phytophthora infestans (Restrepo et al., 2005). The expression of CA (accession number BQ113997) increased in potato (Solanum tuberosum) inoculated with an incompatible P. infestans strain, while it was downregulated in potato inoculated with a compatible P. infestans strain. Recently, Hu et al. also showed that βCA3 confers plant basal immunity in tomato (Hu et al., 2021b). High CO2 and Pst DC3000 increases the induction of βCA3 expression by the transcription factor NAC43, while the phosphorylation of the serine 207 residue of βCA3 by GRACE1 (GERMINATION REPRESSION AND CELL EXPANSION RECEPTOR-LIKE KINASE 1) results in the activation of plant basal immunity related to the cell wall regardless of stomatal movement or SA signaling.
After converting CO2 into HCO3 −, ABA signaling has a central role downstream of the convergence point of CO2 for stomatal closure (Webb and Hetherington, 1997; Negi et al., 2008). Dittrich et al. argued that PYL4 and PYL5 are essential for CO2-induced stomatal closure in Arabidopsis (Dittrich et al., 2019). However, CO2-induced stomatal closure appears to be triggered by an ABA-independent pathway downstream of OST1/SnRK2.6 without direct activation of OST1/SnRK2.6 (Hsu et al., 2018). Another group also reported results in support of this idea. They developed a SnRK2 activity sensor called SNACS based on Förster resonance energy transfer (FRET) and showed that, although basal ABA levels and SnRK2 signaling are essential for CO2-induced stomatal closure, CO2 signaling did not activate SnRK2s including OST1/SnRK2.6 and PYL4 and PYL5 were also not required (Zhang et al., 2020). Therefore, it remains controversial whether CO2 signaling can act upstream of SnRK2 in the ABA signaling cascade.
Moreover, recent studies indicated that ROS signaling is also important for CO2 signaling for stomatal closure. In Arabidopsis, ROS signals as well as ABA signals are necessary for CO2-induced stomatal closure (Chater et al., 2015). He et al. showed that ROS produced by both cell wall peroxidases and NADPH oxidases, together with phytohormones (SA, JA, and ABA), play an important role in CO2-signaling during stomatal closure (He et al., 2020). However, the detailed molecular mechanisms by which ROS modulate CO2 signaling are still unknown. Therefore, we discuss below the effects of CO2 on ROS generation and plant immunity.
Photorespiration was once considered as a wasteful process because it is inefficient compared to the Calvin cycle and occurs when photosynthesis cannot operate. However, many studies have since shown photorespiration is involved in and required for various plant processes (Shi and Bloom, 2021). In particular, photorespiration has a crucial role in plant defenses due to ROS generation (Sørhagen et al., 2013). Hydrogen peroxide (H2O2) is a non-radical ROS that is deeply associated with plant defense responses (Smirnoff and Arnaud, 2019). It is produced mainly in leaf peroxisomes during photorespiration, with peroxisomal glycolate oxidase (GOX) and catalase (CAT) acting as major positive and negative regulators of its production, respectively (Foyer et al., 2009; Corpas et al., 2020).
Photorespiration and the Calvin cycle are competitively controlled by ribulose-1,5-bisphosphate carboxylase/oxygenase (Rubisco); thus, high CO2 levels decrease photorespiration (Long et al., 2004; Busch, 2020). Therefore, high CO2 would be expected to repress plant immunity. However, several studies have shown that high CO2 can increase plant defense responses including SA and JA (Noctor and Mhamdi, 2017). In addition, CAT2 was shown to be involved in SA-mediated auxin and JA inhibition of resistance against biotrophs (Yuan et al., 2017). Recently, Williams et al. demonstrated that CO2 influenced resistance to biotrophic and necrotrophic pathogens differently in Arabidopsis (Williams et al., 2018). Under high CO2 conditions (1200 ppm), resistance to both the biotrophic oomycete Hyaloperonospora arabidopsidis and the necrotrophic fungus Plectosphaerella cucumerina increased compared to ambient CO2 (400 ppm). SA appeared to play a minor role in resistance to the biotrophic pathogen, while JA conferred strong resistance against the necrotrophic pathogen. At low CO2 (200 ppm), resistance to H. arabidopsidis was enhanced through photorespiration-derived H2O2 production, whereas resistance to P. cucumerina declined.
Advances in biotechnology have opened up the possibility of overcoming the deleterious effects of climate change on crop plants. Induction of plant innate immunity compromised by climate change improves disease resistance to pathogen under the unfavorable environmental condition, but the constitutive activation of plant immune response retards growth and reduces crop productivity. To address this problem, scientists focused on the strategy to activate plant defense response spatiotemporally using pathogen-induced promoters and pathogen-responsive upstream open reading frames (Kim et al., 2021). However, this method cannot be free from the issue of genetically modified organisms. Therefore, the genome editing technologies based on SDNs (e.g., CRISPR/Cas9) are necessary for the development of climate resilient crops. However, even though genome editing has successfully increased the disease resistance of various crops, there are still significant hurdle to its application to climate change adaptive crop development due to the negative effects of mutations on the crop’s performance (Karavolias et al., 2021). Therefore, in order to cope with the future food resource crisis, understanding the various plant immune mechanisms affected by climate change and identifying elite genes that can improve disease resistance through genome editing will be one of the most efficient ways to develop climate resilient crops.
We are currently living in an unprecedented era of climate change. The consequences of this changing climate may diminish crop production and access to nutrients for all living creatures, concomitantly with the faster adaptation of microorganisms including phytopathogens due to their short life cycle and rapid propagation compared to other and more complex species, causing more severe damage to crop plants. It is clear that the damage to global crop security due to biotic stresses will pose a great challenge to human life in the future. Scientists have recently achieved remarkable progress in this field. Here, we provide an overview of the known and anticipated effects of climate change such as temperature, high humidity, and CO2 on plant immunity mechanisms. The current efforts to understand how climate change will impact plant immune systems and to develop more efficient NPBTs will make it possible to overcome the incoming crisis through crop improvement that can minimize damage and preserve yields in future pathogen-friendly environmental conditions.
SS conceptualized and wrote the manuscript. SRP supervised. All authors contributed to the article and approved the submitted manuscript.
This research was funded by Research Program for Agricultural Science and Technology Development (Project No. PJ01661001), and supported by the 2022 Fellowship Program (Project No. PJ01661001) of the National Institute of Agricultural Sciences, Rural Development Administration, Republic of Korea.
The authors declare that the research was conducted in the absence of any commercial or financial relationships that could be construed as a potential conflict of interest.
All claims expressed in this article are solely those of the authors and do not necessarily represent those of their affiliated organizations, or those of the publisher, the editors and the reviewers. Any product that may be evaluated in this article, or claim that may be made by its manufacturer, is not guaranteed or endorsed by the publisher.
Albrecht, T., Argueso, C. T. (2017). Should I fight or should I grow now? the role of cytokinins in plant growth and immunity and in the growth–defence trade-off. Ann. Bot. 119 (5), 725–735. doi: 10.1093/aob/mcw211
Alley, R., Berntsen, T., Bindoff, N. L., Chen, Z., Chidthaisong, A., Friedlingstein, P., et al. (2007). Climate change 2007: The physical science basis. contribution of working group I to the fourth assessment report of the intergovernmental panel on climate change. summary for policymakers (Geneva, Switzerland: IPCC Secretariat), 21.
Anderson, J. P., Gleason, C. A., Foley, R. C., Thrall, P. H., Burdon, J. B., Singh, K. B. (2010). Plants versus pathogens: an evolutionary arms race. Funct. Plant Biol. 37 (6), 499–512. doi: 10.1071/FP09304
Argueso, C. T., Ferreira, F. J., Epple, P., To, J. P., Hutchison, C. E., Schaller, G. E., et al. (2012). Two-component elements mediate interactions between cytokinin and salicylic acid in plant immunity. PloS Genet. 8 (1), e1002448. doi: 10.1371/journal.pgen.1002448
Arnaud, D., Hwang, I. (2015). A sophisticated network of signaling pathways regulates stomatal defenses to bacterial pathogens. Mol. Plant 8 (4), 566–581. doi: 10.1016/j.molp.2014.10.012
Aung, K., Jiang, Y., He, S. Y. (2018). The role of water in plant–microbe interactions. Plant J. 93 (4), 771–780. doi: 10.1111/tpj.13795
Axtell, M. J., Staskawicz, B. J. (2003). Initiation of RPS2-specified disease resistance in arabidopsis is coupled to the AvrRpt2-directed elimination of RIN4. Cell 112 (3), 369–377. doi: 10.1016/S0092-8674(03)00036-9
Backer, R., Naidoo, S., Van den Berg, N. (2019). The NONEXPRESSOR OF PATHOGENESIS-RELATED GENES 1 (NPR1) and related family: mechanistic insights in plant disease resistance. Front. Plant Sci. 10, 102. doi: 10.3389/fpls.2019.00102
Beattie, G. A. (2016). A war over water when bacteria invade leaves. Nature 539 (7630), 506–507. doi: 10.1038/539506a
Belkhadir, Y., Nimchuk, Z., Hubert, D. A., Mackey, D., Dangl, J. L. (2004). Arabidopsis RIN4 negatively regulates disease resistance mediated by RPS2 and RPM1 downstream or independent of the NDR1 signal modulator and is not required for the virulence functions of bacterial type III effectors AvrRpt2 or AvrRpm1. Plant Cell 16 (10), 2822–2835. doi: 10.1105/tpc.104.024117
Berens, M. L., Wolinska, K. W., Spaepen, S., Ziegler, J., Nobori, T., Nair, A., et al. (2019). Balancing trade-offs between biotic and abiotic stress responses through leaf age-dependent variation in stress hormone cross-talk. Proc. Natl. Acad. Sci. 116 (6), 2364–2373. doi: 10.1073/pnas.1817233116
Bose, J., Pottosin, I. I., Shabala, S. S., Palmgren, M. G., Shabala, S. (2011). Calcium efflux systems in stress signaling and adaptation in plants. Front. Plant Sci. 2, 85. doi: 10.3389/fpls.2011.00085
Bruessow, F., Bautor, J., Hoffmann, G., Yildiz, I., Zeier, J., Parker, J. E. (2021). Natural variation in temperature-modulated immunity uncovers transcription factor bHLH059 as a thermoresponsive regulator in arabidopsis thaliana. PloS Genet. 17 (1), e1009290. doi: 10.1371/journal.pgen.1009290
Busch, F. A. (2020). Photorespiration in the context of rubisco biochemistry, CO2 diffusion and metabolism. Plant J. 101 (4), 919–939. doi: 10.1111/tpj.14674
Caffarra, A., Rinaldi, M., Eccel, E., Rossi, V., Pertot, I. (2012). Modelling the impact of climate change on the interaction between grapevine and its pests and pathogens: European grapevine moth and powdery mildew. Agriculture Ecosyst. Environ. 148, 89–101. doi: 10.1016/j.agee.2011.11.017
Cai, W., Yang, S., Wu, R., Cao, J., Shen, L., Guan, D., et al. (2021). Pepper NAC-type transcription factor NAC2c balances the trade-off between growth and defense responses. Plant Physiol 186 (4), 2169–2189. doi: 10.1093/plphys/kiab190
Cai, H., Yang, S., Yan, Y., Xiao, Z., Cheng, J., Wu, J., et al. (2015). CaWRKY6 transcriptionally activates CaWRKY40, regulates ralstonia solanacearum resistance, and confers high-temperature and high-humidity tolerance in pepper. J. Exp. Bot. 66 (11), 3163–3174. doi: 10.1093/jxb/erv125
Castroverde, C. D. M., Dina, D. (2021). Temperature regulation of plant hormone signaling during stress and development. J. Exp. Bot. 72 (21), 7436–7458. doi: 10.1093/jxb/erab257
Challinor, A. J., Watson, J., Lobell, D. B., Howden, S., Smith, D., Chhetri, N. (2014). A meta-analysis of crop yield under climate change and adaptation. Nat. Climate Change 4 (4), 287–291. doi: 10.1038/nclimate2153
Chaloner, T. M., Gurr, S. J., Bebber, D. P. (2021). Plant pathogen infection risk tracks global crop yields under climate change. Nat. Climate Change 11 (8), 710–715. doi: 10.1038/s41558-021-01104-8
Chapman, J. M., Muhlemann, J. K., Gayomba, S. R., Muday, G. K. (2019). RBOH-dependent ROS synthesis and ROS scavenging by plant specialized metabolites to modulate plant development and stress responses. Chem. Res. Toxicol. 32 (3), 370–396. doi: 10.1021/acs.chemrestox.9b00028
Chater, C., Peng, K., Movahedi, M., Dunn, J. A., Walker, H. J., Liang, Y.-K., et al. (2015). Elevated CO2-induced responses in stomata require ABA and ABA signaling. Curr. Biol. 25 (20), 2709–2716. doi: 10.1016/j.cub.2015.09.013
Cheng, C., Gao, X., Feng, B., Sheen, J., Shan, L., He, P. (2013). Plant immune response to pathogens differs with changing temperatures. Nat. Commun. 4 (1), 1–9. doi: 10.1038/ncomms3530
Chinchilla, D., Zipfel, C., Robatzek, S., Kemmerling, B., Nürnberger, T., Jones, J. D., et al. (2007). A flagellin-induced complex of the receptor FLS2 and BAK1 initiates plant defence. Nature 448 (7152), 497–500. doi: 10.1038/nature05999
Chisholm, S. T., Coaker, G., Day, B., Staskawicz, B. J. (2006). Host-microbe interactions: shaping the evolution of the plant immune response. Cell 124 (4), 803–814. doi: 10.1016/j.cell.2006.02.008
Choi, J., Choi, D., Lee, S., Ryu, C.-M., Hwang, I. (2011). Cytokinins and plant immunity: old foes or new friends? Trends Plant Sci. 16 (7), 388–394. doi: 10.1016/j.tplants.2011.03.003
Coll, N. S., Epple, P., Dangl, J. L. (2011). Programmed cell death in the plant immune system. Cell Death Differentiation 18 (8), 1247–1256. doi: 10.1038/cdd.2011.37
Conrath, U., Beckers, G. J., Langenbach, C. J., Jaskiewicz, M. R. (2015). Priming for enhanced defense. Annu. Rev. Phytopathol. 53 (1), 97–119. doi: 10.1146/annurev-phyto-080614-120132
Coppinger, P., Repetti, P. P., Day, B., Dahlbeck, D., Mehlert, A., Staskawicz, B. J. (2004). Overexpression of the plasma membrane-localized NDR1 protein results in enhanced bacterial disease resistance in arabidopsis thaliana. Plant J. 40 (2), 225–237. doi: 10.1111/j.1365-313X.2004.02203.x
Corpas, F. J., González-Gordo, S., Palma, J. M. (2020). Plant peroxisomes: A factory of reactive species. Front. Plant Sci. 11, 853. doi: 10.3389/fpls.2020.00853
Corwin, J. A., Kliebenstein, D. J. (2017). Quantitative resistance: more than just perception of a pathogen. Plant Cell 29 (4), 655–665. doi: 10.1105/tpc.16.00915
Cui, H., Gobbato, E., Kracher, B., Qiu, J., Bautor, J., Parker, J. E. (2017). A core function of EDS1 with PAD4 is to protect the salicylic acid defense sector in arabidopsis immunity. New Phytol. 213 (4), 1802–1817. doi: 10.1111/nph.14302
DANG, F. F., WANG, Y. N., Yu, L., Eulgem, T., Lai, Y., LIU, Z. Q., et al. (2013). CaWRKY40, a WRKY protein of pepper, plays an important role in the regulation of tolerance to heat stress and resistance to ralstonia solanacearum infection. Plant Cell Environ. 36 (4), 757–774. doi: 10.1111/pce.12011
Davis, K. R., Schott, E., Ausubel, F. M. (1991). Virulence of selected phytopathogenic pseudomonads in arabidopsis thaliana. Mol. Plant-Microbe Interact. 4 (1), 477–488. doi: 10.1094/MPMI-4-477
Day, B., Dahlbeck, D., Staskawicz, B. J. (2006). NDR1 interaction with RIN4 mediates the differential activation of multiple disease resistance pathways in arabidopsis. Plant Cell 18 (10), 2782–2791. doi: 10.1105/tpc.106.044693
Desaint, H., Aoun, N., Deslandes, L., Vailleau, F., Roux, F., Berthomé, R. (2021). Fight hard or die trying: when plants face pathogens under heat stress. New Phytol. 229 (2), 712–734. doi: 10.1111/nph.16965
Deutsch, C. A., Tewksbury, J. J., Huey, R. B., Sheldon, K. S., Ghalambor, C. K., Haak, D. C., et al. (2008). Impacts of climate warming on terrestrial ectotherms across latitude. Proc. Natl. Acad. Sci. 105 (18), 6668–6672. doi: 10.1073/pnas.0709472105
Dittrich, M., Mueller, H. M., Bauer, H., Peirats-Llobet, M., Rodriguez, P. L., Geilfus, C.-M., et al. (2019). The role of arabidopsis ABA receptors from the PYR/PYL/RCAR family in stomatal acclimation and closure signal integration. Nat. Plants 5 (9), 1002–1011. doi: 10.1038/s41477-019-0490-0
Driesen, E., Van den Ende, W., De Proft, M., Saeys, W. (2020). Influence of environmental factors light, CO2, temperature, and relative humidity on stomatal opening and development: A review. Agronomy 10 (12), 1975. doi: 10.3390/agronomy10121975
Du, L., Ali, G. S., Simons, K. A., Hou, J., Yang, T., Reddy, A., et al. (2009). Ca2+/calmodulin regulates salicylic-acid-mediated plant immunity. Nature 457 (7233), 1154–1158. doi: 10.1038/nature07612
Elad, Y., Pertot, I. (2014). Climate change impacts on plant pathogens and plant diseases. J. Crop Improv. 28 (1), 99–139. doi: 10.1080/15427528.2014.865412
Flors, V., Ton, J., Van Doorn, R., Jakab, G., García-Agustín, P., Mauch-Mani, B. (2008). Interplay between JA, SA and ABA signalling during basal and induced resistance against pseudomonas syringae and alternaria brassicicola. Plant J. 54 (1), 81–92. doi: 10.1111/j.1365-313X.2007.03397.x
Foyer, C. H., Bloom, A. J., Queval, G., Noctor, G. (2009). Photorespiratory metabolism: genes, mutants, energetics, and redox signaling. Annu. Rev. Plant Biol. 60, 455–484. doi: 10.1146/annurev.arplant.043008.091948
French, E., Kim, B.-S., Iyer-Pascuzzi, A. S. (2016). Mechanisms of quantitative disease resistance in plants. Semin. Cell Dev. Biol. 56, 201–208. doi: 10.1016/j.semcdb.2016.05.015
Gangappa, S. N., Berriri, S., Kumar, S. V. (2017). PIF4 coordinates thermosensory growth and immunity in arabidopsis. Curr. Biol. 27 (2), 243–249. doi: 10.1016/j.cub.2016.11.012
Gangappa, S. N., Kumar, S. V. (2018). DET1 and COP1 modulate the coordination of growth and immunity in response to key seasonal signals in arabidopsis. Cell Rep. 25 (1), 29–37. e23. doi: 10.1016/j.celrep.2018.08.096
García, A. V., Blanvillain-Baufumé, S., Huibers, R. P., Wiermer, M., Li, G., Gobbato, E., et al. (2010). Balanced nuclear and cytoplasmic activities of EDS1 are required for a complete plant innate immune response. PloS Pathog. 6 (7), e1000970. doi: 10.1371/journal.ppat.1000970
Garrett, K. A., Dendy, S. P., Frank, E. E., Rouse, M. N., Travers, S. E. (2006). Climate change effects on plant disease: genomes to ecosystems. Annu. Rev. Phytopathol. 44, 489–509. doi: 10.1146/annurev.phyto.44.070505.143420
Glazebrook, J. (2005). Contrasting mechanisms of defense against biotrophic and necrotrophic pathogens. Annu. Rev. Phytopathol. 43, 205. doi: 10.1146/annurev.phyto.43.040204.135923
Guzel Deger, A., Scherzer, S., Nuhkat, M., Kedzierska, J., Kollist, H., Brosché, M., et al. (2015). Guard cell SLAC 1-type anion channels mediate flagellin-induced stomatal closure. New Phytol. 208 (1), 162–173. doi: 10.1111/nph.13435
Ham, J. H., Majerczak, D. R., Arroyo-Rodriguez, A. S., Mackey, D. M., Coplin, D. L. (2006). WtsE, an AvrE-family effector protein from pantoea stewartii subsp. stewartii, causes disease-associated cell death in corn and requires a chaperone protein for stability. Mol. Plant-Microbe Interact. 19 (10), 1092–1102. doi: 10.1094/MPMI-19-1092
Hammoudi, V., Fokkens, L., Beerens, B., Vlachakis, G., Chatterjee, S., Arroyo-Mateos, M., et al. (2018). The arabidopsis SUMO E3 ligase SIZ1 mediates the temperature dependent trade-off between plant immunity and growth. PloS Genet. 14 (1), e1007157. doi: 10.1371/journal.pgen.1007157
Haque, M. A., Marwaha, S., Deb, C. K., Nigam, S., Arora, A., Hooda, K. S., et al. (2022). Deep learning-based approach for identification of diseases of maize crop. Sci. Rep. 12 (1), 6334. doi: 10.1038/s41598-022-10140-z
Havko, N. E., Das, M. R., McClain, A. M., Kapali, G., Sharkey, T. D., Howe, G. A. (2020). Insect herbivory antagonizes leaf cooling responses to elevated temperature in tomato. Proc. Natl. Acad. Sci. 117 (4), 2211–2217. doi: 10.1073/pnas.1913885117
He, J., Zhang, R.-X., Kim, D. S., Sun, P., Liu, H., Liu, Z., et al. (2020). ROS of distinct sources and salicylic acid separate elevated CO2-mediated stomatal movements in arabidopsis. Front. Plant Sci. 11, 542. doi: 10.3389/fpls.2020.00542
Hou, S., Liu, D., He, P. (2021). Phytocytokines function as immunological modulators of plant immunity. Stress Biol. 1 (1), 1–14. doi: 10.1007/s44154-021-00009-y
Hsu, P. K., Dubeaux, G., Takahashi, Y., Schroeder, J. I. (2021). Signaling mechanisms in abscisic acid-mediated stomatal closure. Plant J. 105 (2), 307–321. doi: 10.1111/tpj.15067
Hsu, P.-K., Takahashi, Y., Munemasa, S., Merilo, E., Laanemets, K., Waadt, R., et al. (2018). Abscisic acid-independent stomatal CO2 signal transduction pathway and convergence of CO2 and ABA signaling downstream of OST1 kinase. Proc. Natl. Acad. Sci. 115 (42), E9971–E9980. doi: 10.1073/pnas.1809204115
Huang, J., Zhao, X., Bürger, M., Wang, Y., Chory, J. (2021a). Two interacting ethylene response factors regulate heat stress response. Plant Cell 33 (2), 338–357. doi: 10.1093/plcell/koaa026
Huang, S., Zhu, S., Kumar, P., MacMicking, J. D. (2021b). A phase-separated nuclear GBPL circuit controls immunity in plants. Nature 594 (7863), 424–429. doi: 10.1038/s41586-021-03572-6
Hu, H., Boisson-Dernier, A., Israelsson-Nordström, M., Böhmer, M., Xue, S., Ries, A., et al. (2010). Carbonic anhydrases are upstream regulators of CO2-controlled stomatal movements in guard cells. Nat. Cell Biol. 12 (1), 87–93. doi: 10.1038/ncb2009
Hu, Y., Ding, Y., Cai, B., Qin, X., Wu, J., Yuan, M., et al. (2022). Bacterial effectors manipulate plant abscisic acid signaling for creation of an aqueous apoplast. Cell Host Microbe 30 (4), 518–529. doi: 10.1016/j.chom.2022.02.002
Hu, Z., Ma, Q., Foyer, C. H., Lei, C., Choi, H. W., Zheng, C., et al. (2021b). High CO2-and pathogen-driven expression of the carbonic anhydrase βCA3 confers basal immunity in tomato. New Phytol. 229 (5), 2827–2843. doi: 10.1111/nph.17087
Huot, B., Castroverde, C. D. M., Velásquez, A. C., Hubbard, E., Pulman, J. A., Yao, J., et al. (2017). Dual impact of elevated temperature on plant defence and bacterial virulence in arabidopsis. Nat. Commun. 8 (1), 1–12. doi: 10.1038/s41467-017-01674-2
Hu, Y., Tao, F., Su, C., Zhang, Y., Li, J., Wang, J., et al. (2021a). NBS-LRR gene TaRPS2 is positively associated with the high-temperature seedling plant resistance of wheat against puccinia striiformis f. sp. tritici. Phytopathology 111 (8), 1449–1458. doi: 10.1094/PHYTO-03-20-0063-R
Iqbal, Z., Shariq Iqbal, M., Singh, S. P., Buaboocha, T. (2020). Ca2+/calmodulin complex triggers CAMTA transcriptional machinery under stress in plants: signaling cascade and molecular regulation. Front. Plant Sci. 11, 598327. doi: 10.3389/fpls.2020.598327
Islam, M. T., Gupta, D. R., Hossain, A., Roy, K. K., He, X., Kabir, M. R., et al. (2020). Wheat blast: a new threat to food security. Phytopathol. Res. 2 (1), 1–13. doi: 10.1186/s42483-020-00067-6
Janda, M., Lamparová, L., Zubíková, A., Burketová, L., Martinec, J., Krčková, Z. (2019). Temporary heat stress suppresses PAMP-triggered immunity and resistance to bacteria in arabidopsis thaliana. Mol. Plant Pathol. 20 (7), 1005–1012. doi: 10.1111/mpp.12799
Jones, J. D., Dangl, J. L. (2006). The plant immune system. Nature 444 (7117), 323–329. doi: 10.1038/nature05286
Jung, J.-H., Domijan, M., Klose, C., Biswas, S., Ezer, D., Gao, M., et al. (2016). Phytochromes function as thermosensors in arabidopsis. Science 354 (6314), 886–889. doi: 10.1126/science.aaf6005
Junho, C. V. C., Caio-Silva, W., Trentin-Sonoda, M., Carneiro-Ramos, M. S. (2020). An overview of the role of calcium/calmodulin-dependent protein kinase in cardiorenal syndrome. Front. Physiol. 11, 735. doi: 10.3389/fphys.2020.00735
Kangasjärvi, S., Neukermans, J., Li, S., Aro, E.-M., Noctor, G. (2012). Photosynthesis, photorespiration, and light signalling in defence responses. J. Exp. Bot. 63 (4), 1619–1636. doi: 10.1093/jxb/err402
Karavolias, N. G., Horner, W., Abugu, M. N., Evanega, S. N. (2021). Application of gene editing for climate change in agriculture. Front. Sustain. Food Syst. 5, 685801. doi: 10.3389/fsufs.2021.685801
Kim, J. H., Castroverde, C. D. M., Huang, S., Li, C., Hilleary, R., Seroka, A., et al. (2022). Increasing the resilience of plant immunity to a warming climate. Nature 607 (7918), 339–344. doi: 10.1038/s41586-022-04902-y
Kim, J. H., Hilleary, R., Seroka, A., He, S. Y. (2021). Crops of the future: building a climate-resilient plant immune system. Curr. Opin. Plant Biol. 60, 101997. doi: 10.1016/j.pbi.2020.101997
Kumar, D., Klessig, D. F. (2003). High-affinity salicylic acid-binding protein 2 is required for plant innate immunity and has salicylic acid-stimulated lipase activity. Proc. Natl. Acad. Sci. 100 (26), 16101–16106. doi: 10.1073/pnas.0307162100
Kumar, S. V., Wigge, P. A. (2010). H2A. z-containing nucleosomes mediate the thermosensory response in arabidopsis. Cell 140 (1), 136–147. doi: 10.1016/j.cell.2009.11.006
Lal, N. K., Nagalakshmi, U., Hurlburt, N. K., Flores, R., Bak, A., Sone, P., et al. (2018). The receptor-like cytoplasmic kinase BIK1 localizes to the nucleus and regulates defense hormone expression during plant innate immunity. Cell Host Microbe 23 (4), 485–497. doi: 10.1016/j.chom.2018.03.010
Lapin, D., Bhandari, D. D., Parker, J. E. (2020). Origins and immunity networking functions of EDS1 family proteins. Annu. Rev. Phytopathol. 58, 253–276. doi: 10.1146/annurev-phyto-010820-012840
Lee, S. C., Luan, S. (2012). ABA signal transduction at the crossroad of biotic and abiotic stress responses. Plant Cell Environ. 35 (1), 53–60. doi: 10.1111/j.1365-3040.2011.02426.x
Lefevere, H., Bauters, L., Gheysen, G. (2020). Salicylic acid biosynthesis in plants. Front. Plant Sci. 11, 338. doi: 10.3389/fpls.2020.00338
Legris, M., Klose, C., Burgie, E. S., Rojas, C. C. R., Neme, M., Hiltbrunner, A., et al. (2016). Phytochrome b integrates light and temperature signals in arabidopsis. Science 354 (6314), 897–900. doi: 10.1126/science.aaf5656
Li, N., Han, X., Feng, D., Yuan, D., Huang, L.-J. (2019). Signaling crosstalk between salicylic acid and ethylene/jasmonate in plant defense: do we understand what they are whispering? Int. J. Mol. Sci. 20 (3), 671. doi: 10.3390/ijms20030671
Li, Z., Liu, H., Ding, Z., Yan, J., Yu, H., Pan, R., et al. (2020). Low temperature enhances plant immunity via salicylic acid pathway genes that are repressed by ethylene. Plant Physiol. 182 (1), 626–639. doi: 10.1104/pp.19.01130
Liu, Z., Hou, S., Rodrigues, O., Wang, P., Luo, D., Munemasa, S., et al. (2022b). Phytocytokine signalling reopens stomata in plant immunity and water loss. Nature 605 (7909), 332–339. doi: 10.1038/s41586-022-04684-3
Liu, H., Lu, C., Li, Y., Wu, T., Zhang, B., Liu, B., et al. (2022a). The bacterial effector AvrRxo1 inhibits vitamin B6 biosynthesis to promote infection in rice. Plant Commun. 3 (3), 100324. doi: 10.1016/j.xplc.2022.100324
Long, S. P., Ainsworth, E. A., Leakey, A. D., Nosberger, J., Ort, D. R. (2006). Food for thought: lower-than-expected crop yield stimulation with rising CO2 concentrations. science 312 (5782), 1918–1921. doi: 10.1126/science.1114722
Long, S. P., Ainsworth, E. A., Rogers, A., Ort, D. R. (2004). Rising atmospheric carbon dioxide: plants FACE the future. Annu. Rev. Plant Biol. 55, 591. doi: 10.1146/annurev.arplant.55.031903.141610
Lusser, M., Parisi, C., Plan, D., Rodríguez-Cerezo, E. (2011). New plant breeding techniques: state-of-the-art and prospects for commercial development (Luxembourg: Publications office of the European Union).
Lusser, M., Parisi, C., Plan, D., Rodriguez-Cerezo, E. (2012). Deployment of new biotechnologies in plant breeding. Nat. Biotechnol. 30 (3), 231–239. doi: 10.1038/nbt.2142
Mackey, D., Belkhadir, Y., Alonso, J. M., Ecker, J. R., Dangl, J. L. (2003). Arabidopsis RIN4 is a target of the type III virulence effector AvrRpt2 and modulates RPS2-mediated resistance. Cell 112 (3), 379–389. doi: 10.1016/S0092-8674(03)00040-0
Mackey, D., Holt, B. F., III, Wiig, A., Dangl, J. L. (2002). RIN4 interacts with pseudomonas syringae type III effector molecules and is required for RPM1-mediated resistance in arabidopsis. Cell 108 (6), 743–754. doi: 10.1016/S0092-8674(02)00661-X
Ma, X., Li, Q.-H., Yu, Y.-N., Qiao, Y.-M., Gong, Z.-H. (2020). The CBL–CIPK pathway in plant response to stress signals. Int. J. Mol. Sci. 21 (16), 5668. doi: 10.3390/ijms21165668
McIntyre, K. E., Bush, D. R., Argueso, C. T. (2021). Cytokinin regulation of source-sink relationships in plant-pathogen interactions. Front. Plant Sci. 12, 677585. doi: 10.3389/fpls.2021.677585
Medina-Puche, L., Castelló, M. J., Canet, J. V., Lamilla, J., Colombo, M. L., Tornero, P. (2017). β-carbonic anhydrases play a role in salicylic acid perception in arabidopsis. PloS One 12 (7), e0181820. doi: 10.1371/journal.pone.0181820
Melotto, M., Underwood, W., Koczan, J., Nomura, K., He, S. Y. (2006). Plant stomata function in innate immunity against bacterial invasion. Cell 126 (5), 969–980. doi: 10.1016/j.cell.2006.06.054
Melotto, M., Zhang, L., Oblessuc, P. R., He, S. Y. (2017). Stomatal defense a decade later. Plant Physiol. 174 (2), 561–571. doi: 10.1104/pp.16.01853
Merilo, E., Jalakas, P., Laanemets, K., Mohammadi, O., Horak, H., Kollist, H., et al. (2015). Abscisic acid transport and homeostasis in the context of stomatal regulation. Mol. Plant 8 (9), 1321–1333. doi: 10.1016/j.molp.2015.06.006
Monaghan, J., Zipfel, C. (2012). Plant pattern recognition receptor complexes at the plasma membrane. Curr. Opin. Plant Biol. 15 (4), 349–357. doi: 10.1016/j.pbi.2012.05.006
Monteiro, F., Nishimura, M. T. (2018). Structural, functional, and genomic diversity of plant NLR proteins: An evolved resource for rational engineering of plant immunity. Annu. Rev. Phytopathol. 56, 243–267. doi: 10.1146/annurev-phyto-080417-045817
Mukhtar, M. S., Carvunis, A.-R., Dreze, M., Epple, P., Steinbrenner, J., Moore, J., et al. (2011). Independently evolved virulence effectors converge onto hubs in a plant immune system network. science 333 (6042), 596–601. doi: 10.1126/science.1203659
Murata, Y., Mori, I. C., Munemasa, S. (2015). Diverse stomatal signaling and the signal integration mechanism. Annu. Rev. Plant Biol. 66, 369–392. doi: 10.1146/annurev-arplant-043014-114707
Nanda, A. K., Andrio, E., Marino, D., Pauly, N., Dunand, C. (2010). Reactive oxygen species during plant-microorganism early interactions. J. Integr. Plant Biol. 52 (2), 195–204. doi: 10.1111/j.1744-7909.2010.00933.x
Naveed, Z. A., Wei, X., Chen, J., Mubeen, H., Ali, G. S. (2020). The PTI to ETI continuum in phytophthora-plant interactions. Front. Plant Sci. 11, 593905. doi: 10.3389/fpls.2020.593905
Negi, J., Matsuda, O., Nagasawa, T., Oba, Y., Takahashi, H., Kawai-Yamada, M., et al. (2008). CO2 regulator SLAC1 and its homologues are essential for anion homeostasis in plant cells. Nature 452 (7186), 483–486. doi: 10.1038/nature06720
Nie, H., Zhao, C., Wu, G., Wu, Y., Chen, Y., Tang, D. (2012). SR1, a calmodulin-binding transcription factor, modulates plant defense and ethylene-induced senescence by directly regulating NDR1 and EIN3. Plant Physiol. 158 (4), 1847–1859. doi: 10.1104/pp.111.192310
Noctor, G., Mhamdi, A. (2017). Climate change, CO2, and defense: the metabolic, redox, and signaling perspectives. Trends Plant Sci. 22 (10), 857–870. doi: 10.1016/j.tplants.2017.07.007
Nomura, K., DebRoy, S., Lee, Y. H., Pumplin, N., Jones, J., He, S. Y. (2006). A bacterial virulence protein suppresses host innate immunity to cause plant disease. Science 313 (5784), 220–223. doi: 10.1126/science.1129523
Nomura, K., Mecey, C., Lee, Y.-N., Imboden, L. A., Chang, J. H., He, S. Y. (2011). Effector-triggered immunity blocks pathogen degradation of an immunity-associated vesicle traffic regulator in arabidopsis. Proc. Natl. Acad. Sci. 108 (26), 10774–10779. doi: 10.1073/pnas.1103338108
Oerke, E.-C. (2006). Crop losses to pests. J. Agric. Sci. 144 (1), 31–43. doi: 10.1017/S0021859605005708
Pieterse, C. M., van der Does, D., Zamioudis, C., Leon-Reyes, A., Van Wees, S. C. (2012). Hormonal modulation of plant immunity. Annu. Rev. Cell Dev. Biol. 28, 489–521. doi: 10.1146/annurev-cellbio-092910-154055
Poland, J. A., Balint-Kurti, P. J., Wisser, R. J., Pratt, R. C., Nelson, R. J. (2009). Shades of gray: the world of quantitative disease resistance. Trends Plant Sci. 14 (1), 21–29. doi: 10.1016/j.tplants.2008.10.006
Pruitt, R. N., Locci, F., Wanke, F., Zhang, L., Saile, S. C., Joe, A., et al. (2021). The EDS1–PAD4–ADR1 node mediates arabidopsis pattern-triggered immunity. Nature 598 (7881), 495–499. doi: 10.1038/s41586-021-03829-0
Rasmussen, S., Barah, P., Suarez-Rodriguez, M. C., Bressendorff, S., Friis, P., Costantino, P., et al. (2013). Transcriptome responses to combinations of stresses in arabidopsis. Plant Physiol. 161 (4), 1783–1794. doi: 10.1104/pp.112.210773
Reimers, P., Leach, J. (1991). Race-specific resistance to xanthomonas oryzae pv. oryzae conferred by bacterial blight resistance gene xa-10 in rice (Oryza sativa) involves accumulation of a lignin-like substance in host tissues. Physiol. Mol. Plant Pathol. 38 (1), 39–55. doi: 10.1016/S0885-5765(05)80141-9
Restrepo, S., Myers, K., Del Pozo, O., Martin, G., Hart, A., Buell, C., et al. (2005). Gene profiling of a compatible interaction between phytophthora infestans and solanum tuberosum suggests a role for carbonic anhydrase. Mol. Plant-Microbe Interact. 18 (9), 913–922. doi: 10.1094/MPMI-18-0913
Rising, J., Devineni, N. (2020). Crop switching reduces agricultural losses from climate change in the united states by half under RCP 8.5. Nat. Commun. 11 (1), 1–7. doi: 10.1038/s41467-020-18725-w
Román-Palacios, C., Wiens, J. J. (2020). Recent responses to climate change reveal the drivers of species extinction and survival. Proc. Natl. Acad. Sci. 117 (8), 4211–4217. doi: 10.1073/pnas.1913007117
Romero, F., Cazzato, S., Walder, F., Vogelgsang, S., Bender, S. F., van der Heijden, M. G. (2021). Humidity and high temperature are important for predicting fungal disease outbreaks worldwide. New Phytologist 234 (5), 1553–1556. doi: 10.1111/nph.17340
Roussin-Léveillée, C., Lajeunesse, G., St-Amand, M., Veerapen, V. P., Silva-Martins, G., Nomura, K., et al. (2022). Evolutionarily conserved bacterial effectors hijack abscisic acid signaling to induce an aqueous environment in the apoplast. Cell Host Microbe 30 (4), 489–501. e484. doi: 10.1016/j.chom.2022.02.006
Sørhagen, K., Laxa, M., Peterhänsel, C., Reumann, S. (2013). The emerging role of photorespiration and non-photorespiratory peroxisomal metabolism in pathogen defence. Plant Biol. 15 (4), 723–736. doi: 10.1111/j.1438-8677.2012.00723.x
Samaradivakara, S. P., Chen, H., Lu, Y. J., Li, P., Kim, Y., Tsuda, K., et al. (2022). Overexpression of NDR1 leads to pathogen resistance at elevated temperatures. New Phytologist 235 (3), 1146–1162. doi: 10.1111/nph.18190
Savary, S., Ficke, A., Aubertot, J.-N., Hollier, C. (2012). Crop losses due to diseases and their implications for global food production losses and food security Food Secur. 4, 519–537. doi: 10.1007/s00203-017-1426-6
Savary, S., Willocquet, L. (2020). Modeling the impact of crop diseases on global food security. Annu. Rev. Phytopathol. 58, 313–341. doi: 10.1146/annurev-phyto-010820-012856
Savary, S., Willocquet, L., Pethybridge, S. J., Esker, P., McRoberts, N., Nelson, A. (2019). The global burden of pathogens and pests on major food crops. Nat. Ecol. Evol. 3 (3), 430–439. doi: 10.1038/s41559-018-0793-y
Scholthof, K. B. (2007). The disease triangle: pathogens, the environment and society. Nat. Rev. Microbiol. 5 (2), 152–156. doi: 10.1038/nrmicro1596
Schornack, S., Minsavage, G. V., Stall, R. E., Jones, J. B., Lahaye, T. (2008). Characterization of AvrHah1, a novel AvrBs3-like effector from xanthomonas gardneri with virulence and avirulence activity. New Phytol. 179 (2), 546–556. doi: 10.1111/j.1469-8137.2008.02487.x
Serrano, M., Wang, B., Aryal, B., Garcion, C., Abou-Mansour, E., Heck, S., et al. (2013). Export of salicylic acid from the chloroplast requires the multidrug and toxin extrusion-like transporter EDS5. Plant Physiol. 162 (4), 1815–1821. doi: 10.1104/pp.113.218156
Shapiro, A. D., Zhang, C. (2001). The role of NDR1 in avirulence gene-directed signaling and control of programmed cell death in arabidopsis. Plant Physiol. 127 (3), 1089–1101. doi: 10.1104/pp.010096
Shen, L., Liu, Z., Yang, S., Yang, T., Liang, J., Wen, J., et al. (2016a). Pepper CabZIP63 acts as a positive regulator during ralstonia solanacearum or high temperature–high humidity challenge in a positive feedback loop with CaWRKY40. J. Exp. Bot. 67 (8), 2439–2451. doi: 10.1093/jxb/erw069
Shen, L., Yang, S., Yang, F., Guan, D., He, S. (2020). CaCBL1 acts as a positive regulator in pepper response to ralstonia solanacearum. Mol. Plant-Microbe Interact. 33 (7), 945–957. doi: 10.1094/MPMI-08-19-0241-R
Shen, L., Yang, S., Yang, T., Liang, J., Cheng, W., Wen, J., et al. (2016b). CaCDPK15 positively regulates pepper responses to ralstonia solanacearum inoculation and forms a positive-feedback loop with CaWRKY40 to amplify defense signaling. Sci. Rep. 6 (1), 1–12. doi: 10.1038/srep22439
Shi, X., Bloom, A. (2021). Photorespiration: the futile cycle? Plants 10 (5), 908. doi: 10.3390/plants10050908
Slaymaker, D. H., Navarre, D. A., Clark, D., del Pozo, O., Martin, G. B., Klessig, D. F. (2002). The tobacco salicylic acid-binding protein 3 (SABP3) is the chloroplast carbonic anhydrase, which exhibits antioxidant activity and plays a role in the hypersensitive defense response. Proc. Natl. Acad. Sci. 99 (18), 11640–11645. doi: 10.1073/pnas.182427699
Smirnoff, N., Arnaud, D. (2019). Hydrogen peroxide metabolism and functions in plants. New Phytol. 221 (3), 1197–1214. doi: 10.1111/nph.15488
Song, G., Son, S., Lee, K. S., Park, Y. J., Suh, E. J., Lee, S. I., et al. (2022). OsWRKY114 negatively regulates drought tolerance by restricting stomatal closure in rice. Plants 11 (15), 1938. doi: 10.3390/plants11151938
Son, S., Im, J. H., Song, G., Nam, S., Park, S. R. (2022). OsWRKY114 inhibits ABA-induced susceptibility to xanthomonas oryzae pv. oryzae in rice. Int. J. Mol. Sci. 23 (15), 8825. doi: 10.3390/ijms23158825
Son, S., Park, S. R. (2022). Challenges facing CRISPR/Cas9-based genome editing in plants. Front. Plant Sci. 13, 902413. doi: 10.3389/fpls.2022.902413
Srinivasan, B., Gnanamanickam, S. S. (2005). Identification of a new source of resistance in wild rice, oryza rufipogon to bacterial blight of rice caused by Indian strains of xanthomonas oryzae pv. oryzae. Curr. Sci. 88 (8), 1229–1231.
Sun, T., Huang, J., Xu, Y., Verma, V., Jing, B., Sun, Y., et al. (2020). Redundant CAMTA transcription factors negatively regulate the biosynthesis of salicylic acid and n-hydroxypipecolic acid by modulating the expression of SARD1 and CBP60g. Mol. Plant 13 (1), 144–156. doi: 10.1016/j.molp.2019.10.016
Sun, X., Lapin, D., Feehan, J. M., Stolze, S. C., Kramer, K., Dongus, J. A., et al. (2021). Pathogen effector recognition-dependent association of NRG1 with EDS1 and SAG101 in TNL receptor immunity. Nat. Commun. 12 (1), 1–15. doi: 10.1038/s41467-021-23614-x
Tanaka, K., Heil, M. (2021). Damage-associated molecular patterns (DAMPs) in plant innate immunity: applying the danger model and evolutionary perspectives. Annu. Rev. Phytopathol. 59, 53–75. doi: 10.1146/annurev-phyto-082718-100146
Thomma, B. P., Nurnberger, T., Joosten, M. H. (2011). Of PAMPs and effectors: the blurred PTI-ETI dichotomy. Plant Cell 23 (1), 4–15. doi: 10.1105/tpc.110.082602
Timmermann, A., Yun, K. S., Raia, P., Ruan, J., Mondanaro, A., Zeller, E., et al. (2022). Climate effects on archaic human habitats and species successions. Nature 604 (7906), 495–501. doi: 10.1038/s41586-022-04600-9
Ton, J., Mauch-Mani, B. (2004). β-amino-butyric acid-induced resistance against necrotrophic pathogens is based on ABA-dependent priming for callose. Plant J. 38 (1), 119–130. doi: 10.1111/j.1365-313X.2004.02028.x
Torres, M. A. (2010). ROS in biotic interactions. Physiol. Plant. 138 (4), 414–429. doi: 10.1111/j.1399-3054.2009.01326.x
Tsuda, K., Katagiri, F. (2010). Comparing signaling mechanisms engaged in pattern-triggered and effector-triggered immunity. Curr. Opin. Plant Biol. 13 (4), 459–465. doi: 10.1016/j.pbi.2010.04.006
van Wersch, S., Tian, L., Hoy, R., Li, X. (2020). Plant NLRs: the whistleblowers of plant immunity. Plant Commun. 1 (1), 100016. doi: 10.1016/j.xplc.2019.100016
Vaumourin, E., Laine, A.-L. (2018). Role of temperature and coinfection in mediating pathogen life-history traits. Front. Plant Sci. 9, 1670. doi: 10.3389/fpls.2018.01670
Velasquez, A. C., Castroverde, C. D. M., He, S. Y. (2018). Plant-pathogen warfare under changing climate conditions. Curr. Biol. 28 (10), R619–R634. doi: 10.1016/j.cub.2018.03.054
Velásquez, A. C., Castroverde, C. D. M., He, S. Y. (2018). Plant–pathogen warfare under changing climate conditions. Curr. Biol. 28 (10), R619–R634. doi: 10.1016/j.cub.2018.03.054
Wagner, S., Stuttmann, J., Rietz, S., Guerois, R., Brunstein, E., Bautor, J., et al. (2013). Structural basis for signaling by exclusive EDS1 heteromeric complexes with SAG101 or PAD4 in plant innate immunity. Cell Host Microbe 14 (6), 619–630. doi: 10.1016/j.chom.2013.11.006
Wang, Y.-Q., Feechan, A., Yun, B.-W., Shafiei, R., Hofmann, A., Taylor, P., et al. (2009). S-nitrosylation of AtSABP3 antagonizes the expression of plant immunity. J. Biol. Chem. 284 (4), 2131–2137. doi: 10.1074/jbc.M806782200
Wang, W., Feng, B., Zhou, J. M., Tang, D. (2020b). Plant immune signaling: Advancing on two frontiers. J. Integr. Plant Biol. 62 (1), 2–24. doi: 10.1111/jipb.12898
Wang, J., Tian, W., Tao, F., Wang, J., Shang, H., Chen, X., et al. (2020a). TaRPM1 positively regulates wheat high-temperature seedling-plant resistance to puccinia striiformis f. sp. tritici. Front. Plant Sci. 10, 1679. doi: 10.3389/fpls.2019.01679
Wang, J., Wang, J., Li, J., Shang, H., Chen, X., Hu, X. (2021). The RLK protein TaCRK10 activates wheat high-temperature seedling-plant resistance to stripe rust through interacting with TaH2A. 1. Plant J. 108 (5), 1241–1255. doi: 10.1111/tpj.15513
Waszczak, C., Carmody, M., Kangasjärvi, J. (2018). Reactive oxygen species in plant signaling. Annu. Rev. Plant Biol. 69, 209–236. doi: 10.1146/annurev-arplant-042817-040322
Webb, A. A., Hetherington, A. M. (1997). Convergence of the abscisic acid, CO2, and extracellular calcium signal transduction pathways in stomatal guard cells. Plant Physiol. 114 (4), 1557–1560. doi: 10.1104/pp.114.4.1557
Wiermer, M., Feys, B. J., Parker, J. E. (2005). Plant immunity: the EDS1 regulatory node. Curr. Opin. Plant Biol. 8 (4), 383–389. doi: 10.1016/j.pbi.2005.05.010
Williams, A., Pétriacq, P., Schwarzenbacher, R. E., Beerling, D. J., Ton, J. (2018). Mechanisms of glacial-to-future atmospheric CO2 effects on plant immunity. New Phytol. 218 (2), 752–761. doi: 10.1111/nph.15018
Xin, X.-F., Nomura, K., Aung, K., Velásquez, A. C., Yao, J., Boutrot, F., et al. (2016). Bacteria establish an aqueous living space in plants crucial for virulence. Nature 539 (7630), 524–529. doi: 10.1038/nature20166
Yang, S., Cai, W., Shen, L., Wu, R., Cao, J., Tang, W., et al. (2022). Solanaceous plants switch to cytokinin-mediated immunity against ralstonia solanacearum under high temperature and high humidity. Plant Cell Environ. 45 (2), 459–478. doi: 10.1111/pce.14222
Yang, T., Poovaiah, B. (2003). Calcium/calmodulin-mediated signal network in plants. Trends Plant Sci. 8 (10), 505–512. doi: 10.1016/j.tplants.2003.09.004
Yang, S., Shi, Y., Zou, L., Huang, J., Shen, L., Wang, Y., et al. (2020). Pepper CaMLO6 negatively regulates ralstonia solanacearum resistance and positively regulates high temperature and high humidity responses. Plant Cell Physiol. 61 (7), 1223–1238. doi: 10.1093/pcp/pcaa052
Yuan, H.-M., Liu, W.-C., Lu, Y.-T. (2017). CATALASE2 coordinates SA-mediated repression of both auxin accumulation and JA biosynthesis in plant defenses. Cell Host Microbe 21 (2), 143–155. 10.1016/j.chom.2017.01.007
Yuan, P., Poovaiah, B. (2022). Interplay between Ca2+/Calmodulin-mediated signaling and AtSR1/CAMTA3 during increased temperature resulting in compromised immune response in plants. Int. J. Mol. Sci. 23 (4), 2175. doi: 10.3390/ijms23042175
Yuan, P., Tanaka, K., Poovaiah, B. (2021a). Calcium/Calmodulin-mediated defense signaling: What is looming on the horizon for AtSR1/CAMTA3-mediated signaling in plant immunity. Front. Plant Sci. 12, 795353. doi: 10.3389/fpls.2021.795353
Yuan, P., Tanaka, K., Poovaiah, B. (2021b). Calmodulin-binding transcription activator AtSR1/CAMTA3 fine-tunes plant immune response by transcriptional regulation of the salicylate receptor NPR1. Plant Cell Environ. 44 (9), 3140–3154. doi: 10.1111/pce.14123
Zavaliev, R., Mohan, R., Chen, T., Dong, X. (2020). Formation of NPR1 condensates promotes cell survival during the plant immune response. Cell 182 (5), 1093–1108. e1018. doi: 10.1016/j.cell.2020.07.016
Zhang, J., De-oliveira-Ceciliato, P., Takahashi, Y., Schulze, S., Dubeaux, G., Hauser, F., et al. (2018). Insights into the molecular mechanisms of CO2-mediated regulation of stomatal movements. Curr. Biol. 28 (23), R1356–R1363. doi: 10.1016/j.cub.2018.10.015
Zhang, Y., Goritschnig, S., Dong, X., Li, X. (2003). A gain-of-function mutation in a plant disease resistance gene leads to constitutive activation of downstream signal transduction pathways in suppressor of npr1-1, constitutive 1. Plant Cell 15 (11), 2636–2646. doi: 10.1105/tpc.015842
Zhang, Y., Li, X. (2019). Salicylic acid: biosynthesis, perception, and contributions to plant immunity. Curr. Opin. Plant Biol. 50, 29–36. doi: 10.1016/j.pbi.2019.02.004
Zhang, L., Takahashi, Y., Hsu, P.-K., Kollist, H., Merilo, E., Krysan, P. J., et al. (2020). FRET kinase sensor development reveals SnRK2/OST1 activation by ABA but not by MeJA and high CO2 during stomatal closure. Elife 9, e56351. doi: 10.7554/eLife.56351
Zhang, D., Tian, C., Yin, K., Wang, W., Qiu, J.-L. (2019). Postinvasive bacterial resistance conferred by open stomata in rice. Mol. Plant-Microbe Interact. 32 (2), 255–266. doi: 10.1094/MPMI-06-18-0162-R
Zhao, G., Guo, D., Wang, L., Li, H., Wang, C., Guo, X. (2021). Functions of RPM1-interacting protein 4 in plant immunity. Planta 253 (1), 1–11. doi: 10.1007/s00425-020-03527-7
Keywords: carbon dioxide, climate change, crop nutritional security, humidity, pathogen, plant immunity, temperature
Citation: Son S and Park SR (2022) Climate change impedes plant immunity mechanisms. Front. Plant Sci. 13:1032820. doi: 10.3389/fpls.2022.1032820
Received: 31 August 2022; Accepted: 14 November 2022;
Published: 29 November 2022.
Edited by:
Ravi Gupta, Kookmin University, South KoreaReviewed by:
Deepu Pandita, Government Department of School Education, IndiaCopyright © 2022 Son and Park. This is an open-access article distributed under the terms of the Creative Commons Attribution License (CC BY). The use, distribution or reproduction in other forums is permitted, provided the original author(s) and the copyright owner(s) are credited and that the original publication in this journal is cited, in accordance with accepted academic practice. No use, distribution or reproduction is permitted which does not comply with these terms.
*Correspondence: Sang Ryeol Park, c3JwYXJrQGtvcmVhLmty
Disclaimer: All claims expressed in this article are solely those of the authors and do not necessarily represent those of their affiliated organizations, or those of the publisher, the editors and the reviewers. Any product that may be evaluated in this article or claim that may be made by its manufacturer is not guaranteed or endorsed by the publisher.
Research integrity at Frontiers
Learn more about the work of our research integrity team to safeguard the quality of each article we publish.