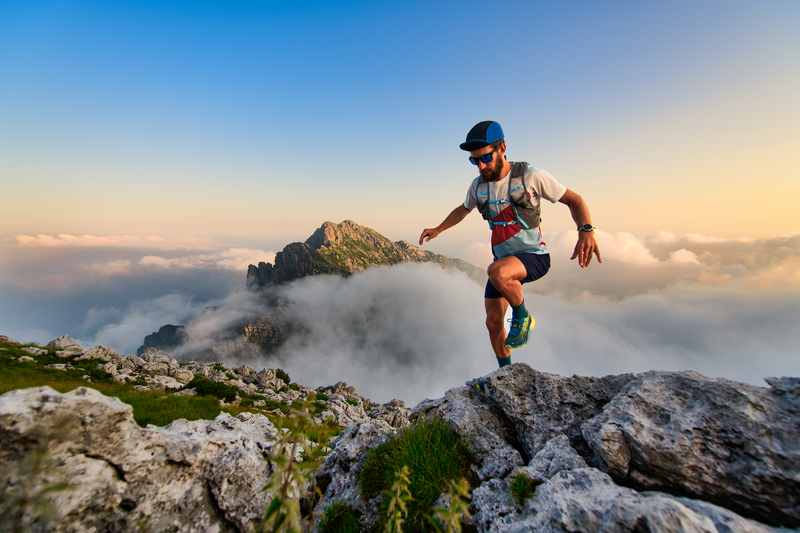
95% of researchers rate our articles as excellent or good
Learn more about the work of our research integrity team to safeguard the quality of each article we publish.
Find out more
REVIEW article
Front. Plant Sci. , 21 October 2022
Sec. Technical Advances in Plant Science
Volume 13 - 2022 | https://doi.org/10.3389/fpls.2022.1029901
This article is part of the Research Topic Advanced Technologies for Energy Saving, Plant Quality Control and Mechanization Development in Plant Factory View all 14 articles
As the unique source of carbon in the atmosphere, carbon dioxide (CO2) exerts a strong impact on crop yield and quality. However, CO2 deficiency in greenhouses during the daytime often limits crop productivity. Crucially, climate warming, caused by increased atmospheric CO2, urges global efforts to implement carbon reduction and neutrality, which also bring challenges to current CO2 enrichment systems applied in greenhouses. Thus, there is a timely need to develop cost-effective and environmentally friendly CO2 enrichment technologies as a sustainable approach to promoting agricultural production and alleviating environmental burdens simultaneously. Here we review several common technologies of CO2 enrichment in greenhouse production, and their characteristics and limitations. Some control strategies of CO2 enrichment in distribution, period, and concentration are also discussed. We further introduce promising directions for future CO2 enrichment including 1) agro-industrial symbiosis system (AIS); 2) interdisciplinary application of carbon capture and utilization (CCU); and 3) optimization of CO2 assimilation in C3 crops via biotechnologies. This review aims to provide perspectives on efficient CO2 utilization in greenhouse production.
Food security requires greater and more consistent crop production against a backdrop of climate change and population growth (Bailey-Serres et al., 2019). Greenhouses offer solutions for protecting crops from extreme weather events and provide more suitable conditions for crop growth than open field cropping (Syed and Hachem, 2019). However, crops grown in greenhouses still suffer from multiple suboptimal conditions, one of which is frequent insufficient CO2 availability, limiting crop yield and quality (Poudel and Dunn, 2017). Due to a relatively airtight environment and crop uptake of CO2, the CO2 concentration in greenhouse drops to only 100~250 µmol mol-1 in the daytime, which is below the ambient CO2 level of 350~450 µmol mol-1 even with effective ventilation, and is far below the optimal concentration required for crop growth, 800~1000 µmol mol-1 (Figure 1; Pascale and Maggio, 2008; Zhang et al., 2014; Merrill et al., 2016).
Figure 1 Schematic diagram of CO2 deficiency in greenhouse production. CO2 in the greenhouse (red curve) accumulates at night due to crop respiration, while the CO2 concentration decreases sharply after light exposure due to CO2 absorption by crops through photosynthesis, which is far below the crop demand (the range within green dotted lines), almost the whole daytime. The blue curve represents the CO2 level in the ambient air outside. The data for plotting the line graphs refer to the text in Zhang et al. (2014); Noctor and Mhamdi (2017); Dong et al. (2018); Li et al. (2018), and figures in Sánchez-Guerrero et al. (2005) and Kläring et al. (2007).
Although various CO2 enrichment technologies have been developed for applications in protected cultivation for decades, CO2 concentration around the crop canopy is still a complex variate in modern agricultural environment control systems (Table 1; Linker et al., 1999; Kläring et al., 2007; Li et al., 2018). Unlike other environmental factors, CO2 needs to be controlled at a micro level (102 ~103 µmol mol-1), and is highly affected by ventilation, plant growth period, and weather (Wang et al., 2016; Li et al., 2018).
Table 1 Application examples of different CO2 enrichment technologies reported in scientific articles.
Currently, the ongoing global climate warming brings challenges to innovating and upgrading existing agricultural CO2 enrichment systems. Several key issues need to be addressed in terms of carbon reduction, such as direct CO2 emissions caused by an imbalance between CO2 supply, crop uptake, and ventilation operation (Vermeulen, 2014; Kozai et al., 2015), and resource consumption during the generation, transportation, storage of pure CO2 (Vermeulen, 2014). Moreover, the promotion of clean energy uses forces greenhouses that obtain CO2 from boiler heating systems to seek new enrichment solutions (Marttila et al., 2021).
Increasing endeavors are being devoted to improving CO2 enrichment in greenhouse production, while comprehensive articles on various techniques and solutions explored in production practices and scientific research are few. Here we review CO2 enrichment technologies and strategies applied in current greenhouse production or laboratory, focusing on their advantages and obstacles, and further summarize three promising directions for future agricultural CO2 enrichment, aiming to provide a sustainable approach to ensure food and climate security through agriculture.
The crops grown in greenhouses are mainly C3 plants, such as tomatoes and cucumbers (Sage, 2017). Due to a lack of efficient mechanisms to cope with CO2 scarcity, C3 crops are more sensitive to changes in CO2 concentrations compared with C4 plants and CAM plants (Long et al., 2015). Importantly, C3 crops have a more positive response to increased CO2 concentrations (Ainsworth and Long, 2020). For instance, a moderate CO2 elevation of 550 ~ 650 µmol mol-1 improves the yield of various C3 crops by an average of 18% (Ainsworth and Long, 2020). Moreover, the CO2 concentration of around 1000 µmol mol-1 promotes the contents of soluble sugar and some nutrients of leafy, fruit and root vegetables by around 10% ~ 60% (Dong et al., 2018). As summarized in Figure 2, elevated CO2 is involved in a multitude of physiological activities in C3 crops including photosynthesis, signaling pathway, organ development, as well as the resistance to biotic and abiotic stresses, and CO2 enrichment further improves the yield and quality, and enhances the utilization efficiency of light and water (Zhang et al., 2015; Hu et al., 2021; Ahammed and Li, 2022). More detailed descriptions can be found in reviews by Xu et al. (2015); Dong et al. (2018); Kazan (2018); Ahammed et al. (2021); Poorter et al. (2021); Roy and Mathur (2021), and Chaudhry and Sidhu (2022).
Figure 2 Schema illustrating effects of elevated CO2 on C3 crops. Elevated CO2 affects a series of plant biological processes (left) in C3 crops, including stomatal development and movement, photosynthesis, and carbon assimilation, signaling pathways, root development and exudate composition, and nutrient acquisition. Meanwhile, macro-production effects (right) can also contribute to promoted yield and quality, enhanced tolerance to abiotic stress and improved resistance to several biotic stresses, which invigorate efficient and safe agricultural production to a certain extent. Created with BioRender.com.
Ventilation allows exchanges of heat and CO2 inside and outside the greenhouse by means of natural ventilation with roof/side windows or forced ventilation (Ishii et al., 2014; Yasutake et al., 2017). Although ventilation can supply CO2 into greenhouses from atmosphere continuously, it is typically to regulate temperature preferentially, and an extra supply of CO2 is necessary for geographically cold regions with restricted ventilation (Stanghellini et al., 2008). Moreover, ventilation alone is not enough to maintain CO2 concentration around crops at an ambient air level (Pascale and Maggio, 2008), and crop yield is more heavily dependent on CO2 at a lower concentration (below 450 µmol mol-1) than a higher concentration (Vermeulen, 2014).
The direct supply of compressed CO2 ensures a stable and clean airflow. However, due to the high market price and transportation cost, it is more commonly used as a complement to other techniques or in scientific research such as Free-Air CO2 Enrichment (FACE) (Sánchez-Guerrero et al., 2005; Allen et al., 2020). In addition, compressed CO2 needs to be equipped with devices for gas storage and pressure control that most often occupy some space in greenhouses (Kuroyanagi et al., 2014; Poudel and Dunn, 2017; Li et al., 2018).
When heating the greenhouse by combustion of natural gas, coal, biomass, and other carbonaceous fuels, CO2 generated during the processes can be delivered to crops or collected and stored for further use (Vermeulen, 2014). As a relatively effective approach to the reduction of carbon emissions and production costs, this technique is adopted widely in current greenhouse production (Dion et al., 2011; Marchi et al., 2018). Moreover, ventilation is often closed during heating, which ensures a better effect of CO2 enrichment (Kläring et al., 2007). A major limitation, however, is that for areas or seasons that do not require heating, burning fuel for CO2 is undesirable.
Given that the gas obtained from the combustion boiler carries too much heat and harmful gases, such as NOx, SO2 and CO, efficient procedures of cooling and purification are essentially required (Roy et al., 2014; Li et al., 2018). In addition, the time and dosage requirements often mismatch between CO2 and heat, resulting in a need for collection and storage devices and flow controllers of CO2 (Dion et al., 2011). Takeya et al. (2017) proposed a system to collect an appropriate amount of CO2 at night when the heating system is turned on and the gas can be released in the daytime when crops have a strong demand for CO2.
Notably, it is an increasingly urgent issue to replace carbonaceous fuels with clean energy to reduce carbon emissions, such as solar energy, hydrogen energy, geothermal energy, and even industrial waste heat (Vermeulen, 2014; Marttila et al., 2021). Meanwhile, the cost of production activities generating carbon emissions has increased drastically. Therefore, greenhouses obtaining CO2 from heating systems are facing a challenge to find alternative CO2 enrichment techniques (Vermeulen, 2014).
The chemical reactions of bicarbonate (such as baking soda) with acid and the decomposition by direct heating are relatively cheap and fast to obtain pure CO2 quantitatively (Syed and Hachem, 2019). The CO2 production rate can be controlled theoretically while the operation in practice is complicated, and a large amount of CO2 generated out of control is wasted and can damage plants (Poudel and Dunn, 2017). Besides, ammonia bicarbonate is sometimes used as a raw material, which can produce by-products used as fertilizers. However, there is a threat of ammonia gas poisoning, so NH3 filtration is mandatory in such cases (Sun et al., 2016).
Decomposition of carbon-rich agricultural wastes by microbial fermentation to release CO2 for crop production is considered a beneficial technology to increase production, reduce agricultural carbon emissions, and lower environmental pollution at the same time (Karim et al., 2020). But there are strict restrictions on C/N ratio, pH, temperature, materials, and other conditions (Jin et al., 2009; Karim et al., 2020). Technologies that use crop-residues and animal-manure composting (CRAM) to increase CO2 were developed to improve vegetable yield and quality (Jin et al., 2009; Karim et al., 2020). Secondary fermentation products could also be reused as a source of CO2 (Liu et al., 2021). Necessary measures should be taken to deal with several weaknesses in compost fermentation, such as 1) associated unpleasant odors; 2) threat of ammonia poisoning (Li et al., 2018); 3) unstable rate of generated CO2 (Karim et al., 2020); and 4) a larger space and more labor input requirements compared with other enrichment techniques (Tang et al., 2022).
The CO2-use efficiency (CUE), defined as the ratio of net photosynthetic rate to CO2 supply rate, suffers from various factors, such as excess supply, natural leakage, sensitive growth state of plants, and other environmental and biological components (Sánchez-Guerrero et al., 2005; Kuroyanagi et al., 2014; Li et al., 2018). The values of CUE in greenhouses are generally lower than 60%, which means that a considerable amount of CO2 is released into the ambient atmosphere (Kozai, 2013; Kuroyanagi et al., 2014). Thereby, numerous attempts have been made on control strategies of CO2 enrichment from various aspects to improve the CUE in the greenhouse.
The uniformity of environmental elements contributes to a unified and efficient management of greenhouse cultivation, while the spatially uneven distribution of CO2 is universal in almost all greenhouse cultivation (Li et al., 2018). Due to the lack of air circulation and the relatively slow diffusion, CO2 concentration is extremely low around the canopy with high leaf density where CO2 is in most demand (Hidaka et al., 2022). Enrichment systems, with single-point outlet, make CO2 more uneven in space, resulting in great waste and failure to meet the production demand (Zhang et al., 2020). Thus, some conveying pipes with holes around the leaves need to be assembled. Hidaka et al. (2022) applied pipe-delivered crop-local CO2 enrichment in strawberry cultivation and achieved increased yield with CO2 supply savings. Another option is by means of internal airflow stirring devices, which is also feasible (Boulard et al., 2017; Syed and Hachem, 2019).
There are various modes in the period setting of CO2 enrichment, such as throughout the day and night, during the daytime, and only in the morning or nighttime. Enrichment throughout the day and night or the whole daytime is generally adopted in controlled chambers for experimental purposes (e.g., Mamatha et al., 2014; Hu et al., 2021). Apparently, it is high energy-consuming and carbon-emitting to elevate CO2 all day in production, especially since the carbon assimilation is typically most intense in the morning of the whole day (Xu et al., 2014). More critically, photosynthetic acclimation can occur with crops over prolonged periods of exposure to elevated CO2 (Wang et al., 2013). Thus, strategies of enriching CO2 only in the morning rather than all daytime have been explored. Treatments of elevating CO2 only in the morning promoted biomass accumulation and flower/fruit quality with no difference from enriching throughout the daytime in some cases (Caliman et al., 2009; Xu et al., 2014). However, another similar strategy of CO2 enrichment with intermittence was found to suppress the promotion of photosynthesis and yield in cotton, wheat, chrysanthemums, soybeans, and tomatoes (Mortensen et al., 1987; Bunce, 2012; Allen et al., 2020). Besides, the effects of nighttime CO2 enrichment are still unclear, which may be species- or cultivar-dependent (Baker et al., 2022).
The concentration gaps between inside and outside the greenhouse (Cin-Cout) and the air exchange rate (dominated by ventilation) are two key factors affecting CUE, besides the crop intrinsic photosynthetic capacity (Kozai et al., 2015; Yasutake et al., 2017). When the setting Cin is higher, e.g., 1000 µmol mol-1, the CUE is less than 50% even in an unventilated greenhouse due to a massive leakage of CO2 (Kuroyanagi et al., 2014). Moderate control systems of CO2 enrichment based on crop absorption rate or Cin-Cout, with a CUE close to 100%, have been reported to improve the yield of cucumbers and tomatoes (Kläring et al., 2007; Kozai et al., 2015). Thus, it is a feasible and sustainable strategy to keep a moderate CO2 concentration slightly higher than the ambient concentration in the cultivation environment, e.g., 550 ~ 650 µmol mol-1, considering economic cost and environmental protection (Vermeule, 2014; Kozai et al., 2015). The resulting gaps in yield and quality compared with crops cultivated in optimal CO2 concentration might be alleviated by controlling other environmental conditions and imposing moderate environmental stresses (Kozai et al., 2015; Dong et al., 2018).
Notably, unlike the consistent conclusion of an increase in yield, the effects of elevated CO2 on crop quality are diverse (Dong et al., 2018), suggesting that the optimal CO2 concentration should be determined by specific production requirements rather than a constant value. Compared with ambient CO2 and a lower CO2 elevation (550 µmol mol-1), the synthesis of glucose and fructose are promoted under higher CO2 concentration (700 - 1000 µmol mol-1), while some amino acids and minerals are deceased (Högy and Fangmeier, 2009; Dong et al., 2018). The changes in health-promoting compounds and flavor substances under elevated CO2, such as flavonoids, lycopene, and ascorbic acid, carotene, are controversial in different vegetable crops, perhaps due to characteristics of different product organs and disturbance of synthesis processes by other environmental conditions (Mamatha et al., 2014; Dong et al., 2018; Hao et al., 2020).
In addition to the challenge of increasing yields and improving quality, the global agricultural production system also faces tremendous pressure to reduce its carbon footprint to mitigate climate change. Even though photosynthesis of crops largely consumes CO2 as the endogenous driving force of agriculture, protected agriculture in various countries and regions is still a carbon emission-intensive process (Marttila et al., 2021; Northrup et al., 2021). Thus, taking full advantage of the crop ability of carbon fixation and combining the advantages of various disciplines should be a sustainable strategy to meet challenges in global food production and climate change simultaneously. In this regard, three novel and potentially feasible directions for future CO2 enrichment (Figure 3) are summarized and discussed as follows.
Figure 3 Overview of directions for future CO2 enrichment in greenhouse production. (A) Interdisciplinary application of carbon capture and utilization. Zeolites/MOFs as carbon adsorption materials can adsorb CO2 molecules from ambient air at low temperatures and desorb them at high temperatures to enrich CO2 into greenhouses. (B) Optimization of CO2 assimilation in C3 crops. Limited CO2 assimilation ability in C3 crops can be improved via biotechnological approaches including gene manipulation and genetic transformation. (C) Agro-Industrial Symbiosis system. A direct connection between the energy industry and greenhouses can be built through a pipeline network to convey CO2 and heat. Created with BioRender.com.
Burning fossil fuels and the operation of non-renewable energy-based industries are being restricted gradually due to their intensive contribution to the majority of global carbon emissions. Strategic management of the agricultural production system has the potential to provide beneficial contributions to the global carbon budget (Marchi et al., 2018; Northrup et al., 2021; Friedlingstein et al., 2022). Thus, a novel agro-industrial symbiosis system (AIS) of channeling industrial waste heat and CO2 to greenhouse productions through pipeline networks is proposed as a viable solution (Marttila et al., 2021). Compared with traditional AIS systems which only transfer heat, this system reduces carbon taxes related to CO2 emissions in industrial processes while increasing revenues of agricultural production (Marchi et al., 2018). Bottlenecks are the initial construction cost and design. The greenhouse needs to be within a limited distance (e.g., 10 km) of the factory, with a matching demand dosage of CO2; and the change of CO2 concentration during the delivery and purification of source gas needs to be considered (Vermeulen, 2014; Marchi et al., 2018).
Carbon dioxide capture, utilization, and storage technologies (CCUS) are being vigorously researched. Compared with the huge cost and risk of leakage of carbon storage, converting CO2 into substances that people need, that is, carbon capture and utilization (CCU), is more attractive (Hepburn et al., 2019). Agriculture has an inherent advantage in this regard owing to the original demand for CO2. But there is a long way to go from now to real applications in agricultural production.
Physical adsorption, with lower energy consumption and milder reaction conditions, may be the most suitable for agricultural production among various methods of carbon capture including absorption solution, calcium looping, membrane technology and microalgal bio-fixation (Ben-Mansour et al., 2016). Target fluid molecules like CO2, can be selectively adsorbed through the huge surface area, specific pore structures, and ions inside the adsorbents (Zhou et al., 2021). Processes of reversible adsorption and desorption are controlled by changing conditions such as temperature and pressure (Ben-Mansour et al., 2016; Zhou et al., 2021).
There are two sources of CO2 capture: 1) industrial exhaust, which is confined and high in concentration; and 2) natural atmosphere, which is widespread and low in concentration. The latter, which is called direct air capture (DAC), is more challenging but also more practically meaningful (Maina et al., 2021). However, the desorption capacity especially required in agricultural production is often overlooked in studies on DAC (Bao et al., 2018). And though there are kinds of adsorbents with various properties for options, the adsorption and desorption capacities are often antagonistic (Zhou et al., 2021). Therefore, the suitable CCU material for agricultural production remains to be explored or transformed.
Most of the control methods of utilizing CCU materials for CO2 enrichment practices in agriculture production are based on variable temperature, as shown in Figure 2. Bao et al. (2018) used a water bath to control the temperature, and calculated that the cost of using 13X zeolite was close to that of the cheapest way of burning natural gas (halved because of the supply of heat), and can be lower considering the carbon tax. Araoz et al. (2021) developed conductive carbon tubes which could realize rapid temperature control of zeolite or metal-organic frameworks (MOFs) filled therein with voltage, providing an application model for greenhouse CO2 enrichment. Tang et al. (2022) reversed the temperature via a rotary regenerative wheel (RAW) loaded with carbon adsorbents, and analyzed the influence of gas flow, rotational speed, and other parameters on its CO2 enrichment performance.
Although related studies are only theoretically feasible with parts of the devices or designs, this direction deserves great attention owing to the carbon neutrality as no CO2 is freshly generated in the whole process. Besides, technological exploration of DAC is receiving increasing interest, and promisingly to play a breakthrough role in agriculture CO2 enrichment technology in the future (Bao et al., 2018).
The requirements of CCU in agriculture systems in future applications can be summarized as follows: 1) strong adsorption in ambient CO2 to provide sufficient pure CO2; 2) sustained desorption to generate a controllable flow of CO2; 3) low energy consumption in desorption or regeneration, such as lower temperature; 4) high adaptability to the agricultural environment with much water vapor and dust to ensure a stable effect in reusing (Wang et al., 2014; Bao et al., 2018).
Except for controlling the environment, modifying plant intrinsic carbon utilization efficiency by altering hereditary substances is a more efficient and revolutionary approach. The capability of CO2 fixation based on photosynthesis is limited especially in C3 plants (Yang et al., 2021). Great efforts have been put into the optimization of the photosynthesis system of C3 crops for decades (Raines, 2011). At present, the complicated mechanism of photosynthesis has been elucidated comprehensively and deeply (Long et al., 2015), preparing the foundation for improving plant carbon fixation via increasingly powerful biotechnologies.
As a crucial restriction enzyme of the C3 cycle, Rubisco catalyzes the binding of CO2 and its receptor ribulose 1,5-bisphosphate (RUBP), with a low activity and competitive dual functions of carboxylation and oxygenation (Long et al., 2015). The latter causes a loss of carbon and nitrogen fixation through photorespiration (Tcherkez, 2013). C4 plants have evolved a transcellular carbon concentration mechanism (CCM) that increases CO2 around rubisco, promoting photosynthetic carbon assimilation and reducing photorespiration significantly; and photosynthetic algae also have CCMs with different organelle structures (Zabaleta et al., 2012). Despite the tall order to introduce a whole CCM into C3 plants, both C4 plants and photosynthetic algae provide vital references and genetic materials for transforming the photosynthetic carbon fixation of C3 crops (Raines, 2011; Rae et al., 2017). For example, overexpression operations of phosphoenolpyruvate carboxylase (PEPC, an enzyme catalyzing the entry of bicarbonate into the C4 cycle), Sedoheptulose-1,7-bisphosphatase (SBPase, an enzyme involved in the regeneration of RUBP) and carbonic anhydrase (CA, an enzyme catalyzing conversion of intracellular CO2 into bicarbonate reversibly) from C4 plants or cyanobacteria, were all found effective to promote photosynthetic capacity in C3 crops (Köhler et al., 2017; Liu et al., 2021; Kandoi et al., 2022).
Naturally, what can be done to improve the CO2 utilization of C3 crops is far beyond modifying the C3 cycle, as listed below:
1) Searching for biological parts of metabolic processes from other organisms (Yang et al., 2021). For example, introducing photorespiratory bypasses from bacteria into rice increased photosynthesis by reducing energy losses in metabolism and releasing CO2 around Rubisco (Wang et al., 2020).
2) Improving the light utilization efficiency by expanding the absorption spectrum of light-harvesting pigments and the photosynthetic electron transport chain, which provide energy to the C3 cycle (Long et al., 2015).
3) Combining with computational modeling. Scientific prediction and analysis would accelerate the understanding and manipulation of complex life activities (Raines, 2011). e.g., Zhao et al. (2021) explained the underlying mechanism of mutual interference of enzymes in the C3 cycle by a dynamic systems model, and pointed out the requirement of balanced activities of enzymes to gain a greater photosynthetic efficiency, which would be further explored by an iterative design-built-test-learn approach (Patron, 2020).
Optimal CO2 concentration has great potential to further improve the yield and quality of agricultural products, especially nowadays when technologies of controlling temperature, light, water, and fertilizer are quite advanced and efficient. Meanwhile, with those intrinsic and emerging conundrums in current CO2 enrichment systems being overcome by multidisciplinary supports, efficient agricultural carbon utilization in greenhouse production would be a promising and sustainable advantageous solution to alleviating the pressure of food security and global warming.
In addition to those mentioned above, directions of improvement in the future agricultural CO2 enrichment can be expanded, such as exploring technologies suitable for open field production, and developing more sensitive sensors and more intelligent CO2 control models on period and concentration for greater CUE. Apart from photosynthesis, the important role of CO2 in plants also needs in-depth studies on mechanisms and improvements of photoadaptation and reduced nutrients requirement under elevated CO2.
KS conceived the paper. AW wrote the paper. KS, JL, and JW revised the paper. All authors contributed to the article and approved the submitted version.
This work was supported by the Natural Science Foundation of Zhejiang Province (LR19C150001), the National Natural Science Foundation of China (32172650), and the Starry Night Science Fund of Zhejiang University Shanghai Institute for Advanced Study (SN-ZJU-SIAS-0011).
The authors declare that the research was conducted in the absence of any commercial or financial relationships that could be construed as a potential conflict of interest.
All claims expressed in this article are solely those of the authors and do not necessarily represent those of their affiliated organizations, or those of the publisher, the editors and the reviewers. Any product that may be evaluated in this article, or claim that may be made by its manufacturer, is not guaranteed or endorsed by the publisher.
Ahammed, G. J., Guang, Y., Yang, Y., Chen, J. (2021). Mechanisms of elevated CO2-induced thermotolerance in plants: the role of phytohormones. Plant Cell Rep. 40, 2273–2286. doi: 10.1007/s00299-021-02751-z
Ahammed, G. J., Li, X. (2022). Elevated carbon dioxide-induced regulation of ethylene in plants. Environ. Exp. Bot. 202, 105025. doi: 10.1016/j.envexpbot.2022.105025
Ainsworth, E. A., Long, S. P. (2020). 30 years of free-air carbon dioxide enrichment (FACE): What have we learned about future crop productivity and its potential for adaptation? Global Change Biol. 27, 27–49. doi: 10.1111/gcb.15375
Allen, L. H., Kimball, B. A., Bunce, J. A., Yoshimoto, M., Harazono, Y., Baker, J. T., et al. (2020). Fluctuations of CO2 in free-air CO2 enrichment (FACE) depress plant photosynthesis, growth, and yield. Agr For. Meteorol. 284, 107899. doi: 10.1016/j.agrformet.2020.107899
Araoz, M., Marcial, A., Gonzalez, J., Avila, A. (2021). Renewable and electroactive biomass-derived tubes for CO2 capture in agroindustrial processes. ACS Sustain. Chem. Eng 9, 7759–7768. doi: 10.1021/acssuschemeng.1c00547
Bailey-Serres, J., Parker, J. E., Ainsworth, E. A., Oldroyd, G. E. D., Schroeder, J. I. (2019). Genetic strategies for improving crop yields. Nature 575, 109–118. doi: 10.1038/s41586-019-1679-0
Baker, J. T., Lascano, R. J., Yates, C., Gitz, III, D. C. (2022). Nighttime CO2 enrichment did not increase leaf area or shoot biomass in cotton seedlings. Agr For. Meteorol. 320, 108931. doi: 10.1016/j.agrformet.2022.108931
Bao, J., Lu, W.-H., Zhao, J., XXXT.Bi, X. (2018). Greenhouses for CO2 sequestration from atmosphere. Carbon Res. Conversion 1, 183–190. doi: 10.1016/j.crcon.2018.08.002
Ben-Mansour, R., Habib, M., Bamidele, O., Basha, M., Qasem, N., Peedikakkal, A., et al. (2016). Carbon capture by physical adsorption: Materials, experimental investigations and numerical modeling and simulations – A review. Appl. Energ. 161, 225–255. doi: 10.1016/j.apenergy.2015.10.011
Boulard, T., Roy, J.-C., Pouillard, J.-B., Fatnassi, H., Grisey, A. (2017). Modelling of micrometeorology, canopy transpiration and photosynthesis in a closed greenhouse using computational fluid dynamics. Biosyst. Eng 158, 110e133. doi: 10.1016/j.biosystemseng.2017.04.001
Bunce, J. A. (2012). Responses of cotton and wheat photosynthesis and growth to cyclic variation in carbon dioxide concentration. Photosynthetica 50, 395–400. doi: 10.1007/s11099-012-0041-7
Caliman, F. R. B., da Silva, D. J. H., Alves, D. P., de Sa Cardoso, T., Mattedi, A. P. (2009). Intermittent CO2 enrichment and analysis of dry matter accumulation and photoassimilate partitioning in tomato. Acta. agronómica. 58, 133–139.
Chaudhry, S., Sidhu, G. (2022). Climate change regulated abiotic stress mechanisms in plants: a comprehensive review. Plant Cell Rep. 41, 1–31. doi: 10.1007/s00299-021-02759-5
Dion, L.-M., Lefsrud, M., Orsat, V. (2011). Review of CO2 recovery methods from the exhaust gas of biomass heating systems for safe enrichment in greenhouses. Biomass Bioenergy 35, 3422–3432. doi: 10.1016/j.biombioe.2011.06.013
Dong, J., Gruda, N., Lam, S. K., Li, X., Duan, Z. (2018). Effects of elevated CO2 on nutritional quality of vegetables: a review. Front. Plant Sci. 9. doi: 10.3389/fpls.2018.00924
Friedlingstein, P., Jones, M. W., O'Sullivan, M., Andrew, R. M., Bakker, D. C., Hauck, J., et al. (2022). Global carbon budget 2021. Earth Syst. Sci. Data 14, 1917–2005. doi: 10.5194/essd-14-1917-2022
Hao, P., Qiu, C., Ding, G., Vincze, E., Zhang, G., Zhang, Y., et al. (2020). Agriculture organic wastes fermentation CO2 enrichment in greenhouse and the fermentation residues improve growth, yield and fruit quality in tomato. J. Clean Prod. 275, 123885. doi: 10.1016/j.jclepro.2020.123885
Hepburn, C., Adlen, E., Beddington, J., Carter, E. A., Fuss, S., Dowell, N., et al. (2019). The technological and economic prospects for CO2 utilization and removal. Nature 575, 87–97. doi: 10.1038/s41586-019-1681-6
Hidaka, K., Nakahara, S., Yasutake, D., Zhang, Y., Okayasu, T., Dan, K., et al. (2022). Crop-local CO2 enrichment improves strawberry yield and fuel use efficiency in protected cultivations. Sci. Hortic. 301 (111104), 1–10. doi: 10.1016/j.scienta.2022.111104
Högy, P., Fangmeier, A. (2009). Atmospheric CO2 enrichment affects potatoes: 2. tuber quality traits. Eur. J. Agron. 30, 85–94. doi: 10.1016/j.eja.2008.07.006
Hu, Z., Ma, Q., Foyer, C. H., Lei, C., Choi, H. W., Zheng, C., et al. (2021). High CO2-and pathogen-driven expression of the carbonic anhydrase βCA3 confers basal immunity in tomato. New Phytol. 229, 2827–2843. doi: 10.1111/nph.17087
Ishii, M., Okushima, L., Moriyama, H., Sase, S., Fukuchi, N. (2014). And both, a Experimental study of natural ventilation in an open-roof greenhouse during the summer. J XXIX Int. Hortic. Congress Horticulture: Sustaining Lives Livelihoods Landscapes (IHC2014): Int. Symposium Innovation New Technol. Protected Cropping 1107, 67–74. doi: 10.17660/ActaHortic.2015.1107.8
Jaffrin, A., Bentounes, N., Joan, A. M., Makhlouf, S. (2003). Landfill biogas for heating greenhouses and providing carbon dioxide supplement for plant growth. Biosyst. Eng 86, 113–123. doi: 10.1016/s1537-5110(03)00110-7
Jin, C., Du, S., Wang, Y., Condon, J., Lin, X., Zhang, Y. (2009). Carbon dioxide enrichment by composting in greenhouses and its effect on vegetable production. J. Plant Nutr. Soil Sci. 172, 418–424. doi: 10.1002/jpln.200700220
Kandoi, D., Ruhil, K., Govindjee, G., Tripathy, B. C. (2022). Overexpression of cytoplasmic C4Flaveria bidentis carbonic anhydrase in C3Arabidopsis thaliana increases amino acids, photosynthetic potential, and biomass. Plant Biotechnol. J. 20, 1518–1532. doi: 10.1111/pbi.13830
Karim, M. F., Hao, P., Nordin, N. H. B., Qiu, C., Zeeshan, M., Khan, A. A., et al. (2020). Effects of CO2 enrichment by fermentation of CRAM on growth, yield and physiological traits of cherry tomato. Saudi J. Biol. Sci. 27, 1041–1048. doi: 10.1016/j.sjbs.2020.02.020
Kazan, K. (2018). Plant-biotic interactions under elevated CO2: A molecular perspective. Environ. Exp. Bot. 153, 249–261. doi: 10.1016/j.envexpbot.2018.06.005
Kläring, H. P., Hauschild, C., Heißner, A., Bar-Yosef, B. (2007). Model-based control of CO2 concentration in greenhouses at ambient levels increases cucumber yield. Agr For. Meteorol. 143, 208–216. doi: 10.1016/j.agrformet.2006.12.002
Köhler, I. H., Ruiz-Vera, U. M., VanLoocke, A., Thomey, M. L., Clemente, T., Long, S. P., et al. (2017). Expression of cyanobacterial FBP/SBPase in soybean prevents yield depression under future climate conditions. J. Exp. Bot. 68, 715–726. doi: 10.1093/jxb/erw435
Kozai, T. (2013). Resource use efficiency of closed plant production system with artificial light: Concept, estimation and application to plant factory. Proc. Jpn. Acad. Ser. B Phys. Biol. Sci. 89, 447–461. doi: 10.2183/pjab.89.447
Kozai, T., Kubota, C., Takagaki, M., Maruo, T. (2015). Greenhouse environment control technologies for improving the sustainability of food production. Acta Hortic. 1107, 1–13. doi: 10.17660/ActaHortic.2015.1107.1
Kuroyanagi, T., Yasuba, K., Higashide, T., Iwasaki, Y., Takaichi, M. (2014). Efficiency of carbon dioxide enrichment in an unventilated greenhouse. Biosyst. Eng 119, 58–68. doi: 10.1016/j.biosystemseng.2014.01.007
Li, Y., Ding, Y., Li, D., Miao, Z. (2018). Automatic carbon dioxide enrichment strategies in the greenhouse: A review. Biosyst. Eng 171, 101–119. doi: 10.1016/j.biosystemseng.2018.04.018
Linker, R., Gutman, P. O., Seginer, I. (1999). Robust controllers for simultaneous control of temperature and CO2 concentration in greenhouses. Control Eng Pract. 7, 851–862. doi: 10.1016/S0967-0661(99)00042-8
Liu, D., Hu, R., Zhang, J., Guo, H. B., Cheng, H., Li, L., et al. (2021). Overexpression of an Agave phosphoenolpyruvate carboxylase improves plant growth and stress tolerance. Cells 10, 582. doi: 10.3390/cells10030582
Long, S. P., Marshall-Colon, A., Zhu, X. G. (2015). Meeting the global food demand of the future by engineering crop photosynthesis and yield potential. Cell 161, 56–66. doi: 10.1016/j.cell.2015.03.019
Maina, J. W., Pringle, J. M., Razal, J. M., Nunes, S., Vega, L., Gallucci, F., et al. (2021). Strategies for integrated capture and conversion of CO2 from dilute flue gases and the atmosphere. ChemSusChem 14, 1805–1820. doi: 10.1002/cssc.202100010
Mamatha, H., Rao, N. S., Laxman, R., Shivashankara, K., Bhatt, R., Pavithra, K. (2014). Impact of elevated CO2 on growth, physiology, yield, and quality of tomato (Lycopersiconesculentum mill) cv. ArkaAshish Photosynthetica 52, 519–528. doi: 10.1007/s11099-014-0059-0
Marchi, B., Zanoni, S., Pasetti, M. (2018). Industrial symbiosis for greener horticulture practices: the CO2 enrichment from energy intensive industrial processes. Proc. CIRP 69, 562–567. doi: 10.1016/j.procir.2017.11.117
Marttila, M. P., Uusitalo, V., Linnanen, L., Mikkilä, M. H. (20219040). Agro-industrial symbiosis and alternative heating systems for decreasing the global warming potential of greenhouse production. Sustainability-Basel 13, 9040. doi: 10.3390/su13169040
Merrill, B. F., Lu, N., Yamaguchi, T., Takagaki, M., Maruo, T., Kozai, T., et al. (2016). Next evolution of agriculture: a review of innovations in plant factories. Handb. Photosynthesis, 723–740.
Mortensen, L. M. (1987). Review: CO2 enrichment in greenhouses. crop responses. Sci. Hortic. 33, 1–25. doi: 10.1016/0304-4238(87)90028-8
Noctor, G., Mhamdi, A. (2017). Climate change, CO2, and defense: the metabolic, redox, and signaling perspectives. Trends Plant Sci. 22, 857–870. doi: 10.1016/j.tplants.2017.07.007
Northrup, D. L., Basso, B., Wang, M. Q., Morgan, C. L. S., Benfey, P. N. (2021). Novel technologies for emission reduction complement conservation agriculture to achieve negative emissions from row-crop production. Proc. Natl. Acad. Sci. U. S. A. 118, e2022666118. doi: 10.1073/pnas.2022666118
Pascale, S., Maggio, A. (2008). Plant stress management in semiarid greenhouse. international workshop on greenhouse environmental control and crop production. Semi-Arid Regions 797, 205–215. doi: 10.17660/ActaHortic.2008.797.28
Patron, N. J. (2020). Beyond natural: synthetic expansions of botanical form and function. New Phytol. 227, 295–310. doi: 10.1111/nph.16562
Pérez-López, U., Miranda-Apodaca, J., Lacuesta, M., Mena-Petite, A., Muñoz-Rueda, A. (2015). Growth and nutritional quality improvement in two differently pigmented lettuce cultivars grown under elevated CO2 and/or salinity. Sci. Hortic. 195, 56–66. doi: 10.1016/j.scienta.2015.08.034
Poorter, H., Knopf, O., Wright, I., Temme, A., Hogewoning, S., Graf, A., et al. (2021). A meta-analysis of responses of C3 plants to atmospheric CO2: dose–response curves for 85 traits ranging from the molecular to the whole-plant level. New Phytol. 223, 1560–1596. doi: 10.1111/nph.17802
Poudel, M., Dunn, B. (2017). Greenhouse carbon dioxide supplementation. Oklahoma Cooperative Extension Service, HLA–6723.
Rae, B. D., Long, B. M., Förster, B., Nguyen, N. D., Velanis, C. N., Atkinson, N., et al. (2017). Progress and challenges of engineering a biophysical CO2-concentrating mechanism into higher plants. J. Exp. Bot. 68, 3717–3737. doi: 10.1093/jxb/erx133
Raines, C. A. (2011). Increasing photosynthetic carbon assimilation in C3 plants to improve crop yield: current and future strategies. Plant Physiol. 155, 36–42. doi: 10.1104/pp.110.168559
Roy, Y., Lefsrud, M., Orsat, V., Filion, F., Bouchard, J., Nguyen, Q., et al. (2014). Biomass combustion for greenhouse carbon dioxide enrichment. Biomass Bioenergy 66, 186e196. doi: 10.1016/j.biombioe.2014.03.001
Roy, S., Mathur, P. (2021). Delineating the mechanisms of elevated CO2 mediated growth, stress tolerance and phytohormonal regulation in plants. Plant Cell Rep. 40, 1345–1365. doi: 10.1007/s00299-021-02738-w
Sage, R. (2017). A portrait of the C4 photosynthetic family on the 50th anniversary of its discovery: species number, evolutionary lineages, and hall of fame. J. Exp. Bot. 68, e11–e28. doi: 10.1093/jxb/erx005
Sánchez-Guerrero, M. C., Lorenzo, P., Medrano, E., Castilla, N., Soriano, T., Baille, A. (2005). Effect of variable CO2 enrichment on greenhouse production in mild winter climates. Agr For. Meteorol. 132, 244–252. doi: 10.1016/j.agrformet.2005.07.014
Stanghellini, C., Incrocci, L., Gázquez, J. C., Dimauro, B. (2008). Carbon dioxide concentration in Mediterranean greenhouses: How much lost production? Acta Hortic. 801, 1541–1550. doi: 10.17660/ActaHortic.2008.801.190
Sun, Q., Cui, S., Song, Y., Yang, Z., Dong, Q., Sun, S., et al. (2016). Impact of illumination and temperature performance of blanket-inside solar greenhouse and CO2 enrichment on cuumber growth and development. Coll. Agronomy Inner Mongolia Agric. Univ. Agricultural Science & Technology. 17, 1757–1761+1776. doi: 10.16175/j.cnki.1009-4229.2016.08.001
Syed, A., Hachem, C. (2019). Review of design trends in lighting, environmental controls, carbon dioxide supplementation, passive design, and renewable energy systems for agricultural greenhouses. J. Biosyst. Eng. 44, 28–36. doi: 10.1007/s42853-019-00006-0
Takeya, S., Muromachi, S., Maekawa, T., Yamamoto, Y., Mimachi, H., Kinoshita, T., et al. (2017). Design of ecological CO2 enrichment system for greenhouse production using TBAB + CO2 semi-clathrate hydrate. Energies 10, 927. doi: 10.3390/en10070927
Tang, C., Gao, X., Shao, Y., Wang, L., Liu, K., Gao, R., et al. (2022). Investigation on the rotary regenerative adsorption wheel in a new strategy for CO2 enrichment in greenhouse. Appl. Therm Eng 205 (118043), 1–13. doi: 10.1016/j.applthermaleng.2022.118043
Tcherkez, G. (2013). Modelling the reaction mechanism of ribulose-1,5-bisphosphate carboxylase/oxygenase and consequences for kinetic parameters. Plant Cell Environ. 36, 1586–1596. doi: 10.1111/pce.12066
Vermeulen, P. (2014). Alternative sources of CO2 for the greenhouse horticulture. Proc. 2nd Int. Symposium Energy Challenges Mechanics (ISECM2) (Aberdeen, UK) 19–21.
Wang, L., Feng, Z., Schjoerring, J. (2013). Effects of elevated atmospheric CO2 on physiology and yield of wheat (Triticum aestivum l.): A meta-analytic test of current hypotheses. Agric. Ecosyst. Environ. 178, 57–63. doi: 10.1016/j.agee.2013.06.013
Wang, T., Huang, J., He, X., Wu, J., Fang, M., Cheng, J. (2014). CO2 fertilization system integrated with a low-cost direct air capture technology. Energy Proc. 63, 6842–6851. doi: 10.1016/j.egypro.2014.11.718
Wang, J., Niu, X., Zheng, L., Zheng, C., Wang, Y. (20161941). Wireless mid-infrared spectroscopy sensor network for automatic carbon dioxide fertilization in a greenhouse environment. Sensors 16 (1941), 1–20. doi: 10.3390/s16111941
Wang, L.-M., Shen, B.-R., Li, B.-D., Zhang, C.-L., Lin, M., Tong, P.-P., et al. (2020). A synthetic photorespiratory shortcut enhances photosynthesis to boost biomass and grain yield in rice. Mol. Plant 13, 1802–1815. doi: 10.1016/j.molp.2020.10.007
Xu, Z., Jiang, Y., Zhou, G. (2015). Response and adaptation of photosynthesis, respiration, and antioxidant systems to elevated CO2 with environmental stress in plants. Front. Plant Sci. 6. doi: 10.3389/fpls.2015.00701
Xu, S., Zhu, X., Li, C., Ye, Q. (2014). Effects of CO2 enrichment on photosynthesis and growth in Gerbera jamesonii. Sci. Hortic. 177, 77–84. doi: 10.1016/j.scienta.2014.07.022
Yang, X., Liu, D., Lu, H., Weston, D., Chen, J.-G., Muchero, W., et al. (2021). Biological parts for plant biodesign to enhance land-based carbon dioxide removal. BioDesign Res. 2021, 1–22. doi: 10.34133/2021/9798714
Yasutake, D., Tanioka, H., Ino, A., Takahashi, A., Yokoyama, T., Mori, M., et al. (2017). Dynamic evaluation of natural ventilation characteristics of a greenhouse with CO2 enrichment. Acad. J. Agric. Res. 5, 312–319. doi: 10.15413/ajar.2017.0164
Zabaleta, E., Martin, V., Braun, H.-P. (2012). A basal carbon concentrating mechanism in plants? Plant Sci. 187, 97–104. doi: 10.1016/j.plantsci.2012.02.001
Zhang, S., Li, X., Sun, Z., Shao, S., Hu, L., Ye, M., et al. (2015). Antagonism between phytohormone signalling underlies the variation in disease susceptibility of tomato plants under elevated CO2. J. Exp. Bot. 66, 1951–1963. doi: 10.1093/jxb/eru538
Zhang, Z., Liu, L., Zhang, M., Zhang, Y., Wang, Q. (2014). Effect of carbon dioxide enrichment on health-promoting compounds and organoleptic properties of tomato fruits grown in greenhouse. Food Chem. 153, 157–163. doi: 10.1016/j.foodchem.2013.12.052
Zhang, Y., Yasutake, D., Hidaka, K., Kitano, M., Okayasu, T. (2020). CFD analysis for evaluating and optimizing spatial distribution of CO2 concentration in a strawberry greenhouse under different CO2 enrichment methods. Comput. Electron. Agric. 179, 105811. doi: 10.1016/j.compag.2020.105811
Zhao, H., Tang, Q., Chang, T., Xiao, Y., Zhu, X.-G. (2021). Why an increase in activity of an enzyme in the Calvin–Benson cycle does not always lead to an increased photosynthetic CO2 uptake rate?–a theoretical analysis. silico Plants 3, diaa009. doi: 10.1093/insilicoplants/diaa009
Keywords: carbon dioxide, elevated CO2, controlled environment agriculture, horticulture, agro-industrial symbiosis system, carbon capture and utilization, CO2 assimilation
Citation: Wang A, Lv J, Wang J and Shi K (2022) CO2 enrichment in greenhouse production: Towards a sustainable approach. Front. Plant Sci. 13:1029901. doi: 10.3389/fpls.2022.1029901
Received: 28 August 2022; Accepted: 07 October 2022;
Published: 21 October 2022.
Edited by:
Yuxin Tong, Institute of Environment and Sustainable Development in Agriculture (CAAS), ChinaReviewed by:
Toyoki Kozai, Japan Plant Factory Association, JapanCopyright © 2022 Wang, Lv, Wang and Shi. This is an open-access article distributed under the terms of the Creative Commons Attribution License (CC BY). The use, distribution or reproduction in other forums is permitted, provided the original author(s) and the copyright owner(s) are credited and that the original publication in this journal is cited, in accordance with accepted academic practice. No use, distribution or reproduction is permitted which does not comply with these terms.
*Correspondence: Kai Shi, a2Fpc2hpQHpqdS5lZHUuY24=
Disclaimer: All claims expressed in this article are solely those of the authors and do not necessarily represent those of their affiliated organizations, or those of the publisher, the editors and the reviewers. Any product that may be evaluated in this article or claim that may be made by its manufacturer is not guaranteed or endorsed by the publisher.
Research integrity at Frontiers
Learn more about the work of our research integrity team to safeguard the quality of each article we publish.