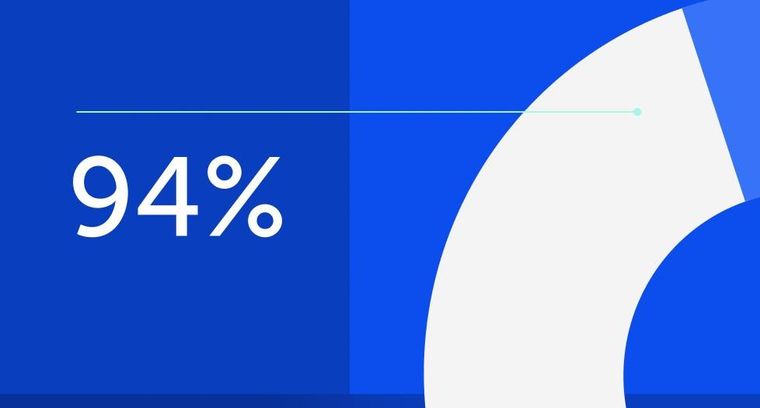
94% of researchers rate our articles as excellent or good
Learn more about the work of our research integrity team to safeguard the quality of each article we publish.
Find out more
ORIGINAL RESEARCH article
Front. Plant Sci., 06 October 2022
Sec. Crop and Product Physiology
Volume 13 - 2022 | https://doi.org/10.3389/fpls.2022.1027551
This article is part of the Research TopicForage Crop Improvement for Improved Livestock Production and NutritionView all 16 articles
Transporters belonging to the natural resistance-associated macrophage protein (Nramp) family play important roles in metal uptake and homeostasis. Although Nramp members have been functionally characterized in plants, the role of Nramp in the important tropical forage legume Stylosanthes guianensis (stylo) is largely unknown. This study aimed to determine the responses of Nramp genes to metal stresses and investigate its metal transport activity in stylo. Five SgNramp genes were identified from stylo. Expression analysis showed that SgNramp genes exhibited tissue preferential expressions and diverse responses to metal stresses, especially for manganese (Mn), suggesting the involvement of SgNramps in the response of stylo to metal stresses. Of the five SgNramps, SgNramp1 displayed the highest expression in stylo roots. A close correlation between SgNramp1 expression and root Mn concentration was observed among nine stylo cultivars under Mn limited condition. The higher expression of SgNramp1 was correlated with a high Mn uptake in stylo. Subsequent subcellular localization analysis showed that SgNramp1 was localized to the plasma membrane. Furthermore, heterologous expression of SgNramp1 complemented the phenotype of the Mn uptake-defective yeast (Saccharomyces cerevisiae) mutant Δsmf1. Mn concentration in the yeast cells expressing SgNramp1 was higher than that of the empty vector control, suggesting the transport activity of SgNramp1 for Mn in yeast. Taken together, this study reveals that SgNramp1 is a plasma membrane–localized transporter responsible for Mn uptake in stylo.
Trace metals, such as manganese (Mn), iron (Fe), zinc (Zn), and copper (Cu), are essential elements for plant growth and productivity. These metal ions involve in multiple physiological and biochemical processes in plants, such as stabilizing the structure of many biomacromolecules and serve as cofactors for key proteins and enzymes (Marschner, 2012). For example, Mn is an essential component in the Mn cluster of the oxygen-evolving complex in photosystem II (PSII) that is required for the electron transport chain in photosynthesis. Mn also acts as a critical cofactor of numerous enzymes, such as superoxide dismutase (SOD), catalase (CAT), decarboxylases, and RNA polymerases (Chen and Li, 2021). Despite its necessity at a low dose, the metal ions presented at excess levels can be harmful to plants, which are similar to the toxic effects of non-essential heavy metals, such as aluminum (Al), cadmium (Cd), arsenic (As), and lead (Pb). The excess amount of trace elements and heavy metals can cause phytotoxicity to plant cells, such as triggering oxidative stress, inhibiting enzyme activity, impeding the structure and function of photosynthetic apparatus, and damaging respiration and energy metabolism, ultimately inhibiting plant growth (Fryzova et al., 2018; Huang et al., 2020; Riyazuddin et al., 2021).
To maintain normal growth, plants have to regulate metal uptake and homeostasis in response to the fluctuated metal status in soils from deficient to toxic levels, which can be achieved via a variety of metal transporters, such as members of the natural resistance-associated macrophage protein (Nramp), Zn-regulated transporter/Fe-regulated transporter-like protein (ZRT/IRT), metal tolerance protein (MTP), and heavy metal ATPase (HMA) (Wang et al., 2020; Kaur et al., 2021; Pottier et al., 2022). Among these metal transporters, Nramp, an integral membrane protein, is a critical transporter for divalent metals. Most Nramp proteins include a unique motif and 10–12 conserved transmembrane domains (TMDs) (Li et al., 2021b). Nramp proteins have been characterized to transport a wide range of metal ions across the cellular membrane, including Mn, Fe, Zn, Cd, or Al (Li et al., 2019). To date, a set of Nramp members have been identified in various plants, such as Arabidopsis (Thomine et al., 2000; Li et al., 2022), rice (Oryza sativa) (Xia et al., 2010; Sasaki et al., 2012; Mani and Sankaranarayanan, 2018; Chang et al., 2020), Medicago truncatula (Tejada-Jiménez et al., 2015), soybean (Glycine max) (Kaiser et al., 2003; Qin et al., 2017), barley (Hordeum vulgare) (Wu et al., 2016) and tobacco (Nicotiana tabacum) (Liu et al., 2022).
For example, six Nramp members are identified in Arabidopsis, and five of them have been functionally characterized (Gao et al., 2018; Li et al., 2022). Among them, AtNramp1 is mainly expressed in roots and is a plasma membrane (PM)–localized transporter responsible for the high-affinity Mn uptake in Arabidopsis (Cailliatte et al., 2010). In addition to Mn, AtNramp1 can also transport Fe and cobalt (Co) in vivo (Curie et al., 2000; Cailliatte et al., 2010). AtNramp2 is found to be localized to the trans-Golgi network and is implicated in remobilization of Mn in Golgi, which is required for Arabidopsis root growth under Mn deficiency (Gao et al., 2018). It has been demonstrated that both AtNramp3 and AtNramp4 are induced by Fe deficiency and are essential for vacuolar Fe mobilization during seed germination in Arabidopsis (Thomine et al., 2003; Lanquar et al., 2005). Both AtNramp3 and AtNramp4 can rescue the growth of the yeast (Saccharomyces cerevisiae) mutant Δsmf1, which is defective in Mn uptake (Thomine et al., 2000); mutation analysis reveals the roles of AtNramp3 and AtNramp4 in intracellular Mn homeostasis in Arabidopsis (Lanquar et al., 2010). AtNramp6 can transport Fe and Cd in yeast cells, and disruption of AtNramp6 reduces lateral root growth in Arabidopsis under Fe deficiency (Cailliatte et al., 2009; Li et al., 2019). Recently, it has been reported that AtNramp6 together with AtNramp1 is cooperatively involved in Mn utilization in Arabidopsis (Li et al., 2022). The transport activities of Nramp homologues for various metal ions are also demonstrated in other plant species, including OsNramps from rice for Mn, Fe, Cd and Al (Xia et al., 2010; Yamaji et al., 2013; Peris-Peris et al., 2017; Li et al., 2021c), MtNramp1 from M. truncatula for Fe and Mn (Tejada-Jiménez et al., 2015), HvNramp5 from barley for Mn and Cd (Wu et al., 2016), MhNramp1 from apple (Malus hupehensis) for Cd (Zhang et al., 2020), FeNramp5 from buckwheat (Fagopyrum esculentum) for Mn and Cd (Yokosho et al., 2021), and NtNramp1 from tobacco for Fe and Cd (Liu et al., 2022).
Although the functions of Nramp homologues have been characterized and analyzed in different plants, the role of the Nramp members in metal transport activity remains to be elucidated in the tropical forage legume, Stylosanthes guianensis (stylo). Stylo is an acid soil adapted, pioneer forage legume, which is widely used for multipurpose including land reclamation and restoration, soil quality improvement, and fodder for farm animals (Tang et al., 2009; Marques et al., 2018; Guo et al., 2019). Stylo displays superior level of tolerance to nutrient and metal stresses that are common in acid soils of the tropics, including phosphorus (P) deficiency, excess Mn stress, and Al toxicity (Sun et al., 2014; Chen et al., 2015; Chen et al., 2021). Considering the key role of Nramp in metal ion uptake and homeostasis, this study aimed to determine the responses of Nramp genes to metal stress and investigate its roles in metal transport activity. Five SgNramps were identified from stylo and their expression patterns were analyzed. A PM-localized transporter SgNramp1 was further demonstrated to be involved in Mn uptake. The results of this study provide a candidate gene for breeding stylo cultivars with high-efficient Mn uptake.
Five Nramp genes were isolated from the previously reported transcriptomic data in stylo (Jiang et al., 2018; Jia et al., 2020; Chen et al., 2021). The full length of each Nramp was amplified from the cDNA library stock of stylo roots (Song et al., 2022). These five Nramp genes were named from SgNramp1 to SgNramp5 under GenBank accession numbers ON338040, ON338041, ON338042, ON338043, and ON338044, respectively. ClustalX and MAGA4 were used to perform multiple alignment and phylogenetic analyses, respectively. TMD was predicted by the HMMER (https://www.ebi.ac.uk/Tools/hmmer/search/hmmscan). The conserved motifs of Nramp proteins were analyzed by MEME (https://meme-suite.org/meme/tools/meme) and Pfam (http://pfam.xfam.org/search/sequence) programs with default parameters.
In this study, the stylo cultivar “Reyan NO.5” was used for gene expression analysis. Stylo seeds were sown on moistened filter papers at 25°C in the dark for 2–3 days. The germinated seedlings were then transplanted into Hoagland solution as previously described (Song et al., 2022). The macronutrients and micronutrients in the modified Hoagland solution included 2 mM Ca(NO3)2, 3 mM KNO3, 0.25 mM KH2PO4, 0.5 mM MgSO4, 25 μM MgCl2, 80 μM Fe-Na-EDTA, 5 μM MnSO4, 0.5 μM ZnSO4, 1.5 μM CuSO4, 0.09 μM (NH4)6Mo7O24, and 23 μM Na2B4O7. The pH of the solution was adjusted to 5.8 every 2 days. Stylo seedlings were grown in a greenhouse at 25–32°C under normal sunlight conditions with a photoperiod of about 13h light. For expression analysis of SgNramps, root, stem, and leaf tissues were harvested at 21 days of growth, whereas flower and seed were harvested at 120 days and 140 days, respectively.
To investigate the responses of SgNramps to deficiencies of Mn, Fe, Zn, and Cu, the 14-day-old stylo seedlings precultured in half-strength Hoagland solution were transferred to nutrient solution without Mn, Fe, Zn, or Cu application for 7 days. Leaf and root were harvested and used to gene expression analysis. To analyze the effects of excess Mn, Fe, Zn, and Cu treatments on the transcripts of SgNramps, the 14-day-old stylo seedlings precultured in half-strength Hoagland solution were transplanted into fresh Hoagland solution supplied with 400 μM MnSO4, 800 μM Fe-Na-EDTA, 20 μM ZnSO4, or 10 μM CuSO4 for excess Mn, Fe, Zn, or Cu treatments, respectively. After 7 days of treatments, leaf and root were harvested and used for gene expression analysis. The stylo seedlings grown in full-strength Hoagland solution for 21 days were set as the control treatment.
In addition, nine stylo cultivars belonging to S. guianensis, including “Reyan NO.2”, “Reyan NO.5”, “Reyan NO.10”, “Reyan NO.13”, “Reyan NO.18,” “Reyan NO.20”, “Reyan NO.21”, “Reyan NO.22”, and “Reyan NO.24”, were used to evaluate the genotypic variations in SgNramp1 expression. Fourteen-day-old stylo seedlings precultured in half-strength Hoagland solution as described above were transplanted into fresh Hoagland solution supplied with 0.1 or 5 μM MnSO4 regarding as low Mn and normal Mn treatments, respectively. After 7 days of Mn treatments, roots were harvested for SgNramp1 expression and Mn concentration analyses.
Total RNA was extracted using TRIzol reagent (TIANGEN Biotech, China), and then it was used to synthesize first strand cDNA via the HiScript III cDNA synthesis kit (Vazyme, Nanjing, China). The synthesized cDNA was further used to perform quantitative real-time polymerase chain reaction (qRT-PCR) analysis using SYBR Green Master mix reagent (Vazyme, Nanjing, China). qRT-PCR reaction was detected by a QuantStudio™ 6 Flex Real-Time equipment (Thermo Fisher Scientific, Massachusetts, USA). Gene specific primers for qRT-PCR analysis are detailed in Supplementary Table S1. The expression of each SgNramp was calculated relative to the housekeeping gene SgEF-1a (Song et al., 2022). Three biological replicates were included in this experiment.
After samples thoroughly dried in an oven for 7 days, the dried samples were digested with concentrated nitric acid at 140°C. Mn concentration in the digested solution was determined by atomic absorption spectroscopy. The standard reference material (GBW07603) was used to validate Mn determination as previously described (Li et al., 2021a).
The open reading frame (ORF) of SgNramp1 was amplified by PCR using SgNramp1-GFP primers (Supplementary Table S1). The PCR product was cloned into the N-terminus of the green fluorescent protein (GFP) of the pBWA(V)HS vector (Li et al., 2021a). The constructs were introduced into Agrobacterium tumefaciens strain Gv3101 and were then transiently expressed in leaves of 5-week-old tobacco (Nicotiana benthamiana) seedlings according to Li et al. (2021). The PM marker (OsMCA1) (Kurusu et al., 2012) fused with red fluorescence protein (mKATE) was used to co-localization with SgNramp1 or empty vector. The fluorescence was detected by a confocal laser scanning TCS SP8 microscopy (Leica, Wetzlar, Germany). GFP fluorescence was detected at 500–530 nm, whereas the red fluorescence was detected at 580–630 nm.
In this study, the Mn uptake-defective mutant Δsmf1 (MATa; his3Δ1; leu2Δ0; met15Δ0; ura3Δ0; YOL122c::kanMX4), the Fe uptake-defective mutant Δfet3fet4 (MATa; his3Δ1; leu2Δ0; met15Δ0; ura3Δ0; YMR058w::kanMX4) and the Cd-sensitive mutant Δycf1 (MATa; his3Δ1; leu2Δ0; met15Δ0; ura3Δ0; YDR135c::kanMX4) were obtained from the Euroscarf. The ORF of SgNramp1 was amplified using SgNramp1-pYES2 primers (Supplementary Table S1). The amplified product was cloned into the yeast expression vector pYES2 (Invitrogen, Carlsbad, United States). The construct and empty vector were then introduced into the above yeast mutants using the LiOAc/PEG method. The yeast transformants were incubated in a liquid synthetic complete (SC–U/Glu) medium consisting of yeast nitrogen base, glucose, and amino acids without uracil at 30°C for about 6 h. After optical density (OD600) of the yeast cells reached to 0.6, the yeast cells were diluted to an OD600 of 0.2 and three 10-fold serial dilutions were prepared. Then, 10 μl of each dilution was spotted onto the induction SC–U plates containing 2% (w) galactose (Gal) and 1% raffinose according to Li et al. (2021a). For Mn transport analysis in Δsmf1, the plate was added with 0, 20, and 25 mM Mn-chelator ethylene glycol tetraacetic acid (EGTA). For Fe transport analysis in Δfet3fet4, the plate was added with 0, 20, and 40 µM FeSO4. For assay on the tolerance to Cd in Δycf1, the plate was added with 0, 40, and 60 µM CdCl2. The yeast cells were incubated at 30°C for 2 d prior to photographing.
To assess yeast growth in liquid medium, the transformed yeast strain Δsmf1 was precultured in SC–U liquid medium containing 2% (w) Gal and 1% raffinose to the log phase. The precultured yeast cells were diluted to an OD600 of 0.05 and were then grown in SC-U liquid medium added with or without 20 mM EGTA. The OD600 value of yeast cells was measured from 0-48h. To determine Mn uptake in yeast, the yeast cells with an initial OD600 of 0.2 were incubated in SC–U liquid medium supplemented with 20 and 40 μМ MnSO4 for 24 h. To analyze kinetics of Mn uptake, the yeast cells with an initial OD600 of 0.2 were incubated in SC–U liquid medium supplemented with 0–10 μМ MnSO4 for 6 h. After treatments, the yeast cells were harvested and washed with 5 mM CaCl2 solution, followed by washing with deionized water twice. The collected cells were used for Mn determination. The Km and Vmax values of Mn uptake in yeast cells expressing SgNramp1 were estimated by Lineweaver-Burke plots of Mn concentrations at various Mn treatments after subtracting the Mn concentration in yeast cells transformed with pYES2 empty vector.
One-way ANOVA and Student’s t-test were analyzed by using SPSS13.0 (SPSS Institute, Chicago, United States).
In this study, five Nramp genes were cloned in stylo, which were named as SgNramp1 to SgNramp5 (Supplementary Table S2). The full-length sequences of SgNramps varied from 1,344 to 1,779 bp, and they encoded proteins differing from 447 to 591 amino acid residues in length with molecular weight ranging from 48.3 to 64.5 kDa (Supplementary Table S2). All of the SgNramp members possessed the conserved Nramp domain (PF01566). Among them, four SgNramp (SgNramp1, 2, 3, and 5) proteins included 12 conserved TMDs, whereas SgNramp4 harbored 10 TMDs (Figure 1 and Supplementary Table S2). Furthermore, the SgNramp proteins carried the unique amino acid residues GQSST(/A)ITGTYAGQF(/Y)I(/V)MQ(/G)GFLN(/D) of plant Nramp proteins (Figure 1). The amino acid sequences of five SgNramps shared 31.8–81.2% identities with each other (Supplementary Figure S1). In addition, SgNramp1 to SgNramp5 shared 32.1–77.0% homology identities with AtNramp1 in Arabidopsis, 35.9–76.0% identities with MtNramp1 in M. truncatula and 34.1–81.8% homology identities with GmDMT1 in soybean (Supplementary Figure S1).
Figure 1 Multiple alignment of SgNramps from S. guianensis and AtNramp1 from Arabidopsis. The transmembrane domains (TMDs) were boxed with red color. The unique amino acid residues of the Nramp proteins were labeled with blue line above the sequence. The following Nramp proteins were used: SgNramp1 (ON338040), SgNramp2 (ON338041), SgNramp3 (ON338042), SgNramp4 (ON338043), SgNramp5 (ON338044), and AtNramp1 (At1g80830). * in the figure indicates the same amino acid residue included in all Nramp proteins.
Phylogenetic analysis was performed using Nramp members from stylo, Arabidopsis, rice, and M. truncatula. Results showed that Nramp proteins were divided into two major groups (Groups I and II) (Figure 2). Group I included three SgNramps (SgNramp1, 4 and 5), two Nramp proteins from Arabidopsis, five Nramp members from rice, and three Nramps from M. truncatula. SgNramp2 and SgNramp3 clustered with four Nramp members from Arabidopsis, two Nramp members from rice, and four Nramps from M. truncatula were classified into Group II (Figure 2). Interestingly, Nramp members in Group I contained five to six conserved motifs, whereas all Nramp members in Group II contained seven conserved motifs. Among them, SgNramp1 and SgNramp5 included six conserved motifs, whereas SgNramp4 only harbored five conserved motifs. SgNramp2 and SgNramp3 contained seven conserved motifs (Figure 2 and Supplementary Table S3). The conserved motifs included 32–50 amino acids, and putative Nramp domain was predicted in the conserved motifs (Supplementary Table S3).
Figure 2 Phylogenetic analysis and conserved motifs of Nramp proteins in stylo and other plants. The first two letters of each Nramp member indicate the plant species. At, Arabidopsis thaliana; Os, Oryza sativa; Mt, Medicago truncatula. The red arrows indicate SgNramps from stylo. The sequences of Nramp proteins were obtained from the previous studies (Tejada-Jiménez et al., 2015; Qin et al., 2017; Mani and Sankaranarayanan, 2018). The phylogenetic tree was constructed by MEGA4. Conserved motifs of Nramp proteins were analyzed by MEME. Each motif is presented by a colored box. The sequences of different motifs of Nramp proteins were summarized in Supplementary Table S3.
The expression patterns of SgNramps in tissues of root, stem, leaf, flower, and seed of stylo were measured via qRT-PCR. Results showed that SgNramps exhibited different expressions in various tissues of stylo (Figure 3). Among them, SgNramp1 mainly expressed in root and seed and exhibited the lowest expression in leaf. SgNramp2 displayed preferential expressions in flower. The expressions of SgNramp3 in stem and flower were higher than those of other tissues, whereas SgNramp4 showed the higher level of expression in flower and seed compared with the other tissues. SgNramp5 exhibited a higher expression in stem than in the other tissues (Figure 3).
Figure 3 Expression analysis of SgNramps in various tissues. (A) Expressions of SgNramps in root, stem, leaf, flower, and seed. The expression of each gene in the roots was set to one, and then the expression level of each gene in other tissues was normalized to its expression in the roots. (B) Expressions of SgNramps in stylo root. The expression of SgNramp1 was set to one, and then the expression level of the other SgNramps was normalized to SgNramp1. Data are means of three biological replicates with standard error (SE). Different letters represent significant differences among various tissue (A) or SgNramp genes (B) at P < 0.05.
To investigate the responses of SgNramps to trace metal stresses, including deficient and excess Mn, Fe, Zn, and Cu treatments, the transcription profiles of SgNramps were measured in leaf and root. Results showed that the transcript of each SgNramp gene was regulated by at least one metal stress in leaf and/or root (Figure 4). For example, SgNramp1 was enhanced, and SgNramp2, SgNramp3 and SgNramp5 were suppressed by Mn limitation in leaf, whereas SgNramp2 was enhanced but SgNramp4 and SgNramp5 were suppressed under Mn deficient condition in root (Figure 4A). In addition, the number of SgNramps regulated by Mn stress with more than twofold (the absolute value of log2 ≥ 1) was higher than those in Fe, Zn, and Cu stresses. For example, a total of 6, 3, and 1 SgNramp genes were regulated by deficiencies of Mn, Fe, and Cu in leaf and/or root, respectively, whereas the transcripts of 6, 4, and 3 SgNramp genes were regulated by excess Mn, Fe, and Cu treatments in leaf and/or root, respectively (Figures 4A, B). Interestingly, the transcripts of SgNramp5 were enhanced by more than twofold under limited or excess Fe stress in both leaf and root (Figure 4).
Figure 4 Expression analyses of SgNramps in leaf and root of stylo under metal stresses. (A) Expressions of SgNramps in leaf (L) and root (R) of stylo grown in nutrient solution without MnSO4 (–Mn), Fe-Na-EDTA (–Fe), ZnSO4 (–Zn), or CuSO4 (–Cu) applications for 7 days. (B) Expressions of SgNramps in leaf (L) and root (R) of stylo grown in nutrient solution supplied with 400 μM MnSO4 (+Mn), 800 μM Fe-Na-EDTA (+Fe), 20 μM ZnSO4 (+Zn), or 10 μM CuSO4 (+Cu) for 7 days. Stylo seedlings grown in full-strength Hoagland solution were set as the control treatment. The data include three biological replicates. The values represented the fold change of each SgNramp expression between metal stress and the control (CK), which were calculated as log2(metal stress/CK). The positive and negative values represent up- and down-regulation, respectively. Asterisks below the values indicate significant differences between metal stress and CK. *P < 0.05; **0.001 < P < 0.01; ***P < 0.001.
Of the five SgNramps, SgNramp1 displayed high similarity with AtNramp1 that is responsible for Mn uptake in roots of Arabidopsis (Supplementary Figure S1). Furthermore, SgNramp1 displayed the highest expression in stylo roots compared with the other four SgNramps (Figure 3B). Thus, we further assessed whether SgNramp1 is responsible for genotypic difference in Mn uptake in various stylo cultivars. Results showed that a close correlation (R2 = 0.64, P < 0.01) was found between SgNramp1 expression and root Mn concentration in nine stylo cultivars under 0.1 μM MnSO4 condition (low Mn treatment) (Figure 5A).
Figure 5 Genotypic variation analysis of SgNramp1. (A) Analysis of correlation between SgNramp1 expression and root Mn concentration in various stylo cultivars under 0.1 μM MnSO4 treatments. The red and blue arrows indicate Reyan NO.18 and Reyan NO.22 cultivars, respectively. (B) Mn concentration in roots of Reyan NO.18 and Reyan NO.22 under 0.1 or 5 μM MnSO4 treatments. (C) Expression of SgNramp1 in roots of Reyan NO.18 and Reyan NO.22 under 0.1 or 5 μM MnSO4 treatments. Fourteen-day-old stylo seedlings were subjected to 0.1 or 5 μM MnSO4 treatments for 7 days that were considered as low Mn and normal Mn treatments, respectively. Stylo roots were used for SgNramp1 expression analysis and Mn determination. Data are means of three biological replicates with SE. Different letters represent significant differences at P < 0.05.
Among the tested nine stylo cultivars, two cultivars, Reyan NO.18 and Reyan NO.22, exhibited significant genotypic variation in SgNramp1 expression and root Mn concentration. Furthermore, Mn concentration in root of Reyan NO.18 was significantly higher than that in Reyan NO.22 under both 0.1 and 5 μM MnSO4 treatments, which were considered as low Mn or normal Mn treatments, respectively (Figure 5B). In addition, compared with normal Mn treatment, the expressions of SgNramp1 were significantly increased by low Mn in Reyan NO.18 but not in Reyan NO.22 (Figure 5C). Therefore, SgNramp1 is likely to be involved in Mn uptake in stylo and was then selected for further analysis.
SgNramp1 was cloned and fused to the N-terminus of GFP (35S:SgNramp1-GFP). Then, subcellular localization analysis was performed in tobacco epidermis cells through transiently expressed 35S:SgNramp1-GFP and empty vector. Results showed that the fluorescence of GFP alone in epidermis cells was found in the cytoplasm, nucleus, and PM, whereas GPF fluorescence of SgNramp1 was found to be co-localized with that of the PM marker in the epidermis cells (Figure 6), suggesting that SgNramp1 is a PM–localized protein.
Figure 6 Subcellular localization of SgNramp1. The 35:SgNramp1-GFP construct and 35:GFP empty vector were transiently expressed in tobacco epidermis cells. SgNramp1 was co-expressed with the plasma membrane (PM) marker, which was fused with red fluorescence protein (mKATE). Signals of GFP fusion protein, PM marker, chlorophyll autofluorescence (Auto), bright-field images (DIC), and the merged images (Merge) were shown from left to right. Scale bar is 20 μm.
Subsequently, the coding sequence of SgNramp1 was cloned into the pYES2 vector and transformed into various yeast strains. As shown in Figure 7, no differences in the growth were observed in the Mn uptake-defective yeast mutants Δsmf1 expressing either the pYES2 empty vector or SgNramp1 gene in the control medium without EGTA supplement. Under Mn limited condition by added with 20 or 25 mM EGTA, SgNramp1 expression rescued the growth of the yeast strain Δsmf1 compared with the empty vector control, although the growth of both strains was inhibited by low Mn stress (Figure 7). No significant differences in growth performance were observed between the Fe uptake-defective yeast mutants Δfet3fet4 transformed with either the pYES2 empty vector or SgNramp1 in the medium with 0, 20, or 40 μМ Fe supplements (Supplementary Figure S2A). In addition, the Cd-sensitive yeast strains Δycf1 expressing either the pYES2 empty vector or SgNramp1 exhibited the same sensitivity to Cd stress in the medium added with 20 or 60 μМ Cd (Supplementary Figure S2B). These results suggest that SgNramp1 can transport Mn but not Fe and Cd.
Figure 7 Manganese transport analysis of SgNramp1 in yeast. The yeast mutant Δsmf1 expressing either the pYES2 empty vector or SgNramp1 was spotted on SC-U/Gal solid medium containing 0, 20, or 25 mM Mn-chelator EGTA. The photo shows the growth of yeast cells at 30°C for 2 days.
To further investigate the differences in yeast growth and Mn uptake, the yeast strains Δsmf1 expressing the pYES2 empty vector or SgNramp1 were grown in a liquid SC-U/Gal medium added with or without 20 mМ EGTA. The growth curve showed the similar growth status of the two strains under without EGTA condition (Figure 8A). In the presence of 20 mМ EGTA, the growth of yeast strain expressing SgNramp1 was better than the yeast strain transformed with the empty vector control. The OD600 values of yeast cells expressing SgNramp1 were 52.3 and 69.8% higher than that of the yeast strain transformed with the empty vector control at 24 h and 48 h of incubation, respectively (Figure 8A). Furthermore, Mn concentration in the yeast cells expressing SgNramp1 was 1.4- and 1.9-fold higher than those in the yeast cells transformed with the empty vector control supplemented with 20 and 40 μМ MnSO4, respectively (Figure 8B). In addition, kinetic analysis of Mn uptake in yeast showed that the Km value was 3.8 ± 0.40 μM and the Vmax value was 1.13 ± 0.09 μg g−1 min−1 (Figure 8C). Thus, SgNramp1 is involved in Mn uptake in yeast cells.
Figure 8 Growth and Mn uptake in yeast mutant Δsmf1 expressing SgNramp1. (A) The yeast mutant Δsmf1 expressing either the pYES2 empty vector or SgNramp1 was grown in SC-U/Gal liquid medium with or without 20 mM EGTA for 0h to 48h. (B) Mn concentrations in the yeast cells grown in SC-U/Gal liquid medium containing 0, 20, or 40 μМ MnSO4 for 24h. (C) The kinetics of Mn uptake in yeast cells. The kinetics parameters are shown next to the figure. Data are means of three biological replicates with SE. Asterisks indicate significant differences between the pYES2 empty vector and SgNramp1. **0.001 < P < 0.01; ***P < 0.001.
Numerous studies demonstrated that Nramp proteins participate in the uptake, translocation, and distribution of metal ions, regulating metal homeostasis in plants (Sasaki et al., 2012; Li et al., 2021c). Although Nramp homologues have been reported in plants, Nramp members and their functional roles in the important tropical legume, stylo, have not been elucidated so far. In this study, we identified five SgNramp genes in stylo. All of them possessed the conserved Nramp domain (PF01566) and carried the unique amino acid residues of Nramp proteins (Figure 1), which have been widely identified in AtNramps from Arabidopsis, OsNramps from rice, GmNramps from soybean, PvNramps from bean (Phaseolus vulgaris), and CsNramps from tea (Camellia sinensis) (Thomine et al., 2000; Yamaji et al., 2013; Qin et al., 2017; Ishida et al., 2018; Li et al., 2021b). Furthermore, Nramp proteins have been reported to include 10–12 TMDs. For example, there are 12 TMDs in AtNramp1/2, 10 TMDs in OsNramp5, 11 TMDs in HvNramp5, and 12 TMDs in FeNramp5 (Curie et al., 2000; Sasaki et al., 2012; Wu et al., 2016; Yokosho et al., 2021). Consistently, all of the SgNramp proteins contained 12 conserved TMDs, except SgNramp4, which harbored 10 TMDs (Figure 1 and Supplementary Table S2). These conserved structures are essential for the functions of Nramp proteins.
Phylogenetic analysis showed that SgNramp1 and SgNramp4, together with AtNramp1 and AtNramp6 from Arabidopsis, MtNramp3 from M. truncatula, and OsNramp3 from rice were grouped into the same cluster in Group I (Figure 2). AtNramp1 is a PM-localized transporter that participates in Mn uptake in Arabidopsis (Cailliatte et al., 2010). AtNramp6 is able to transport Fe and regulates lateral root growth in Arabidopsis under low Fe stress (Cailliatte et al., 2009; Li et al., 2019). Furthermore, AtNramp6 is suggested to cooperate with AtNramp1 in controlling Mn homeostasis in Arabidopsis (Li et al., 2022). OsNramp3 is characterized to be an important node-based transporter essential for preferential Mn distribution, mediating Mn remobilization between young leaves and old tissues of rice in response to variable Mn levels (Yamaji et al., 2013; Yang et al., 2013). As the closest homologue of SgNramp5 in Group I, MtNramp1 is implicated in Fe transport in the yeast cells, and it participates in apoplastic Fe uptake by rhizobia-infected cells in nodules of M. truncatula (Tejada-Jiménez et al., 2015). In Group II, SgNramp2 exhibited high similarity to AtNramp2, which is localized to the trans-Golgi network and is proposed to involve in Mn transport in Golgi, regulating root growth of Arabidopsis under low Mn stress (Gao et al., 2018). SgNramp3 was clustered with AtNramp3/4 and MtNramp5/7 in the same subgroup (Figure 2). In addition to participate in vacuolar Fe mobilization during seed germination, AtNramp3 and AtNramp4 are demonstrated to be responsible for intracellular Mn homeostasis in Arabidopsis (Thomine et al., 2003; Lanquar et al., 2005; Lanquar et al., 2010). Therefore, stylo SgNramps might possess the potential role in regulating metal homeostasis in stylo.
Nramp proteins have been characterized to function in the regulation of metal uptake and homeostasis, and thus they may exhibit preferential expressions in various plant tissues. For example, a set of Nramp homologues are observed to be mainly expressed in roots, such as AtNramp1 from Arabidopsis (Cailliatte et al., 2010), OsNramp4/5 from rice (Xia et al., 2010; Sasaki et al., 2012), MtNramp1 from M. truncatula (Tejada-Jiménez et al., 2015), HvNramp5 from barley (Wu et al., 2016), TtNramp6 from wheat (Triticum turgidum) (Wang et al., 2019a), AhNramp1 from peanut (Arachis hypogaea) (Wang et al., 2019b), FeNramp5 from buckwheat (Yokosho et al., 2021), and NtNramp1 from tobacco (Liu et al., 2022). The root-specific expression of Nramps is beneficial for metal uptake. Similarly, variations in the abundances of SgNramps were observed in different tissues of stylo, such as SgNramp1 mainly expressed in root and seed (Figure 3), suggesting the key role of SgNramp members in regulating metal homeostasis in specific tissues.
In addition, SgNramp genes exhibited diverse responses to Mn, Fe, Zn, and Cu stresses as well as heavy metal toxicity in stylo (Figure 4 and Supplementary Figure S3). In Arabidopsis, the transcripts of AtNramp1 in roots are enhanced by both low Fe and Mn levels, whereas both AtNramp3 and AtNramp4 abundances are increased in roots and shoots by Fe starvation but not Mn deficiency (Curie et al., 2000; Thomine et al., 2000; Cailliatte et al., 2010). In rice, the expression of OsNramp5 gene is up-regulated in roots by Zn and Fe deficiencies but not low Mn and Cu conditions (Xia et al., 2010; Sasaki et al., 2012). On the other hand, although some of the Nramp homologues are not response to metal stresses, they are able to transport metal ions. For example, AtNramp2 and AtNramp6 genes are not regulated by deficiencies of Mn, Fe, and Zn, but AtNramp2 is found to be involved in remobilization of Mn in Golgi for Arabidopsis root growth (Gao et al., 2018), and AtNramp6 is shown to participate in regulation of Fe and Mn homeostasis (Li et al., 2019; Li et al., 2022). Although OsNramp3 is unaffected by deficiencies of Zn, Fe, Mn, and Cu at transcriptional level, its encoding protein is rapidly degraded after high Mn exposure, regulating Mn distribution in young leaves and old tissues (Yamaji et al., 2013). Thus, changes in the abundances of SgNramp genes might potentially contribute to regulate metal homeostasis in various tissues of stylo for dealing with the fluctuated metal levels.
In this study, we found that the transcript levels of SgNramp1 are responsible for the genotypic differences in root Mn levels in stylo, as reflected by a close correlation between SgNramp1 expression and Mn concentration in various stylo cultivars (Figure 5). Furthermore, a higher level of Mn was observed in the stylo cultivar Reyan NO.18 compared with Reyan NO.22, which was correlated with higher SgNramp1 expression in Reyan NO.18 under low Mn condition (Figure 5). Similarly, differences in Mn efficiency have been observed among barley genotypes, which has been attributed to differences in Mn uptake capability; by analyzing two barley genotypes contrasting in Mn acquisition efficiency and gene expression, the role in Mn uptake has been suggested for HvIRT1, which exhibits higher expression in a Mn-efficient barley genotype than a Mn-inefficient genotype and has the ability to restore Mn uptake of Δsmf1 mutant (Pedas et al., 2005, Pedas et al., 2008).
The metal transport activity of SgNramp1, which was localized to the PM, was further analyzed in yeast cells. Heterologous expression of SgNramp1 in yeast mutants showed that SgNramp1 can restore the growth of the yeast strain Δsmf1 through increasing Mn uptake (Figures 7 and 8). It has been demonstrated that a homologue of SgNramp1, AtNramp1, encoding a PM-localized transporter, can restore the growth of yeast mutant Δsmf1 under Mn limitation (Curie et al., 2000; Thomine et al., 2000). Furthermore, loss function of AtNramp1 disrupts the growth and Mn concentration in Arabidopsis under low Mn condition, demonstrating that AtNramp1 is the transporter responsible for Mn uptake in Arabidopsis (Cailliatte et al., 2010). In addition, broad transport substrates of Nramp homologues were observed in other plants, such M. truncatula, rice, barley, and buckwheat (Ishimaru et al., 2012; Tejada-Jiménez et al., 2015; Wu et al., 2016; Yokosho et al., 2021). For example, OsNramp5 in rice transports Mn, Cd, and Fe (Ishimaru et al., 2012), whereas HvNramp5 in barley and FeNramp5 in buckwheat only transport Mn and Cd but not Fe (Wu et al., 2016; Yokosho et al., 2021). Thus, it is reasonable to assume that SgNramp1 might contribute to Mn acquisition in stylo. However, the function of SgNramp1 may be differed in various stylo cultivars. Further research work is needed, such as generation of transgenic stylo plants overexpressing or suppressing SgNramp1, to verify its exact function conducted in both nutrient solutions and soil conditions with different Mn status and availability.
In summary, we identified five SgNramp genes in stylo. Changes observed in the abundances of SgNramps suggest the involvement of these genes in regulating metal homeostasis in tissues of different plant parts of stylo during metal stress conditions. Moreover, SgNramp1 is a PM-localized transporter and it is responsible for Mn uptake. Increases of SgNramp1 expression may contribute to enhance the acquisition of Mn in stylo. Therefore, SgNramp1 might be a good candidate for breeding future stylo cultivars with efficient Mn uptake through gene-editing technology.
The datasets presented in this study can be found in online repositories. The names of the repository/repositories and accession number(s) can be found in the article/Supplementary Material.
ZC designed the research. XZ, RH, LW and GW performed the experiments and analyzed the data. YM and GL prepared the plant material for this work. XZ, RH and ZC wrote the manuscript. IR, GL and ZC discussed and revised the manuscript. All authors contributed to the article and approved the submitted version.
This work was funded by the National Natural Science Foundation of China (32271756, 31861143013), the National Natural Science Foundation of Hainan (321RC645), and the China Agriculture Research System of MOF and MARA (CARS-34).
The authors appreciate Yufen Xing for technical help.
The authors declare that the research was conducted in the absence of any commercial or financial relationships that could be construed as a potential conflict of interest.
All claims expressed in this article are solely those of the authors and do not necessarily represent those of their affiliated organizations, or those of the publisher, the editors and the reviewers. Any product that may be evaluated in this article, or claim that may be made by its manufacturer, is not guaranteed or endorsed by the publisher.
The Supplementary Material for this article can be found online at: https://www.frontiersin.org/articles/10.3389/fpls.2022.1027551/full#supplementary-material
Supplementary Figure 1 | Homology identity analysis of SgNramp proteins. (A) Homology identity among SgNramp proteins. (B) Homology identity of SgNramps with AtNramp1 (accession no. At1g80830) from Arabidopsis. (C) Homology identity of SgNramps with MtNramp1 (accession no. Medtr3g088460) from M. truncatula. (D) Homology identity of SgNramps with GmDMT1 (accession no. AY169405) from soybean. The homology identity (%) was analyzed by Clustal.
Supplementary Figure 2 | Transport activity of SgNramp1 in yeast mutants Δfet3fet4 and Δycf1. (A) The yeast mutant Δfet3fet4 defective in Fe uptake expressing either the pYES2 empty vector or SgNramp1 was grown in SC-U/Gal medium containing 0, 20, or 40 μМ Fe. (B) The yeast mutant Δycf1 sensitive in Cd stress expressing either the pYES2 empty vector or SgNramp1 was grown in SC-U/Gal medium containing 0, 20, or 60 μМ Cd. The photo shows the growth of yeast cells at 30°C for 2 days.
Supplementary Figure 3 | Expression of SgNramps in roots of stylo under heavy metal treatments. Fourteen-day-old stylo seedlings were separately subjected to 0 (CK), 100 μM AlCl3, 40 μM CdCl2, or 20 μM LaCl3 treatments for 2 days. Roots were harvested for gene expression analysis. Data are means of three biological replicates with standard error (SE). Asterisks indicate significant differences between control and treatments. *0.01 < P < 0.05; **0.001 < P < 0.01; ***P < 0.001.
Cailliatte, R., Lapeyre, B., Briat, J. F., Mari, S., Curie, C. (2009). The NRAMP6 metal transporter contributes to cadmium toxicity. Biochem. J. 422, 217–228. doi: 10.1042/BJ20090655
Cailliatte, R., Schikora, A., Briat, J. F., Mari, S., Curie, C. (2010). High-affinity manganese uptake by the metal transporter NRAMP1 is essential for Arabidopsis growth in low manganese conditions. Plant Cell 22, 904–917. doi: 10.1105/tpc.109.073023
Chang, J. D., Huang, S., Yamaji, N., Zhang, W. W., Ma, J. F., Zhao, F. J. (2020). OsNRAMP1 transporter contributes to cadmium and manganese uptake in rice. Plant Cell Environ. 43, 2476–2491. doi: 10.1111/pce.13843
Chen, Z. J., Li, J. F. (2021). “Mechanism of manganese uptake and homeostasis in plant cell,” in Cation transporters in plants, ed s. upadhyay, vol. 13. (Elsevier, Netherlands: Academic Press), 227–246. doi: 10.1016/B978-0-323-85790-1.00025-7
Chen, Z. J., Song, J. L., Li, X. Y., Arango, J., Cardoso, J. A., Rao, I., et al. (2021). Physiological responses and transcriptomic changes reveal the mechanisms underlying adaptation of Stylosanthes guianensis to phosphorus deficiency. BMC Plant Biol. 21, 466. doi: 10.1186/s12870-021-03249-2
Chen, Z. J., Sun, L. L., Liu, P. D., Liu, G. D., Tian, J., Liao, H. (2015). Malate synthesis and secretion mediated by a manganese-enhanced malate dehydrogenase confers superior manganese tolerance in Stylosanthes guianensis. Plant Physiol. 167, 176–188. doi: 10.1104/pp.114.251017
Curie, C., Alonso, J. M., Le Jean, M., Ecker, J. R., Briat, J. F. (2000). Involvement of NRAMP1 from Arabidopsis thaliana in iron transport. Biochem. J. 347, 749–755. doi: 10.1042/0264-6021:3470749
Fryzova, R., Pohanka, M., Martinkova, P., Cihlarova, H., Brtnicky, M., Hladky, J., et al. (2018). Oxidative stress and heavy metals in plants. Rev. Environ. Contam. Toxicol. 245, 129–156. doi: 10.1007/398_2017_7
Gao, H. L., Xie, W. X., Yang, C. H., Xu, J. Y., Li, J. J., Wang, H., et al. (2018). NRAMP2, a trans-golgi network-localized manganese transporter, is required for Arabidopsis root growth under manganese deficiency. New Phytol. 217, 179–193. doi: 10.1111/nph.14783
Guo, P. F., Liu, P. D., Lei, J., Chen, C. H., Qiu, H., Liu, G. D., et al. (2019). Improvement of plant regeneration and Agrobacterium-mediated genetic transformation of Stylosanthes guianensis. Trop. Grassl-Forrajes. 7, 480–492. doi: 10.17138/tgft(7)480-492
Huang, S., Wang, P., Yamaji, N., Ma, J. F. (2020). Plant nutrition for human nutrition: hints from rice research and future perspectives. Mol. Plant 13, 825–835. doi: 10.1016/j.molp.2020.05.007
Ishida, J. K., Caldas, D. G. G., Oliveira, L. R., Frederici, G. C., Leite, L. M. P., Mui, T. S. (2018). Genome-wide characterization of the NRAMP gene family in Phaseolus vulgaris provides insights into functional implications during common bean development. Genet. Mol. Biol. 41, 820–833. doi: 10.1590/1678-4685-GMB-2017-0272
Ishimaru, Y., Takahashi, R., Bashir, K., Shimo, H., Senoura, T., Sugimoto, K., et al. (2012). Characterizing the role of rice NRAMP5 in manganese, iron and cadmium transport. Sci. Rep. 2, 286. doi: 10.1038/srep00286
Jia, Y. D., Li, X. Y., Liu, Q., Hu, X., Li, J. F., Dong, R. S., et al. (2020). Physiological and transcriptomic analyses reveal the roles of secondary metabolism in the adaptive responses of Stylosanthes to manganese toxicity. BMC Genomics 21, 861. doi: 10.1186/s12864-020-07279-2
Jiang, C. D., Liu, L. S., Li, X. F., Han, R. R., Wei, Y. M., Yu, Y. X. (2018). Insights into aluminum-tolerance pathways in Stylosanthes as revealed by RNA-seq analysis. Sci. Rep. 8, 6072. doi: 10.1038/s41598-018-24536-3
Kaiser, B. N., Moreau, S., Castelli, J., Thomson, R., Lambert, A., Bogliolo, S., et al. (2003). The soybean NRAMP homologue, GmDMT1, is a symbiotic divalent metal transporter capable of ferrous iron transport. Plant J. 35, 295–304. doi: 10.1046/j.1365-313x.2003.01802.x
Kaur, R., Das, S., Bansal, S., Singh, G., Sardar, S., Dhar, H., et al. (2021). Heavy metal stress in rice: uptake, transport, signaling, and tolerance mechanisms. Physiol. Plant 173, 430–448. doi: 10.1111/ppl.13491
Kurusu, T., Nishikawa, D., Yamazaki, Y., Gotoh, M., Nakano, M., Hamada, H., et al. (2012). Plasma membrane protein OsMCA1 is involved in regulation of hypo-osmotic shock-induced Ca2+ influx and modulates generation of reactive oxygen species in cultured rice cells. BMC Plant Biol. 12, 11. doi: 10.1186/1471-2229-12-11
Lanquar, V., Lelievre, F., Bolte, S., Hames, C., Alcon, C., Neumann, D., et al. (2005). Mobilization of vacuolar iron by AtNRAMP3 and AtNRAMP4 is essential for seed germination on low iron. EMBO J. 24, 4041–4051. doi: 10.1038/sj.emboj.7600864
Lanquar, V., Ramos, M. S., Lelievre, F., Barbier-Brygoo, H., Krieger-Liszkay, A., Kramer, U., et al. (2010). Export of vacuolar manganese by AtNRAMP3 and AtNRAMP4 is required for optimal photosynthesis and growth under manganese deficiency. Plant Physiol. 152, 1986–1999. doi: 10.1104/pp.109.150946
Li, J. F., Dong, R. S., Jia, Y. D., Huang, J., Zou, X. Y., An, N., et al. (2021a). Characterization of metal tolerance proteins and functional analysis of GmMTP8.1 involved in manganese tolerance in soybean. Front. Plant Sci. 12. doi: 10.3389/fpls.2021.683813
Li, J. Q., Duan, Y., Han, Z. L., Shang, X. W., Zhang, K. X., Zou, Z. W., et al. (2021b). Genome-wide identification and expression analysis of the NRAMP family genes in tea plant (Camellia sinensis). Plants 10, 1055. doi: 10.3390/plants10061055
Li, Y., Li, J. J., Yu, Y. H., Dai, X., Gong, C. Y., Gu, D. F., et al. (2021c). The tonoplast-localized transporter OsNRAMP2 is involved in iron homeostasis and affects seed germination in rice. J. Exp. Bot. 72, 4839–4852. doi: 10.1093/jxb/erab159
Liu, W. H., Huo, C. H., He, L. S., Ji, X., Yu, T., Yuan, J. W., et al. (2022). The NtNRAMP1 transporter is involved in cadmium and iron transport in tobacco (Nicotiana tabacum). Plant Physiol. Biochem. 173, 59–67. doi: 10.1016/j.plaphy.2022.01.024
Li, J. Y., Wang, Y. R., Zheng, L., Li, Y., Zhou, X. L., Li, J. J., et al. (2019). The intracellular transporter AtNRAMP6 is involved in fe homeostasis in Arabidopsis. Front. Plant Sci. 10. doi: 10.3389/fpls.2019.01124
Li, L., Zhu, Z. Z., Liao, Y. H., Yang, C. H., Fan, N., Zhang, J., et al. (2022). NRAMP6 and NRAMP1 cooperatively regulate root growth and manganese translocation under manganese deficiency in arabidopsis. Plant J 110, 1564–1577. doi: 10.1111/tpj.15754
Mani, A., Sankaranarayanan, K. (2018). In silico analysis of natural resistance-associated macrophage protein (NRAMP) family of transporters in rice. Protein J. 37, 237–247.
Marques, A., Moraes, L., Aparecida Dos Santos, M., Costa, I., Costa, L., Nunes, T., et al. (2018). Origin and parental genome characterization of the allotetraploid Stylosanthes scabra Vogel (Papilionoideae, leguminosae), an important legume pasture crop. Ann. Bot. 122, 1143–1159. doi: 10.1093/aob/mcy113
Marschner, H. (2012). Marschner's mineral nutrition of higher plants. 3rd ed. Ed. Marschner, H. (Netherlands, Academic Press: Elsevier).
Pedas, P., Hebbern, C. A., Schjoerring, J. K., Holm, P. E., Husted, S. (2005). Differential capacity for high-affinity manganese uptake contributes to differences between barley genotypes in tolerance to low manganese availability. Plant Physiol. 139, 1411–1420. doi: 10.1104/pp.105.067561
Pedas, P., Ytting, C. K., Fuglsang, A. T., Jahn, T. P., Schjoerring, J. K., Husted, S. (2008). Manganese efficiency in barley: Identification and characterization of the metal ion transporter HvIRT1. Plant Physiol. 148, 455–466. doi: 10.1104/pp.108.118851
Peris-Peris, C., Serra-Cardona, A., Sánchez-Sanuy, F., Campo, S., Ariño, J., San Segundo, B. (2017). Two NRAMP6 isoforms function as iron and manganese transporters and contribute to disease resistance in rice. Mol. Plant Microbe In. 30, 385–398. doi: 10.1094/MPMI-01-17-0005-R
Pottier, M., Le Thi, V. A., Primard-Brisset, C., Marion, J., Bianchi, M., Victor, C., et al. (2022). Duplication of NRAMP3 gene in poplars generated two homologous transporters with distinct functions. Mol. Biol. Evol. 39, msac129. doi: 10.1093/molbev/msac129
Qin, L., Han, P., Chen, L., Walk, T. C., Li, Y., Hu, X., et al. (2017). Genome-wide identification and expression analysis of NRAMP family genes in soybean (Glycine max l.). Front. Plant Sci. 8, 1436 doi: 10.3389/fpls.2017.01436
Riyazuddin, R., Nisha, N., Ejaz, B., Khan, M. I. R., Kumar, M., Ramteke, P. W., et al. (2021). A comprehensive review on the heavy metal toxicity and sequestration in plants. Biomolecules 12, 43. doi: 10.3390/biom12010043
Sasaki, A., Yamaji, N., Yokosho, K., Ma, J. F. (2012). Nramp5 is a major transporter responsible for manganese and cadmium uptake in rice. Plant Cell 24, 2155–2167. doi: 10.1105/tpc.112.096925
Song, J. L., Zou, X. Y., Liu, P. D., Cardoso, J. A., Schultze-Kraftc, R., Liu, G. D., et al. (2022). Differential expressions and enzymatic properties of malate dehydrogenases in the response of Stylosanthes guianensis to nutrient and metal stresses. Plant Physiol. Biochem. 170, 325–337. doi: 10.1016/j.plaphy.2021.12.012
Sun, L. L., Liang, C. Y., Chen, Z. J., Liu, P. D., Tian, J., Liu, G. D., et al. (2014). Superior Al tolerance of Stylosanthes is mainly achieved by malate synthesis through an Al-enhanced malic enzyme, SgME1. New Phytol. 202, 209–219. doi: 10.1111/nph.12629
Tang, Y. Q., Wu, Z. Y., Liu, G. D., Yi, K. X. (2009). Research advances in germplasm resources of Stylosanthes. Chin. Bull. Bot. 44, 752–762. doi: 10.3969/j.issn.1674-3466.2009.06.014
Tejada-Jiménez, M., Castro-Rodríguez, R., Kryvoruchko, I., Lucas, M. M., Udvardi, M., Imperial, J., et al. (2015). Medicago truncatula natural resistance-associated macrophage protein1 is required for iron uptake by rhizobia-infected nodule cells. Plant Physiol. 168, 258–272. doi: 10.1104/pp.114.254672
Thomine, S., Lelievre, F., Debarbieux, E., Schroeder, J. I., Barbier-Brygoo, H. (2003). AtNRAMP3, a multispecific vacuolar metal transporter involved in plant responses to iron deficiency. Plant J. 34, 685–695. doi: 10.1046/j.1365-313x.2003.01760.x
Thomine, S., Wang, R., Ward, J. M., Crawford, N. M., Schroeder, J. I. (2000). Cadmium and iron transport by members of a plant metal transporter family in Arabidopsis with homology to Nramp genes. Proc. Natl. Acad. Sci. U.S.A. 97, 4991–4996. doi: 10.1073/pnas.97.9.4991
Wang, C., Chen, X., Yao, Q., Long, D., Fan, X., Kang, H. Y., et al. (2019a). Overexpression of TtNRAMP6 enhances the accumulation of cd in Arabidopsis. Gene 696, 225–232. doi: 10.1016/j.gene.2019.02.008
Wang, N. Q., Qiu, W., Dai, J., Guo, X. T., Lu, Q. F., Wang, T. Q., et al. (2019b). AhNRAMP1 enhances manganese and zinc uptake in plants. Front. Plant Sci. 10. doi: 10.3389/fpls.2019.00415
Wang, P. T., Yamaji, N., Inoue, K., Mochida, K., Ma, J. F. (2020). Plastic transport systems of rice for mineral elements in response to diverse soil environmental changes. new. Phytol. 226, 156–169. doi: 10.1111/nph.16335
Wu, D. Z., Yamaji, N., Yamane, M., Kashino-Fujii, M., Sato, K., Ma, J. F. (2016). The HvNramp5 transporter mediates uptake of cadmium and manganese, but not iron. plant. Physiol. 172, 1899–1910. doi: 10.1104/pp.16.01189
Xia, J. X., Yamaji, N., Kasai, T., Ma, J. F. (2010). Plasma membrane-localized transporter for aluminum in rice. Proc. Natl. Acad. Sci. U.S.A. 107, 18381–18385. doi: 10.1073/pnas.1004949107
Yamaji, N., Sasaki, A., Xia, J. X., Yokosho, K., Ma, J. F. (2013). A node based switch for preferential distribution of manganese in rice. Nat. Commun. 4, 2442. doi: 10.1038/ncomms3442
Yang, M., Zhang, W., Dong, H. X., Zhang, Y. Y., Lv, K., Wang, D. J., et al. (2013). OsNRAMP3 is a vascular bundles-specific manganese transporter that is responsible for manganese distribution in rice. Plos One 8, e83990. doi: 10.1371/journal.pone.0083990
Yokosho, K., Yamaji, N., Ma, J. F. (2021). Buckwheat FeNramp5 mediates high manganese uptake in roots. Plant Cell Physiol. 62, 600–609. doi: 10.1093/pcp/pcaa153
Keywords: nramp, trace metal, metal homeostasis, gene expression, subcellular localization
Citation: Zou X, Huang R, Wang L, Wang G, Miao Y, Rao I, Liu G and Chen Z (2022) SgNramp1, a plasma membrane-localized transporter, involves in manganese uptake in Stylosanthes guianensis. Front. Plant Sci. 13:1027551. doi: 10.3389/fpls.2022.1027551
Received: 25 August 2022; Accepted: 20 September 2022;
Published: 06 October 2022.
Edited by:
Jiyu Zhang, Lanzhou University, ChinaReviewed by:
Wen-ting Peng, Fujian Agriculture and Forestry University, ChinaCopyright © 2022 Zou, Huang, Wang, Wang, Miao, Rao, Liu and Chen. This is an open-access article distributed under the terms of the Creative Commons Attribution License (CC BY). The use, distribution or reproduction in other forums is permitted, provided the original author(s) and the copyright owner(s) are credited and that the original publication in this journal is cited, in accordance with accepted academic practice. No use, distribution or reproduction is permitted which does not comply with these terms.
*Correspondence: Zhijian Chen, amNoZW5Ac2NhdS5lZHUuY24=
†These authors have contributed equally to this work and share first authorship
Disclaimer: All claims expressed in this article are solely those of the authors and do not necessarily represent those of their affiliated organizations, or those of the publisher, the editors and the reviewers. Any product that may be evaluated in this article or claim that may be made by its manufacturer is not guaranteed or endorsed by the publisher.
Research integrity at Frontiers
Learn more about the work of our research integrity team to safeguard the quality of each article we publish.