- Guangdong Laboratory for Lingnan Modern Agriculture, State Key Laboratory of Conservation and Utilization of Subtropical Agro-bioresources, College of Forestry and Landscape Architecture, South China Agricultural University, Guangzhou, China
Arbuscular mycorrhizal (AM) fungi are symbionts of most terrestrial plants and enhance their adaptability in metal-contaminated soils. In this study, mycorrhized and non-mycorrhized Eucalyptus grandis were grown under different Zn treatments. After 6 weeks of treatment, the growing status and ionome content of plants as well as the expression patterns of metal tolerance proteins and auxin biosynthesis–related genes were measured. In this study, mycorrhized E. grandis showed higher biomass and height at a high level of Zn compared with non-mycorrhized plants. In addition, AM plants accumulated P, Mg, and Mn in roots and P, Fe, and Cu in shoots, which indicate that AM fungi facilitate the uptake of ionome nutrients to promote plant growth. In addition, mycorrhiza upregulated the expression of EgMTP1 and EgMTP7, whose encoding proteins were predicted to be located at the vacuolar membrane. Meanwhile, Golgi membrane transporter EgMTP5 was also induced in AM shoot. Our results suggest that AM likely mitigates Zn toxicity through sequestrating excess Zn into vacuolar and Golgi. Furthermore, the expression of auxin biosynthesis–related genes was facilitated by AM, and this is probably another approach for Zn tolerance.
Introduction
Zinc (Zn) was an utmost important micronutrient for all living organisms, and it acted as catalytic and structural component in a large number of enzymes and regulatory proteins (Maret, 2009; Zhang et al., 2018; Kaur and Garg, 2021; Bae et al., 2022). Zn played an important role in regulating plant growth and development, which involves modulating a wide range of physiological processes: cell proliferation, respiration, auxin biosynthesis, and antioxidative defenses (Broadley et al., 2007; Zhang et al., 2018; Kaur and Garg, 2021; Bae et al., 2022). However, high concentration of Zn can be toxic. Excess Zn strongly decreased fresh weight and inhibited net photosynthetic rate, transpiration, and stomatal conductance in bean seedlings (Vassilev et al., 2011). In rice, a high level of Zn induced the lateral root formation through modulating the redistribution of auxin in root tips (Zhang et al., 2018) as well as inhibited the root-to-shoot translocation and distribution of P into new leaves by downregulating P transporter genes (Ding et al., 2021).
To maintain the intracellular Zn level within physiological limit, plants had developed a dynamic system involving Zn uptake, efflux, transport, and sequestration via particular transporters (Clemens et al., 2002; Stephens et al., 2011; Sinclair and Krämer, 2012). Zn transporters in plant included zinc/iron-regulated transporter-like proteins (ZIP), metal tolerance protein (MTP), heavy metal ATPases (HMA), natural resistance-associated macrophage protein (NRAMP), yellow stripe-like transporter family (YSL), ATP-binding cassette transporters (ABC), zinc-induced facilitator 1 proteins (ZIF1), and plant cadmium resistance proteins (PCR) (Sinclair and Krämer, 2012; Neeraja et al., 2018; Kaur and Garg, 2021). Zn toxicity resulted in suppressed expression of ZmZIP4, ZmZIP5, ZmZIP7, and ZmZIP8 in shoots and ZmZIP3 in maize roots (Li et al., 2013). In response to Zn stress, upregulation of MsZIP2 was a detoxification mechanism to store excess Zn in xylem parenchyma cells of Medicago sativa (Cardini et al., 2021). Enhanced expression of ZIF1 by excess Zn had also been verified in Arabidopsi thaliana (Haydon and Cobbett, 2007). Moreover, the transcript amount of HMA4 was elevated in the roots and shoots of M. sativa exposed to surplus Zn (Cardini et al., 2021).
MTP family as divalent cation transporters involved in metal ion efflux from the cytoplasm into subcellular compartments or to extracellular space (Sinclair and Krämer, 2012) and played a pivotal role in alleviating heavy metal toxicity. Previously, MTP family had been investigated at the genomic level in A. thaliana, Oryza sativa, Citrus sinensis, Populus trichocarpa, and Glycine max (Gustin et al., 2011; Fu et al., 2017; Gao et al., 2020; Haque et al., 2022). According to substrate specificity, the members of MTP family were phylogenetically classified into three subfamily: Zn-CDF (to transport Zn, Cd, Ni, and Co), Zn/Fe-CDF (to transfer Fe, Zn, Cd, Ni, and Co), and Mn-CDF (mostly, to target Mn) (Montanini et al., 2007). In A. thaliana, five MTPs had been reported to transport Zn: AtMTP1, AtMTP2, AtMTP3, AtMTP5, and AtMTP12 (Desbrosses-Fonrouge et al., 2005; Arrivault et al., 2006; Fujiwara et al., 2015; Sinclair et al., 2018).
Arbuscular mycorrhizal (AM) fungi were obligate biotrophic fungi, which formed mutualistic symbiosis with more than 70% of terrestrial vascular plants (Brundrett and Tedersoo, 2018; Tedersoo et al., 2020). In addition, AM fungi were eco-friendly and effective in alleviating heavy metal stress of plants (Ferrol and Tamayo, 2016; Nuria et al., 2016; Tedersoo et al., 2020; Riaz et al., 2021). For example, when plants were cultivated in soils containing toxic amount of Zn, AM fungi symbiosis induced higher phosphorus (P) concentration and lower Zn concentration in shoots than those grown in control conditions (Díaz et al., 1996). In addition, mycorrhization increased the total chlorophyll content of plant grown in metal-polluted soil but diminished the concentration of H2O2 and activity of glutathione reductase (GR), catalase (CAT), guaiacol peroxidase (POD) and ascorbate peroxidase (APX) (Fernández-Fuego et al., 2017). Glomalin-related soil protein, a kind of glycoprotein produced by AM fungi, was able to combine with metal ions to sequester them in soil, consequently, to mitigate metal uptake by plants (Yang et al., 2017). AM fungi increased the resistance of host to Zn stress by upregulating the expression of ZNT:4, COPT/Ctr:2, YSL:3, and CE:1 (Wang et al., 2022).
Eucalypts was well known for its fast growth and superior hardwood, and it had been widely planted as economical tree. Furthermore, eucalypts was popular for reclamation of degraded land in coal mines, because of its ability to uptake heavy metals from contaminated soil (Maiti and Rana, 2017). Previous studies showed that Eucalyptus grandis can form symbiosis relationships with AM fungi in both plantation and natural woodland community, and symbiosis protected it from potential damage of heavy metals (Adams et al., 2006; Chen et al., 2007; Canton et al., 2016). With the publication of E. grandis genome (Myburg et al., 2014), molecular mechanisms of E. grandis on metals stress need further exploration.
To get further insight into the role of E. grandis MTP on Zn homeostasis, we analyzed their expression patterns with/without AM fungi under different Zn treatments. We also assess the effects of Zn and AM fungi on ionome content and expression of auxin biosynthesis–related genes in E. grandis. This study will be helpful to the development of molecular markers for cultivar breeding of E. grandis with a high Zn tolerance.
Materials and methods
Biological materials and growth conditions
Rhizophagus irregularis DAOM197198 was used as the mycorrhizal fungus and was propagated on Zea mays. After inoculation for 3 months, roots were treated with drought for another 2 months. Spores of R. irregularis were collected by modified sucrose-gradient centrifugation (Charoenpakdee et al., 2010).
The roots containing spores were broken with a blender; then, the roots were filtered through 710-, 200-, and 45-µm pore sieves. After backwashing the contents of 45-µm sieve into a 50-ml centrifuge tube, an equal volume of 50% (w/v) sucrose solution was gently added into the centrifuge tube. Then, the tubes were centrifuged at 2,000 rpm for 1 min with bench centrifuge. The spores were collected on 45-µm pore sieve and washed thoroughly to remove traces of sugar solution. Last, spores were backwashed into tube.
One milliliter of the liquid containing spores collected by sucrose-gradient centrifugation was dropped onto Miracloth (Calbiochem). The number of spores on Miracloth was counted with microscope, and the total number of spores was calculated according to the volume of the mixing liquid. Thus, we calculated the volume of liquid containing about 500 spores.
Eucalyptus grandis was used as host plant in this study. Seeds were surface-sterilized with 1.5% sodium hypochlorite for 15 min and washed with sterile water for three times and then were cultured in a quarter-strength Murashige and Skoog medium (pH 5.9) with 3 g L−1 agar. After 4 weeks, the seedlings were transferred to pots that contained sterile sands (the sands were sterilized three times for 2 h at 121°C) and inoculated with or without R. irregularis (about 500 spores per plant). The seedlings were cultivated in a greenhouse at 24°C/18°C day/night temperature under 16-h daylight and 50%–60% humidity. Moreover, the seedlings were fertilized with modified Long-Ashon solution (30 μM KH2PO4; Hewitt, 1966) every 3 days. After 5 weeks, mycorrhiza formation was checked following the MYCOCALC program (http://www2.dijon.inra.fr/mychintec/Mycocalc-prg/download.html). Then, the seedlings were fertilized with the abovementioned modified Long-Ashon solution containing 5, 50, and 150 μM Zn once a week for 6 weeks, respectively (Fu et al., 2017; Gao et al., 2020; Wang et al., 2021). Before harvest, fresh weight and length of root and shoot were measured. Then, roots and shoots were separated and frozen immediately in liquid nitrogen and then were stored in −80°C refrigerator.
Elemental concentration analyses
To measure Zn, P, Mg, Fe, Cu, and Mn concentrations in E. grandis, the roots and shoots were dried in vacuum lyophilizer (Christ, Germany). After fine grounding, the samples were weighed and then were digested in 1 ml of 6 M nitric acids at 90°C for 2 h. The digested product was diluted with equal volume of sterile water and then was filtered. After a further dilution (1:10), the element concentrations were analyzed with inductively coupled plasma optical emission spectrometry (710-ES, VARIAN, USA) (Xie et al., 2021, 2022).
Phylogenetic analyses
The MTP sequences of A. thaliana, O. sativa, G. max, C. sinensis, P. trichocarpa, and E. grandis were obtained from the NCBI (www.ncbi.nlm.nih.gov) and Phytozome database (phytozome-next.jgi.doe.gov) (Gustin et al., 2011; Fu et al., 2017; Gao et al., 2020; Haque et al., 2022). The sequences of identified MTP were listed in Table S1. The sequences were aligned with Clustal W. MEGA 7.0 was used to construct neighbor-joining tree with 1,000 bootstrap trials, and the evolutionary distance was analyzed with the Poisson correction method (Qi et al., 2022).
Gene expression analyses
Total RNA was extracted from the roots and shoots of E. grandis based on the modified CTAB-LiCl approach (Singh et al., 2015). cDNA was synthesized from 1 μg of total RNA with HiScript III RT SuperMix for qPCR (+gDNA wiper) kit (Vazyme, Nanjing, China), and then, it was three-fold diluted with sterile water. Real-time PCR were performed using Bio-Rad iQ5 and ChamQ Universal SYBR qPCR Master Mix (Vazyme, Nanjing, China). The relative expression level of MTP in both roots and shoots of E. grandis, as well as auxin biosynthesis–related genes in roots, were normalized with the normalization factor EgUBI3 and presented as 2−ΔΔCt (Livak and Schmittgen, 2001). For the expression of EgMTPs, the expression level in NM roots with 5 μM Zn was defined as 1. The gene-specific primers for real-time PCR were summarized in Table S2.
Mycorrhizal colonization
Fresh AM roots were fixed in 10% KOH (W/V) solution for 2 weeks under 37°C, and the solution was renewed every 3 days. After washing with sterile water, the roots were neutralized with 2% HCl (W/V) for 15 min. After another washing twice with sterile water, the roots with WGA-Alexa Fluor 488 (WGA488) then stained for 2 h at room temperature (Xie et al., 2021). Mycorrhizal colonization was quantified following the MYCOCALC program. We also launched confocal microscopy analysis performed with Zeiss 780 laser scanning confocal microscope.
Statistical analyses
Data were analyzed by SPSS software version 19.0 (Chicago, USA). The effects of mycorrhization and Zn on gene expression and ionome concentrations were evaluated by one-way analysis of variance. Results were indicated as mean ± standard error of at least three biological replicates. Statistical differences were calculated using the Student’s t-test with P < 0.05 as the significance thresholds. In addition, GraphPad Prism (version 8.0), TBtools (Chen et al., 2020), and iTOL were used to display graphics.
Results
Effect of Zn and mycorrhiza on the growth of E. grandis
Regarding the effects of Zn stress on non-mycorrhizal (NM) plants, Zn treatment led to decrease of root and shoot fresh weight (Figures 1A, B). Inoculation with AM fungi improved both roots and shoots fresh weight of E. grandis, especially under 150 μM Zn treatment (Figures 1A, B). Considering AM plants, Zn stress on the fresh weight of roots was prominent, whereas it was not indistinctive for the shoots.
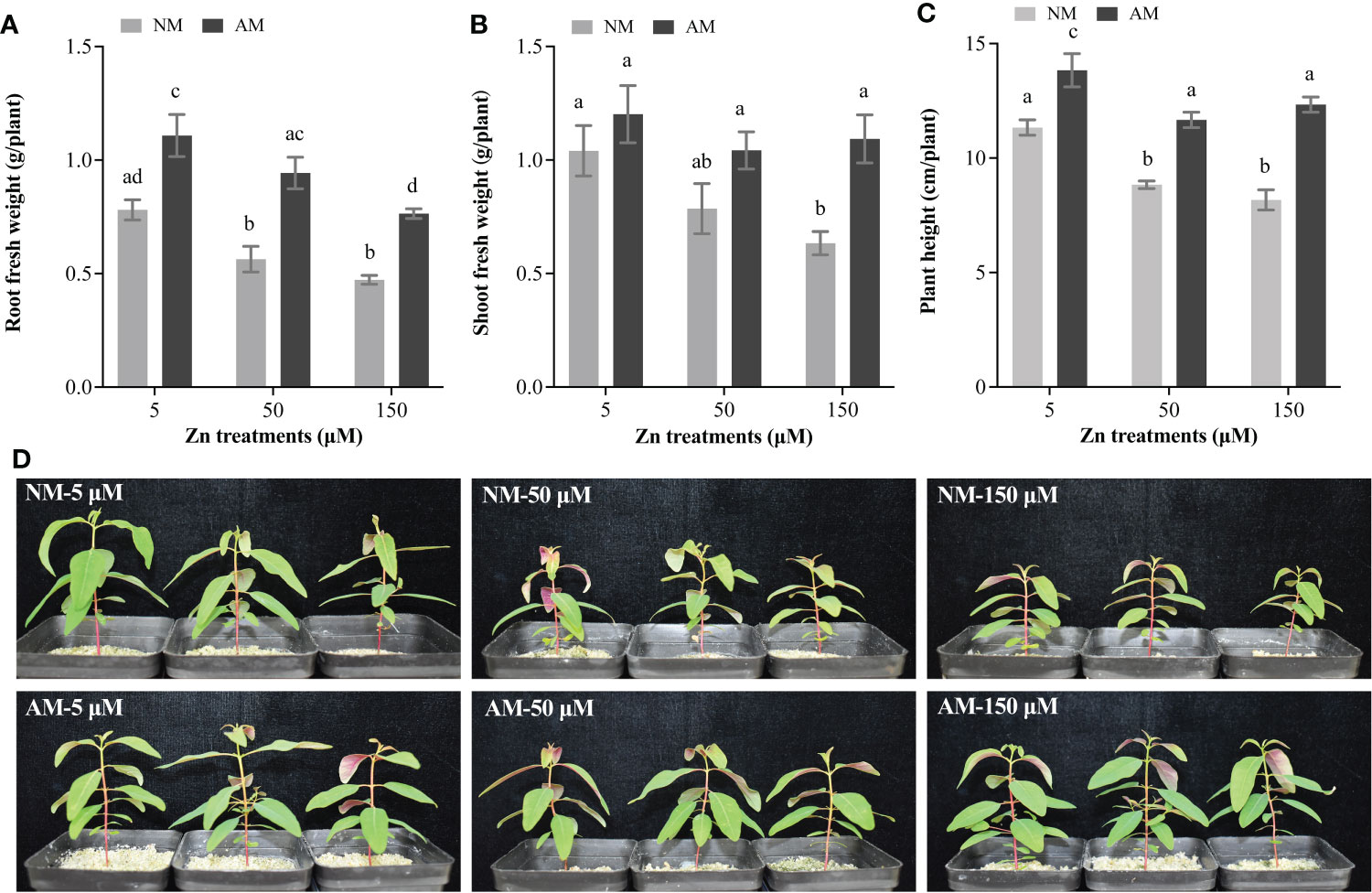
Figure 1 Effects of Zn and AM fungi on the growth of E. grandis. (A) Root fresh weight; (B) shoot fresh weight; (C) plant height; (D) the phenotype of E. grandis with or without AM fungi under 5, 50, and 150 μM Zn treatment. NM, non-mycorrhizal plants; AM, mycorrhizal plants. Values were indicated as mean ± SE of six biological replicates. Different letters above bars indicated significant differences at P < 0.05.
With the increase of Zn concentrations, the plant height was suppressed in NM plants, whereas AM fungi inoculation eased the suppression on the height of E. grandis from Zn (Figure 1C, D). However, mycorrhiza cannot completely eliminate the inhibition by Zn stress on plant height: The height of AM plants at 50 and 150 μM Zn was still significantly lower than at 5 μM Zn (Figure 1C, D).
Effect of Zn and mycorrhiza on the ionome of E. grandis
Nutrient interactions in plants, in response to variable environmental stresses, significantly affect plant survival and development. With Zn treatment, Zn accumulated in NM roots, whereas the contents of P, Mg, Fe, and Cu decreased (Figure 2A). Meanwhile, Zn accumulation also occurred in NM shoots, but the concentrations of P, Mg, Fe, and Mn declined (Figure 2B). Moreover, the concentration of Mn in roots and Cu in shoots was unaffected.
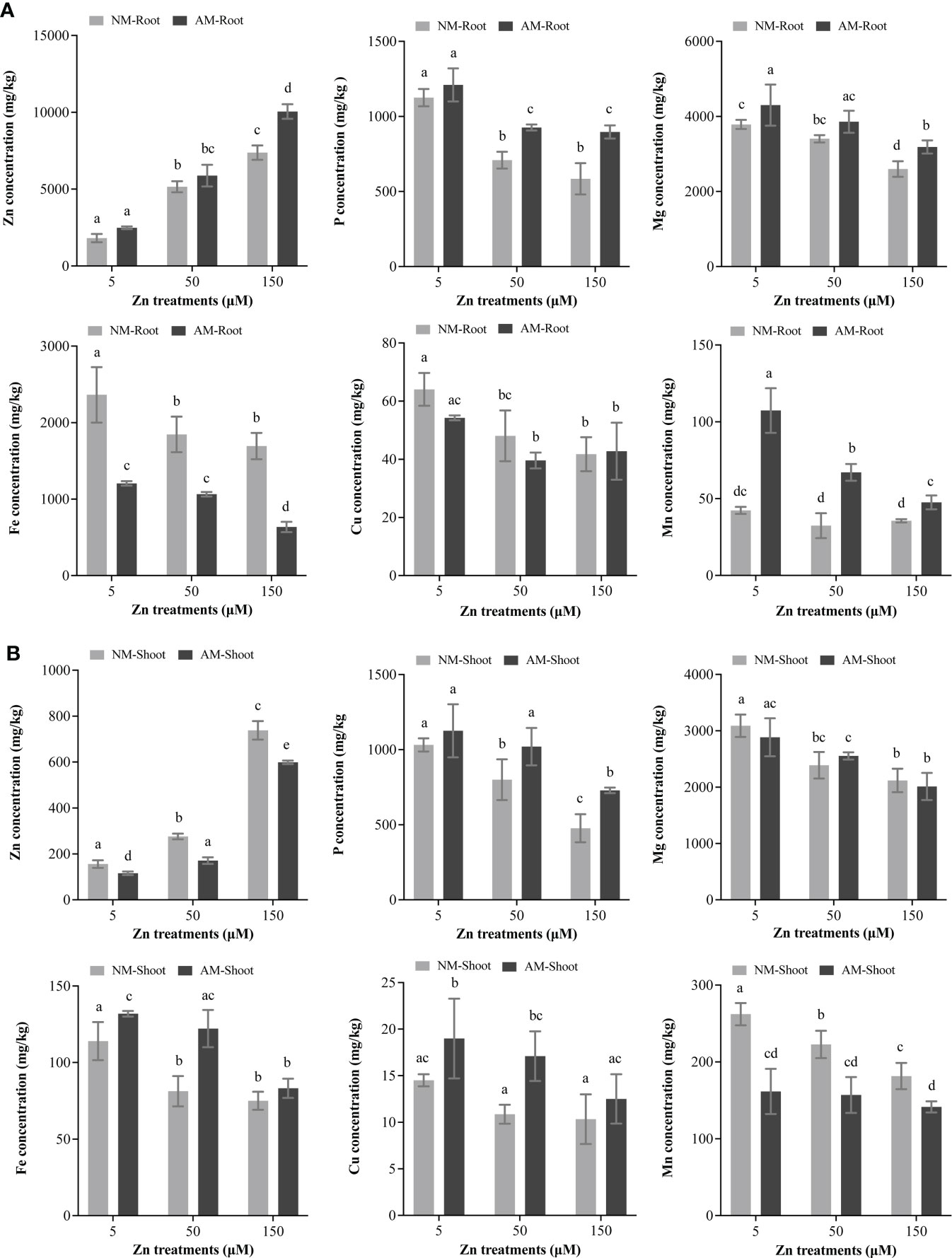
Figure 2 Total mineral element concentrations. The microelements (Zn, Fe, Cu, and Mn) and macroelements (P and Mg) of roots and shoots were analyzed by ICP-OES. NM, non-mycorrhizal plant; AM, mycorrhizal plant. (A) Total mineral concentration in root. (B) Total mineral concentration in shoot. Values were indicated as mean ± SE. Different letters above the bars indicated significant differences between treatments (P < 0.05).
Compared with NM roots, the concentration of Zn, P, Mg, and Mn was much higher in AM roots; nevertheless, Fe decreased, and Cu remained unaffected (Figure 2A). On the other hand, Zn and Mn contents were lower in AM shoots than that in NM shoots; P, Fe, and Cu were accumulated in AM shoots (Figure 2B).
MTPs of E. grandis
MTP sequences of A. thaliana were used as queries to search against E. grandis genome in Phytozome database to identify the MTP genes of E. grandis. After the conserved domain analysis, a total number of 16 MTP encoding genes were identified: EgMTP1, EgMTP2, EgMTP3.1, EgMTP3.2, EgMTP4, EgMTP5, EgMTP6, EgMTP7, EgMTP8.1, EgMTP8.2, EgMTP9.1, EgMTP9.2, EgMTP10, EgMTP11.1, EgMTP11.2, and EgMTP12 (Figure 3).
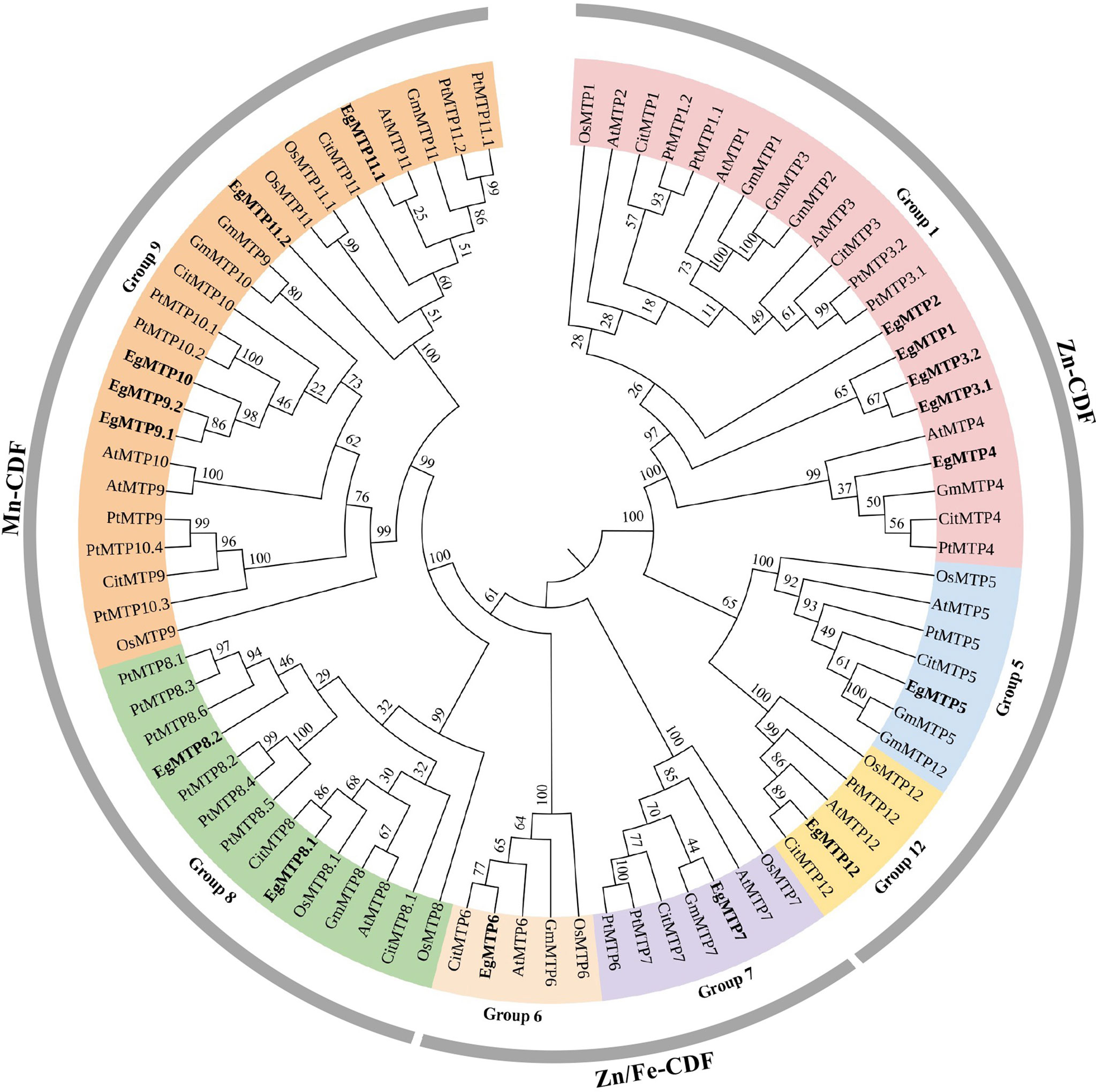
Figure 3 Phylogenetic relationships of MTP. The neighbor-joining tree was generated using MEGA 7.0 with 1,000 bootstrap replicates. E. grandis MTP proteins were in bold. At, Arabidopsis thaliana; Os, Oryza sativa; Gm, Glycine max; Pt, Populus trichocarpa; Cit, Citrus sinensis; Eg, Eucalyptus grandis.
To better understand the evolutionary characteristics and possible functions of the MTP family in E. grandis, a neighbor-joining tree consisting of 84 MTPs (including 12 MTPs from A. thaliana, 10 from O. sativa, 12 from G. max, 12 from C. sativus, 22 from P. trichocarpa, and 16 from E. grandis) was constructed (Figure 3). As previously reported, these proteins were divided into Zn-CDF, Zn/Fe-CDF, and Mn-CDF clusters (Montanini et al., 2007; Gustin et al., 2011), with seven (EgMTP1 to EgMTP5 and EgMTP12), two (EgMTP6 and EgMTP7), and seven (EgMTP8.1 to EgMTP11.2) MTP members, respectively (Figure 3).
Effect of Zn and mycorrhiza on the expression of EgMTPs
Previously, the expression of ZIP2 was upregulated by excess Zn and downregulated by AM symbiosis both in Medicago truncatula and Astragalus sinicus (Burleigh et al., 2003; Xie et al., 2021). Herein, we analyzed the expression patterns of MTPs in NM and AM plants supplied with different concentrations of Zn.
In NM roots, the expression of EgMTP1, EgMTP5, and EgMTP7 were significantly repressed by high-Zn treatment, whereas EgMTP2 presented an overall upward trend, and no significant difference was detected in EgMTP3.1, EgMTP3.2, EgMTP4, EgMTP6, and EgMTP12 (Figure 4A). As for AM root, Zn mostly reduced the expression of EgMTPs, except for EgMTP7. However, compared with NM roots, mycorrhiza induced the expression of EgMTP1, EgMTP5, and EgMTP7 (Figure 4A). Regarding to NM shoots, the expression pattern of EgMTP3.1 showed a downward trend with increased Zn concentration, whereas EgMTP1, EgMTP4, EgMTP5, EgMTP6, and EgMTP7 were upregulated (Figure 4B). In AM shoots, Zn treatment induced the expression of EgMTP1, EgMTP2, EgMTP3.1, and EgMTP5, and upregulated EgMTP1 and EgMTP5 compared with that in NM shoots (Figure 4B). Overall, mycorrhiza induced the expression of EgMTP1, EgMTP5, and EgMTP7 in roots and EgMTP1 and EgMTP5 in shoots.
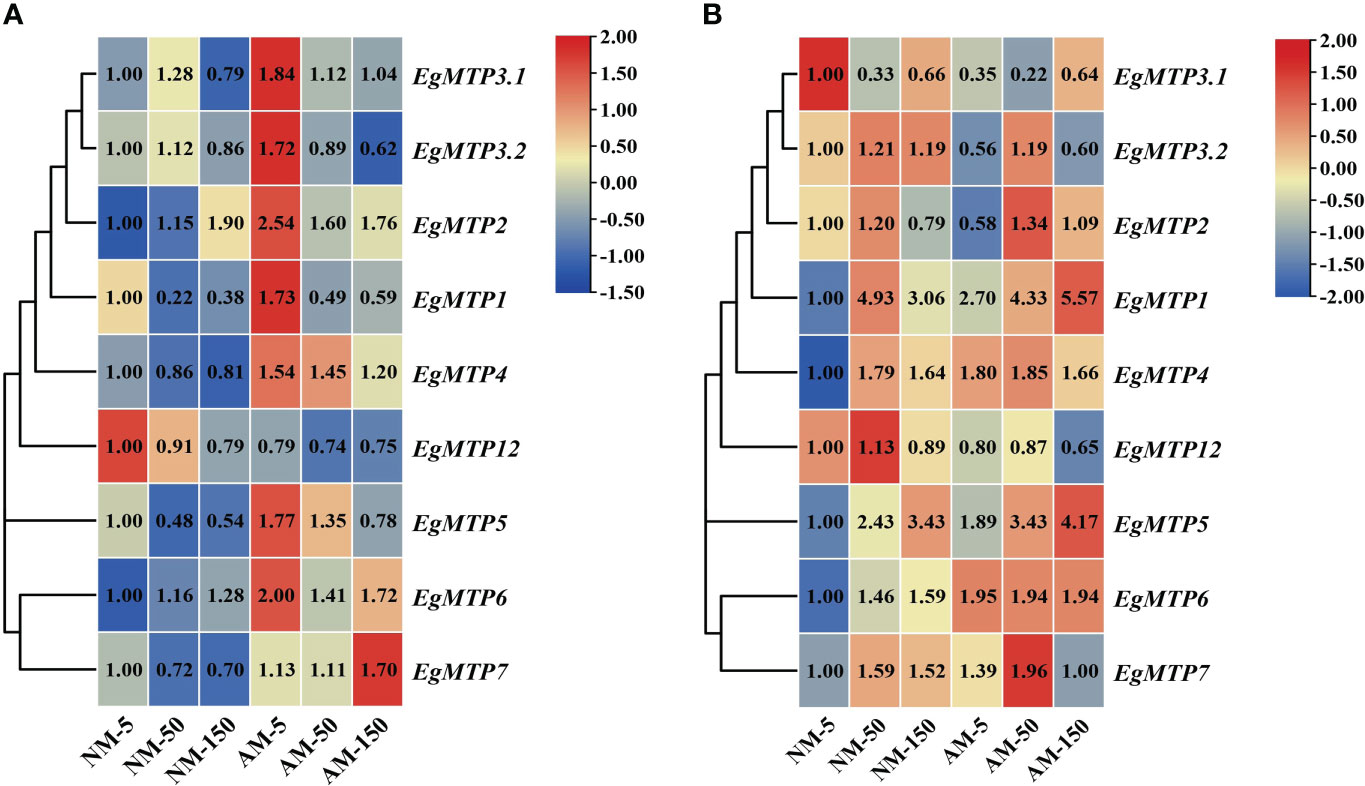
Figure 4 Expression profiles of EgMTP. The heat map was generated using the expression fold changes of EgMTP family. Relative gene expression was calculated by the 2−ΔΔCT method using the EgUBI3 as a normalizer. For each gene, the expression level in NM roots with 5 μM Zn was defined as 1. (A) The expression profiles of EgMTPs in root. (B) The expression profiles of EgMTPs in shoot.
Effect of Zn and mycorrhiza on the expression of the auxin biosynthesis–related gene
To further investigate the effects of Zn stress on auxin biosynthesis in E. grandis, we analyzed the expression patterns of EgAAO3, EgYUC2, EgYUC3, and EgAMI1. EgAAO3 was strongly induced at 50 and 150 μM Zn (Figure 5A). Meanwhile, EgYUC2 and EgYUC3 significantly expressed at 150 μM Zn (Figures 5B, C), and the expression of EgAMI1 was unaffected under any Zn concentration (Figure 5D).
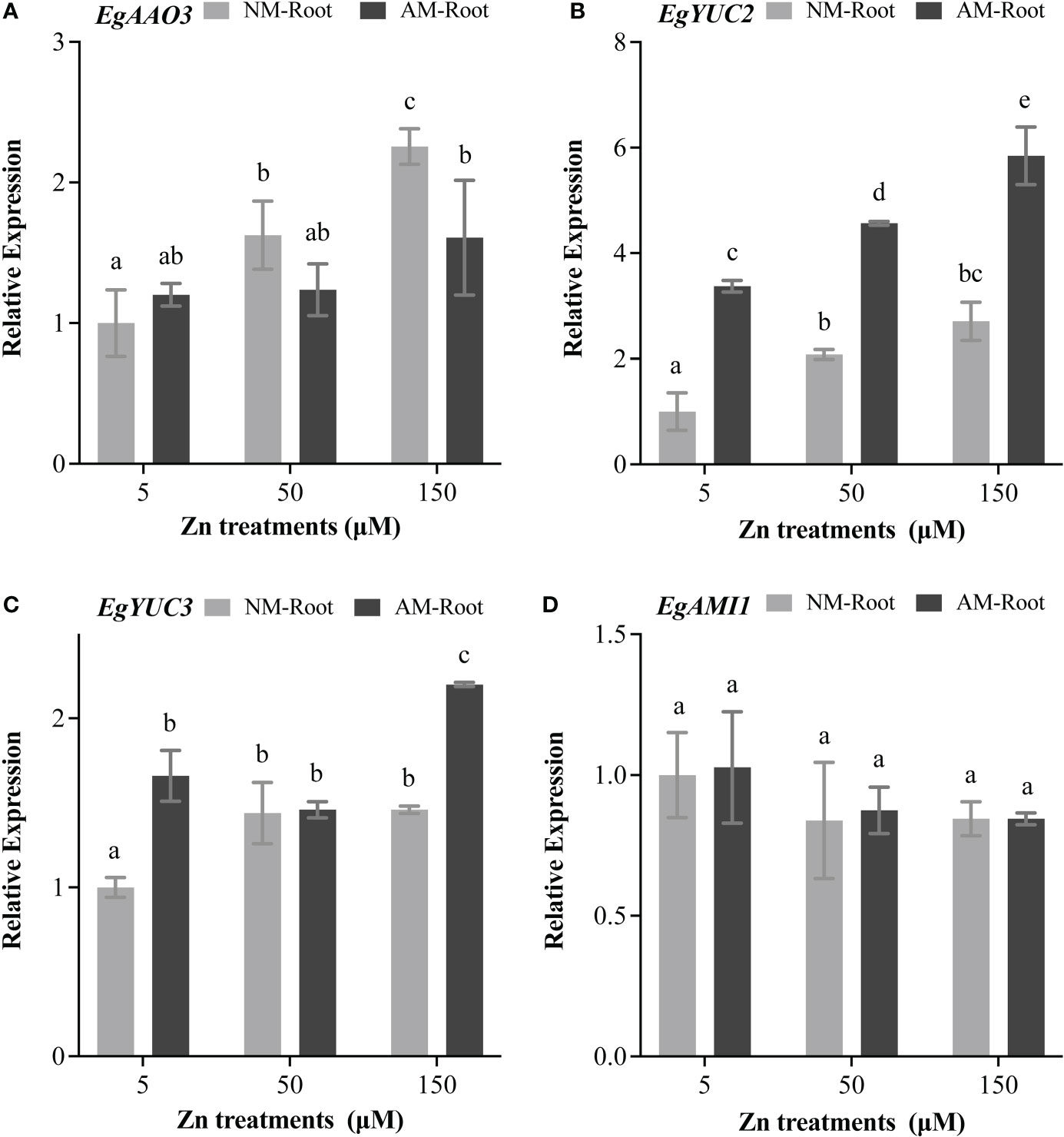
Figure 5 Expression profiles of auxin biosynthesis–related genes. The relative expression of EgAAO3 (A), EgYUC2 (B), EgYUC3 (C), and EgAMI1 (D) was calculated by the 2−ΔΔCT method using the EgUBI3 as a normalizer. For each gene, the expression level in NM roots with 5 μM Zn was defined as 1. NM-Root, the root of non-mycorrhizal plants; AM-Root, the root of mycorrhizal plants. Values were indicated as mean ± SE. Different letters on the histograms indicated significant differences (P < 0.05).
Chareesri et al. (2020) and Wang et al.(2021) demonstrated that, compared to the NM roots, the content of auxin in the mycorrhizal rice and tomato roots significantly increased. To elucidate how mycorrhiza increased auxin accumulation in AM roots, we evaluated the transcript level of EgAAO3, EgYUC2, EgYUC3, and EgAMI1. Compared with NM roots, the expression of EgYUC2 and EgYUC3 was induced in AM roots (Figures 5B, C), whereas EgAAO3 was repressed at 150 μM Zn treatment (Figure 5A).
Mycorrhizal colonization
To analyze the effect of Zn stress on AM fungi development in E. grandis, we quantified the mycorrhizal colonization rates in roots inoculated with R. irregularis. Plants grown with 5, 50, and 150 μM Zn showed similar mycorrhizal frequency (Figure 6A), based on the percentage of roots colonized by AM fungi in the whole roots. Although symbiosis had already existed for 5 weeks before the Zn treatment, mycorrhizal intensity was lower in AM roots grown at 50 and 150 μM Zn compared with that at 5 μM Zn (Figure 6B). Moreover, the arbuscule numbers decreased in AM roots exposed to a high level of Zn (Figure 6C). Roots exposed to 5 μM Zn had normal arbuscules, with full hyphal branches in the cortical cells, but fewer arbuscules were formed with high-Zn treatment (Figure 6D). In addition, intraradical hyphae at 150 μM Zn contained more septa (Figure 6D), which was a morphological signature of degradation of AM fungi. Intraradical hyphae was the channel for transporting nutrients in AM fungi, and the formation of septa prevented transport of nutrients to arbuscule, which led to the death of the arbuscule. In a nutshell, high-Zn treatments disturbed the arbuscule development through forming more septa in intraradical hyphae.
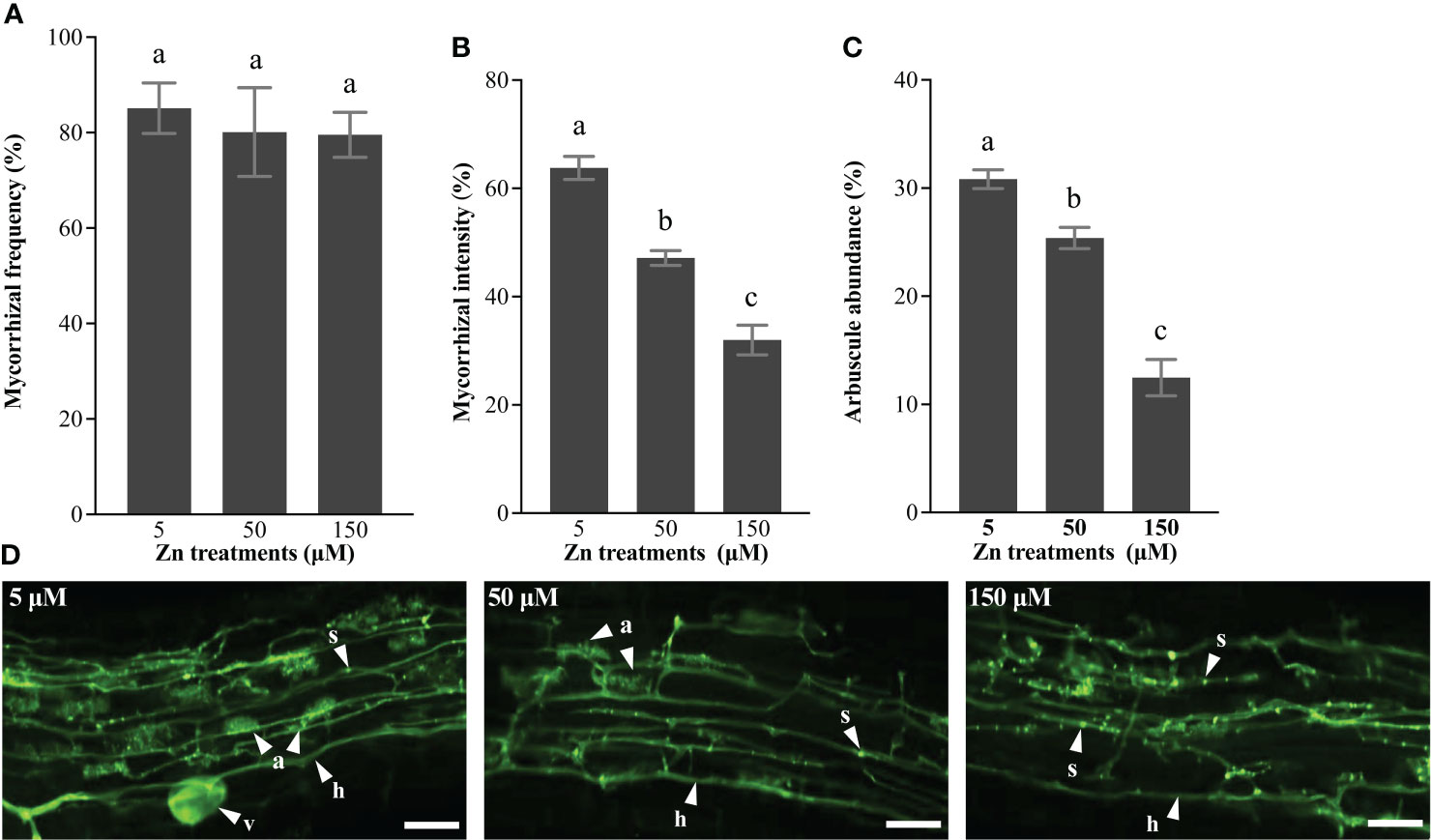
Figure 6 Mycorrhizal colonization. (A) Mycorrhizal frequency, (B) mycorrhizal intensity, and (C) arbuscule abundance were quantified using the MYCOCALC program. Values were indicated as mean ± SE. Different letters on the histograms indicated that the means significant differences (P < 0.05). (D) a, arbuscule; h, hyphae; v, vesicles; s, septa; bar, 50 μm.
Discussion
Mycorrhiza promotes the growth of E. grandis under Zn stress
AM fungi were beneficial symbionts of plants that increased host resistance to various environmental stresses. Mycorrhizal Betula pubescens had higher fresh and dry weight than NM plants in metal-polluted industrial soil (Fernández-Fuego et al., 2017). In Cu-contaminated soils, symbiosis facilitated Cu tolerance of maize with increasing fresh weight (Gómez-Gallego et al., 2022). In our study, significantly higher biomass and height were detected in mycorrhizal E. grandis (Figure 1). AM fungi absorbed nutrients beyond the depletion zone that develops around the roots through external mycelium and then delivers them to host roots (Wang et al., 2017; Xie et al., 2021; Gómez-Gallego et al., 2022). This was a quite effective way to promote plant growth, and higher biomass further enhances the Zn tolerance of plants.
Zn and mycorrhiza affect the uptake of mineral elements
The growth and development of higher plants needed at least 17 essential elements, among which P and Mg were known as macroelements; Fe, Zn, Cu, and Mn were regarded as microelements; and the interactions between macro- and microelement were one of the key processes in the life cycle of plants (Xie et al., 2019; Fan et al., 2021; Kumar et al., 2021). Previous studies indicated that excess Zn triggered P starvation in lettuce and rice (Bouain et al., 2014; Ding et al., 2021). Similarly, we observed Zn increasing and P decreasing in both root and shoot of E. grandis along with elevation of Zn treatment (Figure 2). High Zn downregulated the expression of uptake and transporter-related genes of P (Ding et al., 2021), which inhibited the uptake and translocation of P; thus, less P was transported to shoots under high-Zn treatment (Figure 2). Consisting with the case of rapeseed seedlings (Wang et al., 2009), the concentrations of Fe and Mg in roots and shoots of E. grandis decreased with surplus Zn (Figure 2). On the other hand, when maize was grown in high-Zn condition, the concentrations of K, Ca, Mg, Fe, Mn, Ni, and Co significantly decreased in root, and Mn and Cu diminished in shoot along with the increase of S, Mg, and Mo (Bokor et al., 2015). Excessive Zn affected the level of Fe sensing, resulting in Fe deficiency (Leskova et al., 2017). However, Cu content reduced in roots and Mn increased in shoots of E. grandis with a high level of Zn (Figure 2). Cross-talks between mineral nutrients involved with complicated mechanisms; therefore, multi-level interactions among nutrient elements needed further explore to better understand their availability.
AM fungi can improve shoot biomass and retain metals in roots to restrict their translocation to aerial parts under heavy metal stress (Huang et al., 2018; Janeeshma and Puthur, 2020; Riaz et al., 2021). In our study, more Zn was accumulated in AM roots; less Zn, therefore, was transferred to AM shoots (Figure 2). Moreover, AM fungal hyphal network functionally extended the root system of hosts, granting the hosts the ability to uptake mineral nutrients from enlarged soil volume to enhance the metal tolerance of hosts (Göhre and Paszkowski, 2006; Wang et al., 2017; Gómez-Gallego et al., 2022). For example, Thlaspi praecox grew in soils highly contaminated by Cd, Zn, and Pb, the concentrations of P, S, Ni, and Cu in both AM shoots and roots were found to be increased (Vogel-Mikuš et al., 2006). AM fungi improved the nutritional (P, N, Mg, and Fe) and water status, and stimulated proline biosynthesis of hosts, which enhanced the tolerance to Cd and Zn (Garg and Singh, 2018). In addition, concentrations of P, K, Mg, and Ca in mycorrhizal maize grown in Cu-contaminated soil were often higher than that in NM plants (Gómez-Gallego et al., 2022). Mycorrhiza significantly increased the concentrations of P, Mg, and Mn in E. grandis roots, as well as P, Fe, and Cu in shoots. However, Fe concentration in AM roots was lower than that in NM roots but higher in AM shoots than that in NM shoots (Figure 2), probably because the effect of Zn accumulation was dominant and stimulates Fe transporting from root to shoot.
To sum up, high Zn threatens plant growth by disturbing the homeostasis of nutrient elements, and AM fungi eases the stress to some extent.
Zn and mycorrhiza regulate the expression of EgMTPs
MTPs played vital roles in exporting excess metal ions into subcellular compartments or to extracellular space (Desbrosses-Fonrouge et al., 2005; Arrivault et al., 2006; Fujiwara et al., 2015; Migocka et al., 2018; Sinclair et al., 2018; Gao et al., 2020). Because MTPs of Mn-CDF cluster mostly transported Mn (Montanini et al., 2007), we analyzed the expression patterns of MTPs of Zn-CDF and Zn/Fe-CDF clusters. As showed in Figure 3, EgMTP1, EgMTP2, EgMTP3.1, EgMTP3.2, EgMTP4, EgMTP5, EgMTP12, and EgMTP7 of E. grandis had high similarity with MTPs of A. thaliana, G. max, P. trichocarpa, and C. sinensis, which implied that they shared comparable functions. Previous studies reported that AtMTP1 and AtMTP3 were vacuolar membrane transporters (Desbrosses-Fonrouge et al., 2005; Arrivault et al., 2006), and MTP1, MTP3, MTP4, and MTP7 of G. max, P. trichocarpa, and C. sinensis were also predicted to be located at vacuole (Fu et al., 2017; Gao et al., 2020; Haque et al., 2022). AtMTP5, AtMTP12, and CsMTP5 were localized at the Golgi compartment (Fujiwara et al., 2015; Migocka et al., 2018), whereas AtMTP2 and EgMTP6 were endoplasmic reticulum membrane proteins (Sinclair et al., 2018; Han et al., 2022). According to the grouping in the tree (Figure 3), we thus assumed that EgMTP1, EgMTP3.1, EgMTP3.2, EgMTP4, and EgMTP7 were located at vacuole; EgMTP2 and EgMTP6 were localized in the endoplasmic reticulum membrane; and EgMTP5 and EgMTP12 were membrane transporters of Golgi apparatus.
Desbrosses-Fonrouge et al. (2005) found that AtMTP1 acted to exclude excess Zn into vacuoles and driven Zn accumulation in young leaves. In this study, the expression of EgMTP1 was suppressed in root but induced in NM shoot under Zn treatment (Figure 4); the expression pattern was similar with that of PtrMTP1 (Gao et al., 2020). As Zn can enter plant cell non-specifically through plasma membrane transport proteins (Arrivault et al., 2006), the overaccumulated Zn in root suppressed the expression of EgMTP1 and enhanced the transfer of Zn from root to shoot; then, excessive Zn in shoot upregulated the expression of EgMTP1 to promote the storage of Zn in shoot vacuole. AtMTP3 mostly expressed in root and functioned in the immobilization of Zn in root vacuoles, restricting the movement of Zn from root into shoot (Arrivault et al., 2006). The expression of EgMTP3.1 in NM shoot was reduced under Zn oversupply, indicating that EgMTP3.1 mediated Zn exclusion from shoot. Conversely, EgMTP4 in NM shoot was induced under Zn oversupply (Figure 4). We speculated that EgMTP4 and MTP3 had difference physiological functions, and EgMTP4 promoted the Zn storage in shoot vacuoles. The expression level of EgMTP7 was strongly intensified by Zn in NM shoot (Figure 4), a case similar with PtMTP7 (Gao et al., 2020), suggesting that EgMTP7 transported Zn into shoot vacuole to remit the Zn toxicity.
AtMTP5 and AtMTP12 formed functional heterodimer to load Zn into Golgi, but the expression of AtMTP12 was irrelevant to Zn concentration (Fujiwara et al., 2015). Similarly, the expression of EgMTP12 in NM plants was not affected by Zn (Figure 4). In cucumber, CsMTP5 and CsMTP12 also functioned as a heterodimeric complex, which involved in transporting Zn into Golgi compartment, and the expression of CsMTP5 was obviously upregulated with low-Zn treatment (Migocka et al., 2018). Conversely, a high level of Zn increased the expression of EgMTP5 in both NM roots and shoots (Figure 4). Therefore, we propose that the heterodimeric complex EgMTP5-EgMTP12 functions to deliver excess Zn to Golgi compartment and is regulated by zinc at the level of EgMTP5 transcription.
AtMTP2 contributed to the root-to-shoot Zn translocation through plasmodesmus (Sinclair et al., 2018). The high transcriptional level of EgMTP2 in NM roots responded to excess Zn (Figure 4); therefore, more Zn was transferred to the shoot through symplast pathway. In addition, our previous study found that the expression of EgMTP6 was irrelevant to Zn concentration, and heterologous expression of EgMTP6 in zrc1-mutant yeast enhanced the Zn tolerance of zrc1Δ, which cannot grow in high-Zn condition (Han et al., 2022). Thus, EgMTP6 mediated the sequestration of Zn to endoplasmic reticulum in the non-transcript level.
Recently, Gómez-Gallego et al. (2022) found an enhanced expression of the vacuolar membrane transporters ZmHMA3a and ZmHMA4 in AM plants under Cu stress. Mycorrhiza promoted sequestering Cu into vacuole of root and shoot to reduce Cu translocation to aerial part by regulating the genes of Cu transporters (Gómez-Gallego et al., 2022). In Astragalus sinicus, mycorrhiza downregulated the expression of AsZIP2 to reduce absorbing excessive Zn (Xie et al., 2021). It seems that mycorrhiza downregulates genes involved in metal uptake and upregulates genes related to exportation to protect host plant from metals toxicity. With Zn oversupply, mycorrhiza increased the expression of EgMTP1 and EgMTP7 in roots, as well as EgMTP1 and EgMTP5 in shoots, to facilitate the transport of excess Zn into vacuole and Golgi for plant detoxifying.
Zn and mycorrhiza affect the expression of auxin biosynthesis–related genes
In recent years, the alterations of auxin biosynthesis and transport induced by heavy metals stimuli had been intensively explored (Jiang et al., 2018; López-Ruiz et al., 2020; Angulo-Bejarano et al., 2021; Wang et al., 2021). For instance, toxic Cu, Al, Fe, and Ni disturbed auxin biosynthesis and distribution in root tips to inhibit root growth and development (Wu et al., 2014; Li et al., 2015; Song et al., 2017; Leškovï et al., 2020). Different from excess Se that decreased the auxin biosynthesis via reducing the expression of YUCCA1 and YUCCA3 in rice plants (Malheiros et al., 2019), excess Zn enhanced the expression of EgAAO3, EgYUC2, and EgYUC3 (Figure 5). Previously, a comparative transcriptomic investigation indicated that 24-h treatment of 200 μM Zn significantly induced the expression of auxin biosynthesis genes (ATP SULFURYLASE ARABIDOPSIS1, SUPERROOT1, TRYPTOPHAN AMINOTRANSFERASE OF ARABIDOPSIS1, YUC2, YUC3, CYTOCHROME P450, and AAO3) (Zhang et al., 2018). On the other hand, Cu toxicity was found to inhibit auxin biosynthesis via reducing the expression of TAA1 and YUCCA (Song et al., 2017). In contrast, transcriptomic analyses of auxin biosynthetic-related genes, including auxin amide synthase and tryptophan synthase, showed that Cu induced their expression (Zhao et al., 2009). The discrepancies in results might be related to differences in experimental exposure time and treatment approach. However, excess Zn promoted the expression of AAO3, YUC2, and YUC3 in E. grandis.
AM fungi had a positive effect on the regulation of the auxin levels in plants under salt stress, drought, and biotic stress, and auxin concentration in mycorrhizal plants was higher than nonmycorrhizal plants (He et al., 2017; Liu et al., 2016; Chareesri et al., 2020). Moreover, the activity of synthetic auxin-inducible promoter DR5 increased in roots colonized by R. irregularis, mainly in cells containing arbuscules (Etemadi et al., 2014). Mycorrhiza is thus likely to promote auxin synthesis. Our results showed that EgYUC2 and EgYUC3 were upregulated in AM roots (Figure 5). Furthermore, the positive correlation between auxin content and arbuscule abundance suggested that maintaining cellular auxin homoeostasis was involved in finely tuning AM symbiosis (Hanlon and Coenen, 2011). The auxin content with denser AM fungi colonization was higher than those with sparser colonization in rice (Chareesri et al., 2020). According to this study, the arbuscule number at 150 μM Zn was significantly lower than at 5 μM and 50 μM Zn, but the expression of EgYUC2 and EgYUC3 was higher. The result reveals that, although Zn restrains the growth of mycorrhiza, it remarkably promotes the expression of auxin synthesis genes.
Conclusion
To sum up, we herein propose a mechanism of Zn detoxification in E. grandis (Figure 7): EgMTP1, EgMTP3.1, EgMTP3.2, EgMTP4, and EgMTP7 involve in sequestering Zn in vacuole; EgMTP2 and EgMTP6 mediate the Zn transport into endoplasmic reticulum; and EgMTP5 and EgMTP12 load Zn into Golgi. AM fungi inoculation enhances the expression of two putative tonoplast transporters (EgMTP1 and EgMTP7) and one Golgi transporter (EgMTP5) in E. grandis under Zn toxicity, indicating that mycorrhiza facilitates the transfer of Zn into vacuole and Golgi. In addition, mycorrhiza promotes mineral nutrient uptake to improve the growth of E. grandis and induces the expression of auxin biosynthesis–related genes to improve mycorrhizal colonization to enhance the Zn tolerance. The results will be valuable to the development of molecular markers for cultivar breeding of eucalyptus with a high Zn tolerance. Further functional investigations were required to better understand their role of EgMTPs to alleviate Zn toxicity.
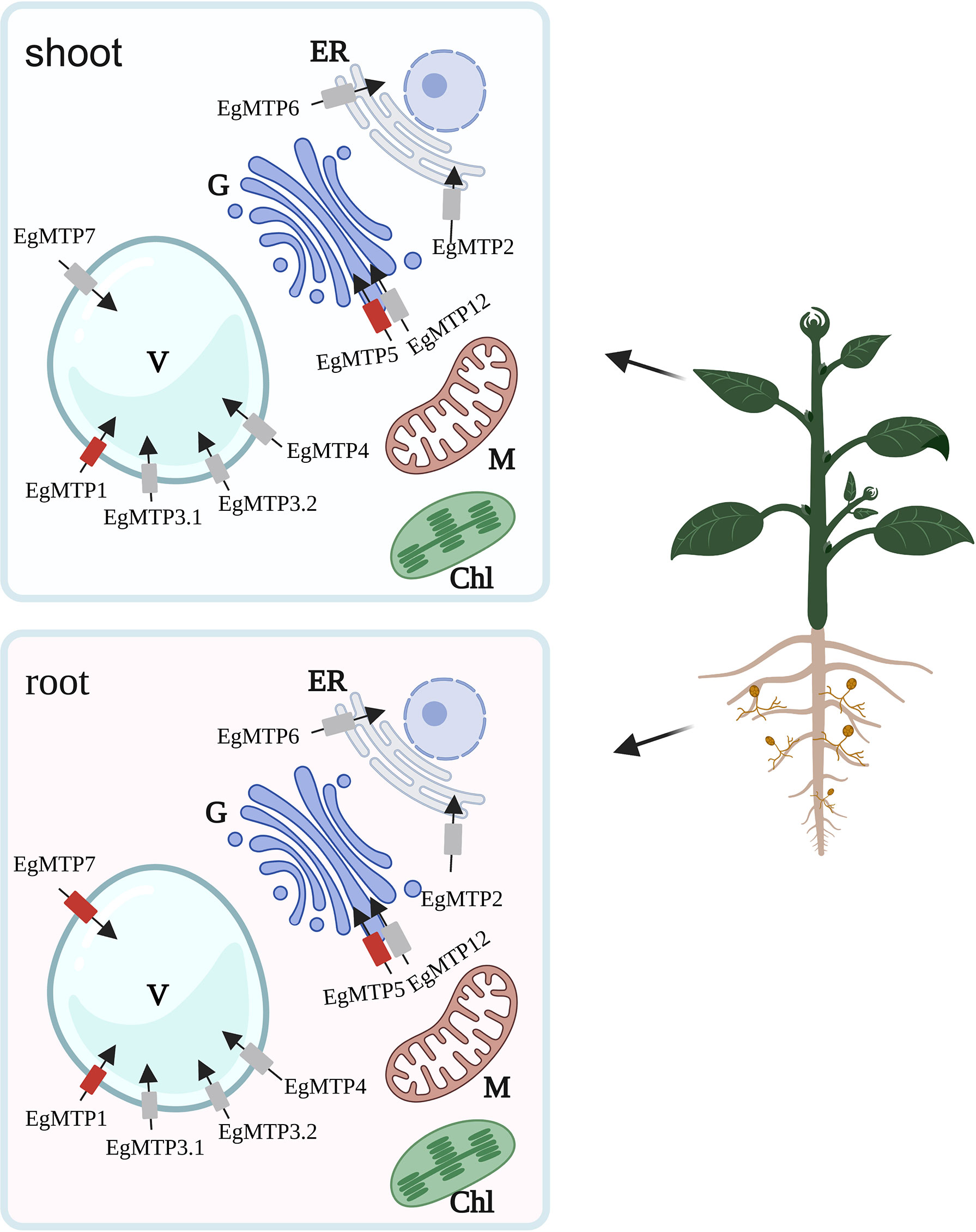
Figure 7 Schematic representation of the major changes induced by Zn in EgMTP expression in mycorrhizal and E. grandis. Red transporters, upregulated EgMTPs by mycorrhizal; ER, endoplasmic reticulum; V, vacuole; G, golgi; Chl, chloroplast; M, mitochondrion.
Data availability statement
The raw data supporting the conclusions of this article will be made available by the authors, without undue reservation.
Author contributions
L-NH: Conceptualization, methodology, data analysis and curation, visualization, writing, and editing. S-JW, YR, and X-YW: Methodology and data analysis. X-AX and W-TH: Methodology. HC and MT: Conceptualization, editing, and funding acquisition. All authors contributed to the article and approved the submitted version.
Funding
This work was supported by the Key Projects of Guangzhou of Science and Technology Plan (grant no. 201904020022), the Laboratory of Lingnan Modern Agriculture Project (grant no. NZ2021025), and the National Natural Science Foundation of China (grant nos. 31800092 and 32071639).
Acknowledgments
We particularly thank Lei Duan of South China Botanical Garden, Chinese Academy of Sciences for refining the text.
Conflict of interest
The authors declare that the research was conducted in the absence of any commercial or financial relationships that could be construed as a potential conflict of interest.
Publisher’s note
All claims expressed in this article are solely those of the authors and do not necessarily represent those of their affiliated organizations, or those of the publisher, the editors and the reviewers. Any product that may be evaluated in this article, or claim that may be made by its manufacturer, is not guaranteed or endorsed by the publisher.
Supplementary material
The Supplementary Material for this article can be found online at: https://www.frontiersin.org/articles/10.3389/fpls.2022.1022696/full#supplementary-material
References
Adams, F., Reddell, P., Webb, M. J., Warren, S. A. (2006). Arbuscular mycorrhizas and ectomycorrhizas on Eucalyptus grandis (Myrtaceae) trees and seedlings in native forests of tropical north-eastern Australia. Aust. J. Bot. 54, 271–281. doi: 10.1071/BT05028
Angulo-Bejarano, P. I., Puente-Rivera, J., Cruz-Ortega, R. (2021). Metal and metalloid toxicity in plants: an overview on molecular aspects. Plants 10 (4), 635. doi: 10.3390/plants10040635
Arrivault, S., Toralf, S., Krämer, U. (2006). The arabidopsis metal tolerance protein AtMTP3 maintains metal homeostasis by mediating zn exclusion from the shoot under fe deficiency and zn oversupply. Plant J. 46, 861–879. doi: 10.1111/j.1365-313X.2006.02746
Bae, B., Park, H., Kang, S. (2022). Quantitative estimation of synergistic toxicity of Cu and zn on growth of Arabidopsis thaliana by isobolographic method. Toxics 10 (4), 195. doi: 10.3390/toxics10040195
Bokor, B., Bokorová, S., Ondoš, S., Švubová, R., Lukačová, Z., Hýblová, M., et al. (2015). Ionome and expression level of Si transporter genes (Lsi1, Lsi2, and Lsi6) affected by zn and Si interaction in maize. Environ. Sci. pollut. Res. 22, 6800–6811. doi: 10.1007/s11356-014-3876-6
Bouain, N., Kisko, M., Rouached, A., Dauzat, M., Lacombe, B., Belgaroui, N., et al. (2014). Phosphate/zinc interaction analysis in two lettuce varieties reveals contrasting effects on biomass, photosynthesis, and dynamics of pi transport. J. Biomed. Biotechnol. 3), 548254. doi: 10.1155/2014/548254
Broadley, M. R., Philip, W. J., John, H. P., Ivan, Z., Lux, A. (2007). Zinc in plants. New Phytol. 173, 677–702. doi: 10.1111/j.1469-8137.2007.01996
Brundrett, M. C., Tedersoo, L. (2018). Evolutionary history of mycorrhizal symbioses and global host plant diversity. New Phytol. 220, 1108–1115. doi: 10.1111/nph.14976
Burleigh, S. H., Kristensen, B. K., Bechmann, I. E. (2003). A plasma membrane zinc transporter from Medicago truncatula is up-regulated in roots by zn fertilization, yet down-regulated by arbuscular mycorrhizal colonization. Plant Mol. Biol. 52, 1077–1088. doi: 10.1023/A:1025479701246
Canton, G. C., Bertolazi, A. A., Cogo, A. J. D., Eutrópio, F. J., Melo, J., de Souza, S. B., et al. (2016). Biochemical and ecophysiological responses to manganese stress by ectomycorrhizal fungus Pisolithus tinctorius and in association with Eucalyptus grandis. Mycorrhiza 26, 475–487. doi: 10.1007/s00572-016-0686-3
Cardini, A., Pellegrino, E., White, P. J., Mazzolai, B., Mascherpa, M. C., Ercoli, L. (2021). Transcriptional regulation of genes involved in zn transport after foliar zn application to Medicago sativa. Plants 10 (3), 476. doi: 10.3390/plants10030476
Chareesri, A., De Deyn, G. B., Sergeeva, L., Polthanee, A., Kuyper, T. W. (2020). Increased arbuscular mycorrhizal fungal colonization reduces yield loss of rice (Oryza sativa l.) under drought. Mycorrhiza 30, 315–328. doi: 10.1007/s00572-020-00953-z
Charoenpakdee, S., Phosri, C., Dell, B., Lumyong, S. (2010). The mycorrhizal status of indigenous arbuscular mycorrhizal fungi of physic nut (Jatropha curcas) in Thailand. Mycosphere 1 (2), 167–181.
Chen, C. J., Chen, H., Zhang, Y., Thomas, H. R., Frank, M. H., He, Y. H., et al. (2020). TBtools: an integrative toolkit developed for interactive analyses of big biological data. Mol. Plant 13, 1194–1202. doi: 10.1016/j.molp.2020.06.009
Chen, Y. L., Liu, S., Dell, B. (2007). Mycorrhizal status of Eucalyptus plantations in south China and implications for management. Mycorrhiza 17, 527–535. doi: 10.1007/s00572-007-0125-6
Clemens, S., Palmgren, M., Kraemer, U. (2002). A long way ahead: understanding and engineering plant metal accumulation. Trends Plant Sci. 7 (7), 309–315. doi: 10.1016/S1360-1385(02)02295-1
Desbrosses-Fonrouge, A. G., Voigt, K., Schröder, A., Arrivault, S., Thomine, S., Krämer, U. (2005). Arabidopsis thaliana MTP1 is a zn transporter in the vacuolar membrane which mediates zn detoxification and drives leaf zn accumulation. FEBS Lett. 579, 4165–4174. doi: 10.1016/j.febslet.2005.06.046
Díaz, G., Azcón-Aguilar, C., Honrubia, M. (1996). Influence of arbuscular mycorrhizae on heavy metal (Zn and Pb) uptake and growth of Lygeum spartum and Anthyllis cytisoides. Plant Soil 180, 241–249. doi: 10.1007/bf00015307
Ding, J. L., Liu, L., Wang, C., Shi, L., Xu, F. S., Cai, H. M. (2021). High level of zinc triggers phosphorus starvation by inhibiting root-to-shoot translocation and preferential distribution of phosphorus in rice plants. Environ. pollut. 277, 116778. doi: 10.1016/j.envpol.2021.116778
Etemadi, M., Gutjahr, C., Couzigou, J. M., Zouine, M., Lauressergues, D., Timmers, A. (2014). Auxin perception is required for arbuscule development in arbuscular mycorrhizal symbiosis. Plant Physiol. 166 (1), 281–292. doi: 10.1104/pp.114.246595
Fan, X. N., Zhou, X. Q., Chen, H., Tang, M., Xie, X. A. (2021). Cross-talks between macro- and micronutrient uptake and signaling in plants. Front. Plant Sci. 122076. doi: 10.3389/fpls.2021.663477
Fernández-Fuego, D., Keunen, E., Cuypers, A., Bertrand, A., González, A. (2017). Mycorrhization protects Betula pubescens ehr. from metal-induced oxidative stress increasing its tolerance to grow in an industrial polluted soil. J. Hazard Mater. 336, 119–127. doi: 10.1016/j.jhazmat.2017.04.065
Ferrol, N., Tamayo, E. (2016). The heavy metal paradox in arbuscular mycorrhizas: from mechanisms to biotechnological applications. J. Exp. Bot. 67 (22), 6253–6265. doi: 10.1093/jxb/erw403
Fujiwara, T., Kawachi, M., Sato, Y., Mori, H., Kutsuna, N., Hasezawa, S. (2015). A high molecular mass zinc transporter MTP12 forms a functional heteromeric complex with MTP5 in the golgi in Arabidopsis thaliana. FEBS J. 282, 1965–1979. doi: 10.1111/febs.13252
Fu, X. Z., Tong, Y. H., Zhou, X., Ling, L. L., Chun, C. P., Cao, L., et al. (2017). Genome-wide identification of sweet orange (Citrus sinensis) metal tolerance proteins and analysis of their expression patterns under zinc, manganese, copper, and cadmium toxicity. Gene 629, 1–8. doi: 10.1016/j.gene.2017.07.072
Gao, Y. F., Yang, F. M., Liu, J. K., Xie, W., Zhang, L., Chen, Z. H., et al. (2020). Genome-wide identification of metal tolerance protein genes in Populus trichocarpa and their roles in response to various heavy metal stresses. Int. J. Mol. Sci. 21 (5), 1680. doi: 10.3390/ijms21051680
Garg, N., Singh, S. (2018). Arbuscular mycorrhiza Rhizophagus irregularis and silicon modulateg growth, proline biosynthesis and yield in Cajanus cajan l. millsp. (pigeonpea) genotypes under cadmium and zinc stress. J. Plant Growth Regul. 37, 46–63. doi: 10.1007/s00344-017-9708-4
Göhre, V., Paszkowski, U. (2006). Contribution of the arbuscular mycorrhizal symbiosis to heavy metal phytoremediation. Planta 223 (6), 1115–1122. doi: 10.1007/s00425-006-0225-0
Gómez-Gallego, T., Valderas, A., van Tuinen, D., Ferrol, N. (2022). Impact of arbuscular mycorrhiza on maize P1B-ATPases gene expression and ionome in copper-contaminated soils. Ecotoxicol. Environ. Saf. 234, 113390. doi: 10.1016/j.ecoenv.2022.113390
Gustin, J. L., Zanis, M. J., Salt., D. E. (2011). Structure and evolution of the plant cation diffusion facilitator family of ion transporters. BMC Evol. Biol. 11, 76. doi: 10.1186/1471-2148-11-76
Hanlon, M. T., Coenen, C. (2011). Genetic evidence for auxin involvement in arbuscular mycorrhiza initiation. New Phytol. 189, 701–709. doi: 10.1111/j.1469-8137.2010.03567.x
Han, L. N., Xie, X. A., Chen, H., Tang, M. (2022). Molecular characteristics and function analyses of EgMTP6 in Eucalyptus grandis. Scienta sylvae sinicae 5 (58), 93–101. doi: 10.11707/j.1001-7488.20220500
Haque, M., Rahman, A., Urmi, D., Rahman, M., Elseehy, M. M., El-shehawi, A. M., et al. (2022). Changes in physiological responses and MTP (metal tolerance protein) transcripts in soybean (Glycine max) exposed to differential iron availability. Plant Physiol. Biochem. 179, 1–9. doi: 10.1016/j.plaphy.2022.03.007
Haydon, M. J., Cobbett, C. S. (2007). A novel major facilitator superfamily protein at the tonoplast influences zinc tolerance and accumulation in Arabidopsis. Plant Physiol. 143 (4), 1705–1719. doi: 10.1104/pp.106.092015
He, L., Li, C., Liu, R. (2017). Indirect interactions between arbuscular mycorrhizal fungi and Spodoptera exigua alter photosynthesis and plant endogenous hormones. Mycorrhiza 27 (6), 525–535. doi: 10.1007/s00572-017-0771-2
Hewitt, E. J. (1966). Sand and water culture methods used in the study of plant nutrition. Commonwealth Agric. Bureaux (UK) 547.
Huang, X., Wang, L., Zhu, S., Ho, S. H., Wu, J., Kalita, P. K., et al. (2018). Unraveling the effects of arbuscular mycorrhizal fungus on uptake, translocation, and distribution of cadmium in Phragmites australis (Cav.) trin. ex steud. Ecotoxicol. Environ. Saf. 149, 43–50. doi: 10.1016/j.ecoenv.2017.11.011
Janeeshma, E., Puthur, J. T. (2020). Direct and indirect influence of arbuscular mycorrhizae on enhancing metal tolerance of plants. Arch. Microbiol. 202 (1), 1–16. doi: 10.1007/s00203-019-01730-z
Jiang, J., Rodriguez-Furlan, C., Wang, J. Z., de Souza, A., Ke, H., Pasternak, T., et al. (2018). Interplay of the two ancient metabolites auxin and MEcPP regulates adaptive growth. Nat. Commun. 9, 1. doi: 10.1038/s41467-018-04708-5
Kaur, H., Garg, N. (2021). Zinc toxicity in plants: a review. Planta 253, 129. doi: 10.1007/s00425-021-03642-z
Kumar, S., Kumar, S., Mohapatra, T. (2021). Interaction between macro- and micro-nutrients in plants. Front. Plant Sci. 12. doi: 10.3389/fpls.2021.665583
Leskova, A., Giehl, R. F. H., Hartmann, A., Fargasova, A., von Wiren, N. (2017). Heavy metal induces iron deficiency responses at different hierarchic and regulatory levels. Plant Physiol. 174, 1648–1668. doi: 10.1104/pp.16.01916
Leškovï, A., Zvarï, K. M., Araya, T., Giehl, R. F. H. (2020). Nickel toxicity targets cell wall related processes and pin2-mediated auxin transport to inhibit root elongation and gravitropic responses in Arabidopsis. Plant Cell Physiol. 61 (3), 519–535. doi: 10.1093/pcp/pcz217
Li, G., Song, H., Li, B., Kronzucker, H. J., Shi, W. (2015). Auxin Resistant1 and PINFORMED2 protect lateral root formation in Arabidopsis under iron stress. Plant Physiol. 169 (4), 2608–2623. doi: 10.1104/pp.15.00904
Liu, H. G., Wang, Y. J., Hart, M., Chen, H., Tang, M. (2016). Arbuscular mycorrhizal symbiosis regulates hormone and osmotic equilibrium of Lycium barbarum l. under salt stress. Mycosphere 7 (6), 828–843. doi: 10.5943/mycosphere/7/6/14
Livak, K. J., Schmittgen, T. D. (2001). Analysis of relative gene expression data using real-time quantitative PCR and the 2-ΔΔCT method. Methods 25, 402–408. doi: 10.1006/meth.2001.1262
Li, S., Zhou, X., Huang, Y., Zhu, L., Zhang, S., Zhao, Y., et al. (2013). Identification and characterization of the zinc-regulated transporters, iron-regulated transporter-like protein (ZIP) gene family in maize. BMC Plant Biol. 13, 114. doi: 10.1186/1471-2229-13-114
López-Ruiz, B. A., Zluhan-Martínez, E., Sanchez, M. P., Alvarez-Buylla, E. R., GarayArroyo, A. (2020). Interplay between hormones and several abiotic stress conditions on Arabidopsis thaliana primary root development. Cells 9 (12), 2576. doi: 10.3390/cells9122576
Maiti, S. K., Rana, V. (2017). Assessment of heavy metals contamination in reclaimed mine soil and their accumulation and distribution in Eucalyptus hybrid. Bull. Environ. Contam. Toxicol. 98, 97–104. doi: 10.1007/s00128-016-1966-5
Malheiros, R. S., Costa, L. C., Avila, R. T., Pimenta, T. M., Teixeira, L. S., Brito, F. A., et al. (2019). Selenium downregulates auxin and ethylene biosynthesis in rice seedlings to modify primary metabolism and root architecture. Planta 250, 333–345. doi: 10.1007/s00425-019-03175-6
Maret, W. (2009). Molecular mspects of human cellular zinc homeostasis: redox control of zinc potentials and zinc signals. Biometals 22, 149–157. doi: 10.1007/s10534-008-9186-z
Migocka, M., Małas, K., Maciaszczyk-Dziubinska, E., Posyniak, E., Migdal, I., Szczech, P. (2018). Cucumber golgi protein CsMTP5 forms a zn-transporting heterodimer with high molecular mass protein CsMTP12. Plant Sci. 277, 196–206. doi: 10.1016/j.plantsci.2018.09.011
Montanini, B., Blaudez, D., Jeandroz, S., Sanders, D., Chalot, M. (2007). Phylogenetic and functional analysis of the cation diffusion facilitator (CDF) family: improved signature and prediction of substrate specificity. BMC Genomics 8, 107. doi: 10.1186/1471-2164-8-107
Myburg, A. A., Grattapaglia, D., Tuskan, G. A., Hellsten, U., Hayes, R. D., Grimwood, J., et al. (2014). The genome of Eucalyptus grandis. Nature 510, 356–362. doi: 10.1038/nature13308
Neeraja, C. N., Kulkarni, K. S., Babu, P. M., Rao, D. S., Surekha, K., Babu, V. R. (2018). Transporter genes identified in landraces associated with high zinc in polished rice through panicle transcriptome for biofortification. PloS One 13 (2), e0192362. doi: 10.1371/journal.pone.0192362
Nuria, F., Tamayo, E., Vargas, P. (2016). The heavy metal paradox in arbuscular mycorrhizas: from mechanisms to biotechnological applications. J. Exp. Bot. 67, 6253–6265. doi: 10.1093/jxb/erw403
Qi, D. Z., Wang, L. M., Liang, M. X., Zhang, Q., Tang, X. L., Geng, B., et al. (2022). Genome-wide analyses of metal tolerance protein genes in apple (Malus domestica): Identification, characterization, expression and response to various metal ion stresses. Environ. Exp. Bot. 201, 104948. doi: 10.1016/j.envexpbot.2022.104948
Riaz, M., Kamran, M., Fang, Y. Z., Wang, Q. Q., Cao, H. Y., Yang, G. L., et al. (2021). Arbuscular mycorrhizal fungi-induced mitigation of heavy metal phytotoxicity in metal contaminated soils: A critical review. J. Hazard Mater. 402, 123919. doi: 10.1016/j.jhazmat.2020.123919
Sinclair, S. A., Krämer, U. (2012). The zinc homeostasis network of land plants. BBA-Mol. Cell Res. 1823, 1553–1567. doi: 10.1016/j.bbamcr.2012.05.016
Sinclair, S. A., Senger, T., Talke, I. N., Cobbett, C. S., Haydon, M. J., Krämer, U. (2018). Systemic upregulation of MTP2- and HMA2-mediated zn partitioning to the shoot supplements local zn deficiency responses. Plant Cell 30 (10), 2463–2479. doi: 10.1105/tpc.18.00207
Singh, N. V., Saminathan, T., Chandra, R., Awachare, C., Babu, D. K., Mundewadikar, D. M., et al. (2015). RNA Isolation from high polyphenol containing tissues of pomegranate. Indian J. Hortic. 72 (2), 273–277. doi: 10.5958/0974-0112.2015.00051.1
Song, Y., Zhou, L., Yang, S., Wang, C., Zhang, T., Wang, J. (2017). Dose-dependent sensitivity of Arabidopsis thaliana seedling root to copper is regulated by auxin homeostasis. Environ. Exp. Bot. 139, 23–30. doi: 10.1016/j.envexpbot.2017.04.003
Stephens, B. W., Cook, D. R., Grusa, M. A. (2011). Characterization of zinc transport by divalent metal transporters of the ZIP family from the model legume Medicago truncatula. Biometals 24, 51–58. doi: 10.1007/s10534-010-9373-6
Tedersoo, L., Bahram, M., Zobel, M. (2020). How mycorrhizal associations drive plant population and community. Science 367 (6480), eaba1223. doi: 10.1126/science.aba1223
Vassilev, A., Nikolova, A., Koleva, L., Lidon, F. (2011). Effects of excess zn on growth and photosynthetic performance of young bean plants. J. Phytol. 3 (6), 58–62. doi: 10.1111/plb.13307
Vogel-Mikuš, K., Pongrac, P., Kump, P., Nečemer, M., Regvar, M. (2006). Colonisation of a zn, cd and Pb hyperaccumulator Thlaspi praecox wulfen with indigenous arbuscular mycorrhizal fungal mixture induces changes in heavy metal and nutrient uptake. Environ. pollut. 139, 362–371. doi: 10.1016/j.envpol.2005.05.005
Wang, X. Y., Liang, J. W., Liu, Z. Y., Kuang, Y. X., Han, L. N., Chen, H., et al. (2022). Transcriptional regulation of metal metabolism- and nutrient absorption-related genes in Eucalyptus grandis by arbuscular mycorrhizal fungi at different zinc concentrations. BMC Plant Biol. 22, 76. doi: 10.1186/s12870-022-03456-5
Wang, J. H., Moeen-ud-din, M., Yang, S. H. (2021). Dose-dependent responses of Arabidopsis thaliana to zinc are mediated by auxin homeostasis and transport. Environ. Exp. Bot. 189, 104554. doi: 10.1016/j.envexpbot.2021.104554
Wang, W. X., Shi, J. C., Xie, Q. J., Jiang, Y. N., Yu, N., Wang, E. T. (2017). Nutrient exchange and regulation in arbuscular mycorrhizal symbiosis. Mol. Plant 10, 1147–1158. doi: 10.1016/j.molp.2017.07.012
Wang, C., Song, H. Z., Pei, F. W., Hou, J., Wen, J. Z., Li, W., et al. (2009). The effect of excess zn on mineral nutrition and antioxidative response in rapeseed seedlings. Chemosphere 75 (11), 1468–1476. doi: 10.1016/j.chemosphere.2009.02.033
Wang, Y., Zhang, W. Z., Liu, W. K., Ahammed, G. J., Wen, W. X., Guo, S. R., et al. (2021). Auxin is involved in arbuscular mycorrhizal fungi-promoted tomato growth and NADP-malic enzymes expression in continuous cropping substrates. BMC Plant Biol. 21, 48. doi: 10.1186/s12870-020-02817-2
Wu, D., Shen, H., Yokawa, K., Baluska, F. (2014). Alleviation of aluminium-induced cell rigidity by overexpression of OsPIN2 in rice roots. J. Exp. Bot. 65 (18), 5305–5315. doi: 10.1093/jxb/eru292
Xie, X. A., Fan, X. N., Chen, H., Tang, M. (2021). Phosphorus starvation-and zinc excess-induced Astragalus sinicus Aszip2 zinc transporter is suppressed by arbuscular mycorrhizal symbiosis. J. Fungi 7, 829. doi: 10.3390/jof7110892
Xie, X. A., Hu, W. T., Fan, X. N., Chen, H., Tang, M. (2019). Interactions between phosphorus, zinc, and iron homeostasis in nonmycorrhizal and mycorrhizal plants. Front. Plant Sci. 10. doi: 10.3389/fpls.2019.01172
Xie, X. A., Lai, W. Z., Che, X. R., Wang, S. J., Ren, Y., Hu, W. T., et al. (2022). A SPX domain-containing phosphate transporter from Rhizophagus irregularis handles phosphate homeostasis at symbiotic interface of arbuscular mycorrhizas. New Phytol. 234 (2), 650–671. doi: 10.1111/nph.17973
Xie, K., Ren, Y., Chen, A. Q., Yang, C. F., Zheng, Q. S., Chen, J., et al. (2021). Plant nitrogen nutrition: The roles of arbuscular mycorrhizal fungi. J. Plant Physiol. 269, 153591. doi: 10.1016/j.jplph.2021.153591
Yang, Y. R., He, C. J., Huang, L., Ban, Y. H., Tang, M. (2017). The effects of arbuscular mycorrhizal fungi on glomalin-related soil protein distribution, aggregate stability and their relationships with soil properties at different soil depths in lead-zinc contaminated area. PloS One 12 (8), e0182264. doi: 10.1371/journal.pone.0182264
Zhang, P., Sun, L. L., Qin, J., Wan, J. P., Wang, R. L., Li, S., et al. (2018). cGMP is involved in zn tolerance through the modulation of auxin redistribution in root tips. Environ. Exp. Bot. 147, 22–30. doi: 10.1016/j.envexpbot.2017.10.025
Keywords: Eucalyptus grandis, auxin biosynthesis–related genes, arbuscular mycorrhiza, metal tolerance protein, nutrient uptake, zinc stress
Citation: Han L-N, Wang S-J, Chen H, Ren Y, Xie X-A, Wang X-Y, Hu W-T and Tang M (2022) Arbuscular mycorrhiza mitigates zinc stress on Eucalyptus grandis through regulating metal tolerance protein gene expression and ionome uptake. Front. Plant Sci. 13:1022696. doi: 10.3389/fpls.2022.1022696
Received: 18 August 2022; Accepted: 17 October 2022;
Published: 07 November 2022.
Edited by:
Diaa Abd El Moneim, Arish University, EgyptReviewed by:
Boutasknit Abderrahim, Cadi Ayyad University, MoroccoMohammad Etemadi, Shiraz University, Iran
Copyright © 2022 Han, Wang, Chen, Ren, Xie, Wang, Hu and Tang. This is an open-access article distributed under the terms of the Creative Commons Attribution License (CC BY). The use, distribution or reproduction in other forums is permitted, provided the original author(s) and the copyright owner(s) are credited and that the original publication in this journal is cited, in accordance with accepted academic practice. No use, distribution or reproduction is permitted which does not comply with these terms.
*Correspondence: Ming Tang, dGFuZ21pbmd5bEAxNjMuY29t