- 1College of Land and Environment, National Engineering Research Center for Efficient Utilization of Soil and Fertilizer Resources, Northeast China Plant Nutrition and Fertilization Scientific Observation and Research Center for Ministry of Agriculture and Rural Affairs, Key Laboratory of Protected Horticulture of Education Ministry and Liaoning Province, Shenyang Agricultural University, Shenyang, China
- 2National Sorghum Improvement Center, Liaoning Academy of Agricultural Sciences, Shenyang, China
- 3The University of Western Australia (UWA) Institute of Agriculture, The University of Western Australia, Perth, WA, Australia
- 4School of Biological Sciences, The University of Western Australia, Perth, WA, Australia
- 5School of Agriculture and Environment, The University of Western Australia, Perth, WA, Australia
- 6Department of Biosystems and Technology, Swedish University of Agricultural Sciences, Alnarp, Sweden
Calcium ions (Ca2+) regulate plant growth and development during exposure to multiple biotic and abiotic stresses as the second signaling messenger in cells. The extracellular calcium-sensing receptor (CAS) is a specific protein spatially located on the thylakoid membrane. It regulates the intracellular Ca2+ responses by sensing changes in extracellular Ca2+ concentration, thereby affecting a series of downstream signal transduction processes and making plants more resilient to respond to stresses. Here, we summarized the discovery process, structure, and location of CAS in plants and the effects of Ca2+ and CAS on stomatal functionality, photosynthesis, and various environmental adaptations. Under changing environmental conditions and global climate, our study enhances the mechanistic understanding of calcium-sensing receptors in sustaining photosynthesis and mediating abiotic stress responses in plants. A better understanding of the fundamental mechanisms of Ca2+ and CAS in regulating stress responses in plants may provide novel mitigation strategies for improving crop yield in a world facing more extreme climate-changed linked weather events with multiple stresses during cultivation.
Introduction
Calcium, a crucial macronutrient for plants, has important physiological functions such as maintaining cell morphology and regulating ion balance and osmotic pressure. Interestingly, it is also a key component for several early signaling pathways involved in plant–environmental stresses interactions (Lambers and Oliveira, 2019; Song et al., 2020; Wu et al., 2020). Most elemental calcium in plant cells exists in ionic forms, which contribute to forming microtubules in the cytoskeleton and maintaining the fluidity of cell membranes (Kong et al., 2020; Liu, 2021). In addition, calcium signals assist in the integration of various signaling pathways to coordinate plants’ growth response to multiple environmental signals (Edel and Kudla, 2016; Kudla et al., 2018; Luan and Wang, 2021; Kim et al., 2022). The extracellular calcium-sensing receptor (CAS) is an important protein that specifically exists in plants and has specific regulatory functions. These proteins regulate intracellular Ca2+ responses by sensing changes in extracellular Ca2+ concentration, thereby affecting a series of downstream signaling activities and fine-tuning plants’ responses to environmental perturbations. We summarized the research progress of Ca2+ and CAS in regulating photosynthesis and stress responses. Here, we focused on the fundamental role of CAS in regulating growth and signal transduction in response to the external environment.
Structural characteristics of the calcium-sensing receptor
Discovery and location of CAS
The extracellular Ca2+ receptor gene was cloned for the first time using the functional gene screening method in Arabidopsis thaliana and named as the calcium-sensing receptor (CAS) (Li et al., 2022). With the aid of fluorescence imaging and proteomics technologies, the CAS was located on the chloroplast thylakoid membrane of Arabidopsis thaliana, spinach, and green algae (Allmer et al., 2006; Naumann et al., 2007; Nomura et al., 2008; Weinl et al., 2008). Interestingly, the CAS was also detected within the purified eyespot of green algae (Trippens et al., 2017). Yamano et al. (2018) fused the CAS-Clover with high-resolution fluorescence imaging to coincide with the eyespot, which also verified the existence of CAS. In addition, high-resolution fluorescence images of CAS were obtained using the sensitive hybrid detector and image deconvolution technology, and its cellular localization on the thylakoid membrane was authenticated and observed with greater clarity.
Structural features of CAS
The carboxyl-terminal of CAS is located within the cell and has two domains (Vainonen et al., 2008); one of which is a non-catalytic thiocyanate domain with high homology, and the other is related to protein interaction which can interact with the 14-3-3 and FHA (forkhead-associated) domain. Under light conditions, the CAS light-regulated kinase STN8 is targeted for phosphorylation at Thr-380 (Vainonen et al., 2008). The amino terminal of CAS is located outside the cell membrane, which may plausibly be the Ca2+ binding region, although its conserved sequence has not been found. It was later verified that the binding site of Ca2+ in CAS was the amino end rather than the carboxyl end in Arabidopsis and Chlamydomonas (Wang et al., 2016a).
CAS and stomatal functionality
The pair of guard cells have a fundamental role in regulating gas exchange between plants and the atmosphere, and biotic infection (Melotto et al., 2008; Chen et al., 2012; Sawinski et al., 2013; Sun et al., 2014; Murata et al., 2015; Agurla et al., 2016; Bharath et al., 2021). Generally, Ca2+ plays an important role in regulating guard cell turgor and the movements of guard cells. Ca2+ ions affect stomatal conductance to water vapor and CO2 by regulating the pore size. Peng et al. (2022) discovered that an exogenous application of 18 Mm Ca2+ could increase the stomatal conductance of Paris polyphylla under high temperatures and strong light conditions. The CO2-induced stomatal closure process is also calcium-dependent and the CPK (calcium-dependent protein kinase) has a role in signal transduction and regulating the ion channels of guard cells. In the pentaploid mutant plants with cpk3/5/6/11/23, the stomatal movements were significantly impaired, suggesting that CPK has important functions in facilitating stomatal movement and is plausibly regulated by CO2 concentration (Schulze et al., 2021). Jakobson et al. (2016) confirmed that there were significant differences in stomatal phenotypes among cas mutants with different alleles and the cas overexpression of Arabidopsis transformants promoted stomatal closure. Li et al. (2017) also demonstrated that the deletion of the CAS gene could disrupt stomatal closure and this phenomenon was attributed to high levels of extracellular Ca2+. It was later verified that the extracellular high Ca2+ in CAS gene-deficient mutants could not induce stomatal closure, indicating that CAS was essential for extracellular Ca2+-induced stomatal closure in Arabidopsis (Qi et al., 2018).
Interestingly, it was also found that CAS has an important role in elevating intracellular Ca2+ concentration when induced by extracellular Ca2+. These studies provided evidence for Arabidopsis chloroplasts in regulating extracellular Ca2+-induced intracellular Ca2+ concentration increases and subsequent stomatal closure (Nomura et al., 2008; Li et al., 2022). Wang et al. (2016b) found that the chloroplastic regulation of stomatal closure required the reduction of quinone, foliar production of H2O2, and the phosphorylation of CAS and LHCII during exposure to unfavorable perturbation. It was confirmed that stomatal movement was associated with NO and the NO acted downstream of the H2O2 step in regulating stomatal movement (Bright et al., 2006). Specifically, the stomatal closure induced by H2O2 and NO requires the involvement of extracellular Ca2+ and the accumulation of H2O2 and NO in guard cells (Li et al., 2009; Zhao et al., 2011). Tang et al. (2007) suggested that CAS might regulate the stomatal closure process through the Ca2+-CAS-IP signaling pathway. Subsequently, Wang et al. (2012) found that the extracellular Ca2+ induced H2O2 and NO accumulation through CAS in Arabidopsis thaliana, which induced transient changes in cytoplasmic Ca2+ concentration and was able to regulate stomatal movement. In addition, Arabidopsis plants expressing PHPLCδ-GFP protein were used for PHPLCδ-GFP imaging of guard cells, which indicated that H2O2 and NO were involved in the CAS-IP3 signaling pathway (Wang, 2013). Li et al. (2017) found that H2O2 might directly or indirectly regulate CAS activity and serve as redox signaling molecules, thereby regulating stomatal closure. Interestingly, the data also revealed that the exogenous NO-induced stomatal closure of CAS guard cells was facilitated by exogenous NO bypassing the damaged CAS. Furthermore, it was indicative that NO has a downstream role relative to CAS in the signal transduction pathway. Recent studies suggested that stomatal movement induced by extracellular Ca2+ was the culmination of multiple interactions among CAS, H2O2, and NO signals (Zhang et al., 2018; Li et al., 2022). Li et al. (2017) used multi-disciplinary approaches to examine the responses of different mutants to extracellular Ca2+ in guard cells. It was found that there was a cascade of H2O2, ABA, and NO in the CAS signaling pathway. With further studies, it was confirmed that seed germination and stomatal closure of mutant cau1 transformants were excessively sensitive to ABA. In addition to extracellular Ca2+, the CAU1-CAS could also respond to ABA through interaction, and ABA and CAUI could also regulate stomatal closure (Ueda and Seki, 2020), although the specific role of CAS in this process required further clarification. Li et al. (2017) found that ABA in CASas mutant plants was able to induce stomatal closure, indicating that the ABA-induced signal transduction pathway was not dependent on CAS; here, CASas refers to the CAS antisense line of Arabidopsis. Based on these studies, the signal transduction cascade is summarized accordingly in a scheme (Figure 1).
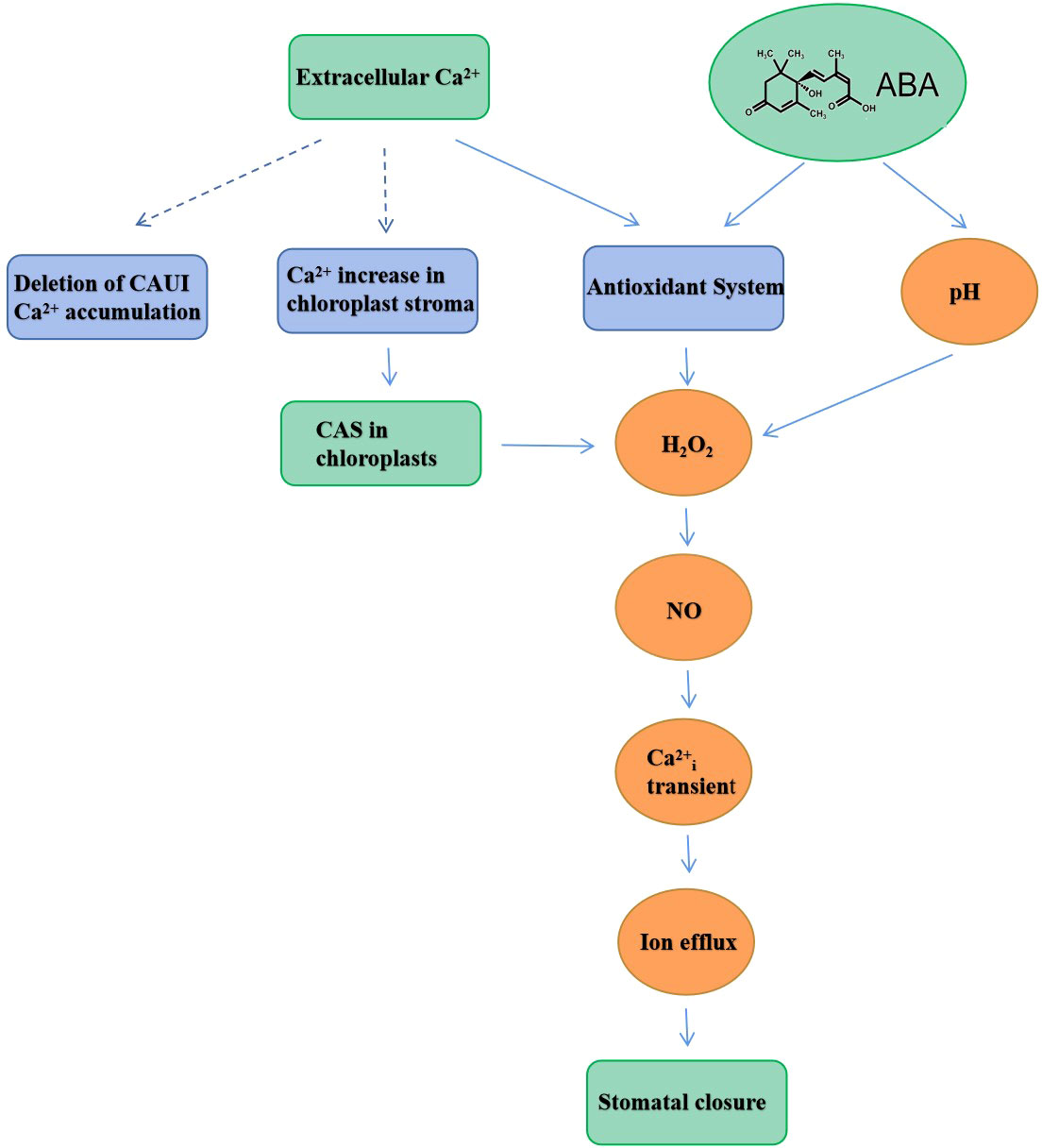
Figure 1 A simplified scheme illustrating the salient signal transduction steps leading to stomatal closure.
CAS and photosynthesis
Photosynthesis is one of the most important biological processes on earth (Liu et al., 2011; Stael et al., 2012; Lambers and Oliveira, 2019). Calcium is involved in regulating various photosynthetic processes, such as CO2 fixation and protein phosphorylation in chloroplasts (Rocha and Vothknecht, 2012; Liu, 2020). Hu et al. (2022) found that low calcium levels caused the decline in photosynthesis of Taxus wallichiana varieties, but this negative effect could be reversed by applying supplementary calcium nutrition, which was consistent with previous findings in grape and cucumber (He et al., 2018; Duan et al., 2020). Harnessing the pull-down analysis (network database GeneCAT) combined with proteomics and gene co-expression method, Wang (2013) found that 52% of the CAS co-expression genes in Arabidopsis were related to photosynthesis. Combining photosynthetic indexes, chloroplast structural observation and gene expression profiling, these observations verified that the photosynthetic level and biomass accumulation of Arabidopsis CASas plants were significantly reduced. Specifically, the inhibition of CAS caused the transcription level of photosynthetic electron-related genes to be down-regulated (Wang et al., 2014). Navazio et al. (2020) found that the transcription expression of the CAS gene in Arabidopsis was up-regulated under strong light conditions, and the CAS transcription level under dark and weak light conditions was also significantly up-regulated compared with that under normal light conditions. Petroutsos et al. (2011) confirmed that CAS played a role in maintaining PSII functionality and helping plants to adapt to strong light. Additionally, Li (2021) found three AtCAS homologous genes in some shade plants such as ginseng, and two AtCAS homologous genes in the non-shade plant such as tomato. As CAS is a phosphorylated protein regulated by light intensity; it is plausible that CAS may be closely related to light adaptability. The stomatal movement of terrestrial plants regulates CO2 entry and exit through HTI protein kinase and carbonic anhydrase (Hu et al., 2010), and aquatic plants maintained stable photosynthesis through the CO2-concentration mechanism (CCM). Studies have indicated that Ca2+ and CAS are related to CCM. In response to low CO2 concentration, the CAS can be transferred to the protein nucleus, and then mediate the chloroplastic retrograde signaling to maintain the expression of HLA3 (high-light activated 3) and LCIA (low-CO2-inducible gene A) (Wang et al., 2016a). Yamano et al. (2018) obtained interesting CAS-Clover high-resolution fluorescence images using a sensitive mixed detector coupled with image deconvolution technology. It was found that CAS moved along the thylakoid membrane in response to low concentration CO2, and gathered spatially in the protein nucleus during the CCM mechanism. As a chloroplastic thylakoid membrane protein, CAS functionality was examined during de-etiolation and chloroplast development in Arabidopsis thaliana (Huang et al., 2012). The thylakoid-localized CAS protein could assist in the production of cytosolic calcium transients, and the activation of the MPK3/MPK6 signal system. Subsequently, the activated MPK3/MPK6 was involved in the nucleus-induced phosphorylation of ABI4. The activation of ABI4 at both the transcriptional and posttranslational levels induced the inhibition of LHCB (Gao et al., 2016). These results showed that the cystoid-located CAS was involved in the retrograde signaling pathway from chloroplast to nucleus (Li et al., 2022).
Protein phosphorylation is vital for photosynthesis and adaptation to multiple environmental stresses (Vener, 2007; Fristedt et al., 2010). Vainonen et al. (2008) found that CAS could be phosphorylated by STN8, and the phosphorylation intensity increased with light intensity enhancement. At the same time, a new phosphorylation site Thr-367 was also found in the CAS of green algae (Lemeille et al., 2010). Cutolo et al. (2019) conducted proteomics analysis and phosphorylation determination of CASas, and identified Thr-376, Ser-378, and Thr-380 as the main phosphorylation sites of STN kinase. Protein phosphorylation is also important to regulate photosynthetic electron transport efficiency (Reiland et al., 2011). The cyclic electron transport (CET) process is involved in chloroplast energy regulation and redox metabolism, which is particularly important for photosynthesis. The studies demonstrated that CET has two pathways, PGR5/PGRL1-mediated pathway and the NDH-mediated pathway (Johnson et al., 2014; Ma et al., 2021a). The protein interaction between CAS and PGRL1 was confirmed in Chlamydomonas reinhardtii, indicating that there is a link between CAS and CET (Terashima et al., 2012; Ma et al., 2021b). Chen et al. (2015) found that Ca2+ controlled the biosynthesis of lipids induced by chloroplastic nitrogen starvation by promoting the expression of CAS in the PGRL1-mediated CET pathway.
CAS, stress resilience, and plant defense
When plants are exposed to environmental stresses, they will improve their resilience by self-regulating several in vivo processes (e.g. changes in intracellular calcium concentration) to cope with these perturbations (Liu, 2020). Salinity is an important environmental factor, which has a negative impact on plant growth and photosynthesis (Acosta-Motos et al., 2017; Ma et al., 2022). Similarly, Ca2+ is also crucial in mediating stress responses (Park et al., 2016). It was verified that Ca2+ can enhance the salt tolerance of plants by improving water balance, regulating sodium secretion, and enhancing membrane integrity (Ahmed et al., 2021). In addition, Ca2+ also enhances resilience to chilling stress. Liu et al. (2013) found that exogenous Ca2+ could mitigate the significant decline in peanut photosynthesis and biomass accumulation during exposures to low night temperatures. It was also confirmed that exogenous Ca2+ ameliorated night chilling-dependent feedback inhibition of photosynthesis by improving sink demand and facilitating nonstructural carbohydrate export from chloroplasts, to restore peanut growth, dry matter production, and leaf photosynthetic capacity (Shi et al., 2020; Song et al., 2020; Wu et al., 2020). Sun et al. (2022) reported that the Ca2+ mediated-CAS was crucial for alleviating photoinhibition in peanut growing under conditions of moderate phosphorus deficiency. In addition, Zhao et al. (2015) confirmed that OsCAS could improve drought tolerance in Arabidopsis. When drought and heat stresses were imposed on the creeping bentgrass (Agrostis stolonifera), the expression of CAS was down-regulated, indicating that the calcium sensitivity to drought and heat stress responses was reduced (Xu and Huang, 2018). The molecular mechanism of proline metabolism regulation under drought also revealed a new drought tolerance pathway mediated by CAU1 (Fu et al., 2018). Huang et al. (2012) provided further evidence to support the role of Ca2+ and CAS in leaf re-greening and chloroplast development of Arabidopsis. Zheng et al. (2017) screened a chloroplast protein QUA1 by harnessing the large-scale forward genetic method. It is plausible that the CAS-mediated salt and drought tolerance of plants through the calcium signaling-linked QUA1 that influence the stability of CAS. It was further suggested that CAS has a regulatory role in the downstream of QUA1. Interestingly, there are some pieces of evidence to indicate that CAS is a prerequisite for chloroplast Ca2+ to induce light response and light-dark transition (Nomura et al., 2012). The expression of cas in Arabidopsis thaliana was significantly reduced under high-temperature treatment. It is plausible that the heat-sensing ability of chloroplasts was partially dependent on CAS during high-temperature exposure (Lenzoni and Knight, 2019).
Plant defense against invasive pathogens is dependent on the dual innate immune system. Meta-analysis indicated that CAS regulated the expression of flg22-induced immune genes through 1O2-mediated retrograde signaling; flg22 is a peptide derived from bacterial flagellins. These studies revealed that the weakened signals, chloroplasts, and superoxide anions were collectively involved in the innate immune system (Sano et al., 2014; Stael et al., 2015). In the study of plant-pathogen interaction mediated by small molecules during the invasion of Fusarium graminearum, Jia et al. (2019) found that the fungal infection or the use of exogenous fusaoctaxin A inhibited the expression of three CAS-like genes and chloroplast genes. Thus, the lowered susceptibility of wheat to Fusarium A, and the inhibition of photosynthesis provided some evidence that chloroplasts might play a key role in regulating early immune responses (de Torres Zabala et al., 2015). Sclerotinia sclerotiorum is a well-known necrotizing fungus that attacks many crops (Bolton et al., 2006). The CAS is involved in the defense response of Arabidopsis thaliana to S. sclerotiorum, and actively regulates the accumulation of salicylic acid (SA) by promoting the expression of SA biosynthesis-related genes, thereby enhancing the resistance of plants to S. sclerotiorum (Tang et al., 2020). HopAU1 is an immune inducer existing in Pseudomonas syringae pv. actinidiae (Psa) which can interact with NbCAS in tobacco. Thus, silencing the NbCAS by RNAi in N. benthamiana greatly attenuated HopAU1-triggered cell death, suggesting that HopAU1 targets CAS and enhances plant immunity. Further study showed that the overexpression of NbCaS in N. benthamiana significantly improved plant resistance against Sclerotinia sclerotiorum and Phytophthora capsici (Zhang et al., 2022). Taken together, the CAS also serves as a promising resistant-related gene for breeding new disease-resistant varieties.
Future outlook
The CAS has an important role in facilitating Ca2+-mediated stomatal closure and is associated with several signaling molecules. As a specific protein located on the chloroplast thylakoid membrane, the CAS functions as the first messenger along the signal transduction pathway in plants. There is some evidence that CAS may participate in various photosynthetic processes such as CO2 fixation and protein phosphorylation. With further research, we could gain insights into the Ca2+-induced signaling pathway and the functional characteristics of CAS in plant cells. In the future, it is important to further explore the following: (i) the various relationships between CAS and the other calcium-linked signals; (ii) the potential cross-talk between CAS and light signaling at the chloroplast thylakoid membranes; (iii) the CAS and its specific role in facilitating plant abiotic and biotic stress responses using advanced techniques/tools (proteomics, transcriptomics, and bioinformatics, etc.); (iv) extrapolating the understanding of CAS in model plants to non-model plants such as the legumes and horticultural crops, etc.
Author contributions
YL, RB, CB, XH, and JY are responsible for the general review described in the manuscript. YL, XH, and JY provided further discussion and edited the manuscript. All authors reviewed and approved the final version of the submitted manuscript.
Funding
This research was funded by the National Natural Science Foundation of China (project nos. 31772391 and 31301842), ARC-Linkage Project (project no. LP200100341), and the Scientific Research Project of the Education Department of Liaoning Province, China (project no. LJKZ0661).
Acknowledgments
We sincerely thank the reviewers for all their valuable comments and recommendations to improve the manuscript.
Conflict of interest
The authors declare that the research was conducted in the absence of any commercial or financial relationships that could be construed as a potential conflict of interest.
Publisher’s note
All claims expressed in this article are solely those of the authors and do not necessarily represent those of their affiliated organizations, or those of the publisher, the editors and the reviewers. Any product that may be evaluated in this article, or claim that may be made by its manufacturer, is not guaranteed or endorsed by the publisher.
References
Acosta-Motos, J., Ortu-no, , Bernal-Vicente, M., Diaz-Vivancos, A. P., Sanchez-Blanco, M., Hernandez, J., et al (2017). Plant responses to salt stress: Adaptive mechanisms. Agronomy 7 (18), 1–38. doi: 10.3390/agronomy7010018
Agurla, S., Gayatri, G., Raghavendra, A. S. (2016). Nitric oxide (NO) measurements in stomatal guard cells. Method. Mol. Biol. 1424, 49–56. doi: 10.1007/978-1-4939-3600-7_5
Ahmed, M. Z., Hussain, T., Gulzar, S., Adnan, M. Y., Khan, M. A. (2021). Calcium improves the leaf physiology of salt treated Limonium stocksii: A floriculture crop. Sci. Hortic. 285 (3), 110190. doi: 10.1016/j.scienta.2021.110190
Allmer, J., Naumann, B., Markert, C., Zhang, M., Hippler, M. (2006). Mass spectrometric genomic data mining: Novel insights into bioenergetic pathways in Chlamydomonas reinhardtii. Proteomics 6 (23), 6207–6220. doi: 10.1002/pmic.200600208
Bharath, P., Gahir, S., Raghavendra, A. S. (2021). Abscisic acid-induced stomatal closure: An important component of plant defense against abiotic and biotic stress. Front. Plant Sci. 12. doi: 10.3389/fpls.2021.615114
Bolton, M. D., Thomma, B., Nelson, B. D. (2006). Sclerotinia sclerotiorum (lib.) de bary: biology and molecular traits of a cosmopolitanpathogen. Mol. Plant Pathol. 7 (1), 1–16. doi: 10.1111/j.1364-3703.2005.00316.x
Bright, J., Desikan, R., Hancock, J. T., Weir, I. S., Neill, S. J. (2006). ABA-induced no generation and stomatal closure in Arabidopsis are dependent on H2O2 synthesis. Plant J. 45 (1), 113–122. doi: 10.1111/j.1365–313X.2005.02615.x
Chen, H., Hu, J., Qiao, Y., Chen, W., Rong, J., Zhang, Y., et al. (2015). Ca2+-regulated cyclic electron flow supplies ATP for nitrogen starvation-induced lipid biosynthesis in green alga. Sci. Rep. 5, 15117. doi: 10.1038/srep15117
Chen, D. H., Wang, M., Wang, H. G., Zhang, W. (2012). A type of voltage-dependent Ca2+ channel on Vicia faba guard cell plasma membrane outwardly permeates k+. Protoplasma 249 (3), 699–708. doi: 10.1007/s00709-011-0313-2
Cutolo, E., Parvin, N., Ruge, H., Pirayesh, N., Vothknecht, U. C. (2019). The high light response in Arabidopsis requires the calcium sensor protein CAS, a target of STN7- and STN8-mediated phosphorylation. Front. Plant Sci. 10. doi: 10.3389/fpls.2019.00974
de Torres Zabala, M., Littlejohn, G., Jayaraman, S., Studholme, D., Bailey, T., Lawson, T., et al. (2015). Chloroplasts play a central role in plant defense and are targeted by pathogen effectors. Nat. Plants. 1, 15074–15074. doi: 10.1038/nplants.2015.74
Duan, S. Y., Wu, Y.S., Zhang, C.J., Wang Song, L. S. R., Ma, C. (2020). Differential regulation of enzyme activities and physio-anatomical aspects of calcium nutrition in grapevine. Sci. Hort. 272, 109423. doi: 10.1016/j.scienta.2020.109423
Edel, K. H., Kudla, J. (2016). Integration of calcium and ABA signaling. Curr. Opin. Plant Biol. 33, 83–91. doi: 10.1016/j.pbi.2016.06.010
Fristedt, R., Granath, P., Vener, A. V. (2010). A protein phosphorylation threshold for functional stacking of plant photosynthetic membranes. PloS One 5 (6), e10963. doi: 10.1371/journal.pone.0010963
Fu, Y., Ma, H., Chen, S., Gu, T., Gong, J. (2018). Control of proline accumulation under drought via a novel pathway comprising the histone methylase CAU1 and the transcription factor ANAC055. J. Exp. Bot. 69 (3), 579–588. doi: 10.1093/jxb/erx419
Gao, G. L., Zhang, X. Y., Chang, Z. Q., Yu, T. F., Zhao, H. (2016). Environmental response simulation and the up-scaling of plant stomatal conductance. Acta Ecol. Sinica. 36 (06), 1491–1500. doi: 10.5846/stxb201408211652
He, L. Z., Yu, L., Li, B., Du, N. S., Guo, S. R. (2018). The effect of exogenous calcium on cucumber fruit quality, photosynthesis, chlorophyll fluorescence, and fast chlorophyll fluorescence during the fruiting period under hypoxic stress. BMC Plant Biol. 18, 180–190. doi: 10.1186/s12870-018-1393-3
Huang, S. S., Chen, J., Dong, X. J., Patton, J., Pei, Z. M., Zheng, H. L. (2012). Calcium and calcium receptor CAS promote Arabidopsis thaliana de-etiolation. Physiol. Plant 144 (1), 73–82. doi: 10.1111/j.1399-3054.2011.01523.x
Hu, H., Boisson-Dernier, A., Israelsson-Nordstrom, M., Bohmer, M., Xue, S., Ries, A., et al. (2010). Carbonic anhydrases are upstream regulators of CO2-controlled stomatal movements in guard cells. Nat. Cell Biol. 12(1), 87–93. doi: 10.1038/ncb2009
Hu, W. J., Liu, T. W., Zhu, C. Q., Wu, Q., Chen, L., Lu, H. L., et al (2022). Physiological, proteomic analysis, and calcium-related gene expression reveal taxus wallichiana var. mairei adaptability to acid rain stress under various calcium levels. Front. Plant Sci. 13, 845107. doi: 10.3389/fpls.2022.845107
Jakobson, L., Vaahtera, L., Toldsepp, K., Nuhkat, M., Brosche, M., Wang, C., et al. (2016). Natural variation in Arabidopsis cvi-0 accession reveals an important role of MPK12 in guard cell CO2 signaling. PloS Biol. 14 (12), e2000322. doi: 10.1371/journal.pbio.2000322
Jia, L. J., Tang, H. Y., Wang, W. Q., Yuan, T. L., Wei, W. Q., Pang, B., et al. (2019). A linear nonribosomal octapeptide from Fusarium graminearum facilitates cell-to-cell invasion of wheat. Nat. Commun. 10 (1), 922. doi: 10.1038/s41467-019-08726-9
Johnson, X., Steinbeck, J., Dent, R. M., Takahashi, H., Richaud, P., Ozawa, S., et al. (2014). Proton gradient regulation 5-mediated cyclic electron flow under ATP- or redox-limited conditions: A study of ΔATPase pgr5 and ΔrbcL pgr5 mutants in the green alga Chlamydomonas reinhardtii. Plant Physiol. 165 (1), 438–452. doi: 10.1104/pp.113.233593
Kim, N. H., Jacob, P., Dangl, J. L. (2022). Con-Ca2+-tenating plant immune responses via calcium-permeable cation channels. New Phytol. 234, 813–818. doi: 10.1111/nph.18044
Kong, X. P., Xu, L. H., Jamieson, P. (2020). Plant sense: the rise of calcium channels. Trends Plant Sci. 25, 838–841. doi: 10.1016/j.tplants.2020.06.002
Kudla, J., Becker, D., Grill, E., Hedrich, R., Hippler, M., Kummer, U., et al. (2018). Advances and current challenges in calcium signaling. New Phytol. 2182, 414–431. doi: 10.1111/nph.14966
Lambers, H., Oliveira, R. S. (2019). “Photosynthesis, respiration, and long-distance transport: photosynthesis,” in Plant physiological ecology (Cham: Springer), 111–114.
Lemeille, S., Turkina, M. V., Vener, A. V., Rochaix, J. D. (2010). Stt7-dependent phosphorylation during state transitions in the green alga Chlamydomonas reinhardtii. Mol. Cell. Proteomics. 9 (6), 1281–1295. doi: 10.1074/mcp.M000020-MCP201
Lenzoni, G., Knight, M. R. (2019). Increases in absolute temperature stimulate free calcium concentration elevations in the chloroplast. Plant Cell Physiol. 60 (3), 538–548. doi: 10.1093/pcp/pcy227
Li, H. (2021). Mechanism of the calcium receptor protein (CAS) involved in nitrogen regulation of flowering time in arabidopsis (Jilin, China: Jilin University). doi: 10.27162/d.cnki.gjlin.2021.004485
Li, Q., Wang, Y. J., Liu, C. K., Pei, Z. M., Shi, W. L. (2017). The crosstalk between aba, nitric oxide, hydrogen peroxide, and calcium in stomatal closing of arabidopsis thaliana. Biologia 72, 1140–1146. doi: 10.1515/biolog-2017-0126
Liu, J. (2021). Research progress of calcium Ion on plant growth and development. Mod. Salt Chem. Indust. 48, 137–39. doi: 10.19465/j.cnki.2095-9710.2021.05.062
Li, B., Hou, L. Y., Song, C. G., Wang, Z. B., Xue, Q. Y., Li, Y. Y., et al. (2022). The biological function of calcium-sensing receptor (CAS) and its coupling calcium signaling in plants. Plant Physiol. Biochem. 180, 74–80. doi: 10.1016/j.plaphy.2022.03.032
Li, J. H., Liu, Y. Q., Lu, P., Lin, H. F., Bai, Y., Wang, X. C., et al. (2009). A signaling pathway linking nitric oxide production to heterotrimeric G protein and hydrogen peroxide regulates extracellular calmodulin induction of stomatal closure in Arabidopsis. Plant Physiol. 150 (1), 114–124. doi: 10.1104/pp.109.137067
Liu, Y. F. (2020). Calcium chemical priming might play a significant role in relieving overnight chilling-dependent inhibition of photosynthesis in crops: A review. Bas. Clin. Pharmacol. Toxicol. 126. doi: 10.3389/fpls.2020.607029
Liu, Y. F., Han, X. R., Zhan, X. M., Yang, J. F., Wang, Y. Z., Song, Q. B., et al. (2013). Regulation of calcium on peanut photosynthesis under low night temperature stress. J. Integr. Agric. 12, 2172–2178. doi: 10.1016/S2095-3119(13)60411-6
Liu, Y. F., Qi, H. Y., Bai, C. M., Qi, M. F., Xu, C. Q., Hao, J. H., et al. (2011). Grafting helps improve photosynthesis and carbohydrate metabolism in the leaves of muskmelon. Int. J. Biol. Sci. 7, 1161–1170. doi: 10.7150/ijbs.7.1161
Luan, S., Wang, C. (2021). Calcium signaling mechanisms across kingdoms. Annu. Rev. Cell Dev. Biol. 37, 311–340. doi: 10.1146/annurev-cellbio-120219-035210
Ma, M. Z., Liu, Y. F., Bai, C., Yang, Y. H., Sun, Z. Y., Liu, X. Y., et al. (2021b). The physiological functionality of PGR5/PGRL1-dependent cyclic electron transport in sustaining photosynthesis. Front. Plant Sci. 12. doi: 10.3389/FPLS.2021.702196
Ma, M., Liu, Y., Bai, C., Yong, J. W. H. (2021a). The significance of chloroplast NAD(P)H dehydrogenase complex and its dependent cyclic electron transport in photosynthesis. Front. Plant Sci. 12. doi: 10.3389/fpls.2021.661863
Ma, Z., Zhao, X., He, A., Cao, Y., Han, Q., Lu, Y., et al. (2022). Mycorrhizal symbiosis reprograms ion fluxes and fatty acid metabolism in wild jujube during salt stress. Plant Physiol. 189, kiac239. doi: 10.1093/plphys/kiac239
Melotto, M., Underwood, W., He, S. Y. (2008). Role of stomata in plant innate immunity and foliar bacterial diseases. Annu. Rev. Phytopathol. 46, 101–122. doi: 10.1146/annurev.phyto.121107.104959
Murata, Y., Mori, I. C., Munemasa, S. (2015). Diverse stomatal signaling and the signal integration mechanism. Annu. Rev. Plant Biol. 66 (1), 369–392. doi: 10.1146/annurev-arplant-043014-114707
Naumann, B., Busch, A., Allmer, J., Ostendorf, E., Zeller, M., Kirchhoff, H., et al. (2007). Comparative quantitative proteomics to investigate the remodeling of bioenergetic pathways under iron deficiency in Chlamydomonas reinhardtii. Proteomics 7 (21), 3964–3979. doi: 10.1002/pmic.200700407
Navazio, L., Formentin, E., Cendron, L., Szabò, I. (2020). Chloroplast calcium signaling in the spotlight. Front. Plant Sci. 11. doi: 10.3389/fpls.2020.00186
Nomura, H., Komori, T., Kobori, M., Nakahira, Y., Shiina, T. (2008). Evidence for chloroplast control of external Ca2+-induced cytosolic Ca2+ transients and stomatal closure. Plant J. 53 (6), 988–998. doi: 10.1111/j.1365-313X.2007.03390.x
Nomura, H., Komori, T., Uemura, S., Kanda, Y., Shimotani, K., Nakai, K., et al. (2012). Chloroplast-mediated activation of plant immune signaling in Arabidopsis. Nat. Commun. 3, 926. doi: 10.1038/ncomms1926
Park, H. J., Kim, W. Y., Yun, D. J. (2016). A New Insight of Salt Stress Signaling in Plant. Mol. Cell. 39 (6), 447–459. doi: 10.14348/molcells.2016.0083
Peng, Y., Hu, Z. T., Zhong, F. G., Li, S. H. (2022). Effects of calcium on physiology and photosynthesis of paris polyphylla SM. under high temperature and strong light stress. Pak. J. Bot. 54 (3), 809–816. doi: 10.30848/PJB2022-3(27
Petroutsos, D., Busch, A., Janssen, I., Trompelt, K., Bergner, S. V., Weinl, S., et al. (2011). The chloroplast calcium sensor CAS is required for photoacclimation in Chlamydomonas reinhardtii. Plant Cell. 23 (8), 2950–2963. doi: 10.1105/tpc.111.087973
Qi, J. S., Song, C. P., Wang, B. S., Zhou, J. M., Kangasjarvi, J., Zhu, J. K., et al. (2018). Reactive oxygen species signaling and stomatal movement in plant responses to drought stress and pathogen attack. J. Integr. Plant Biol. 60 (09), 805–826. doi: 10.1111/jipb.12654
Reiland, S., Finazzi, G., Endler, A., Willig, A., Baerenfaller, K., Grossmann, J., et al. (2011). Comparative phosphoproteome profiling reveals a function of the STN8 kinase in fine-tuning of cyclic electron flow (CEF). Proc. Natl. Acad. Sci. U. S. A. 108 (31), 12955–12960. doi: 10.1073/pnas.1104734108
Rocha, A. G., Vothknecht, U. C. (2012). The role of calcium in the chloroplasts-an intriguing and unresolved puzzle. Protoplasma 249 (4), 957–966. doi: 10.1007/s00709-011-0373-3
Sano, S., Aoyama, M., Nakai, K., Shimotani, K., Yamasaki, K., Sato, M. H., et al. (2014). Light-dependent expression of flg22-induced defense genes in Arabidopsis. Front. Plant Sci. 5. doi: 10.3389/fpls.2014.00531
Sawinski, K., Mersmann, S., Robatzek, S., Bohmer, M. (2013). Guarding the green: pathways to stomatal immunity. Mol. Plant-Microbe Interact. 26 (6), 626–632. doi: 10.1094/MPMI-12-12-0288-CR
Schulze, S., Dubeaux, G., Ceciliato, P., Munemasa, S., Nuhkat, M., Yarmolinsky, D., et al (2021). A role for calcium dependent protein kinases in differential CO2‐and ABA‐controlled stomatal closing and low CO2‐induced stomatal opening in Arabidopsis. New Phytol. 229 (5), 2765–2779. doi: 10.1111/nph.17079
Shi, Q. W., Pang, J. Y, Yong, J. W. H., Bai, C. M., Lambers, H. (2020). Phosphorus-fertilisation has differential effects on leaf growth and photosynthetic capacity of Arachis hypogaea L. Plant Soil 447, 99–116. doi: 10.1007/s11104-019-04041-w
Song, Q. B., Liu, Y. F., Pang, J. Y., Yong, J. W. H., Chen, Y. L., Bai, C. M., et al. (2020). Supplementary calcium restores peanut (Arachis hypogaea) growth and photosynthetic capacity under low nocturnal temperature. Front. Plant Sci. 10. doi: 10.3389/fpls.2019.01637
Stael, S., Kmiecik, P., Willems, P., van der Kelen, K., Coll, N. S., Teige, M., et al. (2015). Plant innate immunity-sunny side up? Trends Plant Sci. 20 (1), 3–11. doi: 10.1016/j.tplants.2014.10.002
Stael, S., Rocha, A. G., Wimberger, T., Anrather, D., Vothknecht, U. C., Teige, M. (2012). Cross-talk between calcium signaling and protein phosphorylation at the thylakoid. J. Exp. Bot. 63 (4), 1725–1733. doi: 10.1093/jxb/err403
Sun, Z. Y., Liu, X. Y., Zhang, S. W., Ma, M. Z., Bai, R., Liu, H., et al (2022). Mechanism of Exogenous Calcium Alleviating Sub - low Phosphorus Photosynthesis Obstacle in Peanut. J. Plant Nutri. Fertil. 28 (6), 1055–1066. doi: 10.11674/zwyf.2021514
Sun, Z. Y., Jin, X. F., Albert, R., Assmann, S. M. (2014). Multi-level modeling of light-induced stomatal opening offers new insights into its regulation by drought. PloS Comput. Biol. 10 (11), e1003930. doi: 10.1371/journal.pcbi.1003930
Tang, R. H., Han, S. C., Zheng, H. L., Cook, C. W., Choi, C. S., Woerner, T. E., et al. (2007). Coupling diurnal cytosolic Ca2+ oscillations to the CAS-IP3 pathway in Arabidopsis. Science 315 (5817), 1423–1426. doi: 10.1126/science.1134457
Tang, L. G., Yang, G. G., Ma, M., Liu, X. F., Li, B., Xie, J. T., et al. (2020). An effector of a necrotrophic fungal pathogen targets the calcium-sensing receptor in chloroplasts to inhibit host resistance. Mol. Plant Pathol. 21 (5), 686–701. doi: 10.1111/mpp.12922
Terashima, M., Petroutsos, D., Hüdlg, M., Tolstygina, I., Trompelt, K., Gäbelein, P., et al. (2012). Calcium-dependent regulation of cyclic photosynthetic electron transfer by a CAS, ANR1, and PGRL1 complex. Proc. Natl. Acad. Sci. U. S. A. 109 (43), 17717–17722. doi: 10.1073/pnas.1300172110
Trippens, J., Reißenwebe, T., Kreimer, G. (2017). The chloroplast calcium sensor protein CAS affects phototactic behavior in Chlamydomonas reinhardtii (Chlorophyceae) at low light intensities. Phycologia 56 (3), 261–270. doi: 10.2216/16-67.1
Ueda, M., Seki, M. (2020). Histone modifications form epigenetic regulatory networks to regulate abiotic stress response. Plant Physiol. 182 (1), 15–26. doi: 10.1104/pp.19.00988
Vainonen, J. P., Sakuragi, Y., Stael, S., Tikkanen, M., Allahverdiyeva, Y., Paakkarinen, V., et al. (2008). Light regulation of CAS, a novel phosphoprotein in the thylakoid membrane of Arabidopsis thaliana. FEBS J. 275 (8), 1767–1777. doi: 10.1111/j.1742-4658.2008.06335.x
Vener, A. V. (2007). Environmentally modulated phosphorylation and dynamics of proteins in photosynthetic membranes Biochim. Biophys. Acta-Bioenerg. 1767 (6), 449–457. doi: 10.1016/j.bbabio.2006.11.007
Wang, W. H. (2013). Studies on signal transduction and CAS function involved in plant calcium receptor CAS (Xiamen: University of XiaMen). Available at: http://dspace.xmu.edu.cn:8080/dspace/handle/2288/78689.
Wang, W. H., Chen, J., Liu, T. W., Chen, J., Han, A. D., Simon, M., et al. (2014). Regulation of the calcium-sensing receptor in both stomatal movement and photosynthetic electron transport is crucial for water use efficiency and drought tolerance in Arabidopsis. J. Exp. Bot. 65 (1), 223–234. doi: 10.1093/jxb/ert362
Wang, W. H., He, E. M., Chen, J., Guo, Y., Chen, J., Liu, X., et al. (2016b). The reduced state of the plastoquinone pool is required for chloroplast-mediated stomatal closure in response to calcium stimulation. Plant J. 86, 132–144. doi: 10.1111/tpj.13154
Wang, L. Y., Yamano, T., Takane, S., Niikawa, Y., Toyokawa, C., Ozawa, S. I., et al. (2016a). Chloroplast-mediated regulation of CO2-concentrating mechanism by Ca2+-binding protein CAS in the green alga Chlamydomonas reinhardtii. Proc. Natl. Acad. Sci. U. S. A. 113 (44), 12586–12591. doi: 10.1073/pnas.1606519113
Wang, W. H., Yi, X. Q., Han, A. D., Liu, T. W., Chen, J., Wu, F. H., et al. (2012). Calcium-sensing receptor regulates stomatal closure through hydrogen peroxide and nitric oxide in response to extracellular calcium in Arabidopsis. J. Exp. Bot. 63 (1), 177–190. doi: 10.4161/psb.18882
Weinl, S., Held, K., Schlucking, K., Steinhorst, L., Kuhlgert, S., Hippler, M., et al. (2008). A plastid protein crucial for Ca2+-regulated stomatal responses. New Phytol. 179 (3), 675–686. doi: 10.1111/j.1469-8137.2008.02492.x
Wu, D., Liu, Y., Pang, J., Yong, J. W. H., Chen, Y., Bai, C., et al. (2020). Exogenous calcium alleviates nocturnal chilling-induced feedback inhibition of photosynthesis by improving sink demand in peanut (Arachis hypogaea). Front. Plant Sci. 11. doi: 10.3389/fpls.2020.607029
Xu, Y., Huang, B. (2018). Comparative transcriptomic analysis reveals common molecular factors responsive to heat and drought stress in Agrostis stolonifera. Sci. Rep. 8 (1), 15181. doi: 10.1038/s41598-018-33597-3
Yamano, T., Toyokawa, C., Fukuzawa, H. (2018). High-resolution suborganellar localization of Ca2+-binding protein CAS, a novel regulator of CO2-concentrating mechanism. Protoplasma 255 (4), 1015–1022. doi: 10.1007/s00709-018-1208-2
Zhang, J., Fang, H., Huo, J. Q., Huang, D. J., Wang, B., Liao, W. B. (2018). Involvement of calcium and calmodulin in nitric oxide-regulated senescence of cut lily flowers. Front. Plant Sci. 9. doi: 10.3389/fpls.2018.01284
Zhang, J. L., Zhou, M. X., Liu, W., Nie, J. J., Huang, L. L.. (2022). Pseudomonas syringae pv. actinidiae effector HopAU1 interacts with calcium-sensing receptor to activate plant immunity. Int. J. Mol. Sci. 23 (1), 508. doi: 10.3390/ijms23010508
Zhao, X., Wang, Y. J., Wang, Y. L., Wang, X. L., Zhang, X. (2011). Extracellular Ca2+ alleviates NaCl-induced stomatal opening through a pathway involving H2O2-blocked na+ influx in Vicia guard cells. J. Plant Physiol. 168 (9), 903–910. doi: 10.1016/j.jplph.2010.11.024
Zhao, X., Xu, M. M., Wei, R. R., Liu, Y. (2015). Expression of OsCAS (Calcium-sensing receptor) in an Arabidopsis mutant increases drought tolerance. PloS One 10 (6), e0131272. doi: 10.1371/journal.pone.0131272
Keywords: abiotic stress, photosynthesis, calcium, biological functionality, calcium-sensing receptor
Citation: Bai R, Bai C, Han X, Liu Y and Yong JWH (2022) The significance of calcium-sensing receptor in sustaining photosynthesis and ameliorating stress responses in plants. Front. Plant Sci. 13:1019505. doi: 10.3389/fpls.2022.1019505
Received: 15 August 2022; Accepted: 22 September 2022;
Published: 11 October 2022.
Edited by:
Mahmoud Magdy, Ain Shams University, EgyptReviewed by:
Congming Lu, Shandong Agricultural University, ChinaShoaib Munir, Huazhong Agricultural University, China
Copyright © 2022 Bai, Bai, Han, Liu and Yong. This is an open-access article distributed under the terms of the Creative Commons Attribution License (CC BY). The use, distribution or reproduction in other forums is permitted, provided the original author(s) and the copyright owner(s) are credited and that the original publication in this journal is cited, in accordance with accepted academic practice. No use, distribution or reproduction is permitted which does not comply with these terms.
*Correspondence: Yifei Liu, eWlmZWlsaXU2QGhvdG1haWwuY29t; bGl1eWlmZWlAc3lhdS5lZHUuY24=
†These authors have contributed equally to this work