- 1Institute of Experimental Botany of the Czech Academy of Sciences, Centre of the Region Haná for Biotechnological and Agricultural Research, Olomouc, Czechia
- 2Field Crops Research Institute, Agricultural Research Centre, Cairo, Egypt
- 3Agricultural Institute, Centre for Agricultural Research, Eötvös Lóránd Kutatási Hálózat (ELKH), Martonvásár, Hungary
- 4Wheat Genetics Resource Center, Kansas State University, Manhattan, KS, United States
Breeding of wheat adapted to new climatic conditions and resistant to diseases and pests is hindered by a limited gene pool due to domestication and thousands of years of human selection. Annual goatgrasses (Aegilops spp.) with M and U genomes are potential sources of the missing genes and alleles. Development of alien introgression lines of wheat may be facilitated by the knowledge of DNA sequences of Aegilops chromosomes. As the Aegilops genomes are complex, sequencing relevant Aegilops chromosomes purified by flow cytometric sorting offers an attractive route forward. The present study extends the potential of chromosome genomics to allotetraploid Ae. biuncialis and Ae. geniculata by dissecting their M and U genomes into individual chromosomes. Hybridization of FITC-conjugated GAA oligonucleotide probe to chromosomes suspensions of the two species allowed the application of bivariate flow karyotyping and sorting some individual chromosomes. Bivariate flow karyotype FITC vs. DAPI of Ae. biuncialis consisted of nine chromosome-populations, but their chromosome content determined by microscopic analysis of flow sorted chromosomes indicated that only 7Mb and 1Ub could be sorted at high purity. In the case of Ae. geniculata, fourteen chromosome-populations were discriminated, allowing the separation of nine individual chromosomes (1Mg, 3Mg, 5Mg, 6Mg, 7Mg, 1Ug, 3Ug, 6Ug, and 7Ug) out of the 14. To sort the remaining chromosomes, a partial set of wheat-Ae. biuncialis and a whole set of wheat-Ae. geniculata chromosome addition lines were also flow karyotyped, revealing clear separation of the GAA-rich Aegilops chromosomes from the GAA-poor A- and D-genome chromosomes of wheat. All of the alien chromosomes represented by individual addition lines could be isolated at purities ranging from 74.5% to 96.6% and from 87.8% to 97.7%, respectively. Differences in flow karyotypes between Ae. biuncialis and Ae. geniculata were analyzed and discussed. Chromosome-specific genomic resources will facilitate gene cloning and the development of molecular tools to support alien introgression breeding of wheat.
Introduction
The gene pool of bread wheat has narrowed during thousands of years of domestication and cultivation. This resulted in the loss of some useful genes, including those underlying resistance to diseases, pests, and abiotic stress as well as those affecting grain quality. Reduced genetic diversity hampers the development of new wheat cultivars with improved quality and stress tolerance. As the wild-crop relatives have not been subjected to human selection, they exhibit large genetic variation and offer a rich source of alleles and genes for crop improvement (Tanksley and McCouch, 1997; Feuillet et al., 2008). Alien gene transfer by chromosome engineering is an effective strategy to exploit this wild genetic diversity for wheat improvement (Schneider and Molnár-Láng, 2009; Ochoa et al., 2015; Zhang et al., 2017).
The tribe Triticeae contains about one hundred annual species, including those of the genus Aegilops, commonly referred to as goatgrasses (van Slageren, 1994). Annual goatgrasses (Aegilops spp.) are closely related to the genus Triticum and represent a rich source of genes with considerable agronomic value (Friebe et al., 1996; Schneider et al., 2008; Kilian et al., 2011). Within the genus, seven different genomes (C, D, M, N, S, T, and U) were identified in eleven diploid and twelve polyploid species (van Slageren, 1994). Among the allopolyploid Aegilops species containing M- and U-genomes are Ae. biuncialis Vis. (2n = 4x = 28, UbUbMbMb) and Ae. geniculata. Roth. (2n = 4x = 28, UgUgMgMg) exhibits a wide eco-geographical distribution in the Mediterranean and Western Asiatic regions (van Slageren, 1994). The wide distribution of these species has been attributed to their intraspecific diversity manifested at DNA sequence level (Arrigo et al., 2010; Ivanizs et al., 2019; Ivanizs et al., 2022), as well as chromosome level structural changes in the genebank collections of Ae. biuncialis and Ae. geniculata (Badaeva et al., 2004; Molnár et al., 2011a). In parallel with the genetic diversity, these allotetraploid species have been reported as sources of resistance to rust diseases (Olivera et al., 2018), powdery mildew, Hessian fly, and greenbug (Gill et al., 1985). Other accessions have high tolerance to abiotic stresses such as frost (Ekmekci and Terzioglu, 2002), salt (Darko et al., 2020), and drought (Molnár et al., 2004; Pradhan et al., 2012; Dulai et al., 2014), or found to have beneficial grain quality traits, such as high micronutrient (Farkas et al., 2014), edible fiber and protein content compared to bread wheat (Rakszegi et al., 2017; Marcotuli et al., 2019; Ivanizs et al., 2022). In order to transfer desirable agronomic traits from these Aegilops species, a partial set of wheat-Ae. biuncialis chromosome addition lines (2Mb, 3Mb, 7Mb, 1Ub, 2Ub, and 3Ub) (Schneider et al., 2005), and a complete set of wheat-Ae. geniculata addition lines were developed (Friebe et al., 1999). In contrast to the potential allelic variations for useful traits, the genetic diversity of Ae. biuncialis and Ae. geniculata remains largely unexploited in wheat breeding, mainly because the selection of alien introgression lines is laborious and time-consuming (Kuraparthy et al., 2009; Farkas et al., 2014; Tiwari et al., 2014).
One way to facilitate the development of wheat-Aegilops translocation lines is to sequence M and U genomes. Assembled chromosome sequences and gene models would provide unique information for the development of DNA markers suitable for selection of wheat lines with introgressed Aegilops fragments (Tiwari et al., 2014; Tiwari et al., 2015; Li et al., 2021; Said et al., 2021); for mining wild alleles of agronomically important genes (Ivanizs et al., 2022); and for cloning resistance genes against pests and diseases (Yu et al., 2022). However, sequencing and assembly of large and allotetraploid genomes of Aegilops remain challenging because of the high proportion of repetitive DNA and allopolyploid genome structure. In fact, the 1C genome sizes of Ae. biuncialis and Ae. geniculata were estimated to be 10,142 Mbp (10.37 pg DNA) and 10,064 Mbp (10.29 pg DNA), respectively (Eilam et al., 2008; Bennett and Leitch, 2011).
The analysis of large and complex nuclear genomes can be simplified by dissecting them into individual chromosomes by flow cytometric sorting (Doležel et al., 2007; Doležel et al., 2014). Chromosome fractions purified by flow sorting can be used as DNA templates for PCR-based assays, including the assignment of wheat molecular markers to chromosomes of Aegilops and the establishment of wheat-alien homoeologous relationships (Molnár et al., 2011a; Molnár et al., 2016). Chromosomal DNA has also been reported to be suitable for the production of chromosome specific BAC libraries and for the development of molecular and cytogenetic markers (Šimková et al., 2008a; Doležel et al., 2012; Doležel et al., 2014; Kapustová et al., 2019). Barley, rye, and wheat flow sorted chromosomes facilitated the establishment of linear gene-order models and allowed assessment of gene synteny with other species (Mayer et al., 2009; Mayer et al., 2011; Martis et al., 2013; International Wheat Genome Sequencing Consortium (IWGSC), 2014; Molnár et al., 2016). Moreover, the development of reference genome assemblies for agronomically important species such as barley (International Barley Genome Sequencing Consortium et al., 2012) and bread wheat (International Wheat Genome Sequencing Consortium (IWGSC), 2018), and for their wild gene source species, Ae. sharonensis (Yu et al., 2022) was enabled through the use of flow sorted chromosomes. Chromosome-based approaches were also developed for cloning agronomically important genes (Sánchez-Martín et al., 2016; Thind et al., 2017; Zwyrtková et al., 2021) and have become an important tool for the functional genomics of cereals.
The application of chromosome-based genomic approaches relies on the ability to discriminate individual chromosomes by flow cytometric analysis. Original protocols developed for Vicia faba used univariate flow karyotyping, where intact mitotic chromosomes are classified according to the fluorescence intensity of a fluorochrome bound to DNA, most frequently DAPI, which provides information about chromosome size or relative DNA content (Doležel et al., 2014). In the case of cereals, similar to most plant species, the size of individual chromosomes is quite similar, and only one to three chromosomes show enough difference to form specific peaks on flow karyotypes, while the remaining chromosomes form either a single composite peak, or several smaller composite peaks. In the case of Aegilops with M and U genomes, univariate flow karyotyping indicated that three chromosomes (1U, 3U, and 6U) could be sorted in high purity from diploid Ae. umbellulata (2n = 4x = 14, UU), two (7Mb and 1Ub) from Ae. biuncialis, while no chromosome specific peaks were detected in Ae. comosa (2n = 4x = 14, MM) and Ae. geniculata (Molnár et al., 2011a).
To overcome the problem of chromosome discrimination, Giorgi et al. (2013) developed a method termed FISHIS (fluorescence in situ hybridization in suspension), which uses FITC conjugated microsatellite probes to visualize GAA clusters on DAPI stained chromosomes prior to flow cytometric analysis. The resulting bivariate DAPI vs. FITC flow karyotypes allow much better discrimination of individual chromosomes and sorting of the majority of them at high purity (Giorgi et al., 2013; Doležel et al., 2014; Akpinar et al., 2015; Molnár et al., 2016; Said et al., 2019a; Said et al., 2021). Bivariate flow karyotyping has been used successfully in plant species whose genomes contain GAA clusters in cytogenetically detectable amounts, as demonstrated in T. aestivum, T. turgidum ssp. durum, and Hordeum vulgare (Doležel et al., 2021). The M and U genomes of Aegilops are also rich in GAA clusters (Molnár et al., 2011a), and bivariate flow cytometric analysis of diploid Ae. comosa and Ae. umbellulata allowed flow sorting of individual chromosomes 1M–7M and 1U–7U, respectively (Molnár et al., 2016; Said et al., 2021). Chromosomes flow sorted from these two species allowed for the study of wheat-Aegilops cross-genome homoeology and the development of molecular tools to support wheat alien chromosome introgression breeding programs (Said et al., 2021). On the other hand, bivariate flow karyotyping has not been applied to allotetraploid Aegilops species so far.
Another approach to discriminating and flow sorting individual chromosomes is to use wheat-alien cytogenetic stocks, such as translocations, disomic chromosome additions, substitutions, or ditelosomic lines (Doležel et al., 2007; Doležel et al., 2014). If the size of the chromosomes containing the alien chromatin differs from that of normal wheat chromosomes, it can be discriminated against and flow sorted at high purity. The availability of wheat-alien addition lines made it possible to flow sort chromosomes of Dasypyrum villosum (Grosso et al., 2012; Xing et al., 2021). This approach was more effective if FISHIS was applied, as reported for sorting chromosome arms of 2US, 2UL, and 7UL from wheat-Ae. umbellulata ditelosomic lines (Molnár et al., 2016). The application of wheat-alien chromosome addition lines was especially important when no individual chromosomes could be resolved in the bivariate flow karyotype of the gene source species, as was the case of Agropyron cristatum, whose chromosomes 1P–6P could only be flow sorted at high purity from wheat-A. cristatum chromosome addition lines (Said et al., 2019a).
Motivated by the need to develop chromosome-specific genomic resources for structural and functional analysis of Ae. biuncialis and Ae. geniculata and to produce molecular tools to facilitate gene introgression into wheat, we investigated the ability to flow sort individual chromosomes 1M–7M and 1U–7U of the Ae. biuncialis and Ae. geniculata genomes. We used FITC-conjugated (GAA)7 microsatellite repeat probe for fluorescent labeling of DAPI stained mitotic chromosomes in suspension prior to flow-cytometric analysis to potentially facilitate sorting individual M and U chromosomes from tetraploid Ae. biuncialis and Ae. geniculata species as well as from wheat-Aegilops addition lines.
Materials and methods
Plant material
Seeds of Ae. geniculata (2n = 4x = 28, UgUgMgMg) accessions AE1311/00 and AE660/83 were obtained from the Leibniz Institute of Plant Genetics and Crop Plant Research, IPK (Gatersleben, Germany). Ae. biuncialis (2n = 4x = 28, UbUbMbMb) accession MvGB382, bread wheat line Mv9kr1 and Mv9kr1-Ae. biuncialis accession MvGB642 chromosome addition lines 2Mb, 3Mb, 7Mb, 1Ub, and 6Ub (double addition) and 3Ub (Schneider et al., 2005) were obtained from the Martonvásár Cereal Genebank (Martonvásár, Hungary). Bread wheat cv. Chinese Spring (CS) and CS-Ae. geniculata accession TA2899 chromosome addition lines 1Mg–7Mg and 1Ug–7Ug (Friebe et al., 1999) were kindly provided by Dr. Bernd Friebe (Kansas State University, Manhattan, Kansas, USA). The information about the plant material used in this work is summarized in Supplementary Table 1.
Mitotic chromosome preparations
For the flow cytometric analysis and chromosome sorting, suspensions of intact mitotic metaphase chromosomes were prepared from the synchronized root tips of allotetraploid Ae. biuncialis and Ae. geniculata, and hexaploid wheat genotypes Mv9kr1, CS, and Mv9kr1-Ae. biuncialis and CS-Ae. geniculata chromosome addition lines following the method of Vrána et al. (2016a, 2016b). Briefly, root tip meristem cells of young seedlings were synchronized using hydroxyurea, accumulated in metaphase using amiprophos-methyl and mildly fixed by formaldehyde as described by Vrána et al. (2016a, 2016b). Mitotic metaphase chromosomes were released from root tips into LB01 buffer by mechanical homogenization.
Flow cytometric analysis and sorting of Aegilops chromosomes
Prior to flow cytometric analysis, chromosomes in suspension were fluorescently labeled by FISHIS using oligonucleotide probe 5’-FITC-(GAA)7-FITC-3’ (Integrated DNA Technologies, Inc., Iowa, USA) and counterstained with DAPI (4´,6-diamidino 2-phenylindole) following Giorgi et al. (2013). Bivariate flow karyotyping and chromosome sorting were done on the FACSAria II SORP flow cytometer and sorter (Becton Dickinson Immunocytometry Systems, San José, USA) as described by Molnár et al. (2016) and Said et al. (2019a). Chromosome samples were analyzed at rates of 900–1,400 particles per second. Bivariate flow karyotypes of FITC pulse area (FITC-A) vs. DAPI pulse area (DAPI-A) fluorescence were acquired and 20,000 events were recorded to create a bivariate flow karyotype for each experiment. Sort regions were set on the flow karyotypes and chromosomes were flow sorted at rates of 12–24 per second. Chromosome content in flow-sorted fractions and identification of contaminating chromosomes were determined by fluorescence in situ hybridization (FISH) on chromosomes sorted onto microscope slides using probes for pSc119.2, Afa family repeat, and 45S rDNA according to Molnár et al. (2016). Briefly, 3,000 chromosomes were flow sorted from each chromosome population onto a microscope slide into a 3 μl drop of PRINS buffer supplemented with 2.5% sucrose (Kubaláková et al., 1997). The slides were air dried and used for FISH experiments. The chromosomes were classified following the karyotype described by Molnár et al. (2011a).
Preparation of probes for FISH
The rye 120-bp repeat family (Bedbrook et al., 1980) was amplified by PCR from DNA clone pSc119.2 using M13 universal primers (Said et al., 2018), whereas Afa family repeat was amplified from genomic DNA of bread wheat CS using primers AS-A and AS-B (Nagaki et al., 1995). The probes pSc119.2 and Afa family repeat were labeled by PCR with digoxigenin-dUTP and biotin-dUTP (Roche, Mannheim, Germany), respectively (Said et al., 2018; Said et al., 2019b). Plasmid pTa71 (45S rDNA) containing a 9-kb fragment from T. aestivum with 18S-5.8S-26S rDNA and intergenic spacers (Gerlach and Bedbrook, 1979) was labeled by nick translation with either biotin-dUTP or digoxigenin-dUTP (Roche) using standard Nick Translation Mix kits (Roche) following the instructions of the manufacturer.
Fluorescence in situ hybridization
All FISH analyses of chromosomes sorted onto microscope slides were done with three probes (45S rDNA, tandem repeats pSc119.2 and Afa family), which were hybridized simultaneously. The experiments, including the denaturation of probe and chromosomal DNA, the hybridization, and the post hybridization washes, followed the protocols of Molnár et al. (2016) and Said et al. (2021) with small modifications. Briefly, the FISH hybridization mix (15 μl per slide) contained 50 ng labeled probe DNA, 50% v/v formamide, 2 × SSC (0.15 mol/l NaCl plus 0.015 mol/l sodium citrate), 10% w/v dextran sulfate, 0.4 μg salmon sperm DNA, and 0.1% w/v sodium dodecyl sulfate. The probes were denatured at 93°C for 10 min and maintained in ice for 5 min. Then the chromosomes and probes were denatured together at 80°C for 45 s under high moisture conditions. Hybridization was carried out overnight at 37°C in a humid chamber. Hybridization signals of digoxigenin-labeled probes were detected using anti-digoxigenin fluorescein isothiocyanate (Roche), while the signals of biotin-labeled probes were detected with Cy3-conjugated streptavidin (Invitrogen, Life Technologies, Carlsbad, USA). Chromosomes were mounted and counterstained with DAPI in an antifade mounting medium (Vectashield, Vector Laboratories, Burlingame, USA).
Microscopy, software, signal capture and image analysis
After FISH, chromosomes on microscope slides were examined using an Axio Imager Z.2 fluorescence microscope (Zeiss, Oberkochen, Germany) equipped with a Cool Cube 1 camera (Metasystems, Altlussheim, Germany) and appropriate filter sets. The signal capture and picture processing were performed using ISIS software (Metasystems). The final image adjustment was done in Adobe Photoshop CS5 (Adobe Systems Incorporated, San Jose, USA). In order to determine the chromosome content of the flow sorted samples, at least 100 chromosomes were identified for each sample using a standard karyotype (Molnár et al., 2011a).
Results
Chromosome flow sorting from tetraploid Ae. biuncialis and Ae. geniculata
We first investigated the potential of univariate flow cytometric analysis based on DAPI fluorescence alone to flow sort individual chromosomes from allotetraploid Ae. biuncialis and Ae. geniculata. The analyses showed that peaks of some chromosomes (7Mb, 1Ub), (5Mg, 6Mg, 1Ug), and (4Mg, 5Mg, 6Mg, 1Ug) from Ae. biuncialis MvGB382, Ae. geniculata 1,311/00, and Ae. geniculata AE660/83, respectively, could be discriminated and the chromosomes flow sorted individually (Supplementary Figure 1). The remaining chromosomes could be sorted only into groups of more than two.
We then applied bivariate flow cytometric analysis to improve the resolution of individual chromosomes of Ae. biuncialis and Ae. geniculata. FISHIS labeling of (GAA)7 clusters on the DAPI stained chromosomes resulted in a bivariate (DAPI-A vs. FITC-A) flow karyotype of Ae. biuncialis acc. MvGB382. On the dot plot flow karyotype (Figure 1), nine chromosome groups (I–IX) were discriminated, which is less than the haploid chromosome number (n = 14) of the species.
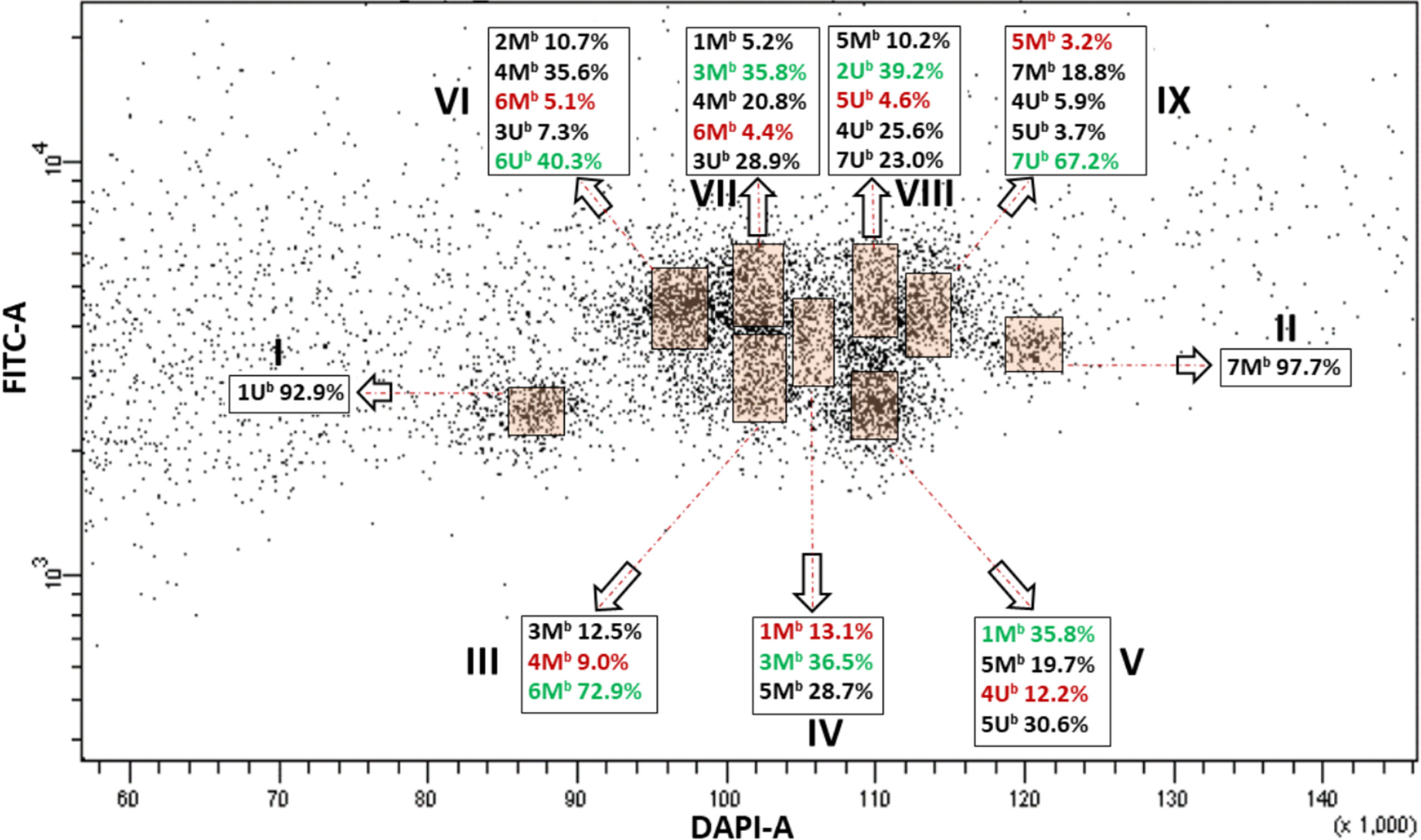
Figure 1 Bivariate flow karyotype (DAPI-A vs. FITC-A) of Ae. biuncialis acc. MvGB382. Flow cytometric analysis of DAPI stained mitotic chromosomes labeled by FISHIS with GAA7-FITC probe discriminated nine chromosome populations (I–IX) representing the whole genome of the species. Chromosomes in the sort windows (brown) were sorted onto microscope slides and classified by FISH. The red and green numbers within the chromosome content of the sorted populations (black rectangles) refer to the lowest and the highest purities, respectively. x-axis, relative DAPI fluorescence. y-axis; relative GAA7-FITC fluorescence.
FISH using probes for 45S rDNA, tandem repeats pSc119.2 and the Afa family on chromosomes flow sorted onto microscope slides enabled determination of the chromosome content of the nine groups of the flow karyotype (Figures 1, 2A). The analysis showed that chromosomes 1Ub (I) and 7Mb (II) could be sorted individually at purities reaching 92.9% and 97.7%, respectively. However, the remaining chromosomes could be flow sorted only into groups of three (III and IV), four (V), or even five (VI–IX) chromosomes. Identification of flow sorted Mb- and Ub-genome chromosomes showed that two groups III and IV consisted of each of three chromosomes with proportions equaling 9.0% (4Mb)–72.9% (6Mb) and 13.1 (1Mb)–36.5 (3Mb), respectively. One group (V) contained four chromosomes with proportions equaling 12.2% (4Ub)–35.8% (1Mb). Four groups (VI–IX) were each comprised of five chromosomes with proportions ranged from 5.1% (6Mb)–40.3% (6Ub), 4.4% (6Mb)–35.8% (3Mb), 4.6% (5Ub)–39.2% (2Ub), and 3.2% (5Mb)–67.2% (7Ub), respectively (Figure 1).
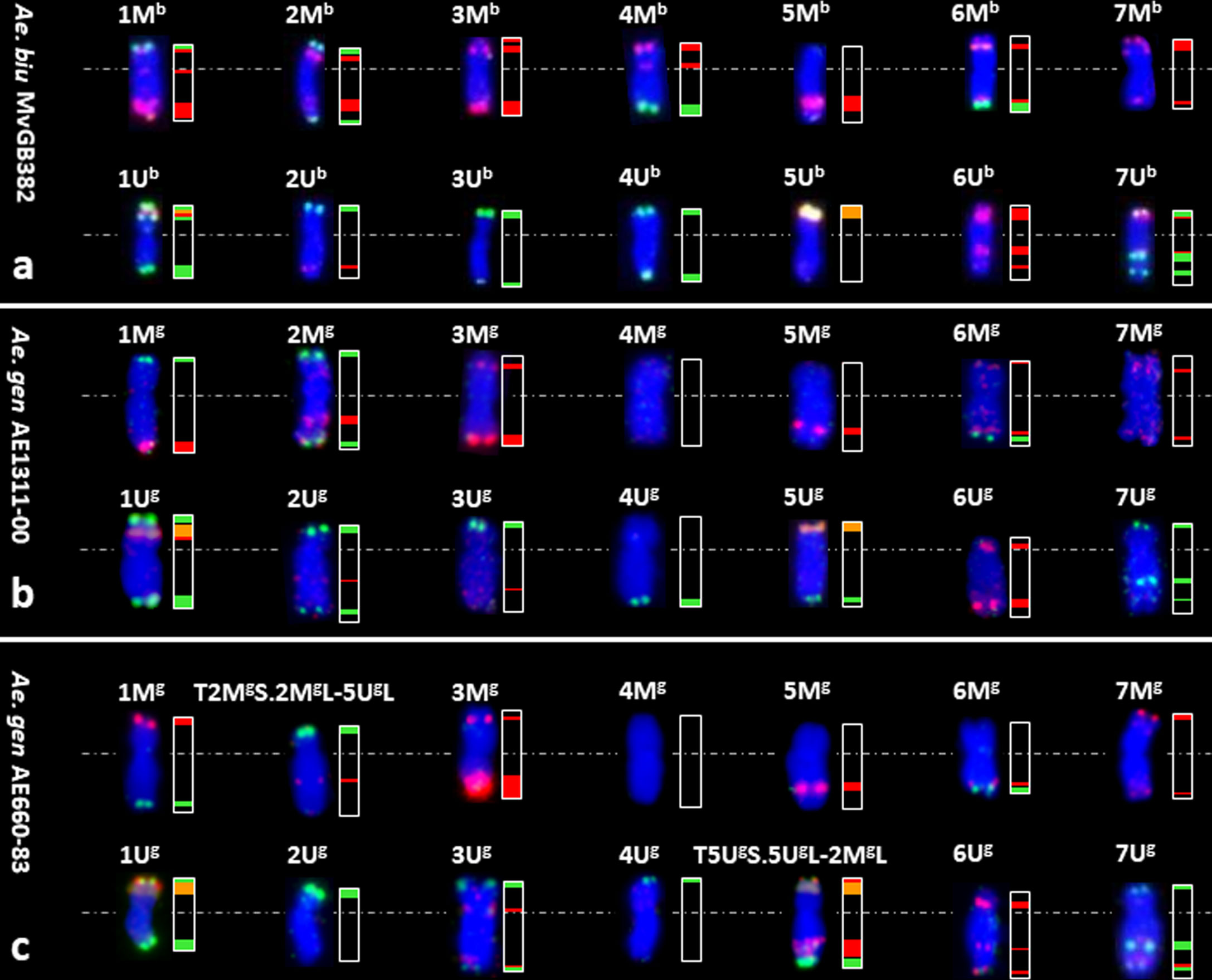
Figure 2 Identification of the M- and U-genome chromosomes (left) flow sorted from Aegilops species and their Idiograms (right). FISH with probes for Afa family repeat (red), 45S rDNA (orange) and pSc119.2 repeat (green) on chromosomes flow sorted from Ae. biuncialis acc. MvGB382 (A), Ae. geniculata acc. AE1311/00 (B) and Ae. geniculata acc. AE660/83 (C). Chromosomes were counterstained with DAPI (blue).
Bivariate (DAPI-A vs. FITC-A) flow karyotype of Ae. geniculata accession AE1311/00 allowed identification of fourteen chromosome groups (I–XIV) corresponding to the haploid chromosome number (n = 14) of the species (Figure 3). FISH on flow sorted chromosomes (Figure 2B) confirmed that nine groups (I–IX) consisted of individual chromosomes (1Ug, 5Mg, 3Mg, 6Mg, 6Ug, 3Ug, 7Ug, 1Mg, and 7Mg), which could be flow sorted separately at purities equaling 98.5%, 96.8%, 78.8%, 81.3%, 80.5%, 94.0%, 72.6%, 95.2%, and 88.0%, respectively. The other four groups (X–XIII) consisted of four chromosomes each and could be flow sorted at proportions ranged from 2.3% (1Mg)–59.0% (4Ug), 43.7% (2Mg)–6.1% (7Ug), 1.3% (2Mg)–57.3% (4Mg), and 0.6% (1Mg and 2Ug)–51.8% (7Mg), respectively. The remaining group (XIV) was identified as a composite of seven chromosomes, which were flow sorted in proportions ranging from 1.3% (7Mg) to 31.4% (7Ug).
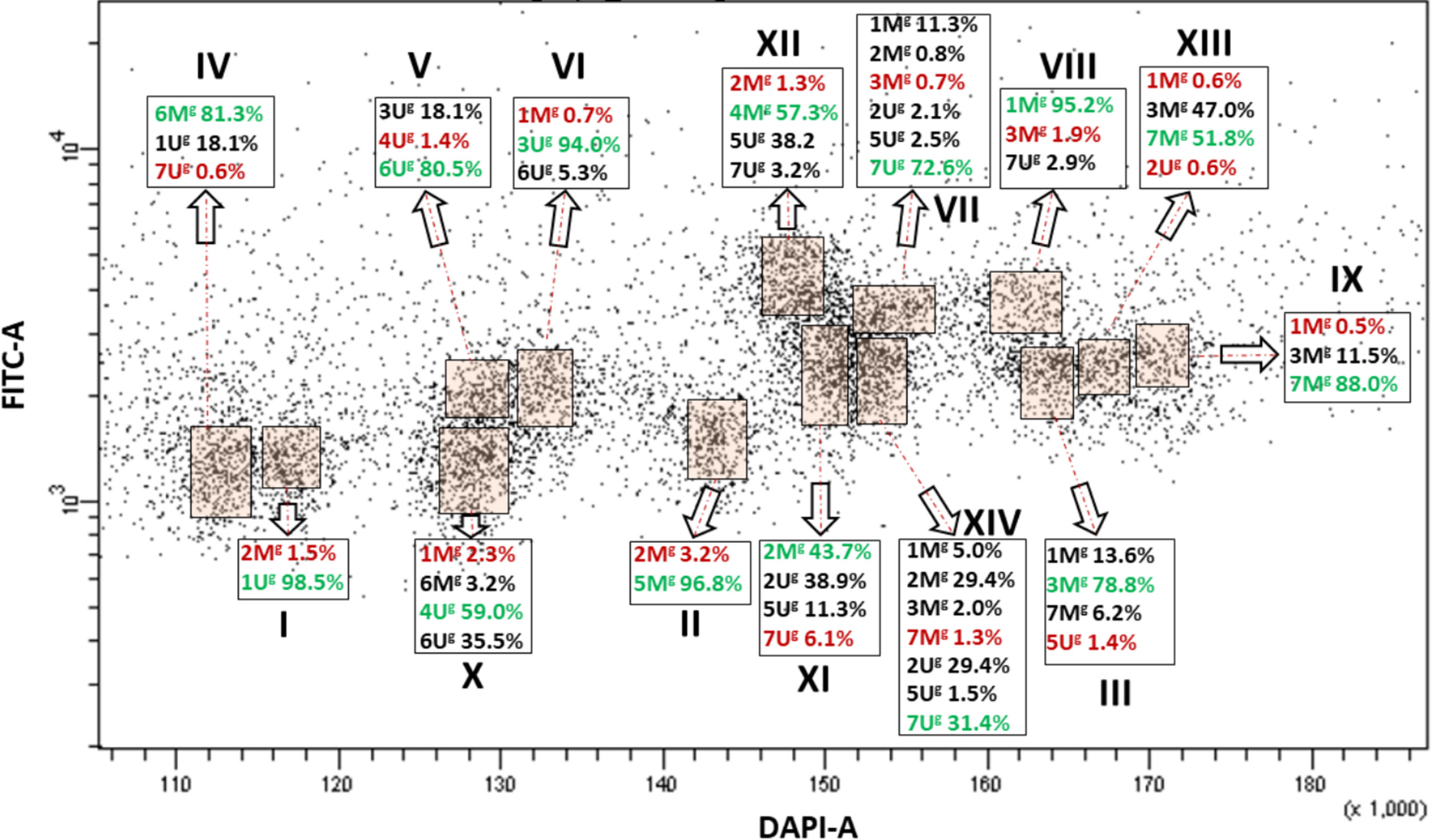
Figure 3 Bivariate flow karyotype (DAPI-A vs. FITC-A) obtained after FISHIS with the GAA7 probe on chromosomes isolated from Ae. geniculata acc. AE1311/00. The analysis permitted discrimination of fourteen chromosome populations (orange boxes) representing the whole genome of the species. The red and green numbers within a group of chromosomes sorted together refer to the lowest and highest purities, respectively. x-axis, relative DAPI fluorescence; y-axis, relative GAA7-FITC fluorescence.
We then wanted to check if there was any effect of the modification in the karyotype on flow sorting of individual chromosomes, therefore we investigated the Ae. geniculata accession AE660-83, which has a 5Ug/2Mg reciprocal translocation (Molnár et al., 2011a) and as shown in Figure 2C. Bivariate (DAPI vs. FITC) flow karyotyping after FISHIS allowed identification of fourteen chromosome groups (I–XIV) representing the whole genome of the species (Figure 4). FISH analysis (Figure 2C) indicated that six chromosomes (6Mg, 1Ug, 1Mg, 5Mg, 4Mg, and 6Ug) could be sorted individually from groups (I–VI) at reasonable purities of 92.7%, 95.7%, 96.1%, 86.3%, 95.2%, and 89.1%, respectively. The remaining chromosomes could be sorted only into groups of three, four, or five. One group (VII) consisted of three chromosomes, which were sorted in proportions ranging from 8.0% (4Ug) to 48.5% (3Mg). Two groups (VIII and IX) contained four chromosomes and could be sorted into proportions ranging from 4.3% (T5UgS.5UgL-2MgL)–58.2% (T2MgS.2MgL-5UgL) and 3.5% (3Ug)–42.2% (2Mg), respectively. The remaining groups (X–XIV) consisted of five chromosomes each and flow sorted at proportions ranged 0.5% (1Ug)–63.4% (6Ug), 1.1% (7Mg)–62.7% (7Ug), 1.5% (T2MgS.2MgL-5UgL)–53.9% (T5UgS.5UgL-2MgL), 32.5% (4Ug)–0.7% (7Ug) and 0.7% (1Ug)–52.2% (3Ug), respectively.
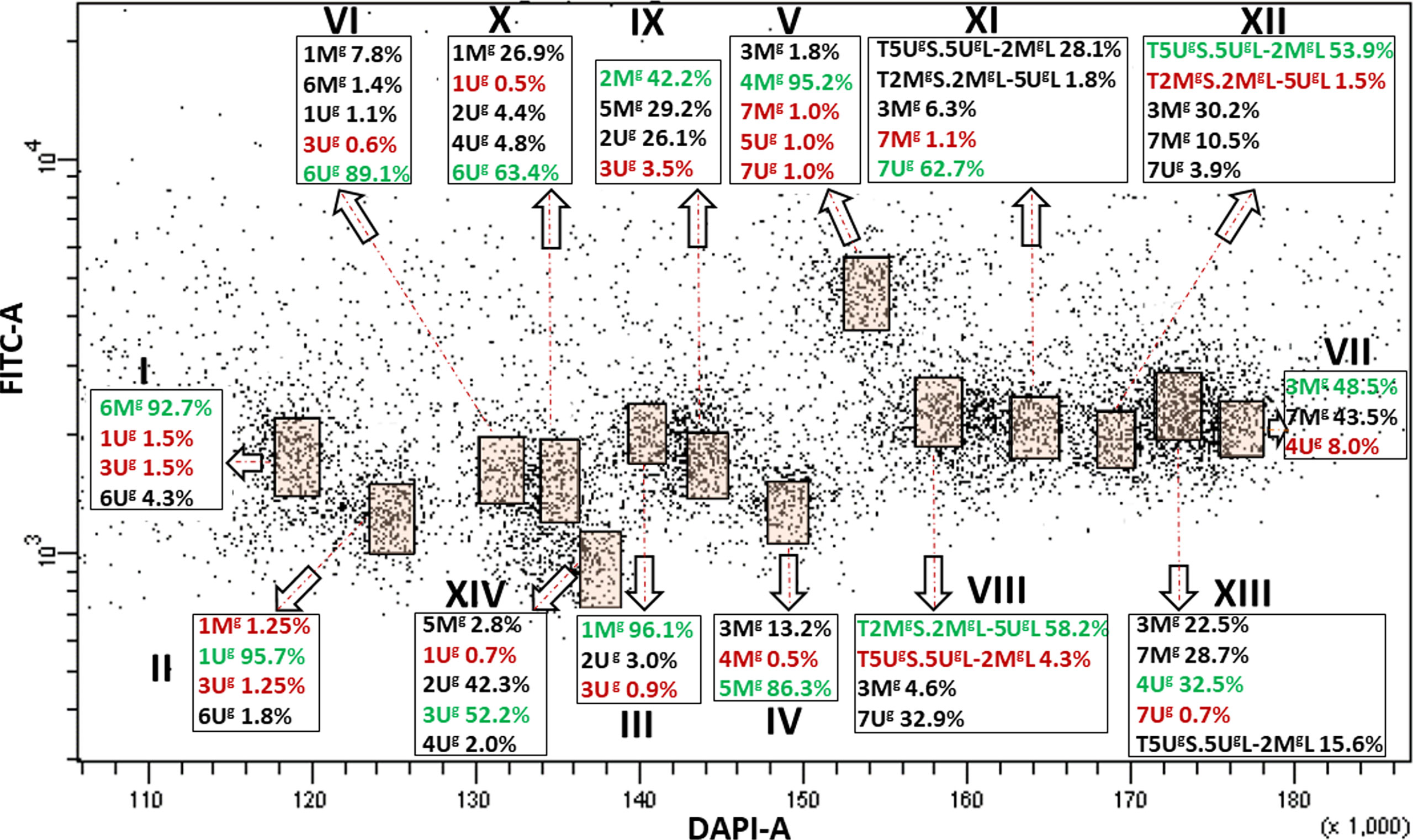
Figure 4 Bivariate flow karyotype (DAPI-A vs. FITC-A) obtained after FISHIS with the GAA7 probe on chromosomes isolated from Ae. geniculata acc. AE660/83. The analysis permitted discrimination of fourteen chromosome populations (orange boxes) representing the whole genome of the species. The red and green numbers within a group of chromosomes sorted together refer to the lowest and highest purities, respectively. x-axis, relative DAPI fluorescence; y-axis, relative GAA7-FITC fluorescence.
In both species, the majority of the chromosome groups were contaminated with chromosomes that were assigned to other groups but were also oscillating between the different chromosome populations as shown in Figures 1, 3, 4.
Chromosome flow sorting from wheat-Aegilops disomic addition lines
Because of the isolation of pure (>80%) fractions was not possible for each of the M- and U-genome chromosomes of allotetraploid Ae. biuncialis and Ae. geniculata, we also tested wheat-Ae. biuncialis and wheat-Ae. geniculata disomic addition lines. As control, flow cytometric analysis of the parental hexaploid wheat genotypes, Mv9kr1 and CS, was carried out first (Figure 5). We tested different settings of the flow cytometer to find the most optimal display of the chromosomal events for discrimination of individual chromosomes. Although the bivariate analysis using the gain of the forward scatter pulse area (FSC-A) and DAPI fluorescence pulse area (DAPI-A) (Figures 5A, D) and monovariate analysis of DAPI-A and number of chromosomal events (Figures 5B, E) are essential, they did not enable flow sorting of individual chromosomes.
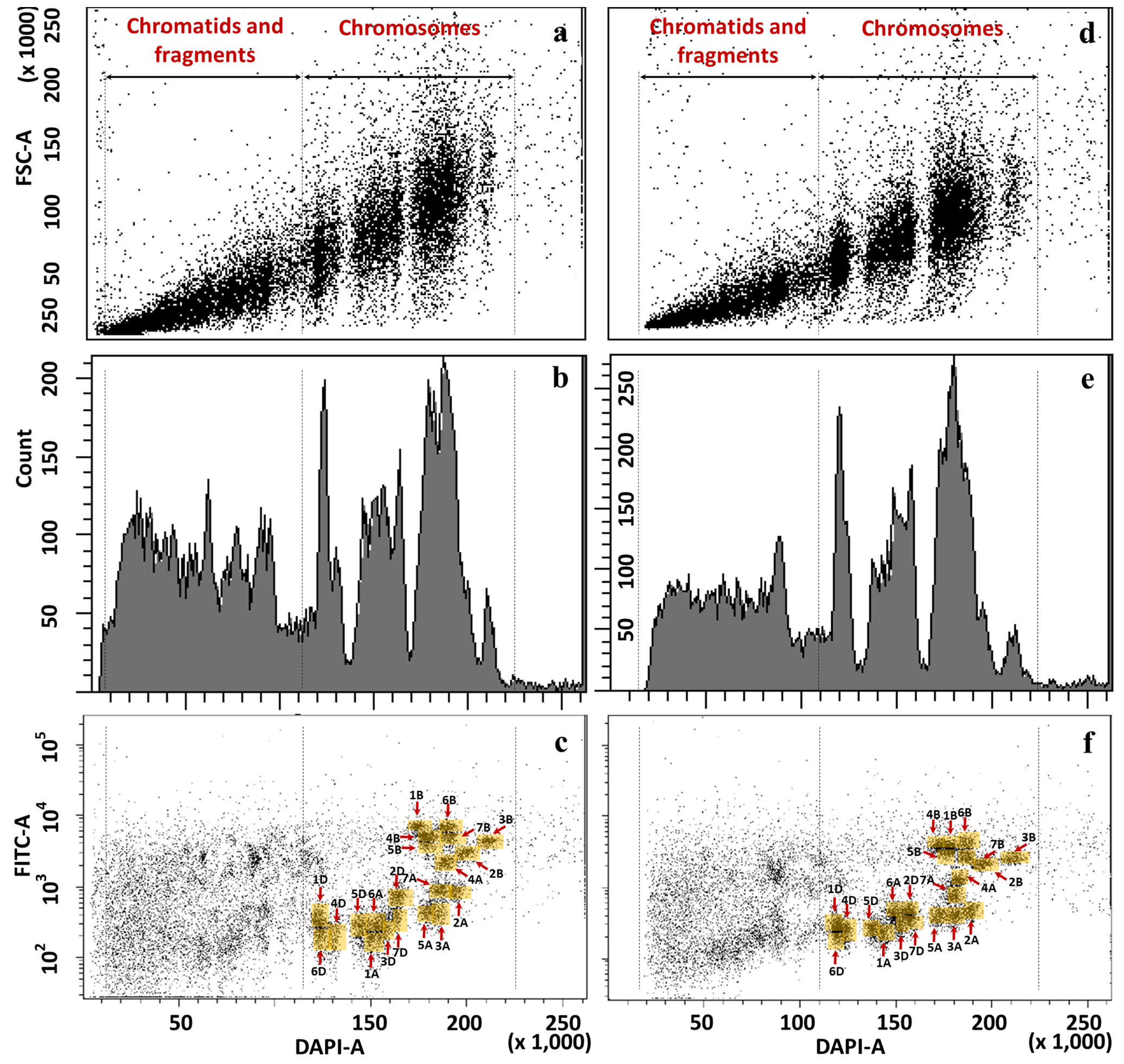
Figure 5 Flow cytometric analysis and sorting in hexaploid wheat cv. Mv9kr1 (A–C) and cv. CS (D–F) chromosomes. (A, D). bivariate flow karyotypes DAPI (x-axis) vs. FSC (y-axis), (B, E) monovariate flow karyotypes DAPI (x-axis) showing count (y-axis), (C, F) bivariate flow karyotypes DAPI (x-axis) vs. FITC (y-axis) obtained after FISHIS with (GAA)7 on chromosomes isolated from wheat Mv9kr1 (C) and wheat CS (F). The analysis permitted discrimination of the 21 chromosome pairs (yellow boxes) of hexaploid wheat.
On the contrary, bivariate flow karyotypes DAPI-A vs. FITC-A obtained from wheat genotypes allowed the resolution of almost all the 21 wheat chromosomes (Figures 5C, F). The positions of the 21 chromosome pairs from ABD genomes of both Mv9kr1 and CS genotypes were also assigned (Figures 5C, F) and confirmed by FISH experiments on chromosomes flow sorted onto microscope slides from each population (data not shown). The position of the chromosomes on the flow karyotype indicated that the D-genome chromosomes have the smallest DNA content, followed by the chromosomes of the A-genome. Because the A- and D-genome chromosomes have a small number of (GAA)7 clusters, they were detected at the lower FITC fluorescence intensities. On the contrary, B-genome chromosomes, having the highest DNA content and a huge number of (GAA)7 clusters, were detected at the flow karyotype areas characterized by higher DAPI and FITC fluorescence intensities.
Bivariate flow karyotypes of wheat-Aegilops addition lines showed that the relative DNA content of Aegilops chromosomes was significantly smaller than the B-genome chromosomes but similar to that of A- and D-genome chromosomes. However, as the tetraploid Aegilops M- and U-genome chromosomes have prominent (GAA)7 clusters, their FITC fluorescence was much higher as compared to wheat A- and D-genome chromosomes. These differences made it possible to discriminate against Aegilops chromosomes from those of wheat A, B, and D genomes (Figures 6–8).
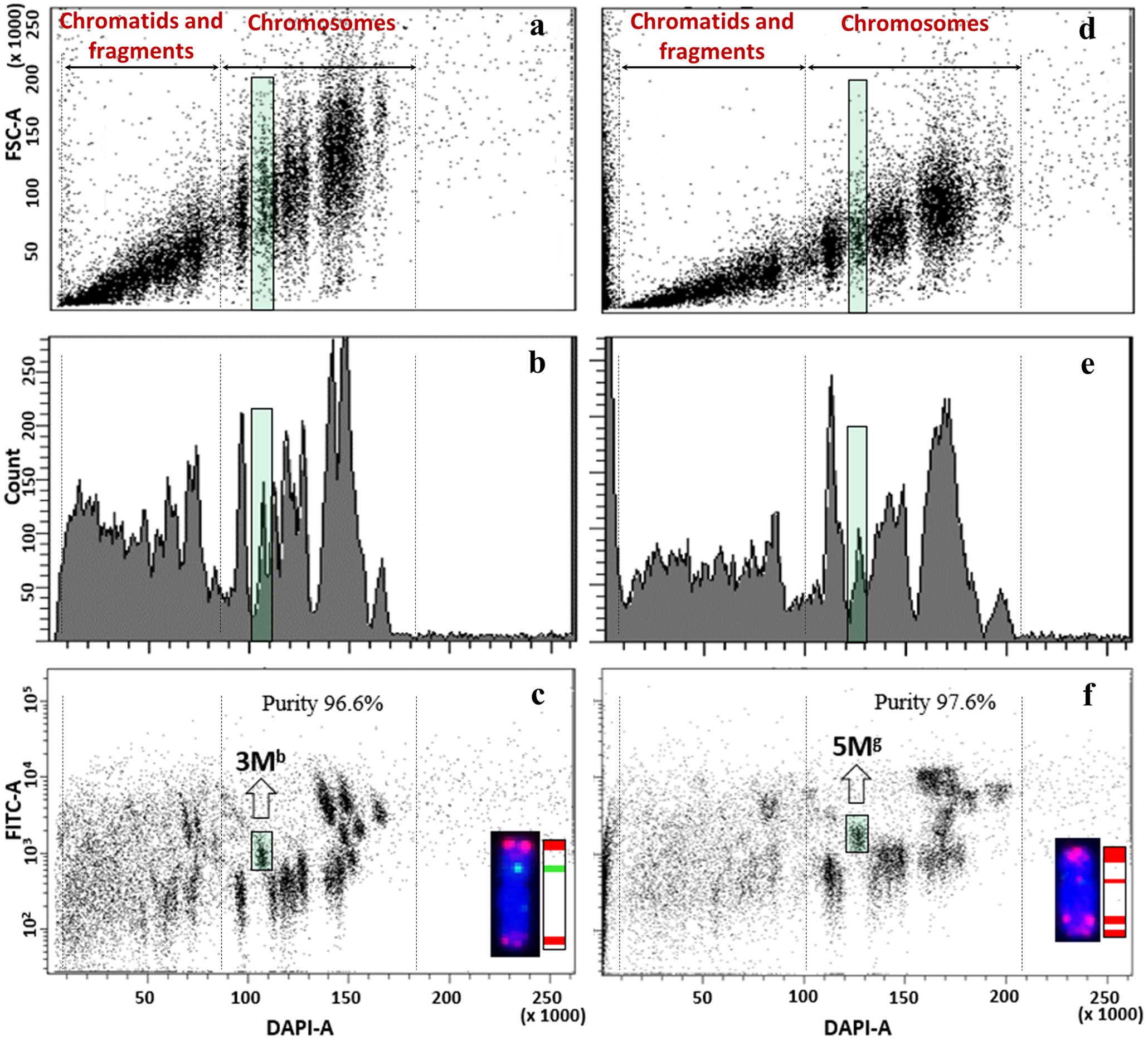
Figure 6 Flow cytometric analysis and sorting of Ae. biuncialis (A-C) and Ae. geniculata (D–F) chromosomes from wheat disomic addition lines. (A, D). bivariate flow karyotypes DAPI (x-axis) vs. FSC (y-axis), (B, E) monovariate flow karyotypes DAPI (x-axis) showing count (y-axis), (C, F) bivariate flow karyotypes DAPI (x-axis) vs. FITC (y-axis) obtained after FISHIS with (GAA)7 on chromosomes isolated from wheat Mv9kr1-Ae. biuncialis acc. MvGB642 (C) and wheat CS-Ae. geniculata acc. TA2899 (F) addition lines. The analysis permitted discrimination and sorting of the tetraploid Aegilops chromosomes (green boxes) in the genetic background of bread wheat (C, F). The chromosomes (inset) were sorted at high purity and identified by FISH on microscope slides with probes for Afa family repeat (red), 45S rDNA (orange) and pSc119.2 repeat (green). Chromosomes were counterstained with DAPI (blue).
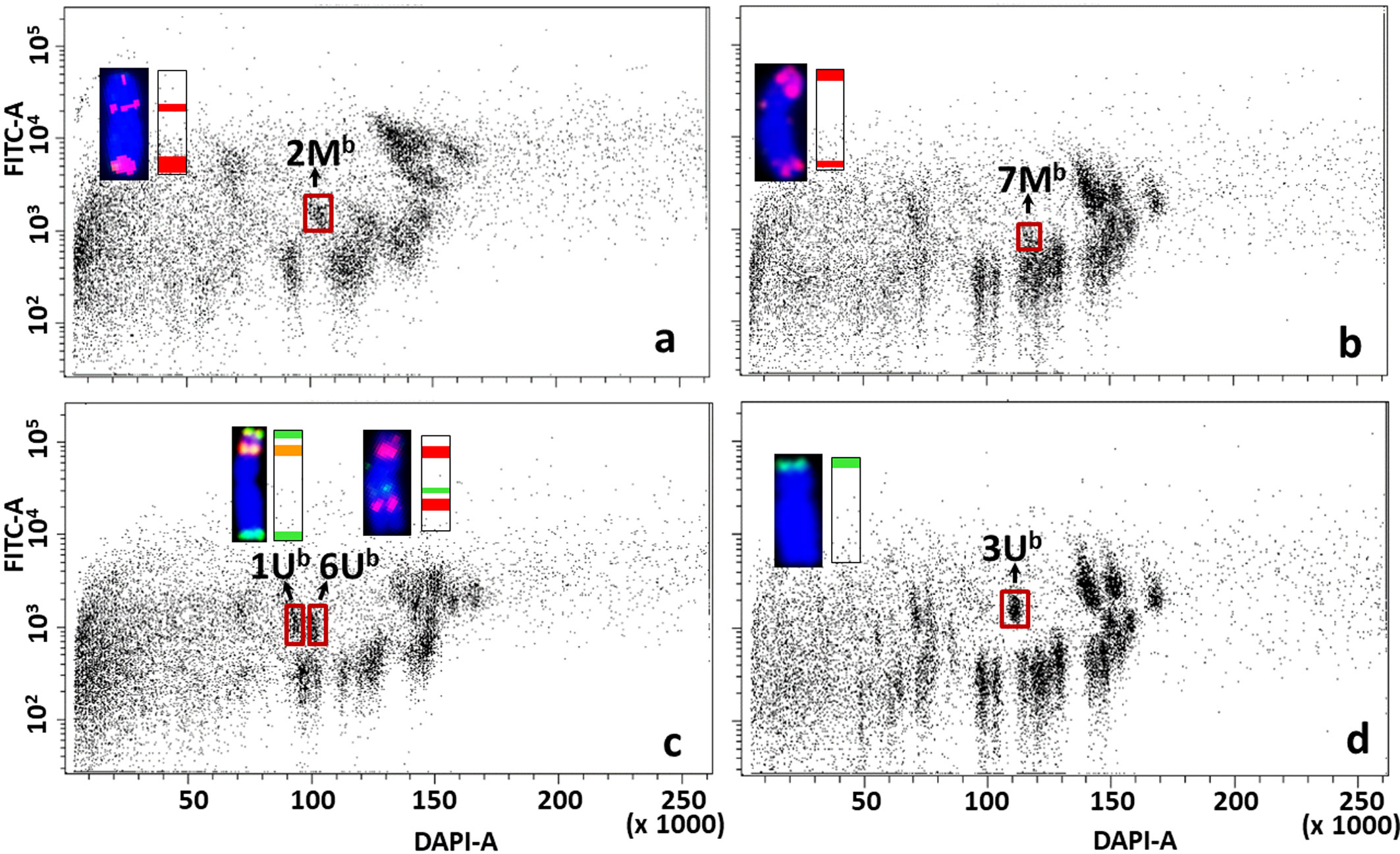
Figure 7 Bivariate flow karyotypes DAPI vs. FITC obtained after FISHIS with (GAA)7 on chromosomes isolated from wheat Mv9kr1-Ae. biuncialis acc. MvGB642 addition lines. The analysis permitted discrimination of chromosomes from tetraploid Ae. biuncialis (brown boxes) in the genetic background of bread wheat (A–D). The chromosomes (inset) were sorted in high purity and identified by FISH on microscope slides with probes for Afa family repeat (red), 45S rDNA (orange) and pSc119.2 repeat (green). Chromosomes were counterstained with DAPI (blue). x-axis, relative DAPI fluorescence; y-axis, relative (GAA)7-FITC fluorescence.
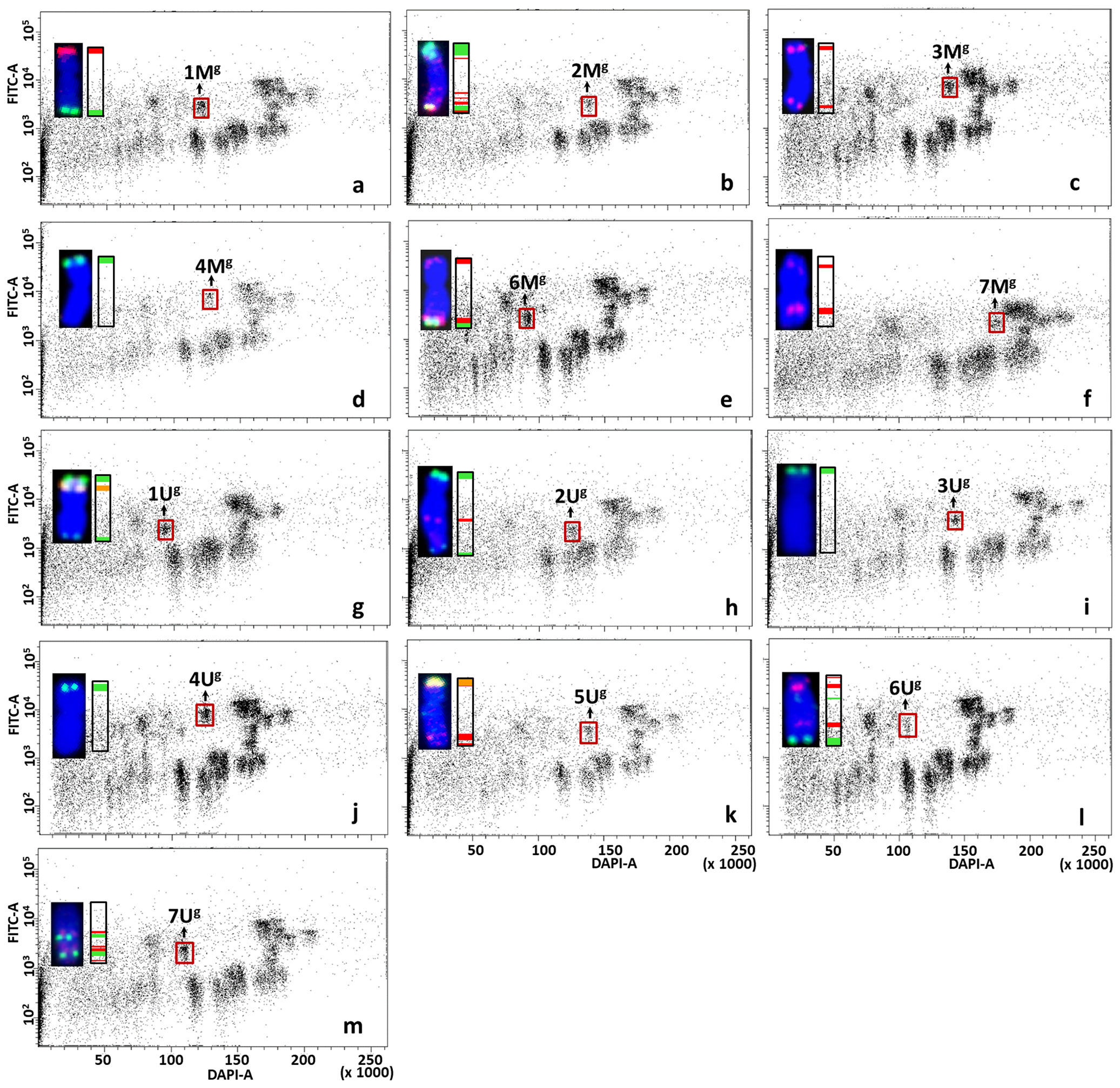
Figure 8 Bivariate flow karyotypes DAPI vs. FITC obtained after FISHIS with GAA7 on chromosomes isolated from wheat CS-Ae. geniculata acc. TA2899 addition lines. The analysis permitted discrimination of the whole chromosome set from tetraploid Ae. geniculata (brown boxes) in the genetic background of bread wheat (A–M). The chromosomes (inset) were sorted in high purity and identified by FISH on microscope slides with probes for Afa family repeat (red), 45S rDNA (orange) and pSc119.2 repeat (green). Chromosomes were counterstained with DAPI (blue). x-axis, relative DAPI fluorescence; y-axis, relative (GAA)7-FITC fluorescence.
Contrasting bivariate (DAPI-A vs. FITC-A) flow karyotypes of Mv9kr1 (Figure 5C) and CS wheats (Figure 5F) with their respective wheat-Aegilops addition lines (Figures 6–8), unambiguously confirmed the positions of all alien chromosomes from Aegilops on bivariate (DAPI-A vs. FITC-A) dot plots (Figures 6–8).
Aegilops chromosomes were flow sorted from wheat Mv9kr1 and CS addition lines onto microscope slides and could be identified unambiguously by FISH with probes for 45S rDNA, tandem repeats pSc119.2 and Afa family repeat (Figures 6–8). FISH analysis revealed that the partial set of Mb and Ub chromosomes of tetraploid Ae. biuncialis could be flow sorted at high purities from wheat Mv9kr1-Ae. biuncialis disomic addition lines (2Mb 93.4%, 3Mb 98.7%, 7Mb 79.9%, 1Ub 90.2%, 3Ub 94.4%, and 6Ub 94.1%). Chromosomes 1Ub and 6Ub were found in a double disomic wheat Mv9kr1-Ae. biuncialis addition line (Figure 7C) and both chromosomes were sorted separately but simultaneously. The purity data on Aegilops chromosomes flow sorted from wheat Mv9kr1-Ae. biuncialis addition lines (Figure 7) are summarized in Table 1.
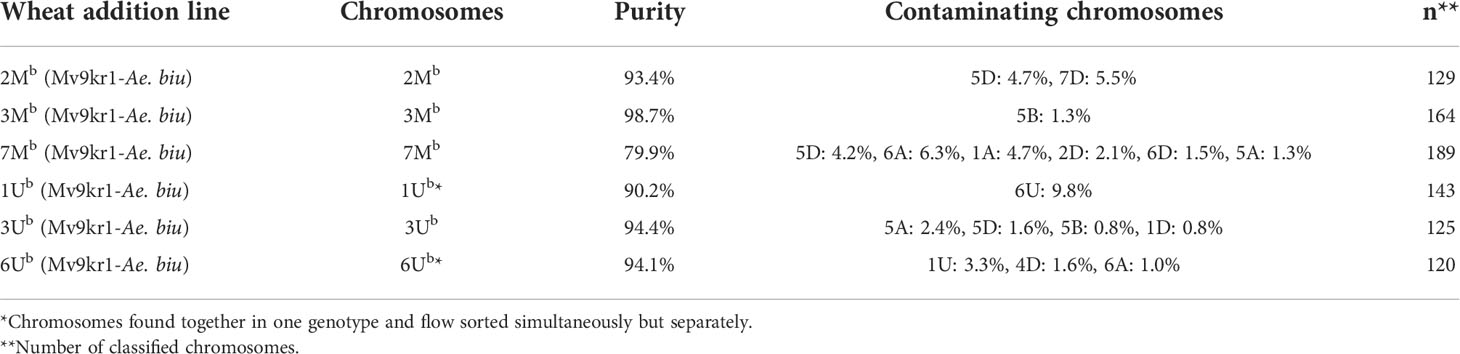
Table 1 Purity in Mb- and Ub-genome chromosome fractions flow sorted from Mv9kr1-Ae. biuncialis acc. MvGB642 disomic addition lines.
The complete set of Mg- and Ug-genome chromosomes was successfully flow sorted from wheat CS-Ae. geniculata disomic addition lines at high purity (1Mg–7Mg: 90.9%, 93.8%, 94.6%, 93.5%, 97.6%, 82.3%, and 96.1%, respectively; 1Ug–7Ug: 87.8%, 94.1%, 97.2%, 93.6%, 97.7%, 68.8%, and 90.8%, respectively). The data about the purity of chromosomes flow sorted from wheat CS-Ae. geniculata addition lines (Figure 8) are summarized in Table 2.
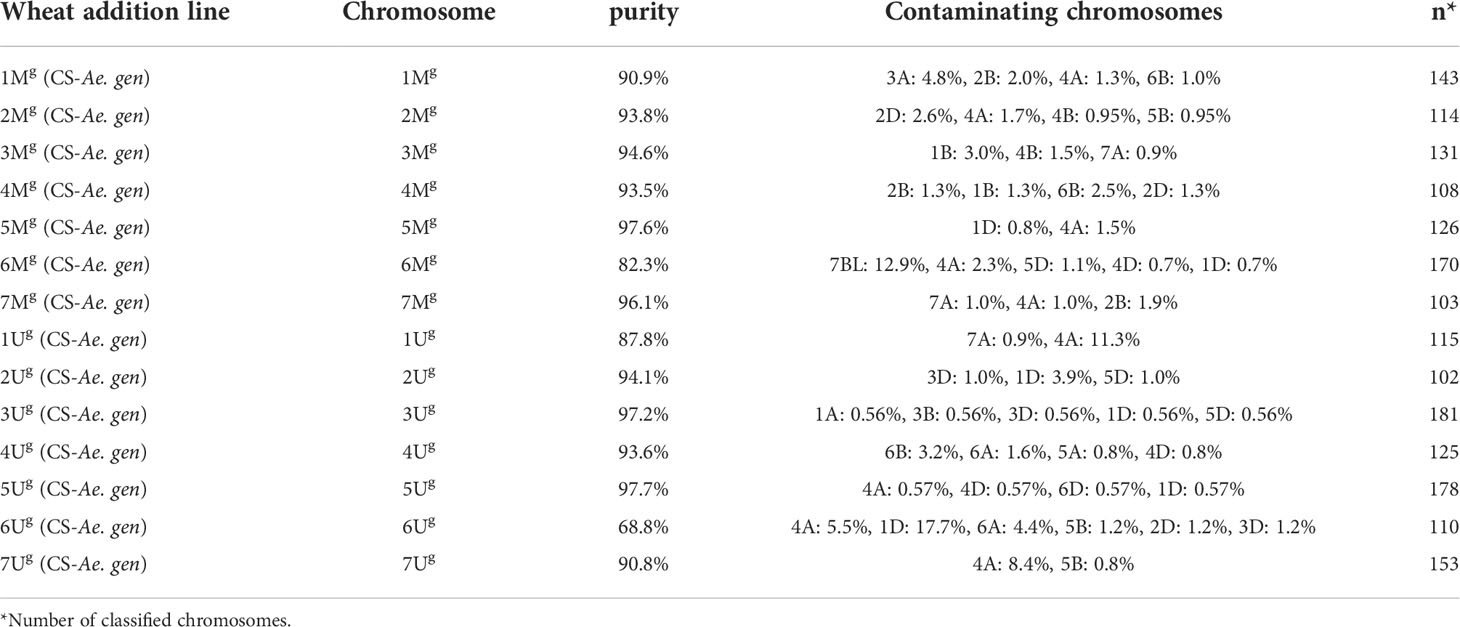
Table 2 Purity of Mg and Ug chromosomes flow sorted from CS-Ae. geniculata acc. TA2899 disomic addition lines.
Discussion
Transfer of genes with agronomical importance from the M and U genomes of Aegilops into cultivated wheat could be facilitated by genomic approaches used for pure fractions of individual chromosomes. One approach is the shotgun sequencing of flow sorted chromosomes, which allows one to determine gene content and develop PCR markers for specific cytogenetic positions to support gene introgression processes as demonstrated for diploid Ae. comosa and Ae. umbellulata (Said et al., 2021). Using bivariate flow cytometric analysis on gene bank accessions and wheat-alien chromosome addition lines, the present study extended the ability of chromosome-based approaches to the allotetraploid Ae. biuncialis and Ae. geniculata.
Over the years, chromosome flow sorting has proven to be a powerful tool in the analysis of complex plant genomes (Zwyrtková et al., 2021). Among other uses, the method was instrumental in the construction of chromosome-specific DNA libraries and the development of molecular and cytogenetic markers (Šimková et al., 2008a; Doležel et al., 2012; Doležel et al., 2014). The availability of purified chromosome fractions facilitated the establishment of wheat-wild relatives cross-genome homoeology (Molnár et al., 2013; Molnár et al., 2016) and the production of draft sequence assemblies for wild gene source species such as Ae. comosa, Ae. umbellulata (Said et al., 2021), and A. cristatum (Zwyrtková et al., 2022). The method was also essential for the development of reference genome assemblies for cereal species with economic importance, such as barley (International Barley Genome Sequencing Consortium et al., 2012) and bread wheat (International Wheat Genome Sequencing Consortium (IWGSC), 2018), as well as for their wild relative Ae. sharonensis (Yu et al., 2022).
Monovariate flow karyotyping
Flow cytometric analysis of chromosome suspensions from Ae. biuncialis and Ae. geniculata based on DAPI fluorescence alone showed that some chromosomes could be flow sorted individually (7Mb and 1Ub from Ae. biuncialis; 5Mg, 6Mg, and 1Ug from Ae. geniculata AE1311/00; and 4Mg, 5Mg, 6Mg, and 1Ug from Ae. geniculata AE660/83). However, the remaining chromosomes could be sorted only into groups of more than two. These observations confirm previous studies carried out on tetraploid Ae. biuncialis and Ae. geniculata, where monovariate flow karyotypes obtained on DAPI-stained chromosomes showed that two peaks specific to chromosome 7Mb and 1Ub could be discriminated in tetraploid Ae. biuncialis and the chromosomes could be sorted with purities exceeding 95.9% and 81.2%, respectively (Molnár et al., 2011b). The remaining chromosomes formed composite peaks and could only be sorted into groups of six. However, no specific peaks for individual chromosomes were discriminated in tetraploid Ae. geniculata. The chromosomes formed composite peaks and could be sorted into groups of two or more (Molnár et al., 2011b). Similar results were obtained in other cultivated and wild cereal species, such as rye, durum wheat, Ae. umbellulata, Ae. comosa, Ae. speltoides, Ae. markgrafii, and diploid and tetraploid A. cristatum (Kubaláková et al., 2003; Kubaláková et al., 2005; Molnár et al., 2011a; Molnár et al., 2011b; Molnár et al., 2014; Molnár et al., 2015; Said et al., 2019a). This is a common situation in cereals and due to similarity in size, only 1–3 chromosomes show enough difference to form separate peaks, while the remaining chromosomes form either a single composite peak, or several smaller composite peaks (Lysák et al., 1999; Vrána et al., 2000; Kubaláková et al., 2003; Šimková et al., 2008b). Although this result indicates that it is not possible to dissect the whole genome into single chromosomes by size, it shows that it is possible to dissect the complex genome of allotetraploid Aegilops species into groups of some specific chromosomes and use these enriched subgenomic fractions for downstream analyses (Vrána et al., 2015).
Monovariate analysis has some significant advantages, such as higher sort yield (typically 400,000–900,000 chromosomes per working day) than bivariate flow sorting (Doležel et al., 2021) and the lack of fluorochrome-labeled DNA in the sorted chromosome fraction, which is required in some downstream applications such as optical mapping. Both of these characteristics allow the purification of high molecular weight (HMW) chromosomal DNA (50–150 kbp). Some genomic approaches, such as BAC library construction (Šafář et al., 2004), optical mapping (Staňková et al., 2016), and chromosome conformation capture (Hi-C) (Mascher et al., 2017), require 600–2,000 ng of HMW DNA, which requires 3–4 weeks of sorting. Monovariate sorting of Aegilops chromosomes opens the way for these genomic applications, which facilitate the development of high-quality reference sequences of these chromosomes.
Bivariate flow karyotyping
Our bivariate flow cytometric analysis indicated that M- and U-genome chromosomes labeled by FISHIS were characterized by high FITC fluorescence intensity, suggesting the presence of large GAA microsatellite clusters in the genomes of Ae. biuncialis and Ae. geniculata. These observations confirmed the previous results of Molnár et al. (2011a) and Abdolmalaki et al. (2019), who reported the presence of strong GAA signals after FISH on root tip chromosome spreads of Ae. biuncialis and Ae. geniculata. These results are also in line with the previous findings that the microscopically detectable GAA clusters in the Aegilops C, M, N, S, and U genomes can also be visualized by FISH in suspension (FISHIS) to label chromosomes prior to flow cytometric analysis (Giorgi et al., 2013), as it was demonstrated for diploid genome progenitor species (Molnár et al., 2016; Said et al., 2021; Yu et al., 2022). Furthermore, our results on Ae. biuncialis and Ae. geniculata also suggest that bivariate flow karyotyping on other allopolyploid Aegilops species containing M- and U-genomes (i.e., Ae. columnaris, Ae. neglecta) or the Aegilops species with other GAA-rich genome combinations such as S- and U genomes (Ae. kotschyi, Ae. peregrina) or C- and U genomes (Ae. triuncialis) (Kilian et al., 2011) can also be carried out.
Although bivariate flow karyotyping improved the resolution of individual chromosomes relative to monovariate flow karyotyping, it was not sufficient to discriminate all chromosomes of Ae. biuncialis, with the exception of 7Mb and 1Ub, which were already flow sorted using univariate flow karyotyping. However, in the case of Ae. geniculata, bivariate flow karyotyping allowed separation of nine out of the 14 chromosomes from the accession AE1311/00 with a wild type karyotype (Molnár et al., 2011a). The significant difference in the pattern of bivariate flow karyotype DAPI vs. FITC between Ae. biuncialis and Ae. geniculata (i.e., differences in chromosome size and GAA abundance) may be related to the structural and functional reorganization processes that lead to genome downsizing after allopolyploidization event (Ozkan et al., 2003; Eilam et al., 2008; Feldman and Levy, 2015). Deletion or proliferation of transposable elements (TE) has been reported as a predominant phenomenon after the allopolyploidization (Leitch et al., 2008). In line with this, some specific TE families, such as Sabine, show contrasting evolutionary trajectories after polyploidization, as significant proliferation was observed in Ae. cylindrica, while its significant elimination was detected in Ae. geniculata relative to diploid genome progenitors (Senerchia et al., 2014). It is feasible that different TE elements were eliminated or proliferated after the formation of Ae. biuncialis and Ae. geniculata, leading to differences in the size of the individual chromosomes. Sequencing of chromosomes flow sorted from these species and repeat analysis (Tiwari et al., 2015) could clarify the role of TE dynamics in changes of chromosome size following allopolyploidization.
Translocation can modify the size of chromosomes, thereby adding another opportunity to sort individual chromosomes or to prepare subgenomic samples enriched for a particular chromosome. Flow karyotype of Ae. geniculata accession AE660/83 having a 2Mg/5Ug reciprocal translocation supports this idea as the translocations T2MgS.2MgL-5UgL and T5UgS.5UgL-2MgL were sorted in purities of 58.2% and 53.8%, respectively, while the non-rearranged chromosomes 2Mg and 5Ug could be sorted at significantly lower purities (43.7% and 38.2%, respectively) from the Ae. geniculata AE1311/00 with normal karyotype. Our results on Ae. geniculata are in line with those of Kubaláková et al. (2002), who used the same approach to flow sort translocations T5BL.7BL and T4AL.4AS-5BL from the wheat cultivars Cappelle Desprez and Jubilar, respectively (Kubaláková et al., 2005).
Another approach to overcome the difficulties in flow sorting chromosomes of interest is the use of chromosome addition lines (Doležel and Lucretti, 1995; Doležel et al., 2014). The effectivity of this approach has been demonstrated earlier for the discrimination and flow cytometric sorting of rye chromosomes 2R–7R from wheat–rye disomic addition lines by Kubaláková et al. (2003). Said et al. (2019a) applied this approach to wheat-A. cristatum disomic addition lines to flow sort six chromosomes (1P–6P). Interestingly, sorting P-genome chromosomes lacking GAA signals was possible due to the fact that the sizes of Agropyron chromosomes were larger than the GAA-poor A-genome chromosomes (2A, 3A, 5A, and 7A) of wheat. The situation was the opposite in the preset work, as the GAA-rich Aegilops chromosomes were smaller than the GAA-rich B-genome chromosomes of wheat. Thus, the alien chromosomes were clearly discriminated against those of wheat. We used wheat Mv9kr1-Ae. biuncialis acc. MvGB642 and wheat CS-Ae. geniculata acc. TA2899 disomic chromosome addition lines, where a partial set of chromosomes of tetraploid Ae. biuncialis and a complete set of chromosomes of tetraploid Ae. geniculata were individually transferred to bread wheat genotype Mv9kr1 (Schneider et al., 2005) and CS (Friebe et al., 1999), respectively.
DAPI fluorescence intensity (equivalent to chromosome size and relative DNA content) of Aegilops chromosomes was lower as compared to wheat B-genome chromosomes and similar to A- and D-genome chromosomes. However, the FITC fluorescence intensity (equivalent to GAA clusters) of M- and U-genome chromosomes was higher as compared to those of wheat A- and D-genomes and lower than B-genome chromosomes, which were strongly labeled by the (GAA)7-FITC probe. This permitted discrimination of Aegilops chromosomes and allowed sorting of them with purities ranging from 79.9% (7Mb) to 98.7% (3Mb) in wheat disomic addition lines Mv9kr1-Ae. biuncialis (Table 1), and from 68.8% (6Ug) to 97.7% (5Ug) in wheat disomic addition lines CS-Ae. geniculata (Table 2). A slightly lower purity of 6Ug could be explained by its small size and overlap of its population on flow karyotype with the region representing the smallest wheat chromosomes 1D, 4D, and 6D (Table 2). A similar phenomenon was reported by Said et al. (2019a), where the low purities of A. cristatum telocentric chromosomes flow sorted from wheat-A. cristatum ditelosomic addition lines were attributed to the fact that the flow karyotype position of P-genome telosomes overlapped with the region of wheat chromosome fragments.
In this work, applying bivariate analysis enabled the dissection of M- and U-genomes into individual chromosomes, each of them representing only 12.8% (1M)–15.2% (3M) and 12.7% (1U)–16.0% (4U) of the M- and U-genomes, respectively (Said et al., 2021). The ability to purify individual chromosomes from tetraploid Ae. biuncialis and Ae. geniculata opens the way for some important genomic applications. The DNA of flow sorted chromosomes is suitable for Illumina sequencing to get insights into their repeat landscape and gene content (Tiwari et al., 2015; Zwyrtková et al., 2021) and thereby makes it possible to carry out comparative phylogenomic analysis of diploid and tetraploid species of Aegilops as well as wheat-Aegilops genome comparisons.
It is known that ph-induced meiotic chromosome pairing is one of the most important methods for homoeologous gene transfer from wild relatives into wheat (Qi et al., 2007). Meiotic chromosome pairing analysis of T. aestivum × Ae. geniculata F1 hybrids indicated that M- and U-genome chromosomes of Aegilops are pairing predominantly with A- and D- genome chromosomes of hexaploid wheat, while their pairing with B-genome chromosomes is extremely rare (Cifuentes et al., 2006; Cifuentes and Benavente, 2009). This chromosome pairing behavior suggests that GAA-rich M- and U-genome chromosome segments can be introgressed most probably into the GAA-poor A- or D- genomes of wheat (Bansal et al., 2020; Steadham et al., 2021). A-Aegilops or D-Aegilops translocations developed in the future will presumably be suitable for flow sorting because of their stronger GAA-FITC signal as compared to normal A- or D-genome chromosomes. If so, a chromosome-based approach can be effectively used to identify introgressed genes with agronomic importance (Zwyrtková et al., 2021). Recently developed gene cloning methods such as “Targeted chromosome-based cloning via long-range assembly” (TACCA) (Thind et al., 2017) and “Mutagenesis Chromosome flow sorting and short-read sequencing” (MutChromSeq) (Sánchez-Martín et al., 2016) provide opportunities to identify genes transferred from wild relatives into wheat (Xing et al., 2018).
Finally, we can conclude that the applied bivariate flow cytometric analysis made it possible to dissect the complex genomes of allotetraploid Ae. biuncialis and Ae. geniculata into individual chromosomes. The chromosome specific subgenomic DNA samples provide attractive resources to perform structural and functional genome analysis of these important wild gene sources of wheat and to develop molecular tools to facilitate the exploitation of tetraploid Ae. biuncialis and Ae. geniculata in wheat-alien introgression breeding.
Data availability statement
The original contributions presented in the study are included in the article/Supplementary Material. Further inquiries can be directed to the corresponding author.
Author contributions
The work involved collaboration between all authors. BF, JD, IM, MS, and PC conceived research theme and discussed results. MS and PC performed lab work and flow sorted the chromosomes. IM and MS designed the experiments, carried out FISH tests and drafted the manuscript. AF, EG, IM, and LI carried out the purity estimations and calculations. All authors contributed to the article and approved the submitted version.
Funding
This work has been supported by the ERDF project ‘Plants as a tool for sustainable global development’ (No. CZ.02.1.01/0.0/0.0/16_019/0000827), the Hungarian National Research, Development and Innovation Office (K135057, TKP2021-NKTA-06, and 2019-2.1.11-TÉT-2019-00074), the Marie Curie Fellowship Grant award ‘AEGILWHEAT’ (H2020-MSCA-IF-2016-746253), and the ELIXIR-CZ project (LM2015047), a component of the international ELIXIR infrastructure that is part of the project “e-Infrastruktura CZ” (LM2018140) within the program Projects of Large Research, Development and Innovations Infrastructures.
Acknowledgments
The authors thank Dr. John Raupp from the Kansas State University, Manhattan, Kansas, USA for helping with providing the seeds of wheat cv. CS and CS-Ae. geniculata accession TA2899 chromosome addition lines 1Mg–7Mg and 1Ug–7Ug. We appreciate technical assistance of Zdeňka Dubská, Romana Šperková, and Jitka Weiserová.
Conflict of interest
The authors declare that the research was conducted in the absence of any commercial or financial relationships that could be construed as a potential conflict of interest.
Publisher’s note
All claims expressed in this article are solely those of the authors and do not necessarily represent those of their affiliated organizations, or those of the publisher, the editors and the reviewers. Any product that may be evaluated in this article, or claim that may be made by its manufacturer, is not guaranteed or endorsed by the publisher.
Supplementary material
The Supplementary Material for this article can be found online at: https://www.frontiersin.org/articles/10.3389/fpls.2022.1017958/full#supplementary-material
References
Abdolmalaki, Z., Mirzaghaderi, G., Mason, A. S., Badaeva, E. D. (2019). Molecular cytogenetic analysis reveals evolutionary relationships between polyploid Aegilops species. Plant Syst. Evol. 305, 459–475. doi: 10.1007/s00606-019-01585-3
Akpinar, B. A., Yuce, M., Lucas, S., Vrána, J., Burešová, V., Doležel, J., et al. (2015). Molecular organization and comparative analysis of chromosome 5B of the wild wheat ancestor Triticum dicoccoides. Sci. Rep. 5, 10763. doi: 10.1038/srep10763
Arrigo, N., Felber, F., Parisod, C., Buerki, S., Alvarez, N., David, J., et al. (2010). Origin and expansion of the allotetraploid Aegilops geniculata, a wild relative of wheat. New Phytol. 187, 1170–1180. doi: 10.1111/j.1469-8137.2010.03328.x
Badaeva, E. D., Amosova, A. V., Samatadze, T. E., Zoshchuk, S. A., Shostak, N. G., Chikida, N. N., et al. (2004). Genome differentiation in Aegilops. 4. evolution of the U-genome cluster. Plant Syst. Evol. 246, 45–76. doi: 10.1007/s00606-003-0072-4
Bansal, M., Adamski, N. M., Toor, P. I., Kaur, S., Molnár, I., Holušová, K., et al. (2020). Aegilops umbellulata introgression carrying leaf rust and stripe rust resistance genes Lr76 and Yr70 located to 9.47-Mb region on 5DS telomeric end through a combination of chromosome sorting and sequencing. Theor. Appl. Genet. 133, 903–915. doi: 10.1007/s00122-019-03514-x
Bedbrook, J. R., Jones, J., O’Dell, M., Thompson, R. D., Flavell, R. B. (1980). A molecular description of telomeric heterochromatin in Secale species. Cell 19, 545–560. doi: 10.1016/0092-8674(80)90529-2
Bennett, M. D., Leitch, I. J. (2011). Nuclear DNA amounts in angiosperms: targets, trends and tomorrow. Ann. Bot. 107, 467–590. doi: 10.1093/aob/mcq258
Cifuentes, M., Benavente, E. (2009). Wheat-alien metaphase I pairing of individual wheat genomes and D genome chromosomes in interspecific hybrids between Triticum Aestivum l. and Aegilops geniculata Roth. Theor. Appl. Genet. 119, 805–813. doi: 10.1007/s00122-009-1090-6
Cifuentes, M., Blein, M., Benavente, E. (2006). A cytomolecular approach to assess the potential of gene transfer from a crop (Triticum turgidum l.) to a wild relative (Aegilops geniculata roth.). Theor. Appl. Genet. 112, 657–664. doi: 10.1007/s00122-005-0168-z
Darko, E., Khalil, R., Dobi, Z., Kovács, V., Szalai, G., Janda, T., et al. (2020). Addition of Aegilops biuncialis chromosomes 2M or 3M improves the salt tolerance of wheat in different way. Sci. Rep. 10, 22327. doi: 10.1038/s41598-020-79372-1
Doležel, J., Kubaláková, M., Paux, E., Bartoš, J., Feuillet, C. (2007). Chromosome-based genomics in the cereals. Chromosome Res. 15, 51–66. doi: 10.1007/s10577-006-1106-x
Doležel, J., Lucretti, S. (1995). High-resolution flow karyotyping and chromosome sorting in Vicia faba lines with standard and reconstructed karyotypes. Theor. Appl. Genet. 90, 797–802. doi: 10.1007/BF00222014
Doležel, J., Lucretti, S., Molnár, I., Cápal, P., Giorgi, D. (2021). Chromosome analysis and sorting. Cytometry Part A 99, 328–342. doi: 10.1002/cyto.a.24324
Doležel, J., Vrána, J., Cápal, P., Kubaláková, M., Burešová, V., Šimková, H. (2014). Advances in plant chromosome genomics. Biotechnol. Adv. 32, 122–136. doi: 10.1016/j.biotechadv.2013.12.011
Doležel, J., Vrána, J., Safář, J., Bartoš, J., Kubaláková, M., Šimková, H. (2012). Chromosomes in the flow to simplify genome analysis. Funct. Integr. Genomics 12, 397–416. doi: 10.1007/s10142-012-0293-0
Dulai, S., Molnár, I., Szopkó, D., Darkó, É., Vojtkó, A., Sass-Gyarmati, A., et al. (2014). Wheat-Aegilops biuncialis Amphiploids have efficient photosynthesis and biomass production during osmotic stress. J. Plant Physiol. 171, 509–517. doi: 10.1016/j.jplph.2013.11.015
Eilam, T., Anikster, Y., Millet, E., Manisterski, J., Feldman, M. (2008). Nuclear DNA amount and genome downsizing in natural and synthetic allopolyploids of the genera Aegilops and Triticum. Genome 51, 616–627. doi: 10.1139/G08-043
Ekmekci, Y., Terzioglu, S. (2002). Changes in the electrophoretic pattern of soluble shoot proteins of wild and cultivated tetraploid wheats following cold acclimation and freezing. Israel J. Plant Sci. 50, 95–102. doi: 10.1560/DXB5-VHCC-LQCF-PUM4
Farkas, A., Molnár, I., Dulai, S., Rapi, S., Oldal, V., Cseh, A., et al. (2014). Increased micronutrient content (Zn, Mn) in the 3Mb(4B) wheat – Aegilops biuncialis substitution and 3Mb.4BS translocation identified by GISH and FISH. Genome 57, 61–67. doi: 10.1139/gen-2013-0204
Feldman, M., Levy, A. A. (2015)“Origin and evolution of wheat and related Triticeae species,”. In: Alien introgression in wheat: Cytogenetics, molecular biology, and genomics (Cham: Springer International Publishing). doi: 10.1007/978-3-319-23494-6_2 (Accessed January 30, 2019).
Feuillet, C., Langridge, P., Waugh, R. (2008). Cereal breeding takes a walk on the wild side. Trends Genet. 24, 24–32. doi: 10.1016/j.tig.2007.11.001
Friebe, B., Jiang, J., Raupp, W. J., McIntosh, R. A., Gill, B. S. (1996). Characterization of wheat-alien translocations conferring resistance to diseases and pests: Current status. Euphytica 91, 59–87. doi: 10.1007/bf00035277
Friebe, B. R., Tuleen, N. A., Gill, B. S. (1999). Development and identification of a complete set of Triticum Aestivum - Aegilops geniculata chromosome addition lines. Genome 42, 374–380. doi: 10.1139/g99-011
Gerlach, W. L., Bedbrook, J. R. (1979). Cloning and characterization of ribosomal RNA genes from wheat and barley. Nucleic Acids Res. 7, 1869–1885. doi: 10.1093/nar/7.7.1869
Gill, B. S., Sharma, H. C., Raupp, W. J., Browder, L. E., Hatchett, J. H., Harvey, T. L., et al. (1985). Evaluation of Aegilops species for resistance to wheat powdery mildew, wheat leaf rust, hessian fly, and greenbug. Plant Dis. 69, 314–316. doi: 10.1094/PD-69-314
Giorgi, D., Farina, A., Grosso, V., Gennaro, A., Ceoloni, C., Lucretti, S. (2013). FISHIS: Fluorescence In situ hybridization in suspension and chromosome flow sorting made easy. PloS One 8, e57994. doi: 10.1371/journal.pone.0057994
Grosso, V., Farina, A., Gennaro, A., Giorgi, D., Lucretti, S. (2012). Flow sorting and molecular cytogenetic identification of individual chromosomes of Dasypyrum villosum l. (H. villosa) by a single DNA probe. PloS One 7, e50151. doi: 10.1371/journal.pone.0050151
International Barley Genome Sequencing Consortium, Mayer, K. F. X., Waugh, R., Brown, J. W. S., Schulman, A., Langridge, P., et al. (2012). A physical, genetic and functional sequence assembly of the barley genome. Nature 491, 711–716. doi: 10.1038/nature11543
International Wheat Genome Sequencing Consortium (IWGSC) (2014). A chromosome-based draft sequence of the hexaploid bread wheat (Triticum Aestivum) genome. Science 345, 1251788. doi: 10.1126/science.1251788
International Wheat Genome Sequencing Consortium (IWGSC). (2018). Shifting the limits in wheat research and breeding using a fully annotated reference genome. Science 361, eaar7191. doi: 10.1126/science.aar7191
Ivanizs, L., Marcotuli, I., Rakszegi, M., Kalapos, B., Szőke-Pázsi, K., Farkas, A., et al. (2022). Identification of new QTLs for dietary fiber content in Aegilops biuncialis. Int. J. Mol. Sci. 23, 3821. doi: 10.3390/ijms23073821
Ivanizs, L., Monostori, I., Farkas, A., Megyeri, M., Mikó, P., Türkösi, E., et al. (2019). Unlocking the genetic diversity and population structure of a wild gene source of wheat, Aegilops biuncialis vis., and its relationship with the heading time. Front. Plant Sci. 10. doi: 10.3389/fpls.2019.01531
Kapustová, V., Tulpová, Z., Toegelová, H., Novák, P., Macas, J., Karafiátová, M., et al. (2019). The dark matter of large cereal genomes: Long tandem repeats. Int. J. Mol. Sci. 20, 2483. doi: 10.3390/ijms20102483
Kilian, B., Mammen, K., Millet, E., Sharma, R., Graner, A., Salamini, F., et al. (2011)“Aegilops,”. In: Wild crop relatives: Genomic and breeding resources (New York, NY: Spring-Verlag). Available at: https://www.academia.edu/19236227/Aegilops (Accessed August 20, 2020).
Kubaláková, M., Kovářová, P., Suchánková, P., Číhalíková, J., Bartoš, J., Lucretti, S., et al. (2005). Chromosome sorting in tetraploid wheat and its potential for genome analysis. Genetics 170, 823–829. doi: 10.1534/genetics.104.039180
Kubaláková, M., Macas, J., Doležel, J. (1997). Mapping of repeated DNA sequences in plant chromosomes by PRINS and c-PRINS. Theor. Appl. Genet. 94, 758–763. doi: 10.1007/s001220050475
Kubaláková, M., Valárik, M., Bartoš, J., Vrána, J., Číhalíková, J., Molnár-Láng, M., et al. (2003). Analysis and sorting of rye (Secale cereale L.) chromosomes using flow cytometry. Genome 46, 893–905. doi: 10.1139/g03-054
Kubaláková, M., Vrána, J., Číhalíková, J., Šimková, H., Doležel, J. (2002). Flow karyotyping and chromosome sorting in bread wheat (Triticum Aestivum l.). Theor. Appl. Genet. 104, 1362–1372. doi: 10.1007/s00122-002-0888-2
Kuraparthy, V., Sood, S., Gill, B. S. (2009). Molecular genetic description of the cryptic wheat-Aegilops geniculata introgression carrying rust resistance genes Lr57 and Yr40 using wheat ESTs and synteny with rice. Genome 52, 1025–1036. doi: 10.1139/G09-076
Leitch, I. J., Hanson, L., Lim, K. Y., Kovarik, A., Chase, M. W., Clarkson, J. J., et al. (2008). The ups and downs of genome size evolution in polyploid species of Nicotiana (Solanaceae). Ann. Bot. 101, 805–814. doi: 10.1093/aob/mcm326
Li, G., Zhang, T., Yu, Z., Wang, H., Yang, E., Yang, Z. (2021). An efficient oligo-FISH painting system for revealing chromosome rearrangements and polyploidization in Triticeae. Plant J. 105, 978–993. doi: 10.1111/tpj.15081
Lysák, M. A., Číhalíková, J., Kubaláková, M., Šimková, H., Künzel, G., Doležel, J. (1999). Flow karyotyping and sorting of mitotic chromosomes of barley (Hordeum vulgare l.). Chromosome Res. 7, 431–444. doi: 10.1023/A:1009293628638
Marcotuli, I., Colasuonno, P., Cutillo, S., Simeone, R., Blanco, A., Gadaleta, A. (2019). β-glucan content in a panel of Triticum and Aegilops genotypes. Genet. Resour Crop Evol. 66, 897–907. doi: 10.1007/s10722-019-00753-1
Martis, M. M., Zhou, R., Haseneyer, G., Schmutzer, T., Vrána, J., Kubaláková, M., et al. (2013). Reticulate evolution of the rye genome. Plant Cell 25, 3685–3698. doi: 10.1105/tpc.113.114553
Mascher, M., Gundlach, H., Himmelbach, A., Beier, S., Twardziok, S. O., Wicker, T., et al. (2017). A chromosome conformation capture ordered sequence of the barley genome. Nature 544, 427–433. doi: 10.1038/nature22043
Mayer, K. F. X., Martis, M., Hedley, P. E., Šimková, H., Liu, H., Morris, J. A., et al. (2011). Unlocking the barley genome by chromosomal and comparative genomics. Plant Cell 23, 1249–1263. doi: 10.1105/tpc.110.082537
Mayer, K. F. X., Taudien, S., Martis, M., Šimková, H., Suchánková, P., Gundlach, H., et al. (2009). Gene content and virtual gene order of barley chromosome 1H. Plant Physiol. 151, 496–505. doi: 10.1104/pp.109.142612
Molnár, I., Cifuentes, M., Schneider, A., Benavente, E., Molnár-Láng, M. (2011a). Association between simple sequence repeat-rich chromosome regions and intergenomic translocation breakpoints in natural populations of allopolyploid wild wheats. Ann. Bot. 107, 65–76. doi: 10.1093/aob/mcq215
Molnár, I., Gáspár, L., Sárvári, É., Dulai, S., Hoffmann, B., Molnár-Láng, M., et al. (2004). Physiological and morphological responses to water stress in Aegilops biuncialis and Triticum Aestivum genotypes with differing tolerance to drought. Funct. Plant Biol. 31, 1149–1159. doi: 10.1071/FP03143
Molnár, I., Kubaláková, M., Šimková, H., Cseh, A., Molnár-Láng, M. (2011b). Chromosome isolation by flow sorting in Aegilops umbellulata and Ae. comosa and their allotetraploid hybrids Ae. biuncialis and Ae. geniculata. PloS One 6, e27708. doi: 10.1371/journal.pone.0027708
Molnár, I., Kubaláková, M., Šimková, H., Farkas, A., Cseh, A., Megyeri, M., et al. (2014). Flow cytometric chromosome sorting from diploid progenitors of bread wheat, t. urartu, Ae. speltoides and Ae. tauschii. Theor. Appl. Genet. 127, 1091–1104. doi: 10.1007/s00122-014-2282-2
Molnár, I., Šimková, H., Leverington-Waite, M., Goram, R., Cseh, A., Vrána, J., et al. (2013). Syntenic relationships between the U and M genomes of Aegilops, wheat and the model species brachypodium and rice as revealed by COS markers. PloS One 8, e70844. doi: 10.1371/journal.pone.0070844
Molnár, I., Vrána, J., Burešová, V., Cápal, P., Farkas, A., Darkó, É., et al. (2016). Dissecting M, S and C genomes of wild relatives of bread wheat (Aegilops spp.) into chromosomes and exploring their synteny with wheat. Plant J. 88, 452–467. doi: 10.1111/tpj.13266
Molnár, I., Vrána, J., Farkas, A., Kubaláková, M., Cseh, A., Molnár-Láng, M., et al. (2015). Flow sorting of C-genome chromosomes from wild relatives of wheat Aegilops markgrafii, Ae. triuncialis and Ae. cylindrica, and their molecular organization. Ann. Bot. 116, 189–200. doi: 10.1093/aob/mcv073
Nagaki, K., Tsujimoto, H., Isono, K., Sasakuma, T. (1995). Molecular characterization of a tandem repeat, Afa family, and its distribution among Triticeae. Genome 38, 479–486. doi: 10.1139/g95-063
Ochoa, V., Madrid, E., Said, M., Rubiales, D., Cabrera, A. (2015). Molecular and cytogenetic characterization of a common wheat-Agropyron cristatum chromosome translocation conferring resistance to leaf rust. Euphytica 201, 89–95. doi: 10.1007/s10681-014-1190-5
Olivera, P. D., Rouse, M. N., Jin, Y. (2018). Identification of new sources of resistance to wheat stem rust in Aegilops spp. in the tertiary genepool of wheat. Front. Plant Sci. 9. doi: 10.3389/fpls.2018.01719
Ozkan, H., Tuna, M., Arumuganathan, K. (2003). Nonadditive changes in genome size during allopolyploidization in the wheat (Aegilops-Triticum) group. J. Heredity 94, 260–264. doi: 10.1093/jhered/esg053
Pradhan, G. P., Prasad, P. V. V., Fritz, A. K., Kirkham, M. B., Gill, B.S (2012). Response of Aegilops species to drought stress during reproductive stages of development. Funct. Plant Biol. 39, 51–59. doi: 10.1071/FP11171
Qi, L., Friebe, B., Zhang, P., Gill, B. S. (2007). Homoeologous recombination, chromosome engineering and crop improvement. Chromosome Res. 15, 3–19. doi: 10.1007/s10577-006-1108-8
Rakszegi, M., Molnár, I., Lovegrove, A., Darkó, É., Farkas, A., Láng, L., et al. (2017). Addition of Aegilops U and M chromosomes affects protein and dietary fiber content of wholemeal wheat flour. Front. Plant Sci. 8. doi: 10.3389/fpls.2017.01529
Šafář, J., Bartoš, J., Janda, J., Bellec, A., Kubaláková, M., Valárik, M., et al. (2004). Dissecting large and complex genomes: flow sorting and BAC cloning of individual chromosomes from bread wheat. Plant J. 39, 960–968. doi: 10.1111/j.1365-313X.2004.02179.x
Said, M., Holušová, K., Farkas, A., Ivanizs, L., Gaál, E., Cápal, P., et al. (2021). Development of DNA markers from physically mapped loci in Aegilops comosa and Aegilops umbellulata using single-gene FISH and chromosome sequences. Front. Plant Sci. 12. doi: 10.3389/fpls.2021.689031
Said, M., Hřibová, E., Danilova, T. V., Karafiátová, M., Čížková, J., Friebe, B., et al. (2018). The Agropyron cristatum karyotype, chromosome structure and cross-genome homoeology as revealed by fluorescence in situ hybridization with tandem repeats and wheat single-gene probes. Theor. Appl. Genet. 131, 2213–2227. doi: 10.1007/s00122-018-3148-9
Said, M., Kubaláková, M., Karafiátová, M., Molnár, I., Doležel, J., Vrána, J. (2019a). Dissecting the complex genome of crested wheatgrass by chromosome flow sorting. Plant Genome 12, 180096. doi: 10.3835/plantgenome2018.12.0096
Said, M., Parada, A. C., Gaál, E., Molnár, I., Cabrera, A., Doležel, J., et al. (2019b). Uncovering homeologous relationships between tetraploid Agropyron cristatum and bread wheat genomes using COS markers. Theor. Appl. Genet. 132, 2881–2898. doi: 10.1007/s00122-019-03394-1
Sánchez-Martín, J., Steuernagel, B., Ghosh, S., Herren, G., Hurni, S., Adamski, N., et al. (2016). Rapid gene isolation in barley and wheat by mutant chromosome sequencing. Genome Biol. 17, 221. doi: 10.1186/s13059-016-1082-1
Schneider, A., Linc, G., Molnár, I., Molnár-Láng, M. (2005). Molecular cytogenetic characterization of Aegilops biuncialis and its use for the identification of 5 derived wheat-Aegilops biuncialis disomic addition lines. Genome 48, 1070–1082. doi: 10.1139/g05-062
Schneider, A., Molnár-Láng, M. (2009). Detection of the 1RS chromosome arm in martonvásár wheat genotypes containing 1Bl.1Rs or 1Al.1Rs translocations using SSR and STS markers. Acta Agronomica. Hungarica. 57, 409–416. doi: 10.1556/AAgr.57.2009.4.3
Schneider, A., Molnár, I., Molnár-Láng, M. (2008). Utilisation of Aegilops (goatgrass) species to widen the genetic diversity of cultivated wheat. Euphytica 163, 1–19. doi: 10.1007/s10681-007-9624-y
Senerchia, N., Felber, F., Parisod, C. (2014). Contrasting evolutionary trajectories of multiple retrotransposons following independent allopolyploidy in wild wheats. New Phytol. 202, 975–985. doi: 10.1111/nph.12731
Šimková, H., Šafář, J., Suchánková, P., Kovářová, P., Bartoš, J., Kubaláková, M., et al. (2008a). A novel resource for genomics of Triticeae: BAC library specific for the short arm of rye (Secale cereale l.) chromosome 1R (1RS). BMC Genomics 9, 237. doi: 10.1186/1471-2164-9-237
Šimková, H., Svensson, J. T., Condamine, P., Hřibová, E., Suchánková, P., Bhat, P. R., et al. (2008b). Coupling amplified DNA from flow-sorted chromosomes to high-density SNP mapping in barley. BMC Genomics 9, 294. doi: 10.1186/1471-2164-9-294
Staňková, H., Hastie, A. R., Chan, S., Vrána, J., Tulpová, Z., Kubaláková, M., et al. (2016). BioNano genome mapping of individual chromosomes supports physical mapping and sequence assembly in complex plant genomes. Plant Biotechnol. J. 14, 1523–1531. doi: 10.1111/pbi.12513
Steadham, J., Schulden, T., Kalia, B., Koo, D.-H., Gill, B. S., Bowden, R., et al. (2021). An approach for high-resolution genetic mapping of distant wild relatives of bread wheat: example of fine mapping of Lr57 and Yr40 genes. Theor. Appl. Genet. 134, 2671–2686. doi: 10.1007/s00122-021-03851-w
Tanksley, S. D., McCouch, S. R. (1997). Seed banks and molecular maps: Unlocking genetic potential from the wild. Science 277, 1063–1066. doi: 10.1126/science.277.5329.1063
Thind, A. K., Wicker, T., Šimková, H., Fossati, D., Moullet, O., Brabant, C., et al. (2017). Rapid cloning of genes in hexaploid wheat using cultivar-specific long-range chromosome assembly. Nat. Biotechnol. 35, 793–796. doi: 10.1038/nbt.3877
Tiwari, V. K., Wang, S., Danilova, T., Koo, D. H., Vrána, J., Kubaláková, M., et al. (2015). Exploring the tertiary gene pool of bread wheat: Sequence assembly and analysis of chromosome 5Mg of Aegilops geniculata. Plant J. 84, 733–746. doi: 10.1111/tpj.13036
Tiwari, V. K., Wang, S., Sehgal, S., Vrána, J., Friebe, B., Kubaláková, M., et al. (2014). SNP discovery for mapping alien introgressions in wheat. BMC Genomics 15, 273. doi: 10.1186/1471-2164-15-273
van Slageren, M. W. S. J. M. (1994). Wild wheats: a monograph of Aegilops l. and Amblyopyrum (Jaub. & spach) eig (Poaceae): a revision of all taxa closely related to wheat, excluding wild Triticum species, with notes on other genera in the tribe Triticcae, especially Triticum (Wageningen, The Netherlands; Aleppo, Syria: Wageningen Agricultural University; International Center for Agricultural Research in the Dry Areas).
Vrána, J., Cápal, P., Číhalíková, J., Kubaláková, M., Doležel, J. (2016a). Flow sorting plant chromosomes. Methods Mol. Biol. 1429, 119–134. doi: 10.1007/978-1-4939-3622-9_10
Vrána, J., Cápal, P., Šimková, H., Karafiátová, M., Čížková, J., Doležel, J. (2016b). Flow analysis and sorting of plant chromosomes. Curr. Protoc. Cytometry 78, 5.3.1–5.3.43. doi: 10.1002/cpcy.9
Vrána, J., Kubaláková, M., Číhalíková, J., Valárik, M., Doležel, J. (2015). Preparation of sub-genomic fractions enriched for particular chromosomes in polyploid wheat. Biol. Plant 59, 445–455. doi: 10.1007/s10535-015-0522-1
Vrána, J., Kubaláková, M., Šimková, H., Číhalíková, J., Lysák, M. A., Doležel, J. (2000). Flow sorting of mitotic chromosomes in common wheat (Triticum Aestivum l.). Genetics 156, 2033–2041. doi: 10.1093/genetics/156.4.2033
Xing, L., Hu, P., Liu, J., Witek, K., Zhou, S., Xu, J., et al. (2018). Pm21 from haynaldia villosa encodes a CC-NBS-LRR protein conferring powdery mildew resistance in wheat. Mol. Plant 11, 874–878. doi: 10.1016/j.molp.2018.02.013
Xing, L., Yuan, L., Lv, Z., Wang, Q., Yin, C., Huang, Z., et al. (2021). Long-range assembly of sequences helps to unravel the genome structure and small variation of the wheat–Haynaldia villosa translocated chromosome 6VS.6AL. Plant Biotechnol. J. 19, 1567–1578. doi: 10.1111/pbi.13570
Yu, G., Matny, O., Champouret, N., Steuernagel, B., Moscou, M. J., Hernández-Pinzón, I., et al. (2022). Aegilops sharonensis genome-assisted identification of stem rust resistance gene Sr62. Nat. Commun. 13, 1607. doi: 10.1038/s41467-022-29132-8
Zhang, Z., Song, L., Han, H., Zhou, S., Zhang, J., Yang, X., et al. (2017). Physical localization of a locus from Agropyron cristatum conferring resistance to stripe rust in common wheat. Int. J. Mol. Sci. 18, 2403. doi: 10.3390/ijms18112403
Zwyrtková, J., Blavet, N., Doležalová, A., Cápal, P., Said, M., Molnár, I., et al. (2022). Draft sequencing crested wheatgrass chromosomes identified evolutionary structural changes and genes and facilitated the development of SSR markers. Int. J. Mol. Sci. 23, 3191. doi: 10.3390/ijms23063191
Keywords: Aegilops biuncialis, Aegilops geniculata, flow karyotyping, chromosome flow sorting, genome dissecting
Citation: Said M, Cápal P, Farkas A, Gaál E, Ivanizs L, Friebe B, Doležel J and Molnár I (2022) Flow karyotyping of wheat-Aegilops additions facilitate dissecting the genomes of Ae. biuncialis and Ae. geniculata into individual chromosomes. Front. Plant Sci. 13:1017958. doi: 10.3389/fpls.2022.1017958
Received: 12 August 2022; Accepted: 09 September 2022;
Published: 03 October 2022.
Edited by:
Azahara Carmen Martin, John Innes Centre, United KingdomReviewed by:
Tingdong Li, Northwest A&F University, ChinaZujun Yang, University of Electronic Science and Technology of China, China
Michał Tomasz Kwiatek, Poznan University of Life Sciences, Poland
Ekaterina D. Badaeva, Russian Academy of Sciences, Russia
Copyright © 2022 Said, Cápal, Farkas, Gaál, Ivanizs, Friebe, Doležel and Molnár. This is an open-access article distributed under the terms of the Creative Commons Attribution License (CC BY). The use, distribution or reproduction in other forums is permitted, provided the original author(s) and the copyright owner(s) are credited and that the original publication in this journal is cited, in accordance with accepted academic practice. No use, distribution or reproduction is permitted which does not comply with these terms.
*Correspondence: Mahmoud Said, c2FpZEB1ZWIuY2FzLmN6
†ORCID: Mahmoud Said, orcid.org/0000-0002-6169-8655
Petr Cápal, orcid.org/0000-0002-8888-9101
András Farkas, orcid.org/0000-0002-9545-0332
Eszter Gaál, orcid.org/0000-0002-1081-9656
László Ivanizs, orcid.org/0000-0003-0887-9729
Brend Friebe, orcid.org/0000-0002-5419-1565
Jaroslav Doležel, orcid.org/0000-0002-6263-0492
István Molnár, orcid.org/0000-0002-7167-9319