- 1Department of Botany, MMV, Banaras Hindu University, Varanasi, India
- 2Department of Botany, School of Basic and Applied Sciences, Central University of Punjab, Bathinda, Punjab, India
- 3Department of Environmental Science, School of Earth Sciences, Central University of Rajasthan, Ajmer, Rajasthan, India
Salinity stress is one of the significant abiotic stresses that influence critical metabolic processes in the plant. Salinity stress limits plant growth and development by adversely affecting various physiological and biochemical processes. Enhanced generation of reactive oxygen species (ROS) induced via salinity stress subsequently alters macromolecules such as lipids, proteins, and nucleic acids, and thus constrains crop productivity. Due to which, a decreasing trend in cultivable land and a rising world population raises a question of global food security. In response to salt stress signals, plants adapt defensive mechanisms by orchestrating the synthesis, signaling, and regulation of various osmolytes and phytohormones. Under salinity stress, osmolytes have been investigated to stabilize the osmotic differences between the surrounding of cells and cytosol. They also help in the regulation of protein folding to facilitate protein functioning and stress signaling. Phytohormones play critical roles in eliciting a salinity stress adaptation response in plants. These responses enable the plants to acclimatize to adverse soil conditions. Phytohormones and osmolytes are helpful in minimizing salinity stress-related detrimental effects on plants. These phytohormones modulate the level of osmolytes through alteration in the gene expression pattern of key biosynthetic enzymes and antioxidative enzymes along with their role as signaling molecules. Thus, it becomes vital to understand the roles of these phytohormones on osmolyte accumulation and regulation to conclude the adaptive roles played by plants to avoid salinity stress.
Introduction
Soil is a complicated system in which physical and biological processes interact. Various natural and anthropogenic activities influences climate change that leads to disturbed physical and chemical characteristics of soil (Pandey and Choudhary, 2019; Ahirwal et al., 2021). Among environmental changes, soil salinization has been known as a severe threat to agricultural fields (Mukhopadhyay et al., 2021). Approximately 20% of the world’s irrigated land [about 60 million (Mha)] has been globally affected due to salinity (FAO and ITPS, 2015), and with continuous climate change, it is projected to increase to 50% by 2050 (Machado and Serralheiro, 2017; Abdelrahman et al., 2018). In addition to this, FAO has reported that increasing soil salinity could pull 0.3–1.5 million hectares agricultural land out of production each year, reducing yield potential by 20–46 million hectares (FAO and ITPS, 2015). Therefore, crops cannot be cultivated if soil salinity is not controlled and rises above specified salinity thresholds (FAO, 2017). Among abiotic stresses, salinity affects plant growth by hampering photosynthesis, CO2 assimilation and excessive ROS production (Chaudhary and Choudhary, 2021; ElSayed et al., 2021; Selem et al., 2022). The detrimental effects of salinity begin with osmotic or water stress (a reduction in the root’s ability to absorb water), followed by ionic toxicity (nutritional imbalance, formation of ROS species), hormonal imbalance and susceptibility to infection by the pathogen (Choudhary et al., 2017; Talbi et al., 2018).
Plants respond to salinity by adapting diverse strategies such as phytohormonal regulation, redox change in potential, osmolyte biosynthesis, and epigenetic control of stress-related genes during stressful conditions. Similarly, salt stress tolerance is a complex trait that includes various signaling pathways, transcription factors, stress-responsive genes (Mansour and Hassan, 2022). However, tolerance to salinity levels varies between plant species, and such plants can be categorized as halophytes or glycophytes. Halophytes are the plants that are endowed with the ability to tolerate salinity up to 200 mM over glycophytes that are salt sensitive under adverse effects of salinity (Flowers and Colmer, 2008; Santos et al., 2016; Song et al., 2016). This salt tolerance mechanism in halophytes involves the reduction of Na+ influx, Na+ compartmentalization, and efflux of Na+ ions (Flowers and Colmer, 2008). In addition to this, ROS scavenging via antioxidant enzymes or quenching them with non-enzymatic molecules such as carotenoids, flavonoids, reduced glutathione, ascorbic acid and compatible osmolytes such as proline, glycine betaine (GB), trehalose sugar (Khan et al., 2015; Per et al., 2018) provide tolerance against salinity stress in the plant (Anjum et al., 2017). Osmolytes majorly contribute to maintaining cellular osmotic adjustment through cell turgidity, protects internal cell components and reduced ionic toxicity.
However, multiple pathways have been explored in the biosynthesis of osmolytes in bacteria as well as in plants. In addition to this, phytohormones undoubtedly regulate osmolyte production and accumulation (Per et al., 2017). Phytohormones interact synergistically with osmolytes and bring tolerance to stress (Iqbal et al., 2014). However, the comprehensive role of phytohormones by modulating the biosynthesis of osmolytes has not been explored properly. Therefore, it becomes imperative to understand the underlying mechanism of phytohormones regulating the biosynthesis of osmolytes. Thus, in this present review, we have focused on the synthesis and role of osmolytes in plants and further their modulation via phytohormones under salinity stress.
Impact of salinity stress on plant growth
The term “Salinity” refers to the presence of an excessive amount of soluble salts in the soil that hinders plant growth (Etikala et al., 2021). High salinity is one of the major abiotic stress that is most widely distributed around the globe (Chaudhry and Sidhu, 2021). Since salinity is one of the stringent problems, it can be categorized as Primary and Secondary salinity. Primary salinity occurs in arid and semi-arid climatic zones due to natural, anthropogenic activities and Secondary salinity occur directly as a consequence of man-made activities (Safdar et al., 2019). The detrimental effect of salinity on crop growth is due to changes in physiological, morphological, biochemical and molecular responses in plant growth (Arif et al., 2020; Figure 1). Inhibitory effects of salt stress is influenced by number of factors including salt content, duration of exposure, plant species and varieties, photochemical quenching capability, plant growth stages, stress type, gas exchange characteristics, photosynthetic pigments, and ambient conditions (Shahverdi et al., 2018). At low levels of soil salinity, it enhances the plant length as concluded in various studies on various crops such as Zea mays (Hamada, 1995), Oryza sativa L. (Lee et al., 2011), Vigna unguiculata L. (Ibrahim, 2016), Brassica campestris L. (Memon et al., 2010) and Vicia faba L. (Hanafy et al., 2013). Higher sodium chloride salt concentrations, on the other hand, lowered the height of Vigna mungo L. (Kapoor and Srivastava, 2010), and Tanacetum parthenium L. (Mallahi et al., 2018) plants. Salt stress affects root and stem growth, and hinders nutrient uptake and translocation (Shrivastava and Kumar, 2015). Reduction in the plant growth is mainly due to decreased chlorophyll content which leads to the reduction in photosynthetic capacity of the plants under salinity stress (Netondo et al., 2004). In the context of plant growth, a recent detailed study on tomato with different salt concentrations (75,150 and 300 mM) exhibited a reduction in fresh and dry weight of roots (86.5% and 78.6%), shoot (71% and 72%), chlorophyll and carotenoid contents (22, 18.6%), and anthocyanin (41.1%), respectively (Alzahib et al., 2021). However, at a relatively higher concentration, 300 mM NaCl, reported an increase in proline content (67.37 mg g-1 fresh weight), antioxidant enzymes such as Superoxide dismutase (SOD), and Catalase (CAT), while reduction in malondialdehyde (MDA) content. An increased salt concentration alters the morphology, physiology and metabolism in these landraces in response to salt stress (Alzahib et al., 2021). Similarly, in Medicago truncatula, the effect of salinity on photosynthesis and chlorophyll fluorescence was studied, and total chlorophyll content was significantly reduced by 43% in TN6.18 and only 6% in TN.8.20 compared to control plants. The same effect was reported with carotenoid content, reduced by 51% in the sensitive TN6.18 line but only 13% in the resistant line (Najar et al., 2019). These recent studies indicate that salinity drastically affects the growth and development of plants. Nutritive imbalance another factor of salt stress that disrupts the osmotic equilibrium and further prevails drought conditions (Riaz et al., 2019). Additional effects include hampering reproductive processes such as inhibiting microsporogenesis, promotion of rapid programmed cell death and senescence of fertilized embryos (Suo et al., 2017). TEM micrographs of Solanum melongena treated with 75, 100, and 150 mM NaCl exhibited bulging chloroplasts and an absence of integrated thylakoid membranes associated with big starch grains (Alkhatib et al., 2021). Salinity influences the antioxidant activity of enzymes. For example, in Andrographis paniculata alteration in the activity of antioxidant enzymes, viz. catalase (CAT) and peroxidase (POD) was observed that further reveals the extent of induced changes modulated by salinity stress (Kumar and Srivastava, 2018). In short, the impact of salinity stress has been illustrated in Figure 1.
Salinity stress tolerance in plants
To comprehend the physiological process of salinity tolerance in plants, one must first understand the cause of growth restriction, which can be due to salt’s osmotic impact on the soil or the toxic effect of salt within the plant body. As a result, plants respond to salinity in two ways: an initial rapid increase in external osmotic pressure at first followed by a gradual response as Na+ accumulates in leaves. In the first phase, plant roots sense the salt concentration above the threshold level, mainly 40 mM NaCl in many plants or less for sensitive plants like rice and Arabidopsis, and significantly decreases the rate of shoot growth. In the second ionic phase, the salt concentration increases excessively in the older leaves due to continuous transport to transpiring leaves over a longer period of time eventually results in higher salt concentration and then leaves die. As a result, if new leaves die faster than they are being produced, the plant’s photosynthetic capacity will no longer meet the carbohydrate requirements of young leaves, declining growth even more (Munns and Tester, 2008). Salinity tolerance is a physiological feature that is linked to a number of mechanisms that influences stress (Roy et al., 2014). However, based on the differential responses of plants, tolerance to salinity can be categorized into three types, viz. osmotic tolerance, ionic tolerance, and tissue tolerance (Chaudhary and Choudhary, 2021).
Osmotic stress tolerance
Osmotic stress tolerance initiates a rapid response by diminishing cell expansion of root tips and young leaves and decreasing stomatal conductance to preserve water. It uses quick, long-distance (root to shoot) signaling pathways (Roy et al., 2014; Isayenkov and Maathuis, 2019), which essentially ignores the osmotic effects of NaCl, KCl, mannitol, and polyethylene glycol (Hassini et al., 2017; Darko et al., 2019; Prodjinoto et al., 2021; Gul et al., 2022). In osmotic tolerance, both organic solutes and inorganic ions are essential. These low molecular weight organic solutes, such as sugar and sugar derivatives including sucrose, polyols, and heterosides, GB and homarine (tertiary nitrogen compounds), amino acids such as proline, glutamate are commonly found in higher plants. Although it was thought that crop species had a wide range of osmotic tolerance, this was difficult to evaluate until recently. The measurements of growth parameters such as leaf growth and stomatal conductance methods are usually time-consuming and destructive. Digital images of plants allow measurements of the plant’s relative growth rate immediately after exposure to salinity and, hence, measure osmotic tolerance. Rice (Al-Tamimi et al., 2016), barley (Tilbrook et al., 2017), durum wheat (James et al., 2008; Sirault et al., 2009), bread wheat (Asif et al., 2018), and wild relatives of wheat, such as T. monococcum, have shown variations in osmotic tolerance based on relative plant growth rate.
Ionic tolerance
The exclusion of ions, particularly Na+, from the shoot is a long-established mechanism for salinity tolerance in agricultural plants. This mechanism has received the most attention as it becomes easier to perform experimentation. Many crops, such as durum wheat (Forster, 2001; Munns and James, 2003), rice (Zhu et al., 2001; Lee et al., 2003), barley (Wei et al., 2003; Garthwaite et al., 2005) and Medicago (Sibole et al., 2003) have shown a substantial link between exclusion and tolerance of salt. In this mechanism, Na+ and Cl− enter the plant’s roots and are quickly transported to the shoot via transpiration stream. To avoid the buildup of these ions into the shoot system, roots exclude most of the Na+ and Cl− dissolved in the soil solution through which the concentration of salt in the shoot as a whole would never increase over that in soil, and the plant could survive indefinitely in saline soil. In this way, the concentration of Na+ and Cl− ions is relatively higher in shoot than in roots that, improve the plant’s salt tolerance. Lauchli et al. (2008) evaluated the concentration of sodium and other ions in different layers of wheat root. In addition to this, the SOS1 antiporter localized to the root epidermis (particularly at the root tip, where roots are undifferentiated) provides the first line of defense against sodium uptake (Assaha et al., 2017; Figure 2).
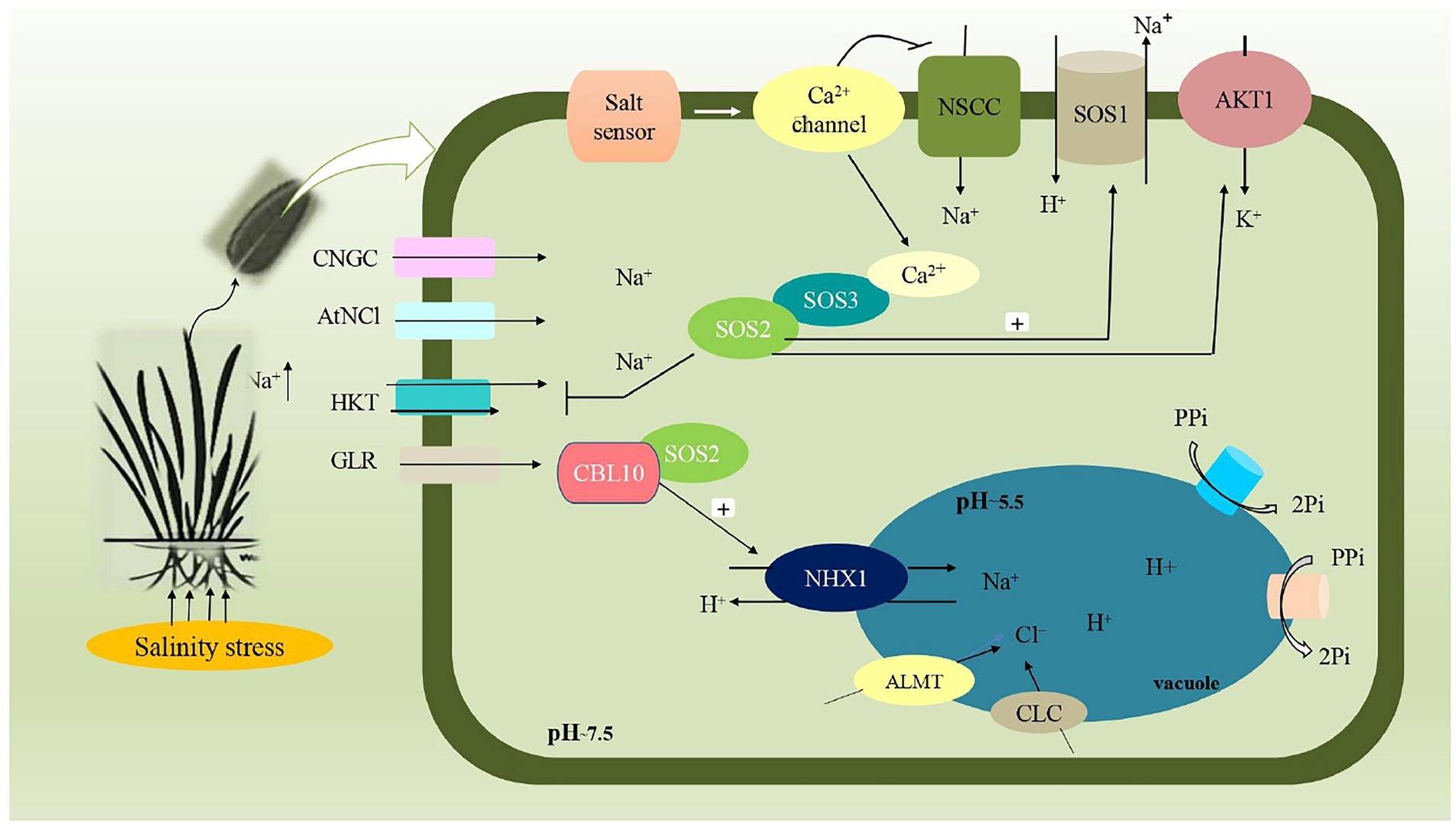
Figure 2. Schematic representation involved in salt overlay sensitive pathway (SOS) pathway during salinity stress. Plasma membrane of plant cell and organelle vacuolar membrane are involved in the transportation of Na+ ion. Apart from this, influx of Na+ into cells are mediated by ion transporters such as Cyclic-nucleotide gated channels (CNGs), Na2+/Ca2+ exchanger (AtNCl), High affinity K+ transporters (HKT), Glutamate receptors (GLR), Non-selective cation channels (NSCC). SOS1 (Na+/H+ antiporter), SOS2 (Serine/threonine protein kinase) and SOS3 (Calcium bindingprotein) are three genes commonly involved in this pathway that regulates the cytosolic concentration of Na+ ion, by subsequent extrusion and compartmentation into vacuoles. Calcineurin B-like (CBL10) along with SOS2 protein mediates the sequestration of Na+ ions into vacuole, through vacuolar membrane Na+/H+antiporter (NHX1), thereby maintaining ion homeostasis. Aluminum activated malate transporter (ALMT), Cation/chloride transporter (CLC) mediates the entry of Cl−ion into vacuole. Electrochemical potential is maintained by vacuolar H+-pyrophosphatase (AVP1) and H+-ATPase (V-ATPase).
Tissue tolerance
Tissue tolerance refers to a tissue’s ability to retain tissue function while accumulating high amounts of intracellular Na+ or Cl− ions (Munns et al., 2016; Negrāo et al., 2017). To avoid the detrimental effect of accumulated Na+ and Cl− ions, compartmentalization into vacuoles or photosynthetically non-active cells avoids the accumulation of Na+ and Cl− ions in the cytoplasm of plant cells where most important metabolic process occurs, i.e., tissue tolerance (Munns and Tester, 2008; Roy et al., 2014; Figure 2) and employing such mechanism will allow a plant to avoid toxicity. There is already a considerable amount of evidence across crop varieties in terms of the rates of accumulation of Na+ and Cl− in the shoots (Munns et al., 2016).
Major osmoprotectants in plants
Osmoprotectants are low molecular weight hydrophilic organic compounds that involve a variety of roles connected to plant defense mechanisms under varying environmental conditions. Unlike inorganic compounds, these compounds are non-toxic at higher cellular concentrations (Nahar et al., 2016; Niazian et al., 2021). During stressful conditions, these osmoprotectants accumulates in plants like proline, ectoine, trehalose, polyols, fructan, and quaternary ammonium compounds (QACs) such as glycinebetaine, alanine betaine, proline betaine, choline-O-sulfate, hydroxyproline betaine, and pipecolate betaine (Singh et al., 2015). Transgenic plants overexpressing biosynthetic enzymes for osmoprotectants, such as mannitol, GB, D-ononitol, or sorbitol, have accumulated these compounds in levels too low to give protective benefits solely through osmotic mass action (Huang et al., 2000).
The fundamental function of osmoprotectants accumulation in plants under salt stress is to maintain cell turgor pressure via osmoregulation, and protection of cellular components via reduction of ionic toxicity. Furthermore, by scavenging of hazardous ROS generated and preserving important antioxidative enzymes, these osmoprotective chemicals boost the antioxidative defense system in plants (Hasanuzzaman et al., 2014, 2019). In addition to this, osmolytes also function in the activation of defense-related genes under various stresses, which designates its prime importance in plants (Wani et al., 2018b). In the upcoming section, we discuss the role of important osmoprotectants under salinity stress in plants. Table 1 summarizes some of the relevant osmoprotectants in plants exposed to salinity stress.
Amino acids
Amino acids derived osmoprotectants such as proline, arginine, alanine, leucine, glycine, serine, valine and γ-aminobutyric acid (GABA; Suprasanna et al., 2014). These osmoprotectants accumulate under salinity stress conditions and decrease the osmotic potential of cells allowing water absorption. They also stabilize protein structures and membranes (Ashraf and Foolad, 2007); also act as nitrogen storing agents and ROS scavengers (Hayat et al., 2012). In stressed conditions, accumulation of mainly proline in relatively higher amounts as compared to other osmoprotectants is an indication of stress conditions. β-alanine being a non-proteinogenic amino acid is a stress response molecule involved in plant protection from biotic and abiotic stresses. Furthermore, it is converted into β-alanine betaine, an osmoprotective compound in some plant species (Parthasarathy et al., 2019).
Proline
Proline can be biosynthesized by two pathways: glutamate and ornithine pathway. Proline is produced from glutamatic acid via the intermediate Δ1-pyrroline-5-carboxylate (P5C), which is catalyzed by Δ1-pyrroline 5-carboxylate synthetase (P5CS) and Δ1-pyrroline5-carboxylate reductase (P5CR) in the glutamate pathway (Dar et al., 2016). However, in an alternate pathway, Proline is synthesized from ornithine (Orn), which is transaminated to Pyrroline-5- carboxylate (P5C) via Orn-δ-aminotransferase (δ-OAT; Verbruggen and Hermans, 2008). It has been claimed that proline buildup aids stress tolerance in various ways. It acts as a molecular chaperone, ensuring the integrity of proteins and enhancing enzyme activity (Ghosh et al., 2022). Recently, engineered plants have high expression of pyrroline-5-carboxylate reductase enzyme, leads to the accumulation of proline. Also, it has been observed that the antioxidant property of proline is helpful as ROS Scavenger (El-Badri et al., 2021). The importance of the Orn pathway in the development of rice seedlings has been explained through the constitutive expression of OsOAT genes. These genes are responsible for enhanced δ-OAT activity, improved antioxidant status, tolerance to drought, and osmotic stress (You et al., 2012). However, under salt stress, the preferential use of the Glu pathway over the Orn pathway is increased due to enhanced expression of P5CS activity. This suggests the pivotal role of Glu pathway in proline accumulation during osmotic adjustment (Zhen and Ma, 2009). Delauney et al. (1993) reported that P5CS mRNA levels were significantly up-regulated while OAT mRNA levels were down-regulated during salt stress in Vigna aconitifolia L. Later, it was confirmed by Lei et al. (2016) and Mansour and Ali (2017) through evaluation studies related to proline biosynthesis in salt stress. Interestingly, exogenous application of proline produced different results. For instance, Zea mays L. under foliar exposure of proline resulted in decreased P5CS activity and increased PDH activity under salt stress (de Freitas et al., 2018). Similar results were obtained in Sorghum bicolor under saline conditions (de Freitas et al., 2019). Seed primed with exogenous proline has been observed with decreased P5CS response while, on the other hand, PDH expression increased significantly in Triticum aestivum L. (Rady et al., 2019). These increased PDH level protect plants from proline toxicity (Deuschle et al., 2001). Over-expression of P5CS gene expression in Lepidium draba leads to proline accumulation and improved antioxidative responses under salt stress (Pakzad et al., 2021).
Quaternary ammonium compounds
Quaternary ammonium compounds like GB, β-alanine betaine, proline-betaine, choline-O-sulfate etc. (Koyro et al., 2012) accumulate under salt stress conditions. Among these compounds, GB generally gets accumulated in a more considerable amount as compared to others, mainly accumulated in the chloroplast. GB helps to maintain intracellular osmotic equilibrium by regulating water flow into the cells (Ranganayakulu et al., 2013). More benefits are reflected in terms of the protection to thylakoids membrane, maintenance of photosynthetic activity and stomatal conductance, and photorespiration reduction etc. Transgenic approaches by overexpressing Betaine aldehyde dehydrogenase (BADH) enzyme provide better tolerance under stressful conditions (Sulpice et al., 2003).
Glycine betaine
Glycine betaine (GB) is zwitterionic, neutral at physiological pH and a quaternary ammonium compound that is N-methylated derivative of glycine (Ashraf and Foolad, 2007). GB is widely synthesized in chloroplasts of young tissues protecting the membrane enzymes and proteins under stressful environmental conditions. Because GB is not actively destroyed or metabolized in plant tissues, its concentration is determined by synthesis, transport, and dilution of plants (Annunziata et al., 2019). GB is biosynthesized as spatio-temporal under abiotic stress conditions (Annunziata et al., 2019). Usually, it is synthesized at modest levels and gently rises in young tissues /organs when abiotic stress occurs. Furthermore, unlike proline, the GB is swiftly re-translocated to younger leaves even if it is exogenously supplied to older sections. Thus, it can be inferred that GB cannot be metabolized and plays a critical role in the protection of young tissues.
Under normal and stressful conditions, some plant species accumulate GB spontaneously (Chen and Murata, 2008) such as major cereals that are completely devoid of the potential to accumulate GB (Kurepin et al., 2015). An attempt to transfer GB biosynthetic genes in these plants via genetic engineering is considered a protective strategy for increasing salinity stress tolerance (Chen and Murata, 2011). Upregulation of the BADH gene has been reported as a potential biomarker in salt-stressed wheat plants (Lv et al., 2016). Similarly, Arabidopsis plants transformed with a novel BADH gene, ScBADH, resulted in the accumulation of SOD, proline and GB under salt stress (Wang et al., 2016). In another study, transgenic maize introduced with BADH gene from Artiplex micrantha L. reported higher GB content that is sufficient to impart tolerance against salt stress.
Similarly, japonica and rice cultivars mediate up-regulated expression of the BADH1 gene in salt stress (Fitzgerald et al., 2008). Thus, it could be inferred that BADH act as a positive regulator in the treatment of salt stress via MAPK pathway in plants. Furthermore, the codA gene (choline oxidase) isolated from Arthrobacter globiformis can potentially alleviate phosphate deficiency in tomato plants through GB action (Li et al., 2019). In addition, GB regulates ion channels and transporters by maintaining Na+/K+ levels that are helpful in the transportation of phosphate under salt-stressed conditions (Haruta and Sussman, 2012; Wei et al., 2017; Yuan et al., 2017).
Trimethylamine N-oxide
Plant osmolytes, besides enhancing osmotic homeostasis and stabilization, also act as chaperons in preserving the folding of proteins under stressful conditions and thus providing stability and function (Slama et al., 2015; Rabbani and Choi, 2018). In animals, TMAO is a quintessential osmolyte that act as a chaperone that retains the folding status of protein and protects against denaturants (Yancey, 2005; Strambini and Gonnelli, 2008; Jethva and Udgaonkar, 2018). Interestingly, a recent study demonstrated that the plant also synthesizes TMAO endogenously from FAOs and further illustrates that its level rises under abiotic stress conditions. Thus, TMAO enhances plant tolerance to freezing, drought, temperatures and high salt (Catala et al., 2021).
Sugars
During salt stress, carbohydrates such as sugars (e.g., glucose, fructose, trehalose) and starch accumulate (Parida et al., 2004). The significant role played by these sugars involves osmoprotectant and scavenging of ROS for mitigating salt stress. It has also been reported that with an increase in the level of salt stress, the level of reducing sugars (sucrose, fructans) also increases significantly (Kerepesi and Galiba, 2000; Gangola and Ramadoss, 2018).
Trehalose sugar
Trehalose (Tre) is a non-reducing sugar that is made up of two glucose residues (D-glucopyranose units) linked together by an extremely stable linkage (Ahluwalia, 2022). It’s a suitable solute or osmoprotectant even at high cellular concentrations because it’s non-reducing in nature and very soluble (Lunn et al., 2014).
Plants produce Tre through the trehalose-6-phosphate synthase/phosphatase (OtsA–OtsB) pathway, which is dependent on two essential molecules: uridine-diphospho-glucose (UDP-Glc) and glucose-6-phosphate (Glc-6-P) in a two-reaction process (Paul et al., 2008; Kosar et al., 2018). Tre functions as a protective molecule for cellular, membrane, and proteinaceous structures due to this particular characteristic (López-Gómez and Lluch, 2012; Abdallah et al., 2016). Tre preserves membrane and protein structures under stress by forming an amorphous glass structure and influencing the surrounding polar phospholipid head groups or amino acids via hydrogen bonding (Einfalt et al., 2013; Poonam et al., 2016). The creation of this amorphous glassy structure protects biomolecules from the negative effects of abiotic stresses, particularly dehydration and aids in the recovery of their specific functions when normal non-stress environmental conditions prevail (Kosar et al., 2018). Transgenic plants have conferred enhanced tolerance to various abiotic stresses through the expression of Tre biosynthesis genes (Iordachescu and Imai, 2008). TPP (trehalose-6-phosphate phosphatase) and TSS (trehalose-6-phosphate synthase) genes are commonly present in the genomes of higher plants (Leyman et al., 2001). OsTPP1, a member of the TPP gene family, is involved in circumventing salt tolerance through transient up-regulated expression (Chao et al., 2005). For instance, the overexpressed OsTPP1 gene in rice plants has been found to tolerate salt stress by promoting the expression of stress-responsive genes (Ge et al., 2008). Similarly, Tre accumulation takes place in transgenic rice plants through regulated expression of fusion genes involving E. coli biosynthetic genes (OtsA and OtsB) along with TPS and TPP genes (Garg et al., 2002). These plants exhibited high tolerance levels to salt stress, further necessitating the importance of these genes in the design of transgenic plants. In Arabidopsis, AtTPPD is a chloroplast-localized enzyme that has been speculated to enhance tolerance toward high salinity (Krasensky et al., 2014). Higher accumulation of soluble sugars and starch levels achieved through over-expression of AtTPPD suggested its putative role in the metabolism of sugars under salt stress. The introduction of TPP gene from rice into maize plants resulted in a 20%–31% higher yield than non-transgenic controls (Nuccio et al., 2015).
Sugar alcohol
Sugar alcohols such as pinitol, mannitol, myo-inositol and sorbitol show an influential role in mitigating stress conditions by adjusting osmotic equilibrium. These are also well-known as polyols. Myo-inositol and Pinitol have a cyclic structure, while mannitol and sorbitol have a linear structure. Their accumulation in plants is thought to serve a variety of functions, including osmotic adjustment, ROS regulation and molecular chaperons (Upadhyay et al., 2015; Bhattacharya and Kundu, 2020). Mannitol, a sugar alcohol, formed by the action of enzyme mannose-6-phosphate reductase on the mixture of glucose/fructose transforms into mannitol and gluconic acid. Mannitol aids in osmotic regulation and helps to remove oxygen radicals produced by stress (Kaya et al., 2013). Sorbitol is an osmoprotectant that is produced during photosynthesis (Wu et al., 2010). Zhou et al. (2003) utilized sorbitol-6-phosphate to produce sorbitol by dephosphorylating sorbitol-6-phosphatase. D-Ononitol, on the other hand, is a sugar alcohol that acts as an osmolyte, reducing water loss in plants during drought stress. The myo-inositol O-methyltransferase gene from Mesembryanthemum crystallinum was transferred into tobacco, which resulted in increased D-ononitol production and improved drought and salt resistance (Vinocur and Altman, 2005). Pinitol is generated by the methylation of myo-inositol and has found in many halophytic species. Ononitol epimerization also results in the formation of pinitol (Sengupta et al., 2008; Slama et al., 2015; Dumschott et al., 2019).
Mannitol
Mannitol is an acyclic polyol consisting of six carbon atoms (Slama et al., 2015). It is essential in quenching hydroxyl radicals (Gill and Tuteja, 2010a,b). Mannose-6-P isomerase (phosphomannose isomerase), mannose-6-phosphate reductase, and mannose-1-phosphate phosphatase are among the enzymes that start the production of mannitol in plants from Fructose-6-P (Loescher et al., 1992). As mannitol accumulates spontaneously in all plant species, adding it to non-mannitol accumulators can improve their resistance to adverse environmental conditions. Similarly, the incorporation of mannitol biosynthetic genes from accumulator species to non-accumulator species enhances their resistance to abiotic challenges, which is also a successful strategy for mitigating adverse impact of climate change. Eggplants engineered with mtlD gene have established tolerance against high NaCl stress (200 mM) (Prabhavathi et al., 2002). Populus tomentosa, a transgenic woody plant transformed with the mtlD gene encoding mannitol-1-phosphate dehydrogenase, has survived salt stress as a result of mannitol’s oxidative stress protection (Hu et al., 2005). This E. coli related mtlD gene encodes non-specific phosphatases that convert mannitol-1-phosphate to mannitol in transgenic plants.
Further, mannitol-synthesizing transgenic peanut plants resulted in the accumulation of mannitol by over-expression of the mtlD gene from E. coli under salt stress (Bhauso et al., 2014). Moreover, the antioxidant genes might influence the expression of the mtlD gene in maintaining cellular homeostasis through detoxification of ROS species during salt stress (Patel et al., 2016). Recently, an attempt to the production of mannitol synthesis in Arabidopsis thaliana has been reported by expression of two biosynthetic genes, namely, mannitol-1-phosphate dehydrogenase and mannitol-1-phosphatase genes from brown algae Ectocarpus sp. strain Ec32, that results in the production of 42.3–52.7 nmol g−1 fresh weight of mannitol, sufficient to impart salinity stress tolerance (Rathor et al., 2020).
Inositol
Inositol or more preciously myo-inositol is a sugar-like carbohydrate produced in many plants (Valluru and Van den Ende, 2011; Nisa et al., 2016). Being an osmoprotectant, its derivatives including pinitol, galactinol and ononitol, also perform diverse functions as osmoprotectant (Handa et al., 2018). It also regulates the synthesis of phytohormone such as auxin, phytic acid biosynthesis, and plant defense mechanism (Hazra et al., 2019). Inositol and other related molecules are suggested to prove salt tolerance in two ways: (1) protection of cellular structures from ROS, (2) maintaining cell turgor pressure inside the cell. The production of inositol is a two-step metabolic route that begins with the enzymatic conversion of d-glucose 6-P into myo-inositol-1-P catalyzed by myo-inositol-1-P synthase (Majumder et al., 1997), followed by dephosphorylation of myo-inositol-1-P resulting to form myo-inositol that further produces different inositol-containing compounds such as phospholipids (Dastidar et al., 2006). Introgression of salt tolerant MIPS (L-myo-inositol 1-phospahte synthase) protein encoded by PcINO1 gene of Porteresia coarctata resulted in inositol accumulation in tobacco plants (Majee et al., 2004). Transcriptome analysis studies revealed the potential of over-expression of the IbMIPS1 gene in transgenic sweet potato plants induced via salt stress (Zhai et al., 2016). Another MIPS gene, MdMIPS1 (myo-inositol-1-phosphate synthase1) overexpressed in transgenic apple plants, has promoted the biosynthesis of myo-inositol along with the accumulation of other osmoprotectants to alleviate salinity-induced osmotic stress (Hu et al., 2020).
Polyamines
Polyamines (PAs) are nitrogen-containing compounds having a low molecular weight that are present in cellular compartments. The most common PAs found in plants are spermidine (Spd), putrescine (Put), and spermine (Spm), classified as plant growth substances (Bano et al., 2020). Several other PAs such as cadaverine, homospermidine, canavalamine, and 1, 3-diamino propane are synthesized from amino acids. PAs generally occur in free or conjugated form with macromolecules or phenolic compounds. Put, Spd, and Spm are the most abundant PA that can be formed from arginine with the help of N-carbamoyl putrescine and agmatine (Urano et al., 2003). This putrescine is also converted into spermine and spermidine by synthase enzyme. In saline conditions, out of these polyamines, putrescine is mainly accumulated. They interact with the membrane surface and with the help of their polyanionic nature, stabilize the membrane structure (Gill and Tuteja, 2010a,b). PAs also increase the membrane fluidity and act as nitrogen reserve so that plants use them after stress conditions are over. They also support in maintaining cellular pH and ionic balance. The main functions of polyamines are osmotic adjustment, scavenging hydroxyl radicals via modulating enzyme activities and ammonia detoxification (Kuznetsov et al., 2007; Mustafavi et al., 2018; Chen et al., 2019). It has been suggested that Spd level is a salt tolerance indicator (Li and He, 2012). Exogenously application of Spd, improved plant development via increased reactive oxygen metabolism and photosynthesis under salinity stress (Meng et al., 2015; Baniasadi et al., 2018). Various transgenic approaches are used to improve stress tolerance by expressing polyamine biosynthesis enzymes such as arginine decarboxylase (ADC), ornithine decarboxylase (ODC), spermidine synthase (SPDS) and S-adenosyl methionine decarboxylase (SAMDC; Gill and Tuteja, 2010a,b). Regulation of PAs becomes important in salt-stressed plants after confirmation of poor performance of transgenic plants mutated with PA synthesis genes (Urano et al., 2004; Marco et al., 2015). Up-regulated expression of Calvin-cycle-related genes mediated by PAs is responsible for the mitigation of detrimental effects of salinity in Brassica napus L. (ElSayed et al., 2022). The key enzymes involved in the Calvin cycle consist of FBPase, PRKase, SBPase, and Rubisco, which are important for CO2 fixation (Raines, 2003). Limitation in Rubisco activity has been deduced as one of the major constraints in the down-regulation of photosynthesis in salinity stress (Lu et al., 2009). However, exogenous application of Spd has altered the expression of RbcL and RbcS genes, which subsequently influences the Rubisco structure and function (Spreitzer, 2003). Polyamine oxidases (PAOs) are catabolic enzymes of PAs that are flavin adenine dinucleotide- dependent (Wu et al., 2022). Different PAOs, such as AtPAOS and ZmPAO, have been identified in Arabidopsis and tobacco plants (Cona et al., 2006; Moschou et al., 2008). For instance, atpao5 in Arabidopsis stimulated the metabolic and transcriptional activities induced via salt stress (Zarza et al., 2017). Rice and wheat plants are identified with genes encoding proteins having PAO activity, suggesting an important function in salinity tolerance (Liu et al., 2014a,b; Xiong et al., 2017). Plant Polyamine oxidase (PAO) enzyme is responsible for H2O2 production during Put and Spd catabolism in plant tissues (Wang et al., 2019). Contrary to this, the OsPAO3 gene has exhibited a positive effect in rice plants through the enhanced accumulation of polyamines, which is sufficient to eliminate overproduction of H2O2 and exclude Na+ (Liu et al., 2022).
Salt overlay sensitive pathway
In terms of metabolic energy, plants use ions to balance water potential in tissues, unlike the use of carbohydrates or amino acids, which requires a significantly larger amount of energy. On the other hand, concentrations of ions should be maintained at an optimum level otherwise, it could be toxic to many cytosolic enzymes; therefore, compartmentalization of ions in the vacuoles becomes necessary (Binzel et al., 1998). As NaCl is the most common salt encountered by the plants during salinity stress, the salt overlay sensitive pathway is one of the main strategic approaches adopted by the plants. Plants perceive high Na+ concentration through downstream signaling of stress responses (Gong, 2021). The activation of Ca2+ channels always accompanies changes in ion concentrations and osmotic pressure. A putative osmosensor, OSCA1, is responsible for downstream Ca2+ signaling induced via osmotic stress (Yuan et al., 2014; Zhang et al., 2020). Similarly, the Arabidopsis mutant moca1 (monocation-induced Ca2+) delivered a hypersensitive response to salt stress. These are identified as Na+-gated Ca2+ channels involved in the enhancement of Ca2+ concentration. GIPs (glycosyl inositol phosphorylceramide) are Na+ sensors encoded by MOCA1 to increase the influx of Ca2+ ions (Jiang et al., 2019). Cell-wall integrity is maintained by plasma membrane-positioned receptors-like kinases, FERONIA (FER), under salt stress (Feng et al., 2018). FER, along with BAK1 phosphorylate CNGCS (cyclic nucleotide-gated ion channels) involved in Ca2+ signaling (Pan et al., 2019; Tian et al., 2019). Since salt stress triggers an overproduction of ROS, i.e., H2O2, a HPCA1 sensor located in the plasma membrane senses an increase in H2O2 concentration (Wu et al., 2020).
In a salt overlay sensitive pathway, SOS1, SOS2 and SOS3 are three genes commonly involved (Wu et al., 1996). During salinity stress, elevated Na+ stimulates a rise in cytosolic Ca2+ concentration, which interacts with Ca2+ binding protein SOS3, and further interaction with Serine/threonine kinase protein SOS2 mediates the efflux of Na+ from the cells through Na+/H+ antiporter (SOS1; Shi et al., 2003; Kim et al., 2007). SOS3 and SOS2 kinase complex directly phosphorylates SOS1. Furthermore, SOS1, SOS2 and SOS3 work together to provide resistance to salinity stress (Mahajan and Tuteja, 2005; Park et al., 2016). Furthermore, another member of SOS3 family, Calcineurin B-like (CBL10), forms a complex with SOS2, which is thought to regulate both the exclusion of Na+ ion through SOS1 and the compartmentalization of Na+ ion into the vacuole by activating Na+/H+ antiporter (NHX). A schematic representation of salt overlay sensitive signaling has been depicted in Figure 2.
Salt stress signaling pathways regulates osmoprotectants accumulation
Plants perceive abiotic stress by activating signal-transduction pathways that allow them to adapt to even minor environmental changes. A wide array of complex transduction pathways are involved in sensing salt stress and generating a response during salinity stress. Pathways such as Salt overlay sensitive (SOS) pathway, phytohormone signaling, Calcium signaling, and Mitogen-Activated Protein Kinase (MAPK) network are involved in production and accumulation of osmolytes in regulating osmotic homeostasis during salinity stress (Roychoudhury and Banerjee, 2017).
The biosynthesis and accumulation of osmolytes is one of the important events in the activation of stress signaling pathways in plants during exposure to abiotic stress such as salt, heavy metals, cold, drought etc., that enables the plants to adapt quickly to changing environmental conditions. The SOS pathway is reported to be regulated by MAP Kinase pathways and the level of osmoprotectant GB also affects this pathway (Ashraf and Foolad, 2007). Similarly, abscisic acid signaling regulates proline accumulation in response to salinity stress as well as drought (Verslues and Bray, 2005).
In order to withstand constant stressful conditions, phytohormone mediating stress tolerance has been found to be crucial in plant response to salinity stress. Since phytohormones makes a great contribution is sensing salinity stress and adaption, nine plant hormones are commonly involved, which are divided into two groups: growth promoting hormones and stress response hormones (Yu et al., 2020). Auxins, gibberellins (GAs), cytokinins (CKs), brassinosteroids (BRs) and strigolactones (SLs) are growth-promoting hormones, while abscisic acid (ABA), ethylene, salicylic acid (SA) and jasmonic acid (JA) are stress response hormones. Among all, abscisic acid is most important in regulating salinity stress responses. Under stressful conditions, ABA accumulation takes place, which further activates kinase cascades and initiates stress defense reactions (Zhu, 2016). The Sucrose-nonfermenting-1-protein kinases 2 s (SnRK2s) are the main components in abscisic acid signaling pathways.
Phytohormone signaling mediates osmoprotectants biosynthesis under salinity stress
The plant responds to salinity stress by the accumulation of compatible solutes as already discussed above. In order to alleviate osmotic stress, osmolytes further modulate the enhanced expression of genes involved in synthesis of plant hormones such as abscisic acid (ABA), cytokinins (CK), salicylic acid (SA), jasmonic acid (JA), auxins, polyamines, brassinosteroids (BRs) and gibberellins (GRs) (Fahad et al., 2015; Rao et al., 2016). These hormones has diverse role in modulating the signaling pathways during the emergence of salinity stress (Ali et al., 2020). Since, osmolytes and phytohormones have been elucidated to have a significant role under demanding environmental conditions; it is therefore becoming crucial to understand the regulation of osmolytes and phytohormones and further correlate the roles of the same. The interaction of phytohormones with osmoprotectants has been explained in Figure 3.
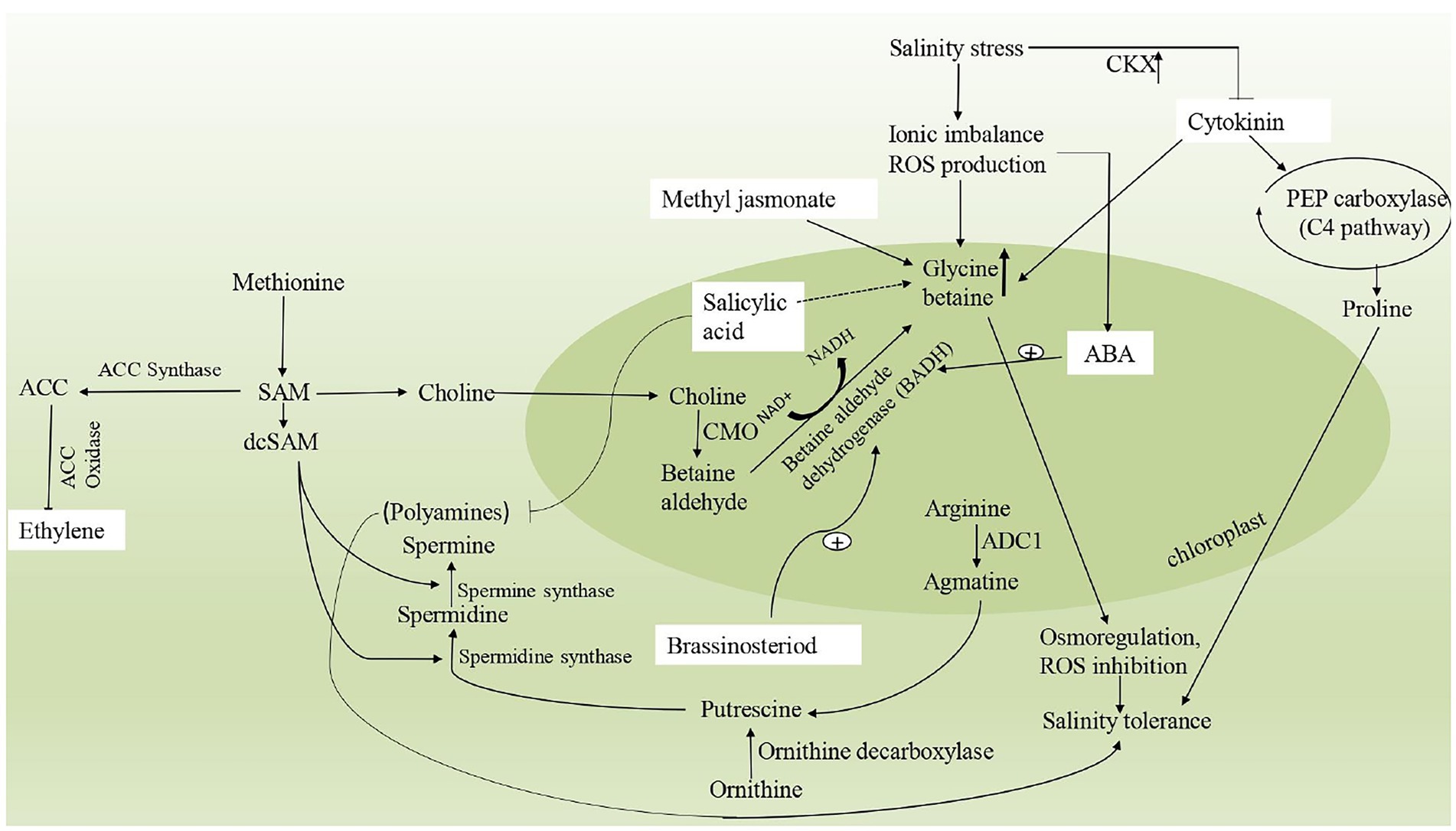
Figure 3. A schematic representation describing the interaction of different osmoprotectants with phytohormones under salinity stress. Glycine betaine and Ethylene are interlinked by a common pathway involving enzymes, BADH (betaine aldehyde dehydrogenase), CMO (choline monooxygenase), SAM (S-adenosylmethionine), ACC synthase and ACC oxidase. Polyaminebiosynthesis includes ornithine and arginine decarboxylation, catalyzed by ornithine decarboxylase (ODC) and arginine decarboxylase (ADC), respectively. Spermidine (Spd) is synthesized from putrescine via spermidine synthase (SPDS) with the addition of aminopropyl moiety donated by decarboxylated S-adenosylmethionine (dcSAM). Similarly, Spermine (Spm) is produced from spermidine (Spd) via spermine synthase (SPMS) with the same aminopropyl rendered by dcSAM. Cytokinins are deactivated by CKX1 (cytokinin oxidase/dehydrogenase) gene on exposure to high salinity stress.
Brassinosteroids
Many physiological processes in plants are controlled by brassinosteroid signaling during salt stress. BRL3, a member of the brassinosteroid (BR) receptor family, regulates biosynthesis of many key osmoprotectants (Fabregas et al., 2018). Soluble osmolytes sugars maintain osmotic homeostasis and helps in ROS scavenging (Parvaiz and Satyawati, 2008). Proline acts as a molecular chaperone by scavenging free radicals and also stabilizes redox reactions inside cytosol (Kumar et al., 2013). Applications of proline in addition to brassinosteroid (24-epibrassinolide) to cultivars of Brassica juncea that grow in saline environment minimize harmful effects of salinity and improves yield (Wani et al., 2019). GB is a type of compatible osmoprotectant, which never changes at neutral pH; hence highly water soluble but in the hydration sphere of proteins, is insoluble. So these sugar osmolytes withhold water and preserve the protein structure (Kurepin et al., 2017). Artificial supply of brassinosteroids provides salt stress tolerance to plants by enhancing GB secretion as illustrated in Figure 3. Exogenous application of 24-epibrassinolide to tolerant and sensitive varieties of Pisum sativum plants was reported in enhancing the GB content and further helps in mitigation of salinity stress (Shahid et al., 2014). In another study, Cd stressed Pisum sativum plants when subjected to 24-epibrassinolide it causes an additional increase in the level of GB, providing a stress tolerance ability to plants (Ahmad et al., 2018). Treatment of Zea mays L. seedlings with HBL (28-homobrassionolide) and EBL (epibrassinolide) resulted in the modulation of antioxidative enzyme activities and compatible osmolytes that accounts for osmotic adjustment during salt stress (Rattan et al., 2020). Hence, brassinosteroid has stress defending capacity by increasing osmolyte.
Ethylene
Ethylene performs various physiological processes in the plant involving seed germination, plant development, senescence, fruit ripening, it has also been known to have a significant role in stress tolerance. It regulates abiotic stress by accumulating osmoprotectants (Iqbal et al., 2015). S-adenosyl methionine (SAM), the precursor for ethylene biosynthesis, is also a precursor for GB biosynthesis (Figure 3). Increasing GB and lower ethylene content (by inhibiting the activity of ACC synthase enzyme) in salinity stress enhances glutathione concentration (GSH) hence reducing oxidative stress (Khan et al., 2014). Proline along with ethylene gives salt tolerance capacity to Brassica juncea (Iqbal et al., 2015). Exogenous application of ethephon (ethylene source) with both doses of N and S on salt-stressed mustard plants increased the metabolism of proline, which is responsible for reducing oxidative stress (Jahan et al., 2021). The reason behind this fact is that increased nitrogen levels subsequently enhanced proline accumulation to provide salt tolerance (Iqbal et al., 2015). The biosynthesis of polyamines is also linked to ethylene production in terms of presence of precursor (S-adenosylmethionine, SAM; Petruzzelli et al., 2000). According to reports, ethylene and PAs are highly correlated in response to (ROS) production in the leaves of spring wheat seedlings under osmotic stress (Li et al., 2004). Increased PAs content, reduced ROS production, and ethylene synthesis were found in plants in response to stressful conditions.
Cytokinins
Cytokinins are growth-promoting phytohormones and regulate plant growth and development (Pavlu et al., 2018). It promotes cell division in plant tissue culture and also regulates the cell cycle (Schaller et al., 2014). For osmolytes synthesis and accumulation in stress, ethylene cell signaling, MAPK plays a crucial function in plants (Shen et al., 2014). In abiotic stress, Cytokinin Oxidase/Dehydrogenase (CKX1) gene encodes for the enzyme that deactivates active cytokinins and increases the accumulation of GB content (Kathuria et al., 2009). Similarly, in Physcomitrella patens, overexpression of PpCKX1 lowers cytokinin levels and increases salt tolerance (Hyoung et al., 2019). These CKX-induced cytokinin-deficient plants are more valuable for researching the role of cytokinin than ipt mutants (Werner et al., 2003). The supply of cytokinin with NaCl gives signals that stimulate PEP carboxylase accumulation along with proline. Cytokinin activates PEP carboxylase that is responsible for increased proline levels in M. crystallinum (Thomas et al., 1992) and improved salinity stress tolerance (Figure 3). Cytokinins also regulate GB biosynthesis by modulating the GB pathway. In Solanum lycopersicum, the potential of Kinetin (Kn) and epibrassinolide (EML), either separately or in combination, was investigated under salinity stress. Results suggest that by enhancing the other physiological process, also leads to accumulation of GB through a crosstalk mechanism (Ahanger et al., 2020). Interestingly, several studies demonstrate that cytokinins and PAs govern various physiological and biochemical processes in plants, with a strong link between their levels, and operate as inter and intracellular messengers, regulating biotic and abiotic stresses (Galston, 1983; Wimalasekera and Scherer, 2009). However, the mechanism by which cytokinins influence the accumulation of PAs in plants is still unknown.
Abscisic acid
Abscisic acid (ABA) maintains osmotic adjustment in salt-stressed plants by regulating physiological processes for osmolyte regulation (Karimi and Ershadi, 2015). At a molecular level, ABA controls synthetic pathways of osmolytes by acting as signaling molecules (Pal et al., 2018). It also increases proline synthesis and accumulation to protect plant cells from damaging effects (Karimi and Ershadi, 2015). This increased proline accumulation is due to an increase in the transcription of genes encoding essential enzymes in proline biosynthesis. In Medicago truncatula, it was reported that proline accumulation occurs under water deficit stress conditions controlled by ABA levels (Planchet et al., 2014). ABA increases the biosynthesis route of GB, resulting in increased accumulation of this osmolyte in plant cells, which aids in abiotic stress resistance (Zhang et al., 2012). Under abiotic stress after adding ABA to plant synthesis of GB level enhanced due to increased activity of GB biosynthetic enzyme betaine-aldehyde dehydrogenase (BADH; Yang et al., 2015; Figure 3). By analyzing drought-stressed plants after fluridone treatment, these researchers were able to confirm the significance of ABA in betaine-aldehyde dehydrogenase upregulation. In PAs production, ABA regulates critical transcriptional processes. For instance, gene transcript of arginine decarboxylase 2 (ADC2), spermidine synthase1 (SPDS1), and spermine synthase (SPMS) were found to be up-regulated under drought conditions (Alcázar et al., 2006). Similar trends were also reported in arginine decarboxylase (ADC) expression patterns in response to salinity stress (Urano et al., 2004). In plants, such as Atriplex halimus, Oryza sativa, Phaseolus vulgaris and Zea mays, the impact of ABA levels in response to salinity stress has also been reported (Liu et al., 2005; Ben Hassine et al., 2009; Shevyakova et al., 2013). ABA priming has been used to provide tolerance to abiotic stress such as drought, cold, or salt stress (Savvides et al., 2016). In addition, one-time ABA priming in Vicia faba grown under 50 mM salinity has been found to alleviate salt stress through alteration in gene expression patterns over time, maintaining the ionic and osmotic balance and increasing photosynthesis and growth (Sagervanshi et al., 2021).
Jasmonic acid
In abiotic stress, jasmonic acid (JA) includes several plant reactions such as gene regulation, synthesis of particular proteins, and secondary metabolism. JA regulates the detrimental effects of environmental stress through a cascade of plant responses (Choudhary and Agrawal, 2014a,b; Choudhary et al., 2021). For example, JA increased the levels of non-enzymatic antioxidants such as proline, which has been reported in several studies (Anjum et al., 2011; Shan et al., 2015). Under salinity stress, methyl jasmonate greatly mitigated the adverse effects of salinity on soybean growth (Yoon et al., 2009). Exogenous jasmonates (JAs) supplement enhances plant growth because of their effect on metabolites. Application of JAs increases the accumulation of constituents of Krebs cycle and finally gives resistance to stressed plants (Sharma et al., 2016). In Solanum lycopersicum, the application of JAs increases the production of GB and thus enhance plant growth and development under salinity stress conditions (Ahmad et al., 2017, 2018; Figure 3). Salinity-induced peroxidation have been observed under exogenous application of methyl jasmonate in Brassica napus due to enhanced soluble sugar levels in leaves (Ahmadi et al., 2018). Furthermore, JA signaling have also been implicated in the production of PAs in fruit ripening, insect-pathogen tolerance, low-temperature injuries. Due to their primary role in activating essential antioxidant enzymes, Spd levels increased in barley genotypes and protected membranes from peroxidation (Bandurska et al., 2003). Similarly, under any form of stress, sugar level rises, JA has been demonstrated to enhance sugar content in a variety of crop plants, including Triticum aestivum, Brassica napus, and Ipomea batata, as well as improve overall plant performance under abiotic conditions (El-Khallal, 2001; Harpreet et al., 2013).
Salicylic acid
Salicylic acid (SA), as a vital phytohormone, has multifaceted role in plant growth and developmental processes such as photosynthesis, mineral ion absorption and assimilation, antioxidant, and tolerance to stress (Choudhary and Agrawal, 2014a,b; Moravcová et al., 2018; Choudhary et al., 2021). The importance of SA in increasing resilience to environmental challenges has been well documented (Ahmad et al., 2011; Khan et al., 2015). During abiotic stress, the synthesis of osmolytes like GB, proline and sugar are influenced by SA (Misra and Misra, 2012; Jangra et al., 2022). The elevation in proline accumulation in salt-stress plants attributed to a stress tolerance mechanism (Misra and Saxena, 2009). In Rauvolfia serpentina, SA involves in enhancing proline production under salt stress and regulating cell turgor. It also mitigates salt stress in the seedling of Torreya grandis by accumulating proline. More proline concentration enhances the synthesis of stress protective proteins increasing stress tolerance (Li et al., 2014). SA enhances the accumulation of proline by regulating proline biosynthesis gene expression pattern. For instance, the exogenous application of SA mediates upregulation of important genes like P5CSA and P5CSB (encodes pyrroline-5-carboxylate synthase) and downregulation of PDH (encoding proline dehydrogenase; Lee et al., 2019).
SA improves the overall growth of the plant by influencing the concentration of GB (Misra and Misra, 2012; Farhadi and Ghassemi-Golezani, 2020). On the other hand, by applying exogenous SA and its analogs, scientists have determined its imperative role in ameliorating salt stress on various plants (Gharbi et al., 2017; Shaki et al., 2018). Recently, in Vigna radiata plants, supply of 0.5 mM SA enhanced the accumulation of GB by more than 40% with respect to control and thus minimizing the adverse of salinity (Syeed et al., 2021; Figure 3). On application of exogenous SA to salinity stressed Zea mays, SA increases the level of soluble sugars in this plant (Khodary, 2004). On the other hand, SA, prevents the accumulation of PAs under salt stress conditions (Palma et al., 2013).
Conclusion and future prospects
Salt stress in crop plants is a potential threat for agricultural crop productivity and ultimately to food security under the current and futuristic Climate-changing scenarios, worldwide. The mechanism of salinity tolerance in plants is complex involving osmotic stress and ion toxicity causing a major loss of yield and quality. The role of phytohormones in regulating responses to adverse effects of salinity has been well documented however the studies related to phytohormone mediating osmolyte biosynthesis require more research insight to get a clear mechanism of crops tolerance. Therefore, in the present article we have summarized the roles of phytohormones in regulating osmolytes and further enhance our knowledge by explaining the cross talk at the physiological level on exposure to salinity. Apart from this, still, molecular dissection studies are required to unravel the mechanism behind the modulation of osmolytes. Among all phytohormones, the role of cytokinin remains contradictory, which needs to be focused. Various transgenic approaches have been elucidated in salt-sensitive plants that successfully impart salinity tolerance in plants. However, the deployment of novel approaches involving phytohormone engineering metabolism could be considered a method of choice to produce salt-resilient crops with higher yields.
Author contributions
PS, SG, MS, BT, and SS: conceptualization, writing original draft, and writing—review and editing. NC: visualization and writing—review and editing. KC: conceptualization, visualization, supervision, and writing—review, and editing. All authors contributed to the article and approved the submitted version.
Acknowledgments
PS is thankful to CSIR, New Delhi, India for the Junior Research Fellowship (F. No: 09/0013(12956)/2021-EMR-I). KKC is grateful to UGC-BSR Start-up Grant (No. F. 30-432/2018(BSR)) New Delhi, India and to seed grant, Institute of Eminence (IoE - Dev. Scheme no. 6031), Banaras Hindu University, Varanasi, India. NC is thankful to UGC BSR Start up (No. F.30-547/2021(BSR)) Grant, New Delhi, India.
Conflict of interest
The authors declare that the research was conducted in the absence of any commercial or financial relationships that could be construed as a potential conflict of interest.
Publisher’s note
All claims expressed in this article are solely those of the authors and do not necessarily represent those of their affiliated organizations, or those of the publisher, the editors and the reviewers. Any product that may be evaluated in this article, or claim that may be made by its manufacturer, is not guaranteed or endorsed by the publisher.
References
Abdallah, M. M. S., Abdelgawad, Z. A., and El-Bassiouny, H. M. S. (2016). Alleviation of the adverse effects of salinity stress using trehalose in two rice varieties. South Afr. J. Bot. 103, 275–282. doi: 10.1016/j.sajb.2015.09.019
Abdelrahman, M., Jogaiah, S., Burritt, D. J., and Tran, L. P. (2018). Legume genetic resources and transcriptome dynamics under abiotic stress conditions. Plant Cell Environ. 41, 1972–1983. doi: 10.1111/pce.13123
Ahanger, M. A., Mir, R. A., Alyemeni, M. N., and Ahmad, P. (2020). Combined effects of brassinosteroid and kinetin mitigates salinity stress in tomato through the modulation of antioxidant and osmolyte metabolism. Plant Physiol. Biochem. 147, 31–42. doi: 10.1016/j.plaphy.2019.12.007
Ahirwal, J., Kumari, S., Singh, A. K., Kumar, A., and Maiti, S. K. (2021). Changes in soil properties and carbon fluxes following afforestation and agriculture in tropical forest. Ecol. Indic. 123:107354. doi: 10.1016/j.ecolind.2021.107354
Ahluwalia, V. K. (2022). “Stereochemistry of biomolecules,” in Stereochemistry of Organic Compounds. ed. Ahluwalia (Cham: Springer), 477–502.
Ahmad, P., Abd-Allah, E. F., Alyemeni, M. N., Wijaya, L., Alam, P., Bhardwaj, R., et al. (2018). Exogenous application of calcium to 24-epibrassinosteroid pre-treated tomato seedlings mitigates NaCl toxicity by modifying ascorbate–glutathione cycle and secondary metabolites. Sci. Rep. 8:13515. doi: 10.1038/s41598-018-31917-1
Ahmad, P., Alyemen, M. N., Wijaya, L., Alam, P., Ahanger, M. A., and Alamri, S. A. (2017). Jasmonic acid alleviates negative impacts of cadmium stress by modifying osmolytes and anti-oxidants in faba bean (Viciafaba L.). Arch. Agron. Soil Sci. 63, 1889–1899. doi: 10.1080/03650340.2017.1313406
Ahmad, P., Nabi, G., and Ashraf, M. (2011). Cadmium-induced oxidative damage in mustard [Brassica juncea (L.) Czern. &Coss.] plants can be alleviated by salicylic acid. S. Afr. J. Bot. 77, 36–44. doi: 10.1016/j.sajb.2010.05.003
Ahmadi, F. I., Karimi, K., and Struik, P. C. (2018). Effect of exogenous application of methyl jasmonate on physiological and biochemical characteristics of Brassica napus l.cv. Talaye under salinity stress. S. Afr. J. Bot. 115, 5–11. doi: 10.1016/j.sajb.2017.11.018
Ahn, C. H., Hossain, M. A., Lee, E., Kanth, B. K., and Park, P. B. (2018). Increased salt and drought tolerance by D-pinitol production in transgenic Arabidopsis thaliana. Biochem. Biophys. Res. Commun. 504, 315–320. doi: 10.1016/j.bbrc.2018.08.183
Alcázar, R., Cuevas, J. C., Patron, M., Altabella, T., and Tiburcio, A. F. (2006). Abscisic acid modulates polyamine metabolism under water stress in Arabidopsis thaliana. Physiol. Plant. 128, 448–455. doi: 10.1111/j.1399-3054.2006.00780.x
Ali, Q., Shahid, S., Nazar, N., Hussain, B., and Hussain, S. M. (2020). “Use of phytohormones in conferring tolerance to environmental stress,” in Plant Ecophysiology and Adaptation Under Climate Change: Mechanisms and Perspectives II: Mechanisms of Adaptation and Stress Amelioration. ed. M. Hasanuzzaman (Singapore: Springer), 245–355.
Alkhatib, R., Abdo, N., and Mheidat, M. (2021). Photosynthetic and Ultrastructural properties of eggplant (Solanum melongena) under salinity stress. Horticulturae 7:181. doi: 10.3390/horticulturae7070181
Al-Tamimi, N., Brien, C., Oakey, H., Berger, B., Saade, S., Ho, Y. S., et al. (2016). Salinity tolerance loci revealed in rice using high-throughput non-invasive phenotyping. Nat. Commun. 7:13342. doi: 10.1038/ncomms13342
Alzahib, R. H., Migdadi, H. M., Al Ghamdi, A. A., Alwahibi, M. S., Ibrahim, A. A., and Al-Selwey, W. A. (2021). Assessment of morpho-physiological, biochemical and antioxidant responses of tomato landraces to salinity stress. Plants (basel) 10:696. doi: 10.3390/plants10040696
Anjum, S. A., Ashraf, U., Tanveer, M., Khan, I., Hussain, S., Shahzad, B., et al. (2017). Drought induced changes in growth, Osmolyte accumulation and antioxidant metabolism of three maize hybrids. Front. Plant Sci. 8:69. doi: 10.3389/fpls.2017.00069
Anjum, S. A., Wang, L., Farooq, M., Khan, I., and Xue, L. (2011). Methyl Jasmonate-induced alteration in lipid peroxidation, Antioxidative Defence system and yield in soybean under drought. J. Agron. Crop Sci. 197, 296–301. doi: 10.1111/j.1439-037X.2011.00468.x
Annunziata, M. G., Ciarmiello, L. F., Woodrow, P., Dell’ Aversana, E., and Carillo, P. (2019). Spatial and temporal profile of glycine betaine accumulation in plants under abiotic stresses. Front. Plant Sci. 10, 1–13. doi: 10.3389/fpls.2019.00230
Arif, Y., Singh, P., Siddiqui, H., Bajguz, A., and Hayat, S. (2020). Salinity induced physiological and biochemical changes in plants: An omic approach towards salt stress tolerance. Plant Physiol. Biochem. 156, 64–77. doi: 10.1016/j.plaphy.2020.08.042
Ashraf, M. F. M. R., and Foolad, M. (2007). Roles of glycine betaine and proline in improving plant abiotic stress resistance. Environ. Exp. Bot. 59, 206–216. doi: 10.1016/j.envexpbot.2005.12.006
Asif, M., Schilling, R. K., Tilbrook, J., Brien, C., Dowling, K., Rabie, H., et al. (2018). Mapping of novel salt tolerance QTL in an Excalibur kukri doubled haploid wheat population. Theor. Appl. Genet. 131, 2179–2196. doi: 10.1007/s00122-018-3146-y
Assaha, D. V., Ueda, A., Saneoka, H., Al-Yahyai, R., and Yaish, M. W. (2017). The role of Na+ and K+ transporters in salt stress adaptation in glycophytes. Front. Physiol. 8:509. doi: 10.3389/fphys.2017.00509
Bandurska, H., Stroinski, A., and Kubi’s, J. (2003). The effect of jasmonic acid on the accumulation of ABA, proline and spermidine and its influence on membrane injury under water deficit in two barley genotypes. Acta Physiol. Plant. 25, 279–285. doi: 10.1007/s11738-003-0009-0
Baniasadi, F., Saffari, V. R., and Moud, A. A. M. (2018). Physiological and growth responses of Calendula officinalis L. plants to the interaction effects of polyamines and salt stress. Sci. Horticult. 234, 312–317. doi: 10.1016/j.scienta.2018.02.069
Bano, C., Amist, N., and Singh, N. B. (2020). “Role of polyamines in plants abiotic stress tolerance: advances and future prospects,” in Plant Life Under Changing Environment. eds. D. K. Tripathi, V. P. Singh, D. K. Chauhan, S. Sharma, S. M. Prasad, and N. K. Dubey, et al. (Cambridge: Academic Press), 481–496.
Ben Hassine, A., Ghanem, M. E., Bouzid, S., and Lutts, S. (2009). Abscisic acid has contrasting effects on salt excretion and polyamine concentrations of an inland and a coastal population of the Mediterranean xero-halophyte species Atriplex halimus. Ann. Bot. 104, 925–936. doi: 10.1093/aob/mcp174
Bhattacharya, S., and Kundu, A. (2020). “Sugars and sugar polyols in overcoming environmental stresses” in Protective Chemical Agents in the Amelioration of Plant Abiotic Stress. eds. A. Roychoudhury and D. K. Tripathi (Hoboken, NJ: John Wiley and Sons), 71–101.
Bhauso, T. D., Radhakrishnan, T., Kumar, A., Mishra, G. P., Dobaria, J. R., Patel, K., et al. (2014). Overexpression of bacterial mtlD gene in peanut improves drought tolerance through accumulation of mannitol. Sci. World J. 2014:125967. doi: 10.1155/2014/125967
Binzel, M. L., Hess, F. D., Bressan, R. A., and Hasegawa, P. M. (1998). Intracellular compart mentation of ions in salt adapted tobacco cells. Plant Physiol. 86, 607–614. doi: 10.1104/pp.86.2.607
Catala, R., López-Cobollo, R., Berbís, M. Á., Jiménez-Barbero, J., and Salinas, J. (2021). Trimethylamine N-oxide is a new plant molecule that promotes abiotic stress tolerance. Sci. Adv. 7:eabd9296. doi: 10.1126/sciadv.abd9296
Chao, D. Y., Luo, Y. H., Shi, M., Luo, D., and Lin, H. X. (2005). Salt-responsive genes in rice revealed by cDNA microarray analysis. Cell Res. 15, 796–810. doi: 10.1038/sj.cr.7290349
Chaudhary, N., and Choudhary, K. K. (2021). “Photosynthetic responses and tolerance mechanism of crop plants against salt stress,” in Salt Stress Responses in Plants: Perception, Signaling, Omics and Tolerance Mechanisms. eds. P. K. Srivastava, J. Kumar, and S. M. Prasad (Hauppauge, New York: Nova Science Publishers), 65–84.
Chaudhry, S., and Sidhu, G. P. S. (2021). Climate change regulated abiotic stress mechanisms in plants: a comprehensive review. Plant Cell Rep. 41, 1–31. doi: 10.1007/s00299-021-02759-5
Chen, T. H. H., and Murata, N. (2008). Glycine betaine: an effective protectant against abiotic stress in plants. Trends Plant Sci. 13, 499–505. doi: 10.1016/j.tplants.2008.06.007
Chen, T. H. H., and Murata, N. (2011). Glycinebetaine protects plants against abiotic stress: mechanisms and biotechnological applications. Plant Cell Environ. 34, 1–20. doi: 10.1111/j.1365-3040.2010.02232.x
Chen, D., Shao, Q., Yin, L., Younis, A., and Zheng, B. (2019). Polyamine function in plants: metabolism, regulation on development, and roles in abiotic stress responses. Front. Plant Sci. 9:1945. doi: 10.3389/fpls.2018.01945
Choudhary, K. K., and Agrawal, S. B. (2014a). Cultivar specificity of tropical mung bean (Vigna radiate L.) to elevated ultraviolet-B: changes in antioxidative defense system, nitrogen metabolism and accumulation of jasmonic and salicylic acids. Environ. Exp. Bot. 99, 122–132. doi: 10.1016/j.envexpbot.2013.11.006
Choudhary, K. K., and Agrawal, S. B. (2014b). Ultraviolet-B induced changes in morphological, physiological, and biochemical parameters of two cultivars of pea (Pisum sativum L.). Ecotox. Environ. Safe 100, 178–187. doi: 10.1016/j.ecoenv.2013.10.032
Choudhary, K. K., Chaudhary, N., Agrawal, S. B., and Agrawal, M. (2017). “Reactive oxygen species: generation, damage, and quenching in plants during stress,” in Reactive Oxygen Species in Plants: Boon or Bane—Revisiting the Role of ROS. eds. V. P. Singh, S. Singh, D. K. Tripathi, S. M. Prasad, and D. K. Chauhan (Hoboken, NJ, USA: John Wiley & Sons Ltd), 89–115.
Choudhary, K. K., Singh, S., Agrawal, M., and Agrawal, S. B. (2021). “Role of Jasmonic and salicylic acid signaling in plants under UV-B stress,” in Jasmonates and Salicylates Signaling in Plants. Signaling and Communication in Plants. eds. T. Aftab and M. Yusuf (Cham: Springer), 45–63.
Cona, A., Rea, G., Angelini, R., Federico, R., and Tavladoraki, P. (2006). Functions of amine oxidases in plant development and defence. Trends Plant Sci. 11, 80–88. doi: 10.1016/j.tplants.2005.12.009
Dar, M. I., Naikoo, M. I., Rehman, F., Naushin, F., and Khan, F. A. (2016). “Proline accumulation in plants: roles in stress tolerance and plant development,” in Osmolytes and Plants Acclimation to Changing Environment: Emerging Omics Technologies. eds. N. Iqbal, R. Nazar, and N. A. Khan (New Delhi: Springer), 155–166.
Darko, E., Végh, B., Khalil, R., Marček, T., Szalai, G., Pál, M., et al. (2019). Metabolic responses of wheat seedlings to osmotic stress induced by various osmolytes under iso-osmotic conditions. PLoS One 14:e0226151. doi: 10.1371/journal.pone.0226151
Dastidar, K. G., Maitra, S., Goswami, L., Roy, D., Das, K. P., and Majumder, A. L. (2006). An insight into the molecular basis of salt tolerance of L-myo-inositol 1-P synthase (PcINO1) from Porteresiacoarctata (Roxb.) Tateoka, a halophytic wild rice. Plant Physiol. 140, 1279–1296. doi: 10.1104/pp.105.075150
de Freitas, P. A. F., de Carvalho, H. H., Costa, J. H., Miranda, R. D. S., Saraiva, K. D. D. C., de Oliveira, F. D. B., et al. (2019). Salt acclimation in sorghum plants by exogenous proline: physiological and biochemical changes and regulation of proline metabolism. Plant Cell Rep. 38, 403–416. doi: 10.1007/s00299-019-02382-5
de Freitas, P. A. F., de Souza Miranda, R., Marques, E. C., Prisco, J. T., and Gomes-Filho, E. (2018). Salt tolerance induced by exogenous proline in maize is related to low oxidative damage and favorable ionic homeostasis. J. Plant Growth Regul. 37, 911–924. doi: 10.1007/s00344-018-9787-x
Delauney, A. J., Hu, C. A., Kishor, P. B., and Verma, D. P. (1993). Cloning of ornithine delta-aminotransferase cDNA from Vigna aconitifolia by trans-complementation in Escherichia coli and regulation of proline biosynthesis. J. Biol. Chem. 268, 18673–18678. doi: 10.1016/S0021-9258(17)46682-8
Deuschle, K., Funck, D., Hellmann, H., Däschner, K., Binder, S., and Frommer, W. B. (2001). A nuclear gene encoding mitochondrial Δ1-pyrroline-5-carboxylate dehydrogenase and its potential role in protection from proline toxicity. Plant J. 27, 345–356. doi: 10.1046/j.1365-313X.2001.01101.x
dos Santos, T. B., and Vieira, L. G. E. (2020). Involvement of the galactinol synthase gene in abiotic and biotic stress responses: a review on current knowledge. Plant Gene 24:100258. doi: 10.1016/j.plgene.2020.100258
Dubos, C., Huggins, D., Grant, G. H., Knight, M. R., and Campbell, M. M. (2003). A role for glycine in the gating of plant NMDA-like receptors. Plant J. 35, 800–810. doi: 10.1046/j.1365-313X.2003.01849.x
Dumschott, K., Dechorgnat, J., and Merchant, A. (2019). Water deficit elicits a transcriptional response of genes governing D-pinitol biosynthesis in soybean (Glycine max). Int. J. Mol. Sci. 20:2411. doi: 10.3390/ijms20102411
Dutta, T., Neelapu, N. R. R., Wani, S. H., and Surekha, C. (2019). “Role and regulation of osmolytes as signaling molecules to abiotic stress tolerance,” in Plant Signal. Mol: Role and Regulation Under Stressful Environments. eds. M. I. R. Khan, P. S. Reddy, A. Ferrante and N. A. Khan (Sawston, Cambridge: Woodhead Publishing), 459–477.
Einfalt, T., Planinsek, O., and Hrovat, K. (2013). Methods of amorphization and investigation of the amorphous state. Acta Pharma. 63, 305–334. doi: 10.2478/acph-2013-0026
El-Badri, A. M., Batool, M., AA Mohamed, I., Wang, Z., Khatab, A., Sherif, A., et al. (2021). Antioxidative and metabolic contribution to salinity stress responses in two rapeseed cultivars during the early seedling stage. Antioxidants 10:1227. doi: 10.3390/antiox10081227
El-Khallal, S. (2001). Some physiological roles of jasmonic acid in adaptation of pea seedlings to salt stress. Egypt. J. Biotechnol. 10, 249–271.
ElSayed, A. I., Mohamed, A. H., Rafudeen, M. S., Omar, A. A., Awad, M. F., and Mansour, E. (2022). Polyamines mitigate the destructive impacts of salinity stress by enhancing photosynthetic capacity, antioxidant defense system and upregulation of Calvin cycle-related genes in rapeseed (Brassica napus L.). Saudi. J. Biol. Sci. 29, 3675–3686. doi: 10.1016/j.sjbs.2022.02.053
ElSayed, A. I., Rafudeen, M. S., Gomaa, A. M., and Hasanuzzaman, M. (2021). Exogenous melatonin enhances the ROS metabolism, antioxidant defense-related gene expression and photosynthetic capacity of Phaseolus vulgaris L. to confer salt stress tolerance. Physiol. Plant. 173, 1369–1381. doi: 10.1111/ppl.13372
Etikala, B., Adimalla, N., Madhav, S., Somagouni, S. G., and Keshava Kiran Kumar, P. L. (2021). “Salinity problems in groundwater and management strategies in arid and semi-arid regions” in Groundwater Geochemistry: Pollution and Remediation Methods. eds. S. Madhav and P. Singh (Nova Jersey: John Wiley and Sons), 42–56.
Fabregas, N., Lozano-Elena, F., Blasco-Escamez, D., Tohge, T., Martinez-Andujar, C., Albacete, A., et al. (2018). Overexpression of the vascular brassinosteroid receptor BRL3 confers drought resistance without penalizing plant growth. Nat. Commun. 9:4680. doi: 10.1038/s41467-018-06861-3
Fahad, S., Hussain, S., Matloob, A., Khan, F. A., Khaliq, A., and Saud, S. (2015). Phytohormones and plant responses to salinity stress: a review. Plant Growth Regul. 75, 391–404. doi: 10.1007/s10725-014-0013-y
FAO (2017). Report of the 25th session of the committee on Agriculture (Rome, 21–30 September, 2016). Available at: http://www.fao.org/3/a-mr949e.pdf
FAO and ITPS (2015). “Status of the World's Soil Resources—Main Report,” in FAO (Rome: FAO and ITPS). eds. L. Montanarella, M. Badraoui, V. Chude, I. D. S. B. Costa, T. Mamo and M. Yemefack, et al.
Farhadi, N., and Ghassemi-Golezani, K. (2020). Physiological changes of Mentha pulegium in response to exogenous salicylic acid under salinity. Sci. Hortic. 267:109325. doi: 10.1016/j.scienta.2020.109325
Feng, W., Kita, D., Peaucelle, A., Cartwright, H. N., Doan, V., Duan, Q., et al. (2018). The FERONIA receptor kinase maintains cell-wall integrity during salt stress through Ca2+ signaling. Curr. Biol. 28, 666–675.e5. doi: 10.1016/j.cub.2018.01.023
Fitzgerald, T. L., Waters, D. L. E., and Henry, R. J. (2008). The effect of salt on betaine aldehyde dehydrogenase transcript levels and 2-acetyl-1-pyrroline concentration in fragrant and non-fragrant rice (Oryza sativa). Plant Sci. 175, 539–546. doi: 10.1016/j.plantsci.2008.06.005
Flowers, T. J., and Colmer, T. D. (2008). Salinity tolerance in halophytes. New Phytol. 179, 945–963. doi: 10.1111/j.1469-8137.2008.02531.x
Forde, B. G., and Lea, P. J. (2007). Glutamate in plants: metabolism, regulation, and signalling. J. Experimental Bot. 58, 2339–2358. doi: 10.1093/jxb/erm121
Forster, B. (2001). Mutation genetics of salt tolerance in barley: an assessment of Golden promise and other semi-dwarf mutants. Euphytica 120, 317–328. doi: 10.1023/A:1017592618298
Galili, G. (2011). The aspartate-family pathway of plants: linking production of essential amino acids with energy and stress regulation. Plant Signal. Behav. 6, 192–195. doi: 10.4161/psb.6.2.14425
Galston, A. W. (1983). Polyamines as modulators of plant development. Bioscience 33, 382–388. doi: 10.2307/1309107
Gangola, M. P., and Ramadoss, B. R. (2018). “Sugars play a critical role in abiotic stress tolerance in plants,” in Biochemical, Physiological and Molecular Avenues for Combating Abiotic Stress Tolerance in Plants. ed. S. H. Wani (Amsterdam, The Netherlands: Academic Press), 17–38.
Garg, A. K., Kim, J. K., Owens, T. G., Ranwala, A. P., Do Choi, Y., Kochian, L. V., et al. (2002). Trehalose accumulation in rice plants confers high tolerance levels to different abiotic stresses. P. Natl. Acad. Sci. U. S. A. 99, 15898–15903. doi: 10.1073/pnas.252637799
Garthwaite, A. J., von Bothmer, R., and Colmer, T. D. (2005). Salt tolerance in wild Hordeum species is associated with restricted entry of Na+ and cl−into the shoots. J. Exp. Bot. 56, 2365–2378. doi: 10.1093/jxb/eri229
Ge, L. F., Chao, D. Y., Shi, M., Zhu, M. Z., Gao, J. P., and Lin, H. X. (2008). Overexpression of the trehalose-6-phosphate phosphatase gene OsTPP1 confers stress tolerance in rice and results in the activation of stress responsive genes. Planta 228, 191–201. doi: 10.1007/s00425-008-0729-x
Gharbi, E., Martínez, J. P., Benahmed, H., Dailly, H., Quinet, M., and Lutts, S. (2017). The salicylic acid analog 2, 6-dichloroisonicotinic acid has specific impact on the response of the halophyte plant species Solanum chilense to salinity. Plant Growth Regul. 82, 517–525. doi: 10.1007/s10725-017-0278-z
Ghosh, U. K., Islam, M. N., Siddiqui, M. N., Cao, X., and Khan, M. A. R. (2022). Proline, a multifaceted signalling molecule in plant responses to abiotic stress: understanding the physiological mechanisms. Plant Biol. 24, 227–239. doi: 10.1111/plb.13363
Gill, S. S., and Tuteja, N. (2010a). Polyamines and abiotic stress tolerance in plants. Plant Signal. Behav. 5, 26–33. doi: 10.4161/psb.5.1.10291
Gill, S. S., and Tuteja, N. (2010b). Reactive oxygen species and anti-oxidant machinery in abiotic stress tolerance in crop plants. Plant Physiol. Biochem. 48, 909–930. doi: 10.1016/j.plaphy.2010.08.016
Gong, Z. (2021). Plant abiotic stress: new insights into the factors that activate and modulate plant responses. J. Integr. Plant Biol. 63, 429–430. doi: 10.1111/jipb.13079
Gul, A., Khan, M. S., Ullah, M., and Munir, I. (2022). Polyethylene glycol (peg) mediated in vitro characterization of sugarcane (CP-77/400) Calli and Regenerated plantlets. Sarhad J. Agric. 38, 1–10. doi: 10.17582/journal.sja/2022/38.1.01.10
Hagihara, M., Takei, A., Ishii, T., Hayashi, F., Kubota, K., Wakamatsu, K., et al. (2012). Inhibitory effects of choline-O-sulfate on amyloid formation of human islet amyloid polypeptide. FEBS Open Bio. 2, 20–25. doi: 10.1016/j.fob.2012.02.001
Hamada, A. M. (1995). Alleviation of the adverse effects of NaCl on germination, seedling, growth and metabolic activities of maize plants by calcium salts. Bull Fac. Sci. Assiut. Univ. 24, 211–220.
Hanafy, M. S., El-Banna, A., Schumacher, H. M., Jacobsen, H. J., and Hassan, F. S. (2013). Enhanced tolerance to drought and salt stresses in transgenic faba bean (Vicia faba L.) plants by heterologous expression of the PR10a gene from potato. Plant Cell Rep. 32, 663–674. doi: 10.1007/s00299-013-1401-x
Handa, N., Kohli, S. K., Kaur, R., Sharma, A., Kumar, V., Thukral, A. K., et al. (2018). “Role of compatible solutes in enhancing antioxidative defense in plants exposed to metal toxicity” in Plants Under Metal and Metalloid Stress. eds. M. Hasanuzzaman, K. Nahar, and M. Fujita (Singapore: Springer), 207–228.
Hanson, A. D., Rathinasabapathi, B., Rivoal, J., Burnet, M., Dillon, M. O., and Gage, D. A. (1994). Osmoprotective compounds in the Plumbaginaceae: a natural experiment in metabolic engineering of stress tolerance. Proc. Natl. Acad. Sci. U. S. A. 91, 306–310. doi: 10.1073/pnas.91.1.306
Harpreet, K., Poonam, S., and Geetika, S. (2013). Sugar accumulation and its regulation by jasmonic acid in Brassica napusiL. under salt stress. J. Stress Physiol. Biochem. 9, 53–64.
Haruta, M., and Sussman, M. R. (2012). The effect of a genetically reduced plasma membrane protonmotive force on vegetative growth of Arabidopsis. Plant Physiol. 158, 1158–1171. doi: 10.1104/pp.111.189167
Hasanuzzaman, M., Alam, M. M., Rahman, A., Hasanuzzaman, M., Nahar, K., and Fujita, M. (2014). Exogenous proline and glycine betaine mediated upregulation of antioxidant defense andglyoxalyase systems provides better protection against salt-induced oxidative stress in two rice (Oryza sativa L.) varieties. Biomed. Res. Int. 757219. doi: 10.1155/2014/757219
Hasanuzzaman, M., Anee, T. I., Bhuiyan, T. F., Nahar, K., and Fujita, M. (2019). “Emerging role of osmolytes in enhancing abiotic stress tolerance in rice,” in Advances in Rice Research for Abiotic Stress Tolerance. eds. M. Hasanuzzaman, M. Fujita, K. Nahar, and J. K. Biswas (Amsterdam: Woodhead Publications), 677–708.
Hassini, I., Baenas, N., Moreno, D. A., Carvajal, M., Boughanmi, N., and Martinez Ballesta, M. D. C. (2017). Effects of seed priming, salinity and methyl jasmonate treatment on bioactive composition of Brassica oleracea var. capitata (white and red varieties) sprouts. J. Sci. Food Agr. 97, 2291–2299. doi: 10.1002/jsfa.8037
Hayat, S., Hayat, Q., Alyemeni, M. N., Wani, A. S., Pichtel, J., and Ahmad, A. (2012). Role of proline under changing environments: a review. Plant Signal. Behav. 7, 1456–1466. doi: 10.4161/psb.21949
Hazra, A., Dasgupta, N., Sengupta, C., and Das, S. (2019). MIPS: functional dynamics in evolutionary pathways of plant kingdom. Genomics 111, 1929–1945. doi: 10.1016/j.ygeno.2019.01.004
Hu, L., Lu, H., Liu, Q., Chen, X., and Jiang, X. (2005). Overexpression of mtlD gene in transgenic Populus tomentosa improves salt tolerance through accumulation of mannitol. Tree Physiol. 25, 1273–1281. doi: 10.1093/treephys/25.10.1273
Hu, L., Zhou, K., Liu, Y., Yang, S., Zhang, J., Gong, X., et al. (2020). Overexpression of MdMIPS1 enhances salt tolerance by improving osmosis, ion balance, and antioxidant activity in transgenic apple. Plant Sci. 301:110654. doi: 10.1016/j.plantsci.2020.110654
Huang, J., Hirji, R., Adam, L., Rozwadowski, K. L., Hammerlindl, J. K., Keller, W. A., et al. (2000). Genetic engineering of glycinebetaine production toward enhancing stress tolerance in plants: metabolic limitations. Plant Physiol. 122, 747–756.
Hyoung, S., Cho, S. H., Chung, J. H., So, W. M., Cui, M. H., and Shin, J. S. (2019). Cytokinin oxidase PpCKX1 plays regulatory roles in development and enhances dehydration and salt tolerance in Physcomitrella patens. Plant Cell Rep. 39, 419–430. doi: 10.1007/s00299-019-02500-3
Ibrahim, E. A. (2016). Seed priming to alleviate salinity stress in germinating seeds. J. Plant Physiol. 192, 38–46. doi: 10.1016/j.jplph.2015.12.011
Iordachescu, M., and Imai, R. (2008). Trehalose biosynthesis in response to abiotic stresses. J. Integr. Plant Biol. 50, 1223–1229. doi: 10.1111/j.1744-7909.2008.00736.x
Iqbal, N., Umar, S., and Khan, N. A. (2015). Nitrogen availability regulates proline and ethylene production and alleviates salinity stress in mustard (Brassica juncea). J. Plant Physiol. 178, 84–91. doi: 10.1016/j.jplph.2015.02.006
Iqbal, N., Umar, S., Khan, N. A., and Khan, M. I. R. (2014). A new perspective of phytohormones in salinity tolerance: regulation of proline metabolism. Environ. Exp. Bot. 100, 34–42. doi: 10.1016/j.envexpbot.2013.12.006
Isayenkov, S. V., and Maathuis, F. J. (2019). Plant salinity stress: many unanswered questions remain. Front. Plant Sci. 10:80. doi: 10.3389/fpls.2019.00080
Jahan, B., Iqbal, N., Fatma, M., Sehar, Z., Masood, A., Sofo, A., et al. (2021). Ethylene supplementation combined with split application of nitrogen and sulfur protects salt-inhibited photosynthesis through optimization of proline metabolism and antioxidant system in mustard (Brassica juncea L.). Plan. Theory 10:1303. doi: 10.3390/plants10071303
James, R. A., von Caemmerer, S., Condon, A. T., Zwart, A. B., and Munns, R. (2008). Genetic variation in tolerance to the osmotic stress component of salinity stress in durum wheat. Funct. Plant Biol. 35, 111–123. doi: 10.1071/FP07234
Jangra, M., Devi, S., Kumar, N., Goyal, V., and Mehrotra, S. (2022). Amelioration effect of salicylic acid under salt stress in Sorghum bicolor L. Appl. Biol. Biotech. 194:3802. doi: 10.1007/s12010-022-03915-7
Jethva, P. N., and Udgaonkar, J. B. (2018). The osmolyte TMAO modulates protein folding cooperativity by altering global protein stability. Biochemistry 57, 5851–5863. doi: 10.1021/acs.biochem.8b00698
Jiang, Z., Zhou, X., Tao, M., Yuan, F., Liu, L., Wu, F., et al. (2019). Plant cell-surface GIPC sphingolipids sense salt to trigger Ca2+ influx. Nature 572, 341–346. doi: 10.1038/s41586-019-1449-z
Kaplan, F., and Guy, C. L. (2004). β-Amylase induction and the protective role of maltose during temperature shock. Plant Physiol. 135, 1674–1684. doi: 10.1104/pp.104.040808
Kaplan, F., Sung, D. Y., and Guy, C. L. (2006). Roles of β-amylase and starch breakdown during temperatures stress. Physiol. Plantarum 126, 120–128. doi: 10.1111/j.1399-3054.2006.00604.x
Kapoor, K., and Srivastava, A. (2010). Assessment of salinity tolerance of Vigna mungo Var. Pu-19 using ex vitro and in vitro methods. Asian J. Biotechnol. 2, 73–85. doi: 10.3923/ajbkr.2010.73.85
Karimi, R., and Ershadi, A. (2015). Role of exogenous abscisic acid in adapting of ‘Sultana’grapevine to low-temperature stress. Acta Physiol. Plant. 37:151. doi: 10.1007/s11738-015-1902-z
Kathuria, H., Giri, J., Nataraja, K. N., Murata, N., Udayakumar, M., and Tyagi, A. K. (2009). Glycinebetaine-induced water-stress tolerance in codA-expressing transgenic indica rice is associated with up-regulation of several stress responsive genes. Plant Biotechnol. J. 7, 512–526. doi: 10.1111/j.1467-7652.2009.00420.x
Kaya, C., Sonmez, O., Aydemir, S., Ashraf, M., and Dikilitas, M. (2013). Exogenous application of mannitol and thiourea regulates plant growth and oxidative stress responses in salt-stressed maize (Zea mays L.). J. Plant Interact. 8, 234–241. doi: 10.1080/17429145.2012.725480
Kerepesi, I., and Galiba, G. (2000). Osmotic and salt stress-induced alteration in soluble carbohydrate content in wheat seedlings. Crop Sci. 40, 482–487. doi: 10.2135/cropsci2000.402482x
Khan, M. I. R., Asgher, M., and Khan, N. A. (2014). Alleviation of salt-induced photosynthesis and growth inhibition by salicylic acid involves glycine betaine and ethylene in mung bean (Vigna radiata L.). Plant Physiol. Biochem. 80, 67–74. doi: 10.1016/j.plaphy.2014.03.026
Khan, M. I. R., Fatma, M., Per, T. S., Anjum, N. A., and Khan, N. A. (2015). Salicylic acid-induced abiotic stress tolerance and underlying mechanisms in plants. Front. Plant Sci. 6:462. doi: 10.3389/fpls.2015.00462
Khodary, S. E. A. (2004). Effect of salicylic acid on the growth, photosynthesis and carbohydrate metabolism in salt stressed maize plants. Int. J. Agric. Biol. 6, 5–8.
Kim, B. G., Waadt, R., Cheong, Y. H., Pandey, G. K., Dominguez-Solis, J. R., Schultke, S., et al. (2007). The calcium sensor CBL10 mediates salt tolerance by regulating ion homeostasis in Arabidopsis. Plant J. 52, 473–484. doi: 10.1111/j.1365-313X.2007.03249.x
Kosar, F., Akram, N. A., Sadiq, M., Al-Qurainy, F., and Ashraf, M. (2018). Trehalose: A key organic osmolyte effectively involved in plant abiotic stress tolerance. J. Plant Growth Regul. 38, 606–618. doi: 10.1007/s00344-018-9876-x
Koyro, H. W., Ahmad, P., and Geissler, N. (2012). “Abiotic stress responses in plants: an overview” in Environmental Adaptations and Stress Tolerance of Plants in the Era of Climate Change. eds. P. Ahmad and M. Prasad (New York, NY: Springer), 1–28.
Krasensky, J., Broyart, C., Rabanal, F. A., and Jonak, C. (2014). The redox-sensitive chloroplast trehalose-6-phosphate phosphatase AtTPPD regulates salt stress tolerance. Antioxid. Redox. Sign. 21, 1289–1304. doi: 10.1089/ars.2013.5693
Kumar, K., Kumar, M., Kim, S. R., Ryu, H., and Cho, Y. G. (2013). Insights into genomics of salt stress response in rice. Rice 6, 1–15. doi: 10.1186/1939-8433-6-27
Kumar, R., and Srivastava, M. (2018). Study of growth and antioxidant enzymes in Andrographis paniculata (Burm f.) wall ex Nees. As influenced by salinity and alkalinity. Int. J. Agric. Environ. Biotech. 11, 525–530. doi: 10.30954/0974-1712.06.2018.14
Kurepin, L. V., Ivanoov, A. G., Zaman, M., Pharis, R. P., Allakhverdiev, S. I., Hurry, V., et al. (2015). Stress-related hormones and glycine betaine interplay in protection of photosynthesis under abiotic stress conditions. Photosynth. Res. 126, 221–235. doi: 10.1007/s11120-015-0125-x
Kurepin, L. V., Ivanov, A. G., Zaman, M., Pharis, R. P., Hurry, V., and Hüner, N. P. A. (2017). “Interaction of glycine Betaine and plant hormones: protection of the photosynthetic apparatus during abiotic stress” in Photosynthesis: Structures, Mechanisms, and Applications. eds. H. Hou, M. Najafpour, G. Moore, and S. Allakhverdiev (Cham: Springer), 185–202.
Kusano, M., Tabuchi, M., Fukushima, A., Funayama, K., Diaz, C., Kobayashi, M., et al. (2011). Metabolomics data reveal a crucial role of cytosolic glutamine synthetase 1; 1 in coordinating metabolic balance in rice. Plant J. 66, 456–466. doi: 10.1111/j.1365-313X.2011.04506.x
Kuznetsov, V., Shorina, M., Aronova, E., Stetsenko, L., Rakitin, V., and Shevyakova, N. (2007). NaCl-and ethylene-dependent cadaverine accumulation and its possible protective role in the adaptation of the common ice plant to salt stress. Plant Sci. 172, 363–370. doi: 10.1016/j.plantsci.2006.09.012
Lauchli, A., James, A. R., Huang, X. C., McCully, M., and Munns, R. (2008). Cell-specific localization of Na+ in roots of durum wheat and possible control points for salt exclusion. Plant Cell Environ. 31, 1565–1574. doi: 10.1111/j.1365-3040.2008.01864.x
Lea, P. J., Sodek, L., Parry, M. A., Shewry, P. R., and Halford, N. G. (2007). Asparagine in plants. Ann. Appl. Biol. 150, 1–26. doi: 10.1111/j.1744-7348.2006.00104.x
Lee, K. S., Choi, W. Y., Ko, J. C., Kim, T. S., and Gregorio, G. B. (2003). Salinity tolerance of japonica and indica rice (Oryza sativa L.) at the seedling stage. Planta 216, 1043–1046. doi: 10.1007/s00425-002-0958-3
Lee, D. G., Park, K. W., An, J. Y., Sohn, Y. G., Ha, J. K., Kim, H. Y., et al. (2011). Proteomics analysis of salt-induced leaf proteins in two rice germplasms with different salt sensitivity. Can. J. Plant Sci. 91, 337–349. doi: 10.4141/CJPS10022
Lee, B. R., Zhang, Q., Park, S. H., Islam, M. T., and Kim, T. H. (2019). Salicylic acid improves drought-stress tolerance by regulating the redox status and proline metabolism in Brassica rapa. Hortic. Environ. Biote. 60, 31–40. doi: 10.1007/s13580-018-0099-7
Lei, P., Xu, Z., Liang, J., Luo, X., Zhang, Y., Feng, X., et al. (2016). Poly (γ-glutamic acid) enhanced tolerance to salt stress by promoting proline accumulation in Brassica napus L. Plant Growth Regul. 78, 233–241. doi: 10.1007/s10725-015-0088-0
Leyman, B., Van Dijck, P., and Thevelein, J. M. (2001). An unexpected plethora of trehalose biosynthesis genes in Arabidopsis thaliana. Trends Plant Sci. 6, 510–513. doi: 10.1016/S1360-1385(01)02125-2
Li, L., Dou, N., Zhang, H., and Wu, C. (2021). The versatile GABA in plants. Plant Sign. Behav. 16:1862565. doi: 10.1080/15592324.2020.1862565
Li, Y., and He, J. (2012). Advance in metabolism and response to stress of polyamines in plant. Acta Agric. Boreali Sinica 27, 240–245. doi: 10.3969/j.issn.1000-7091.2012.z1.048
Li, T., Hu, Y., Du, X., Tang, H., Shen, C., and Wu, J. (2014). Salicylic acid alleviates the adverse effects of salt stress in Torreyagrandis cv. Merrillii seedlings by activating photosynthesis and enhancing anti-oxidant systems. PLoS One 9:e109492. doi: 10.1371/journal.pone.0109492
Li, C. Z., Jiao, J., and Wang, G.-X. (2004). The important roles of reactive oxygen species in the relationship between ethylene and polyamines in leaves of spring wheat seedlings under root osmotic stress. Plant Sci. 166, 303–315. doi: 10.1016/j.plantsci.2003.09.019
Li, D., Zhang, T., Wang, M., Liu, Y., Brestic, M., Chen, T. H., et al. (2019). Genetic engineering of the biosynthesis of glycine betaine modulates phosphate homeostasis by regulating phosphate acquisition in tomato. Front. Plant Sci. 9:1995. doi: 10.3389/fpls.2018.01995
Liu, G., Jiang, W., Tian, L., Fu, Y., Tan, L., Zhu, Z., et al. (2022). Polyamine oxidase 3 is involved in salt tolerance at the germination stage in rice. J. Genet. Genomics 49, 458–468. doi: 10.1016/j.jgg.2022.01.007
Liu, J., Jiang, M. Y., Zhou, Y. F., and Liu, Y. L. (2005). Production of polyamines is enhanced by endogenous abscisic acid in maize seedlings subjected to salt stress. J. Integr. Plant Biol. 47, 1326–1334. doi: 10.1111/j.1744-7909.2005.00183.x
Liu, T., Kim, D. W., Niitsu, M., Berberich, T., and Kusano, T. (2014a). Oryza sativa polyamine oxidase 1 back-converts tetraamines, spermine and thermospermine, to spermidine. Plant Cell Rep. 33, 143–151. doi: 10.1007/s00299-013-1518-y
Liu, T., Kim, D. W., Niitsu, M., Maeda, S., Watanabe, M., Kamio, Y., et al. (2014b). Polyamine oxidase 7 is a terminal catabolism-type enzyme in Oryza sativa and is specifically expressed in anthers. Plant Cell Physiol. 55, 1110–1122. doi: 10.1093/pcp/pcu047
Llebres, M. T., Pascual, M. B., Debille, S., Trontin, J. F., Harvengt, L., Avila, C., et al. (2018). The role of arginine metabolic pathway during embryogenesis and germination in maritime pine (Pinus pinasterAvit.). Tree Physiol. 38, 471–484. doi: 10.1093/treephys/tpx133
Loescher, W. H., Tyson, R. H., Everard, J. D., Redgwell, R. J., and Bieleski, R. L. (1992). Mannitol synthesis in higher plants: evidence for the role and characterization of a NADPH-dependent mannose 6-phosphate reductase. Plant Physiol. 98, 1396–1402. doi: 10.1104/pp.98.4.1396
Lomelino, C. L., Andring, J. T., McKenna, R., and Killberg, M. S. (2017). Asparagine synthetase: function, structure, and role in disease. J. Biol. Chem. 292, 19952–19958. doi: 10.1074/jbc.R117.819060
López-Gómez, M. L., and Lluch, C. (2012). “Trehalose and abiotic stress tolerance” in Abiotic Stress Responses in Plants: Metabolism, Productivity and Sustainability. eds. P. Ahmad and M. N. V. Prasad (New York, NY: Springer), 253–265.
Lu, K. X., Cao, B. H., Feng, X. P., He, Y., and Jiang, D. A. (2009). Photosynthetic response of salt-tolerant and sensitive soybean varieties. Photosynthetica 47, 381–387. doi: 10.1007/s11099-009-0059-7
Lunn, J. E., Delorge, I., Figueroa, C. M., Van Dijick, P., and Stitt, M. (2014). Trehalose metabolism in plants. Plant J. 79, 544–567. doi: 10.1111/tpj.12509
Lv, D. W., Zhu, G. R., Zhu, D., Bian, Y. W., Liang, X. N., Cheng, Z. W., et al. (2016). Proteomic and phosphoproteomic analysis reveals the response and defense mechanism in leaves of diploid wheat T. monococcum under salt stress and recovery. J. Proteome 143, 93–105. doi: 10.1016/j.jprot.2016.04.013
Machado, R. M. A., and Serralheiro, R. P. (2017). Soil salinity: effect on vegetable crop growth. Management practices to prevent and mitigate soil salinization. Horticulturae 3:30. doi: 10.3390/horticulturae3020030
Mahajan, S., and Tuteja, N. (2005). Cold, salinity and drought stresses: an overview. Arch. Biochem. Biophys. 444, 139–158. doi: 10.1016/j.abb.2005.10.018
Majee, M., Maitra, S., Dastidar, K. G., Pattnaik, S., Chatterjee, A., Hait, N. C., et al. (2004). A novel salt-tolerant L-myo-inositol-1-phosphate synthase from Porteresiacoarctata (Roxb.) Tateoka, a halophytic wild rice: molecular cloning, bacterial overexpression, characterization, and functional introgression into tobacco-conferring salt tolerance phenotype. J. Biol. Chem. 279, 28539–28552. doi: 10.1074/jbc.M310138200
Majumder, A. L., Chatterjee, A., Ghosh Dastider, K., and Majee, M. (2003). Diversification and evolution of L-myo-inositol 1-phosphate synthase. FEBS Lett. 553, 3–10. doi: 10.1016/S0014-5793(03)00974-8
Majumder, A. L., Johnson, M. D., and Henry, S. A. (1997). 1L-myo-inositol1-phosphate synthase. Biochim. Biophys. Acta 1348, 245–256. doi: 10.1016/S0005-2760(97)00122-7
Mallahi, T., Saharkhiz, M. J., and Javanmardi, J. (2018). Salicylic acid changes morpho-physiological attributes of feverfew (Tanacetum parthenium L.) under salinity stress. Acta Ecol. Sin. 38, 351–355. doi: 10.1016/j.chnaes.2018.02.003
Mansour, M. M. F., and Ali, E. F. (2017). Evaluation of proline functions in saline conditions. Phytochemistry 140, 52–68. doi: 10.1016/j.phytochem.2017.04.016
Mansour, M. M. F., and Hassan, F. A. (2022). How salt stress-responsive proteins regulate plant adaptation to saline conditions? Plant Mol. Biol. 108, 175–224. doi: 10.1007/s11103-021-01232-x
Marco, F., Bitrián, M., Carrasco, P., Alcázar, R., and Tiburcio, A. F. (2015). “Polyamine biosynthesis engineering as a tool to improve plant resistance to abiotic stress,” in Genetic Manipulation in Plants for Mitigation of Climate Change. eds. P. Jaiwal, R. Singh, and O. Dhankher (New Delhi: Springer), 103–116.
Marco, F., Buso, E., Lafuente, T., and Carrasco, P. (2019). Spermine confers stress resilience by modulating abscisic acid biosynthesis and stress responses in Arabidopsis plants. Front. Plant Sci. 10:972. doi: 10.3389/fpls.2019.00972
Memon, S. A., Hou, X., and Wang, L. J. (2010). Morphological analysis of salt stress response of pak Choi. EJEAFChe 9, 248–254.
Meng, D., Hou, L., and Yang, S. (2015). Exogenous polyamines alleviating salt stress on peanuts (Arachis hypogaea) grown in pots. Chin. J. Plant Eco. 39, 1209–1215. doi: 10.17521/cjpe.2015.0117
Misra, N., and Misra, R. (2012). Salicylic acid changes plant growth parameters and proline metabolism in Rauwolfia serpentina leaves grown under salinity stress. Am. Eurasian J. Agric. Environ. Sci. 12, 1601–1609.
Misra, N., and Saxena, P. (2009). Effect of salicylic acid on proline metabolism in lentil grown under salinity stress. Plant Sci. 177, 181–189. doi: 10.1016/j.plantsci.2009.05.007
Miyashita, Y., Dolferus, R., Ismond, K. P., and Good, A. G. (2007). Alanine aminotransferase catalyses the breakdown of alanine after hypoxia in Arabidopsis thaliana. Plant J. 49, 1108–1121. doi: 10.1111/j.1365-313X.2006.03023.x
Moravcová, Š., Tuma, J., Duˇcaiová, Z. K., Waligórski, P., Kula, M., Saja, D., et al. (2018). Influence of salicylic acid pretreatment on seeds germination and some defence mechanisms of Zea mays plants under copper stress. Plant Physiol. Biochem. 122, 19–30. doi: 10.1016/j.plaphy.2017.11.007
Moschou, P. N., Paschalidis, K. A., Delis, I. D., Andriopoulou, A. H., Lagiotis, G. D., Yakoumakis, D. I., et al. (2008). Spermidine exodus and oxidation in the apoplast induced by abiotic stress is responsible for H2O2 signatures that direct tolerance responses in tobacco. Plant Cell 20, 1708–1724. doi: 10.1105/tpc.108.059733
Mukhopadhyay, R., Sarkar, B., Jat, H. S., Sharma, P. C., and Bolan, N. S. (2021). Soil salinity under climate change: challenges for sustainable agriculture and food security. J. Environ. Manag. 280:111736. doi: 10.1016/j.jenvman.2020.111736
Munns, R., and James, R. A. (2003). Screening methods for salinity tolerance: a case study with tetraploid wheat. Plant Soil 253, 201–218. doi: 10.1023/A:1024553303144
Munns, R., James, R. A., Gilliham, M., Flowers, T. J., and Colmer, T. D. (2016). Tissue tolerance: an essential but elusive trait for salt-tolerant crops. Funct. Plant Biol. 43, 1103–1113. doi: 10.1071/FP16187
Munns, R., and Tester, M. (2008). Mechanisms of salinity tolerance. Annu. Rev. Plant Biol. 59, 651–681. doi: 10.1146/annurev.arplant.59.032607.092911
Mustafavi, S. H., Badi, H. N., Sękara, A., Mehrafarin, A., Janda, T., Ghorbanpour, M., et al. (2018). Polyamines and their possible mechanisms involved in plant physiological processes and elicitation of secondary metabolites. Acta Physiol. Plant. 40:102. doi: 10.1007/s11738-018-2671-2
Nahar, K., Hasanuzzaman, M., and Fujita, M. (2016). “Roles of Osmolytes in plant adaptation to drought and salinity” in Osmolytes and Plants Acclimation to Changing Environment: Emerging Omics Technologies. eds. N. Iqbal, R. Nazar, and N. A. Khan (New Delhi: Springer), 37–68.
Najar, R., Aydi, S., Sassi-Aydi, S., Zarai, A., and Abdelly, C. (2019). Effect of salt stress on photosynthesis and chlorophyll fluorescence in Medicago truncatula. Plant Biosys. – An Int. J. Dealing All Aspects Plant Biol. 153, 88–97. doi: 10.1080/11263504.2018.1461701
Nazoa, P., Vidmar, J. J., Tranbarger, T. J., Mouline, K., Damiani, I., Tillard, P., et al. (2003). Regulation of the nitrate transporter gene AtNRT2.1 in Arabidopsis thaliana: responses to nitrate, amino acids and developmental stage. Plant Mol. Biol. 52, 689–703. doi: 10.1023/A:1024899808018
Negrāo, S., Schmockel, S. M., and Tester, M. (2017). Evaluating physiological responses of plants to salinity stress. Ann. Bot. 119, 1–11. doi: 10.1093/aob/mcw191
Netondo, G. W., Onyango, J. C., and Beck, E. (2004). Sorghum and salinity: II. Gas exchange and chlorophyll fluorescence of sorghum under salt stress. Crop Sci. 44, 806–811. doi: 10.2135/cropsci2004.8060
Niazian, M., Sadat-Noori, S. A., Tohidfar, M., Mortazavian, S. M. M., and Sabbatini, P. (2021). Betaine aldehyde dehydrogenase (BADH) vs. Flavodoxin (Fld): two important genes for enhancing plants stress tolerance and productivity. Front. Plant Sci. 12:695110. doi: 10.3389/fpls.2021.650215
Nisa, Z. U., Chen, C., Yu, Y., Chen, C., Mallano, A. I., Xiang-bo, D., et al. (2016). Constitutive overexpression of myoinositol-1-phosphate synthase gene (GsMIPS2) from Glycine soja confers enhanced salt tolerance at various growth stages in Arabidopsis. J. Northeast. Agric. Univ. 23, 28–44. doi: 10.1016/S1006-8104(16)30045-9
Nuccio, M. L., Wu, J., Mowers, R., Zhou, H. P., Meghji, M., Primavesi, L. F., et al. (2015). Expression of trehalose-6-phosphate phosphatase in maize ears improves yield in well-watered and drought conditions. Nat. Biotechnol. 33, 862–869. doi: 10.1038/nbt.3277
Pakzad, R., Goharrizi, K. J., Riahi-Madvar, A., Amirmahani, F., Mortazavi, M., and Esmaeeli, L. (2021). Identification of Lepidium draba Δ1-pyrroline-5-carboxylate Synthetase (P5CS) and assessment of its expression under NaCl stress: P5CS identification in L. draba plant. P. Natl. A. Sci. India 91, 195–203. doi: 10.1007/s40011-020-01207-w
Pál, M., Szalai, G., Gondor, O. K., and Janda, T. (2021). Unfinished story of polyamines: role of conjugation, transport and light-related regulation in the polyamine metabolism in plants. Plant Sci. 308:110923. doi: 10.1016/j.plantsci.2021.110923
Pal, M., Tajti, J., Szalai, G., Peeva, V., Vegh, B., and Janda, T. (2018). Interaction of polyamines, abscisic acid and proline under osmotic stress in the leaves of wheat plants. Sci. Rep.-UK 8:12839. doi: 10.1038/s41598-018-31297-6
Palma, F., Lopez-Gomez, M., Tejera, N. A., and Lluch, C. (2013). Salicylic acid improves the salinity tolerance of Medicago sativa in symbiosis with Sinorhizobiummeliloti by preventing nitrogen fixation inhibition. Plant Sci. 208, 75–82. doi: 10.1016/j.plantsci.2013.03.015
Pan, Y., Chai, X., Gao, Q., Zhou, L., Zhang, S., Li, L., et al. (2019). Dynamic interactions of plant CNGC subunits and calmodulins drive oscillatory Ca2+ channel activities. Dev. Cell 48, 710–725.e5. doi: 10.1016/j.devcel.2018.12.025
Pandey, B., and Choudhary, K. K. (2019). “Air pollution: role in climate change and its impact on crop plants,” in Climate Change and Agricultural Ecosystems. eds. K. K. Choudhary, A. Kumar, and A. K. Singh (Sawston Cambridge, UK: Woodhead Publications), 211–247.
Parida, A. K., Das, A. B., and Mohanty, P. (2004). Investigations on the antioxidative defense responses to NaCl stress in a mangrove, Bruguiera parviflora: differential regulations of isoforms of some antioxidative enzymes. Plant Growth Regul. 42, 213–226. doi: 10.1023/B:GROW.0000026508.63288.39
Park, H. J., Kim, W. Y., and Yun, D. J. (2016). A new insight of salt stress signaling in plant. Mol. Cells 39, 447–459. doi: 10.14348/molcells.2016.0083
Parthasarathy, A., Savka, M. A., and Hudson, A. O. (2019). The synthesis and role of β-alanine in plants. Front. Plant Sci. 10:921. doi: 10.3389/fpls.2019.00921
Parvaiz, A., and Satyawati, S. (2008). Salt stress and phyto-biochemical responses of plants-a review. Plant Soil Environ. 54, 89–99. doi: 10.17221/2774-PSE
Patel, K. G., Mandaliya, V. B., Mishra, G. P., Dobaria, J. R., and Thankappan, R. (2016). Transgenic peanut overexpressing mtlD gene confers enhanced salinity stress tolerance via mannitol accumulation and differentialantioxidative responses. Acta Physiol. Plant. 38, 1–14. doi: 10.1007/s11738-016-2200-0
Paul, M. J., Primavesi, L. F., Jhurreea, D., and Zhang, Y. (2008). Trehalose metabolism and signaling. Anu. Rev. Plant Biol. 59, 417–441. doi: 10.1146/annurev.arplant.59.032607.092945
Pavlu, J., Novak, J., Koukalova, V., Luklova, M., Brzobohaty, B., and Cerny, M. (2018). Cytokinin at the crossroads of abiotic stress signalling pathways. Int. J. Mol. Sci. 19:2450. doi: 10.3390/ijms19082450
Per, T. S., Khan, M. I. R., Anjum, N. A., Masood, A., Hussain, S. J., and Khan, N. A. (2018). Jasmonates in plants under abiotic stresses: crosstalk with other phytohormones matters. Environ. Exp. Bot. 145, 104–120. doi: 10.1016/j.envexpbot.2017.11.004
Per, T. S., Khan, N. A., Reddy, P. S., Masood, A., Hasanuzzaman, M., Khan, M. I. R., et al. (2017). Approaches in modeling proline metabolism in plants for salt and drought tolerance: phytohoromes, mineral nutrients and transgenics. Plant Physiol. Biochem. 115, 126–140. doi: 10.1016/j.plaphy.2017.03.018
Petruzzelli, L., Coraggio, I., and Leubner-Metzger, G. (2000). Ethylene promotes ethylene biosynthesis during pea seed germination by positive feedback regulation of 1-aminocyclo-propane-1-carboxylic acid oxidase. Planta 211, 144–149. doi: 10.1007/s004250000274
Planchet, E., Verdu, I., Delahaie, J., Cukier, C., Girard, C., Morere-Le Paven, M. C., et al. (2014). Abscisic acid-induced nitric oxide and proline accumulation in independent pathways under water-deficit stress during seedling establishment in Medicago truncatula. J. Exp. Bot. 65, 2161–2170. doi: 10.1093/jxb/eru088
Ponnu, J., Wahl, V., and Schmid, M. (2011). Trehalose-6-phosphate: connecting plant metabolism and development. Front. Plant Sci. 2:70. doi: 10.3389/fpls.2011.00070
Poonam, R. B., Handa, N., Kaur, H., Rattan, A., Bali, S., Gautam, V., et al. (2016). Sugar signalling in plants: a novel mechanism fordrought stress management. Water Stress Crop Plants 11, 287–302. doi: 10.1002/9781119054450.ch19
Prabhavathi, V., Yadav, J. S., Kumar, P. A., and Rajam, M. V. (2002). Abiotic stress tolerance in transgenic eggplant (Solanum melongena L.) by introduction of bacterial mannitol phosphodehydrogenase gene. Mol. Breeding 9, 137–147. doi: 10.1023/A:1026765026493
Prodjinoto, H., Irakoze, W., Gandonou, C., Lepoint, G., and Lutts, S. (2021). Discriminating the impact of Na+ and cl− in the deleterious effects of salt stress on the African rice species (Oryza glaberrimaSteud.). Plant Growth Regul. 94, 201–219. doi: 10.1007/s10725-021-00709-5
Rabbani, G., and Choi, I. (2018). Roles of osmolytes in protein folding and aggregation in cells and their biotechnological applications. Int. J. Biol. Sci. 109, 483–491. doi: 10.1016/j.ijbiomac.2017.12.100
Rady, M. M., Elrys, A. S., El-Maati, M. F. A., and Desoky, E. S. M. (2019). Interplaying roles of silicon and proline effectively improve salt and cadmium stress tolerance in Phaseolus vulgaris plant. Plant Physiol. Bioch. 139, 558–568. doi: 10.1016/j.plaphy.2019.04.025
Raines, C. A. (2003). The Calvin cycle revisited. Photosynth. Res. 75, 1–10. doi: 10.1023/A:1022421515027
Ranganayakulu, G. S., Veeranagamallaiah, G., and Chinta, S. (2013). Effect of salt stress on osmolyte accumulation in two groundnut cultivars (Arachishypogaea L.) with contrasting salt tolerance. African J. Plant Sci. 7, 586–592. doi: 10.5897/AJPS11.063
Rao, A. Q., Ud Din, S., Akhtar, S., Sarwar, M. B., Ahmed, M., Rashid, B., et al. (2016). “Genomics of salinity tolerance in plants,” in Plant Genomics in Tech. ed. I. Y. Abdurakhmonov (London: BoD-Books on Demand), 273–299.
Rathor, P., Borza, T., Liu, Y., Qin, Y., Stone, S., Zhang, J., et al. (2020). Low mannitol concentrations in Arabidopsis thaliana expressing Ectocarpus genes improve salt tolerance. Plan. Theory 9:1508. doi: 10.3390/plants9111508
Rattan, A., Kapoor, D., Kapoor, N., Bhardwaj, R., and Sharma, A. (2020). Brassinosteroids regulate functional components of Antioxidative defense system in salt stressed maize seedlings. J. Plant Growth Regul. 39, 1465–1475. doi: 10.1007/s00344-020-10097-1
Riaz, M., Arif, M. S., Ashraf, M. A., Mahmood, R., Yasmeen, T., Shakoor, M. B., et al. (2019). “A comprehensive review on rice responses and tolerance to salt stress,” in Advances in Rice Research for Abiotic Stress Tolerance. eds. M. Hasannuzzaman, M. Fujita, K. Nahar and J. K. Biswas (Cambridge: Woodhead Publishing), 133–158.
Roy, S. J., Negrão, S., and Tester, M. (2014). Salt resistant crop plants. Curr. Opin. Biotechnol. 26, 115–124. doi: 10.1016/j.copbio.2013.12.004
Roychoudhury, A., and Banerjee, A. (2017). “Abscisic acid signaling and involvement of mitogen activated protein kinases and calcium-dependent protein kinases during plant abiotic stress” in Mechanism of Plant Hormone Signaling Under Stress. ed. G. K. Pandey (Hoboken, NJ: Wiley), 197–241.
Ruan, Y. L. (2014). Sucrose metabolism: gateway to diverse carbon use and sugar signaling. Annu. Rev. Plant Biol. 65, 33–67. doi: 10.1146/annurev-arplant-050213-040251
Safdar, H., Amin, A., Shafiq, Y., Ali, A., Yasin, R., Shoukat, A., et al. (2019). A review: impact of salinity on plant growth. Nat. Sci. 17, 34–40. doi: 10.7537/marsnsj170119.06
Sagervanshi, A., Naeem, A., Geilfus, C. M., Kaiser, H., and Muhling, K. H. (2021). One-time abscisic acid priming induces long-term salinity resistance in Vicia faba: changes in key transcripts, metabolites, and ionic relations. Physiol. Plant. 172, 146–161. doi: 10.1111/ppl.13315
Santos, J., Al-Azzawi, M., Aronson, J., and Flowers, T. J. (2016). HALOPH a database of salt-tolerant plants: helping put halophytes to work. Plant Cell Physiol. 57:e10. doi: 10.1093/pcp/pcv155
Savvides, A., Ali, S., Tester, M., and Fotopoulos, V. (2016). Chemical priming of plants against multiple abiotic stresses: mission possible? Trends Plant Sci. 21, 329–340. doi: 10.1016/j.tplants.2015.11.003
Schaller, G. E., Street, I. H., and Kieber, J. J. (2014). Cytokinin and the cell cycle. Curr. Opin. Plant Biol. 21, 7–15. doi: 10.1016/j.pbi.2014.05.015
Selem, E., Hassan, A. A., Awad, M. F., Mansour, E., and Desoky, E. S. M. (2022). Impact of exogenously sprayed antioxidants on Physio-biochemical, agronomic, and quality parameters of potato in salt-affected soil. Plan. Theory 11:210. doi: 10.3390/plants11020210
Sengupta, S., Patra, B., Ray, S., and Majumder, A. L. (2008). Inositol methyl transferase from a halophytic wild rice, PorteresiacoarctataRoxb. (Tateoka): regulation of pinitol synthesis under abiotic stress. Plant Cell Environ. 30, 1442–1459. doi: 10.1111/j.1365-3040.2008.01850.x
Shahid, M. A., Balal, R. M., Pervez, M. A., Garcia-Sanchez, F., Gimeno, V., Abbas, T., et al. (2014). Treatment with 24-epibrassinolide mitigates NaCl-induced toxicity by enhancing carbohydrate metabolism, osmolyte accumulation, and antioxidant activity in Pisum sativum. Turk. J. Bot. 38, 511–525. doi: 10.3906/bot-1304-45
Shahverdi, M. A., Omidi, H., and Tabatabaei, S. J. (2018). Plant growth and steviol glycosides as affected by foliar application of selenium, boron, and iron under NaCl stress in Stevia rebaudiana Bertoni. Ind. Crop. Prod. 125, 408–415. doi: 10.1016/j.indcrop.2018.09.029
Shaki, F., Maboud, H. E., and Niknam, V. (2018). Growth enhancement and salt tolerance of safflower (Carthamus tinctorius L.), by salicylic acid. Curr. Plant Biol. 13, 16–22. doi: 10.1016/j.cpb.2018.04.001
Shan, C., Zhou, Y., and Liu, M. (2015). Nitric oxide participates in the regulation of the ascorbate-glutathione cycle by exogenous jasmonic acid in the leaves of wheat seedlings under drought stress. Protoplasma 252, 1397–1405. doi: 10.1007/s00709-015-0756-y
Sharma, A., Kumar, V., Singh, R., Thukral, A. K., and Bhardwaj, R. (2016). Effect of seed pre-soaking with 24-epibrassinolide on growth and photosynthetic parameters of Brassica juncea L. in imidacloprid soil. Ecotox. Environ. Safe 133, 195–201. doi: 10.1016/j.ecoenv.2016.07.008
Shen, X., Wang, Z., Song, X., Xu, J., Jiang, C., Zhao, Y., et al. (2014). Transcriptomic profiling revealed an important role of cell wall remodeling and ethylene signaling pathway during salt acclimation in Arabidopsis. Plant Mol. Biol. 86, 303–317. doi: 10.1007/s11103-014-0230-9
Shevyakova, N., Musatenko, L., Stetsenko, L., Vedenicheva, N., Voitenko, L., Sytnik, K., et al. (2013). Effects of abscisic acid on the contents of polyamines and proline in common bean plants under salt stress. Russ. J. Plant Physiol. 60, 200–211. doi: 10.1134/S102144371301007X
Shi, H., Lee, B., Wu, S. J., and Zhu, J. K. (2003). Overexpression of a plasma membrane Na+/H+ antiporter gene improves salt tolerance in Arabidopsis thaliana. Nat. Biotechnol. 21, 81–85. doi: 10.1038/nbt766
Shrivastava, P., and Kumar, R. (2015). Soil salinity: a serious environmental issue and plant growth promoting bacteria as one of the tools for its alleviation. Saudi J. Biol. Sci. 22, 123–131. doi: 10.1016/j.sjbs.2014.12.001
Sibole, J. V., Cabot, C., Poschenrieder, C., and Barceló, J. (2003). Efficient leaf partitioning, an overriding condition for abscisic acid-controlled stomatal and leaf growth responses to NaCl salinization in two legumes. J. Exp. Bot. 54, 2111–2119. doi: 10.1093/jxb/erg231
Singh, M., Kumar, J., Singh, S., Singh, V. P., and Prasad, S. M. (2015). Roles of osmoprotectants in improving salinity and drought tolerance in plants: a review. Rev. Environ. Sci. Biotechnol. 14, 407–426. doi: 10.1007/s11157-015-9372-8
Sirault, X. R. R., James, R. A., and Furbank, R. T. (2009). A new screening method for osmotic component of salinity tolerance in cereals using infrared thermography. Funct. Plant Biol. 36, 970–977. doi: 10.1071/FP09182
Slama, I., Abdelly, C., Bouchereau, A., Flowers, T., and Savoure, A. (2015). Diversity, distribution and roles of osmoprotective compounds accumulated in halophytes under abiotic stress. Ann. Bot. 115, 433–447. doi: 10.1093/aob/mcu239
Slocum, R. D. (2005). Genes, enzymes and regulation of arginine biosynthesis in plants. Plant Physiol. Biochem. 43, 729–745. doi: 10.1016/j.plaphy.2005.06.007
Song, J., Zhou, J. C., Zhao, W. W., Xu, H. L., Wang, F. X., and Xu, Y. G. (2016). Effects of salinity and nitrate on production and germination of dimorphic seeds applied both through the mother plant and exogenously during germination in Suaeda salsa. Plant Spec. Biol. 31, 19–28. doi: 10.1111/1442-1984.12071
Sonoda, Y., Ikeda, A., Saiki, S., Yamaya, T., and Yamaguchi, J. (2003). Feedback regulation of the ammonium transporter gene family AMT1 by glutamine in rice. Plant Cell Physiol. 44, 1396–1402. doi: 10.1093/pcp/pcg169
Spreitzer, R. J. (2003). Role of the small subunit in ribulose-1, 5-bisphosphate carboxylase/oxygenase. Arch. Biochem. Biophys. 414, 141–149. doi: 10.1016/S0003-9861(03)00171-1
Strambini, G. B., and Gonnelli, M. (2008). Singular efficacy of trimethylamine N-oxide to counter protein destabilization in ice. Biochemistry 47, 3322–3331. doi: 10.1021/bi702473g
Streeter, J. G. (1985). Identification and distribution of ononitol in nodules of Pisum sativum and Glycine max. Phytochemistry 24, 174–176. doi: 10.1016/S0031-9422(00)80831-6
Sulpice, R., Tsukaya, H., Nonaka, H., Mustardy, L., Chen, T. H. H., and Murata, N. (2003). Enhanced formation of flowers in salt-stressed Arabidopsis after genetic engineering of the synthesis of glycinebetaine. Plant J. 36, 165–176. doi: 10.1046/j.1365-313X.2003.01873.x
Suo, J., Zhao, Q., David, L., Chen, S., and Dai, S. (2017). Salinity response in chloroplasts insights from gene characterization. Int. J. Mol. Sci. 18:1011. doi: 10.3390/ijms18051011
Suprasanna, P., Rai, A. N., Kumari, P., Kumar, S. A., and Kavi Kishor, P. B. (2014). “Modulation of proline: implications in plant stress tolerance and development” in Plant Adaptation to Environmental Change. eds. N. A. Anjum, S. S. Gill, and R. Gil (UK: CABI Publishers), 68–93.
Syeed, S., Sehar, Z., Masood, A., Anjum, N. A., and Khan, N. A. (2021). Control of elevated ion accumulation, oxidative stress, and lipid peroxidation with salicylic acid-induced accumulation of glycine Betaine in salinity-exposed Vigna radiata L. Appl. Biochem. Biotechnol. 193, 3301–3320. doi: 10.1007/s12010-021-03595-9
Talbi, O. Z., Slama, I., Trabelsi, N., Hamdi, A., Smaoui, A., and Abedlly, C. (2018). Combined effects of salinity and phosphorus availability on growth, gas exchange and nutrient status of Catapodiumrigidum. Arid Land Res. Manag. 32, 277–290. doi: 10.1080/15324982.2018.1427640
Thomas, J. C., McElwain, E. F., and Bohnert, H. J. (1992). Convergent induction of osmotic stress-responses: abscisic acid, cytokinin, and the effects of NaCl. Plant Physiol. 100, 416–423. doi: 10.1104/pp.100.1.416
Tian, W., Hou, C., Ren, Z., Wang, C., Zhao, F., Dahlbeck, D., et al. (2019). A calmodulin-gated calcium channel links pathogen patterns to plant immunity. Nature 572, 131–135. doi: 10.1038/s41586-019-1413-y
Tilbrook, J., Schilling, R. K., Berger, B., Garcia, A. F., Trittermann, C., Coventry, S., et al. (2017). Variation in shoot tolerance mechanisms not related to ion toxicity in barley. Funct. Plant Biol. 44, 1194–1206. doi: 10.1071/FP17049
Tognetti, J. A., Pontis, H. G., and Martinez-Noel, G. M. (2013). Sucrose signaling in plants: a world yet to be explored. Plant Signal. Behav. 8:e23316. doi: 10.4161/psb.23316
Trinchant, J. C., Boscari, A., Spennato, G., Van de Sype, G., and Le Rudulier, D. (2004). Proline betaine accumulation and metabolism in alfalfa plants under sodium chloride stress. Exploring its compartmentalization in nodules. Plant Physiol. 135, 1583–1594. doi: 10.1104/pp.103.037556
Upadhyay, R. S., Meena, M., Prasad, V., Zehra, A., and Gupta, V. K. (2015). Mannitol metabolism during pathogenic fungal–host interactions under stressed conditions. Front. Microbiol. 6:1019. doi: 10.3389/fmicb.2015.01019
Urano, K., Yoshiba, Y., Nanjo, T., Igarashi, Y., Seki, M., Sekiguchi, F., et al. (2003). Characterization of Arabidopsis genes involved in biosynthesis of polyamines in abiotic stress responses and developmental stages. Plant Cell Environ. 26, 1917–1926. doi: 10.1046/j.1365-3040.2003.01108.x
Urano, K., Yoshiba, Y., Nanjo, T., Ito, T., Yamaguchi-Shinozaki, K., and Shinozaki, K. (2004). Arabidopsis stress-inducible gene for arginine decarboxylase AtADC2 is required for accumulation of putrescine in salt tolerance. Biochem. Biophys. Res. Commun. 313, 369–375. doi: 10.1016/j.bbrc.2003.11.119
Valluru, R., and Van den Ende, W. (2011). Myo-inositol and beyond–emerging networks under stress. Plant Sci. 181, 387–400. doi: 10.1016/j.plantsci.2011.07.009
Verbruggen, N., and Hermans, C. (2008). Proline accumulation in plants: a review. Amino Acids 35, 753–759. doi: 10.1007/s00726-008-0061-6
Verslues, P. E., and Bray, E. A. (2005). Role of abscisic acid (ABA) and Arabidopsis thaliana ABA-insensitive loci in low water potential-induced ABA and proline accumulation. J. Exp. Bot. 57, 201–212. doi: 10.1093/jxb/erj026
Vidmar, J. J., Zhuo, D., Siddiqi, M. Y., Schoerring, J. K., Touraine, B., and Glass, A. D. M. (2000). Regulation of high affinity nitrate transporter genes and high affinity nitrate influx by nitrogen pools in plant roots. Plant Physiol. 123, 307–318. doi: 10.1104/pp.123.1.307
Vincentz, M., Moureaux, T., Leydecker, M. T., Vaucheret, H., and Caboche, M. (1993). Regulation of nitrate and nitrite reductase expression in Nicotiana plumbaginifolialeaves by nitrogen and carbon metabolites. Plant J. 3, 315–324. doi: 10.1111/j.1365-313X.1993.tb00183.x
Vinocur, B., and Altman, A. (2005). Recent advances in engineering plant tolerance to abiotic stress: achievements and limitations. Curr. Opin. Biotech. 16, 123–132. doi: 10.1016/j.copbio.2005.02.001
Wang, W., Paschalidis, K., Feng, J. C., Song, J., and Liu, J. H. (2019). Polyamine catabolism in plants: a universal process with diverse functions. Front. Plant Sci. 10:561. doi: 10.3389/fpls.2019.00561
Wang, F. W., Wang, M. L., Guo, C., Wang, N., Li, X. W., Chen, H., et al. (2016). Cloning and characterization of a novel betaine aldehyde dehydrogenase gene from Suaedacorniculata. Genet. Mol. Res. 15:gmr.15027848. doi: 10.4238/gmr.15027848
Wani, A. S., Ahmad, A., Hayat, S., and Tahir, I. (2019). Epibrassinolide and proline alleviate the photosynthetic and yield inhibition under salt stress by acting on anti-oxidant system in mustard. Plant Physiol. Bioch. 135, 385–394. doi: 10.1016/j.plaphy.2019.01.002
Wani, S. H., Tripathi, P., Zaid, A., Challa, G. S., Kumar, A., Kumar, V., et al. (2018b). Transcriptional regulation of osmotic stress tolerance in wheat (Triticum aestivum L.). Plant Mol. Bio. 97, 469–487. doi: 10.1007/s11103-018-0761-6
Wei, W., Bilsborrow, P. E., Hooley, P., Fincham, D. A., Lombi, E., and Forster, B. P. (2003). Salinity induced differences in growth, ion distribution and partioning in barley between the cultivar Maythorpean its derivated mutant Golden promise. Plant Soil 250, 183–191. doi: 10.1023/A:1022832107999
Wei, D., Zhang, W., Wang, C., Meng, Q., Li, G., Chen, T. H., et al. (2017). Genetic engineering of the biosynthesis of glycine betaine leads to alleviate salt-induced potassium efflux and enhances salt tolerance in tomato plants. Plant Sci. 257, 74–83. doi: 10.1016/j.plantsci.2017.01.012
Werner, T., Motyka, V., Laucou, V., Smets, R., Onckelen, H. V., and Schmulling, T. (2003). Cytokinin-deficient transgenic Arabidopsis plants show multiple developmental alterations indicating opposite functions of cytokinins in the regulation of shoot and root meristem activity. Plant Cell 15, 2532–2550. doi: 10.1105/tpc.014928
Wimalasekera, R., and Scherer, G. F. (2009). “Polyamines and cytokinin: is nitric oxide biosynthesis the key to overlapping functions?” in Nitric Oxide in Plant Physiology. eds. S. Hayat, M. Mori, J. Pichtel, and A. Ahmad (Hoboken, NJ: Wiley-Blackwell), 65–76.
Wind, J., Smeekens, S., and Hanson, J. (2010). Sucrose: metabolite and signaling molecule. Phyto Chemistry 71, 1610–1614. doi: 10.1016/j.phytochem.2010.07.007
Winter, G., Todd, C. D., Trovato, M., Forlani, G., and Funck, D. (2015). Physiological implications of arginine metabolism in plants. Front. Plant Sci. 6:534. doi: 10.3389/fpls.2015.00534
Wu, F., Chi, Y., Jiang, Z., Xu, Y., Xie, L., Huang, F., et al. (2020). Hydrogen peroxide sensor HPCA1 is an LRR receptor kinase in Arabidopsis. Nature 578, 577–581. doi: 10.1038/s41586-020-2032-3
Wu, Y., Lei, D., and Zhu, J. K. (1996). SOS1, a genetic locus essential for salt tolerance and potassium acquisition. Plant Cell 8, 617–627. doi: 10.2307/3870339
Wu, J., Liu, W., Jahan, M. S., Shu, S., Sun, J., and Guo, S. (2022). Characterization of polyamine oxidase genes in cucumber and roles of CsPAO3 in response to salt stress. Environ. Exp. Bot. 194:104696. doi: 10.1016/j.envexpbot.2021.104696
Wu, L. Y., Ma, Z. M., Fan, X. L., Zhao, T., Liu, Z. H., Huang, X., et al. (2010). The anti-necrosis role of hypoxic preconditioning after acute anoxia is mediated by aldose reductase and sorbitol pathway in PC12 cells. Cell Stress Chaperon 15, 387–394. doi: 10.1007/s12192-009-0153-6
Xiong, H., Guo, H., Xie, Y., Zhao, L., Gu, J., Zhao, S., et al. (2017). RNAseq analysis reveals pathways and candidate genes associated with salinity tolerance in a spaceflight-induced wheat mutant. Sci Rep-UK 7, 1–13. doi: 10.1038/s41598-017-03024-0
Yancey, P. H. (2005). Organic osmolytes as compatible, metabolic and counteracting cytoprotectants in high osmolarity and other stresses. J. Exp. Biol. 208, 2819–2830. doi: 10.1242/jeb.01730
Yang, C., Zhou, Y., Fan, J., Fu, Y., Shen, L., Yao, Y., et al. (2015). SpBADH of the halophyte Sesuviumportulacastrum strongly confers drought tolerance through ROS scavenging in transgenic Arabidopsis. Plant Physiol. Bioch. 96, 377–387. doi: 10.1016/j.plaphy.2015.08.010
Yoon, J. Y., Hamayun, M., Lee, S. K., and Lee, I. J. (2009). Methyl jasmonate alleviated salinity stress in soybean. J. Crop. Sci. Biotechnol. 12, 63–68. doi: 10.1007/s12892-009-0060-5
You, J., Hu, H., and Xiong, L. (2012). An ornithine δ-aminotransferase gene OsOAT confers drought and oxidative stress tolerance in rice. Plant Sci. 197, 59–69. doi: 10.1016/j.plantsci.2012.09.002
Yu, Z., Duan, X., Luo, L., Dai, S., Ding, Z., and Xia, G. (2020). How plant hormones mediate salt stress responses. Trends Plant Sci. 25, 1117–1130. doi: 10.1016/j.tplants.2020.06.008
Yuan, F., Yang, H., Xue, Y., Kong, D., Ye, R., Li, C., et al. (2014). OSCA1 mediates osmotic-stress-evoked Ca2+ increasesvital for osmosensing in Arabidopsis. Nature 514, 367–371. doi: 10.1038/nature13593
Yuan, W., Zhang, D., Song, T., Xu, F., Lin, S., Xu, W., et al. (2017). Arabidopsis plasma membrane H+-ATPase genes AHA2 and AHA7 have distinct and overlapping roles in the modulation of root tip H+ efflux in response to low-phosphorus stress. J. Exp. Bot. 68, 1731–1741. doi: 10.1093/jxb/erx040
Zarza, X., Atanasov, K. E., Marco, F., Arbona, V., Carrasco, P., Kopka, J., et al. (2017). Polyamine oxidase 5 loss-of-function mutations in Arabidopsis thaliana trigger metabolic and transcriptional reprogramming and promote salt stress tolerance. Plant Cell Environ. 40, 527–542. doi: 10.1111/pce.12714
Zhai, H., Wang, F., Si, Z., Huo, J., Xing, L., An, Y., et al. (2016). A myo-inositol-1-phosphate synthase gene, Ib MIPS1, enhances salt and drought tolerance and stem nematode resistance in transgenic sweet potato. Plant Biotechnol. J. 14, 592–602. doi: 10.1111/pbi.12402
Zhang, L., Gao, M., Hu, J., Zhang, X., Wang, K., and Ashraf, M. (2012). Modulation role of abscisic acid (ABA) on growth, water relations and glycine betaine metabolism in two maize (Zea mays L.) cultivars under drought stress. Int. J. Mol. Sci. 13, 3189–3202. doi: 10.3390/ijms13033189
Zhang, S., Wu, Q. R., Liu, L. L., Zhang, H. M., Gao, J. W., and Pei, Z. M. (2020). Osmotic stress alters circadian cytosolic Ca2+ oscillations and OSCA1 is required in circadian gated stress adaptation. Plant Signal. Behav. 15:1836883. doi: 10.1080/15592324.2020.1836883
Zhen, W.-B., and Ma, Q.-H. (2009). Proline metabolism in response to salt stress in common reed [Phragmites australis (Cav.) Trin. Ex Steud]. Bot. Mar. 52, 341–347. doi: 10.1515/BOT.2009.007
Zhou, R., Cheng, L., and Wayne, R. (2003). Purification and characterization of sorbitol-6-phosphate phosphatase from apple leaves. Plant Sci. 165, 227–232. doi: 10.1016/S0168-9452(03)00166-3
Zhu, J. K. (2016). Abiotic stress signaling and responses in plants. Cells 167, 313–324. doi: 10.1016/j.cell.2016.08.029
Keywords: Brassinosteroids, ethylene, abscisic acid, cytokinins, Jasmonates, salicylic acids, osmolytes, salt stress
Citation: Singh P, Choudhary KK, Chaudhary N, Gupta S, Sahu M, Tejaswini B and Sarkar S (2022) Salt stress resilience in plants mediated through osmolyte accumulation and its crosstalk mechanism with phytohormones. Front. Plant Sci. 13:1006617. doi: 10.3389/fpls.2022.1006617
Edited by:
Anirudh Kumar, Indira Gandhi National Tribal University, IndiaReviewed by:
Abhay Kumar, University of Tuscia, ItalyPiush Srivastava, University of Illinois at Chicago, United States
Copyright © 2022 Singh, Choudhary, Chaudhary, Gupta, Sahu, Tejaswini and Sarkar. This is an open-access article distributed under the terms of the Creative Commons Attribution License (CC BY). The use, distribution or reproduction in other forums is permitted, provided the original author(s) and the copyright owner(s) are credited and that the original publication in this journal is cited, in accordance with accepted academic practice. No use, distribution or reproduction is permitted which does not comply with these terms.
*Correspondence: Krishna Kumar Choudhary, Y2hvdWRoYXJ5LmtyaXNobmEyQGdtYWlsLmNvbQ==; a2tjQGJodS5hYy5pbg==