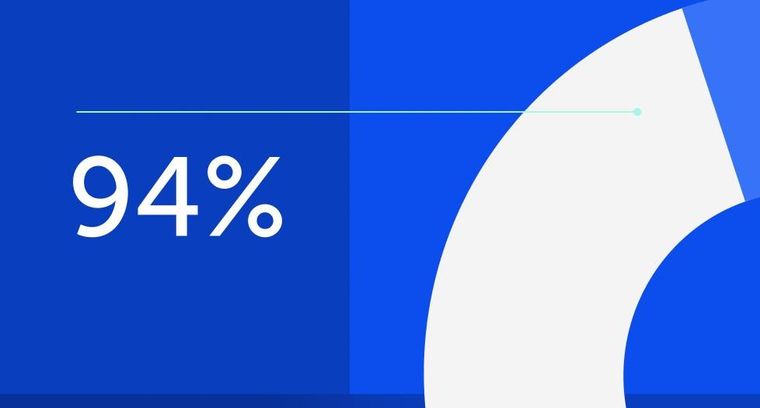
94% of researchers rate our articles as excellent or good
Learn more about the work of our research integrity team to safeguard the quality of each article we publish.
Find out more
REVIEW article
Front. Plant Sci., 20 September 2022
Sec. Plant Nutrition
Volume 13 - 2022 | https://doi.org/10.3389/fpls.2022.1003953
This article is part of the Research TopicImplications of metal uptake and resistance in plants for phytoremediation, biofortification and food safety concerns, Volume IIView all 6 articles
The increasing cadmium (Cd) pollution in paddy fields has severely threatened China’s ecological and food safety. Cultivation of low Cd accumulation varieties to reduce Cd content in rice or cultivation of Cd-tolerant varieties for phytoremediation are considered effective methods to control Cd pollution in paddy fields. However, the underlying molecular mechanism of Cd absorption and transport by rice plants needs to be deciphered to cultivate these varieties. Here, we summarized the molecular mechanisms underlying Cd absorption and transport in rice, as well as the variation of Cd accumulation among rice varieties, the QTLs related to Cd accumulation in rice, and discusses the direction of future research.
Rice is one of the most important food crops grown worldwide. Cadmium (Cd) pollution in rice has attracted great attention from governments around the world. Cd is a notorious heavy metal, which can cause phytotoxicity and human diseases. Thus, effective monitoring of the release of Cd into the environment is imperative. Cd has a strong chemical activity in the soil and is easily absorbed by plants. Cd gets accumulated in the human body through the food chain, thereby severely impacting human health. The rapid increase in Cd pollution in the soil is attributed to the lack of awareness of industrialization and environmental protection in the past three decades. Soils in several parts of China have become more acidic, thus increasing the activity of Cd in soil and its easy uptake by crops (Zhao et al., 2015). Therefore, it is highly essential to understand the absorption and transport mechanism of Cd in rice to reduce its phytotoxicity and human diseases.
Heavy metal pollution has become a common concern worldwide for agriculture and human health (Bertin and Averbeck, 2006; Mohammed et al., 2011; Clemens et al., 2013; Akesson et al., 2014). Pollution caused by excessive discharge of Cd is prominent; it has been reported that Cd is the third most harmful pollutant to the environment (Jamers et al., 2013) because of its ubiquitous and highly toxic nature (Akesson et al., 2014). Cd can exist in soil for a long time, and its pollution occurs through its irreversible accumulation in the soil (Ismael et al., 2019). In China, nearly 2.786 × 105 ha of farmland have been polluted by Cd, including 5 × 104 t of rice were polluted by Cd, causing serious economic losses (Xu et al., 2014; Liu et al., 2015; Song E. et al., 2015; Li et al., 2017). In addition, Cd pollution is also widespread in Europe (Redondo-Gómez et al., 2010).
Cadmium is produced through industrial activities, including metal mining, zinc refining, and extensive use of herbicides and fertilizers. Once present in the atmosphere, soil, and water, Cd can cause serious problems for all organisms through its bioaccumulation in the food chain (Redondo-Gómez et al., 2010). Contamination of food by Cd primarily occurs via contamination of soil and its efficient transfer from soil to plants. Because the biological half-life of Cd is very long (Branca et al., 2020), its content in the body continues to increase with time and eventually settles in the human kidneys. According to a recent Chinese nutrition study, the average Cd intake in China has more than doubled in the 25 years from 1990 to 2015 (Song E. et al., 2017). Therefore, even very low levels of chronic exposure can lead to serious health risks. Cd is the only metal that poses a threat to human and animal health at the plant tissue level—the level that is generally non-phytotoxic (Wang et al., 2011). This implies that plants may not show any toxic symptoms in places with low Cd contamination. However, plants can accumulate Cd in the edible part above allowable levels of human beings. Once these plants enter the food chain, they can result in health problems.
In plants, after Cd enters plant cells, it first acts on mitochondria and chloroplasts and interferes with the electron transport chain (Cannino et al., 2009; Branca et al., 2020). Cd stress can induce the formation of excessive superoxide free radicals and leads to the peroxidation of the cell membrane system (Shah et al., 2001; Haider et al., 2021). Cd is a mutagen that can also inactivate the mismatch repair system in cells (Jin et al., 2003). In addition, Cd can inhibit water transport in plants, resulting in water stress (Haider et al.,2021). It also interferes with the absorption, transport, distribution, and metabolism of essential elements (Rizwan et al., 2016; Haider et al.,2021). With half of the world’s population living on rice, it is an important food crop in the world. When rice grows in the Cd-contaminated soil, excessive Cd accumulates in rice roots, stems, leaves, and grains, which not only hinders the normal growth and development of rice but also seriously affects the quality of rice grain and endangers human health (Uraguchi and Fujiwara, 2012). Therefore, uncovering the uptake and distribution of Cd in plants is essential to help us better understand its accumulation and the tolerance mechanism of rice exposure to Cd.
Cadmium is a non-essential element of plants; it enters plants through the absorption channels of essential elements such as calcium (Ca), iron (Fe), manganese (Mn), and zinc (Zn) (Clemens et al., 2006a). Cd can enter root cells either in the form of free ion Cd2+ or in the form of chelating compounds such as Cd-phytochelatin (PC) and Cd-glutathione (GSH). The entry of Cd into root cells requires the participation of ion transporters. Several ion transporters exist that can transport Cd2+ to root cells (Lux et al., 2011); these ion transporters have element specificity and transport only one or several kinds of metal ions. In addition, there exists a class of ions free of specific cation channel proteins that can transport free Cd2+ into cells; these include depolarization-activated calcium channels (DACC), hyperpolarization-activated calcium channels (HACC), and voltage-sensing channels (VICC). These ion channels have no selectivity for cations and thus can transport all cations (Verbruggen et al., 2009; Lux et al., 2011). In addition, Cd can be transported in the form of chelates into root cells by the yellow stripe 1-like (YSL) transporter (Curie et al., 2009).
The process of Cd absorption and accumulation by plants can be divided into the following steps: absorption of Cd by roots, loading, and transport of Cd into the xylem, transport of Cd from the xylem to the phloem, redistribution of Cd between aboveground stems and leaves, and the accumulation of Cd in grains (Uraguchi et al., 2009). The specific process is shown in Figure 1. Cd in the soil environment passes through layers of obstacles and finally reaches the root xylem, where it is loaded and subsequently transported and unloaded by the xylem and transported longitudinally to different tissues of plant stems, leaves, flowers, and other organs. In addition, after Cd is transported to the aboveground part of the plant, the transport from the xylem to the phloem is completed via the dispersed vascular bundle at the stem node (Tanakak et al., 2007).
Figure 1. Summary of the contribution of genes function in Cd homeostasis. Cd accumulation in plants is first through root absorption, storage in root vacuoles, xylem loading, xylem to phloem transfer and redistribution. Cd is uptake by rice root in soil. OsNramp1, OsNramp5, OsCd1, and OsITR1/2 are suggested to mediate this process. OsHMA3 and OsABCB9 are response for Cd sequestration into vacuoles in root. OsHMA2 and OsCAL1 contributes to the loading process of Cd into xylem in root. OsLCT1/2, OsHMA2, OsZIP3/7 and OsCAL1 are responsible for intervascular Cd transfer at nodes. Co-expression of OsLCT1-OsHMA2-OsZIP3 could reduce the redistribution of Cd in grains.
Higher plants can absorb Cd in soil and water through roots, depending on its availability and concentration in the external medium. In addition, a small part of Cd can be absorbed directly from the atmosphere (Clemens, 2006a). Several factors, such as soil type, pH, climate (temperature, water), the composition of plant rhizosphere microbial community, and genotypic differences of plants, can influence the availability of Cd and plant absorption and transport of Cd (Eriksson, 1989). For example, in Cd-contaminated soils, plant Cd levels were higher at pH 4.0 than at pH 5.0. Soils in several parts of China have become more acidic (especially in the south), such that the activity of Cd in soil has increased and it is easily absorbed by crops (Zhao et al., 2015). The concentration of organic acids in the rhizosphere exerts a great effect on the accumulation of Cd (Lux et al., 2011). The rhizosphere is a small soil area directly affected by root activities; it is affected by root exudates and soil microbial activities. Root exudates play an important role in the bioavailability and toxicity of Cd through controlling rhizosphere pH, redox potential, amount and activity of rhizosphere microorganisms, and chelating ability to Cd. In addition, low molecular weight organic acids secreted by plant roots play an important role in Cd solubility and availability and may mediate the uptake and transport of Cd by plants (Eriksson, 1989). In sandy soils, plants can absorb higher levels of Cd than in clayey soil (Mench and Martin, 1991; Mann and Ritchie, 1995), since most of Cd in sandy soil tend to stay in soluble or exchangeable form (Mann and Ritchie, 1995).
Plants have developed adaptive mechanisms to cope with heavy metal stress, including regulating the absorption of heavy metal ions (Clemens et al., 2002), detoxifying heavy metals through chelation (Clemens, 2006b), and intracellular sequestration (Florijn and Beusichem, 1993) to minimize the exposure to non-essential metal ions (Wang et al., 2011). Plant defense mechanisms against Cd toxicity mainly involve scavenging Cd from active tissues and sequestering it into inactive tissue cells (Song Y. et al., 2017). It is reported that 98% of total Cd remains in Phaseolus vulgaris roots, and only 2% of it is transferred to the shoots, and most of the Cd in the roots is located in the apoplast or vacuole (Ouariti et al., 1997). In Pteris vittata, Cd is largely distributed in less biologically active tissues such as trichomes and scales (Balestri et al., 2014). However, the uptake and accumulation of Cd by rice was significantly different among different rice varieties (Yu et al., 2006; Ye et al., 2012; Song E. et al., 2015; Luo et al., 2018), for instance, Cd content in indica polished grain was higher than that in japonica and hybrid grain (Ye et al., 2012). The response of plants to Cd stress is a complex physiological process. Cd enters the plants through the absorption pathway of essential elements (Clemens, 2006a). Studies in yeast, Arabidopsis, and rice have revealed that the ATP binding cassette (ABC) family, heavy metal transporting P-type ATPase (HMA) family, ZRT and IRT-like protein (ZIP) family, and natural resistance-associated macrophage proteins (NRAMP) family are involved in the response to Cd homeostasis.
ABC transporters are one of the largest families of plants (Tanakak et al., 2007; Uraguchi et al., 2009) and are present in all organisms. These depend on ATP hydrolysis to provide energy and can transfer substances into or out of cells. In plants, ABC transporters are first considered to transport heavy metals and other exogenous substances only on the vacuolar membrane and participate in plant detoxification (Martinoia et al., 1993). Later studies found that they have a wide range of biological functions, such as plant disease resistance, membrane lipid transport, plant inositol formation, and plant hormone transport. Therefore, they play an important role in organ development, plant nutrition uptake, stress resistance, and interaction with the environment (Tanakak et al., 2007). There are 132 members in the ABC family in rice (Garcia et al., 2004; Verrier et al., 2008). Transcriptome studies have demonstrated that several members are involved in the response process of Cd stress and are induced by it; however, its mechanism remains unclear. Among them, OsABCC9 contributes to Cd vacuolar sequestration in rice roots (Yang et al., 2021). OsABCC9 is located in the tonoplasts of the parenchyma cells, and Cd induces the expression of OsABCC9. Knockout of OsABCC9 resulted in increased sensitivity of rice plants to Cd, these plants accumulated more Cd in roots and shoots, with increased concentration of Cd in the xylem sap and grain, indicating that more Cd is distributed from roots to shoots and grains in OsABCC9 knocked out plants. OsABCG43/OsPDR5 and OsABCG36/OsPDR9 possess Cd transport capacity; which are mainly expressed in rice roots (Moons, 2003; Oda et al., 2011). Heterologous expression of OsABCG43 in yeast can improve the resistance of yeast to Cd (Oda et al., 2011). In addition, short-time Cd treatment can significantly increase the expression of OsABCG36 in the roots (Fu et al., 2011). OsABCG36 is located in the plasma membrane (PM). Knocking out OsABCG36 leads to the accumulation of Cd in the roots and increases the sensitivity of roots to Cd. The expression of OsABCG36 in yeast shows that it has the efflux activity of Cd (Fu et al., 2011). This indicates that various ABC family genes perform different functions in uptake, transport and distribution of Cd. The genes involved in Cd uptake and transport are summarized in Table 1.
Heavy metal transporting P-type ATPase is a subfamily of P-type ATPase. The subfamily has relatively conserved domains, including eight transmembrane domains, one CPx domain involved in the transport, and one C-terminal metal ion-binding domain (Colangelo and Guerinot, 2006). Rice genome encodes nine HMA transporters. OsHMA1-OsHMA3 are transporters of Zn/Cd/Pb/Co divalent cation, whereas OsHMA4-OsHMA9 belong to Cu/Ag monovalent cation transporters (Williams and Mills, 2005). OsHMA2 is located in the plasma membrane and loads Cd and Zn into the xylem and participates in the transport of Cd and Zn from the roots to shoots. The content of Cd in the seeds of OsHMA2-overexpressed lines and OsSUT1 promoter driving OsHMA2 transgenic lines is half that of the wild-type; however, the content of other metals is the same as that of the wild-type (Satoh-Nagasawa et al., 2012; Takahashi et al., 2012; Yamaji et al., 2013). Knockout of OsHMA2 reduced the levels of Cd and Zn in the reproductive tissues of rice (Satoh-Nagasawa et al., 2012; Takahashi et al., 2012; Yamaji et al., 2013). AtHMA3 has been reported to play a function in the transport of Cd, Zn, Co, and Pb (Morel et al., 2009), whereas NcHMA3 (Noccaea caerulescens) can transport Cd and Zn (Ueno et al., 2011). In rice, OsHMA3 is mainly expressed in the roots and does not respond to Cd exposure (Ueno et al., 2010). OsHMA3 is located in the tonoplast, and its silencing leads to increased Cd translocation from roots to shoots, whereas its overexpression produces the opposite effect (Ueno et al., 2010; Sasaki et al., 2014). These results suggest that OsHMA3 functions in the transport of Cd into vacuoles and Cd sequestration in the roots, thereby reducing the transport of Cd to shoots (Ueno et al., 2010; Miyadate et al., 2011; Sasaki et al., 2014). Overexpression of OsHMA3 can also significantly reduce the content of Cd in rice grains. The C-terminal region of OsHMA3, especially the first 105 amino acids, plays an important role in OsHMA3 activity during Cd stress (Kumagai et al., 2014). OsHMA9 has Cu transport activity and can transport Cu outside the cells, which plays an important role in the stability of intracellular concentration of Cu. The expression of OsHMA9 was induced by Zn and Cd. OsHMA9 knockout plants were reported to be sensitive to Cd, Zn, Cu, and Pb, and showed a high accumulation of these elements (Lee et al., 2007).
Natural resistance-associated macrophage proteins widely exist in microorganisms, plants, and animals, and play an important role in maintaining the dynamic balance of metal ions in organisms. Most of NRAMPs can transport a variety of metal ions, such as Cu, Co, Ni, Cd, Fe, Mn, and Zn (Colangelo and Guerinot, 2006; Nevo and Nelson, 2006; Xia et al., 2010). The rice genome contains seven NRAMP genes. OsNRAMP1 is mainly expressed in the roots and its expression is highly induced by Fe deficiency. OsNRAMP1 is located in the plasma membrane and functions in the transport of Cd and Fe. The difference in Cd accumulation observed in rice varieties is caused by the varied expression of OsNRAMP1 in the roots (Takahashi et al., 2011). OsNRAMP5 is an important transporter responsible for Mn uptake by roots, which is necessary for high Mn accumulation in the rice bud. In addition, OsNRAMP5 plays a key role in mediating the entry of Cd into root cells from the outside medium (Sasaki et al., 2012). OsNRAMP5 is primarily expressed in the exodermis and endodermis of basal root zones, and OsNRAMP5 is located in the plasma membrane in both onion cells and rice protoplasts (Sasaki et al., 2012). The absorption of Cd was greatly reduced in the roots of osnramp5 mutant, thus reducing the accumulation of Cd in the stems and grains (Ishikawa et al., 2012). Moreover, the osnramp5 mutant was found to be more sensitive to Mn and Fe deficiencies (Yang et al., 2014). OsNRAMP2 has two haplotypes owing to four amino acid differences (OsNRAMP2-L and OsNRAMP2-H) that result in low and high Cd accumulation in rice accessions (Zhao et al., 2018). Using the yeast heterologous assay, the study showed that OsNRAMP2-L is the functional form of OsNRAMP2; OsNRAMP2-L could increase the sensitivity and accumulation of Cd in yeast, whereas OsNRAMP2-H could not. However, these four amino acid differences do not affect the localization of distinct haplotype of OsNRAMP2, both OsNRAMP2-L and OsNRAMP2-H were located in the tonoplast, which is different from the localization of OsNRAMP1 and OsNRAMP5 to the plasma membrane (Takahashi et al., 2011; Sasaki et al., 2012).
The ZIP family is named for its similarity sequence to ZRT1 (Zn-regulated transporter 1) in yeast and IRT1 (Iron-regulated transporter 1) in Arabidopsis. It plays an important role in the uptake of metals and is found in several organisms (Connolly et al., 2002). In plants, the ZIP family has been identified both in dicots and monocots (Zheng et al., 2018), such as rice (Chen et al., 2008), Arabidopsis (Milner et al., 2013), maize (Li et al., 2013), Medicago (Stephens et al., 2011), and barley (Tiong et al., 2015). The rice genome consists of 18 ZIP genes (Milner et al., 2013). In rice, OsITR1 is primarily expressed in rice roots and its expression is induced by Fe deficiency (Bughio et al., 2002). Under Fe deficiency, rice plants tend to accumulate more Cd in roots, indicating that Cd absorption is activated by Fe deficiency. In addition, heterologous expression of OsIRT1 and OsIRT2 in yeast increases the sensitivity of Cd and its accumulation in yeast cells (Nakanishi et al., 2006; Lee and An, 2009). OsZIP1 is considered to be a Zn uptake transporter and induced by Zn deficiency (Ramesh et al., 2003; Bashir et al., 2012; Ramegowda et al., 2013). Further study showed that OsZIP1 is a metal detoxification transporter that prevents excessive accumulation of Zn, Cu, and Cd in rice (Liu et al., 2019). The OsZIP1-overexpression lines grew better and accumulated fewer metals. In contrast, oszip1 mutants and RNA interference (RNAi) lines accumulated more metals in the roots (Liu et al., 2019). OsZIP7 loads Zn and Cd into the xylem in rice roots and participates in the intervascular transfer in the nodes by cooperating with OsZIP3 and OsHMA2 (Yamaji et al., 2013; Sasaki et al., 2015; Tian et al., 2019). In addition, co-expression of OsLCT1-OsHMA2-OsZIP3 can effectively reduce the transport and accumulation of Cd in grains and oxidative stress caused by Cd and Zn stress (Tian et al., 2019). OsZIP5 and OsZIP9 are highly expressed in roots and weakly expressed in shoots. OsZIP5 and OsZIP9 function redundantly in Zn/Cd uptake and translocation (Tan et al., 2020).
Besides the above ion transporter families, other ion transporters are involved in the absorption and translocation of Cd in rice. For example, a low-affinity cation transporter (LCT) was first found in wheat; TaLCT1 can transport Ca and Cd and its heterologous expression in yeast leads to increased Cd content in yeast cells, increasing the sensitivity of yeast cells to Cd stress (Clemens et al., 1998). OsLCT1 is located in the plasma membrane of internodal phloem parenchyma cells in rice and promotes the loading of Cd into the phloem sieve tube. Knockout of OsLCT1 reduced the concentration of Cd in rice grains (Uraguchi et al., 2011). An important role of microRNA (miRNA) has been reported in Cd tolerance in rice (Ding et al., 2018). Reduced expression of miR166 was found in rice roots under Cd exposure. Overexpression of miR166 increased Cd tolerance and reduced its transport from roots to shoots, thereby reducing the accumulation of Cd in grains. OsHB4, a target gene of miR166, was induced by Cd treatment and downregulated by the overexpression of miR166 in transgenic rice plants. Plants overexpressing OsHB4 showed increased Cd sensitivity and Cd accumulation in leaves. Conversely, silencing of OsHB4 enhanced Cd tolerance in transgenic plants (Ding et al., 2018). A putative serine hydroxymethyl transferase OsCADT1 is known to be involved in Cd tolerance; it is a negative regulator of sulfate/selenate absorption and assimilation. In oscadt1 mutants, the expression of sulfate/selenate transport gene OsSULTR1 increased, resulting in increased absorption of sulfur and selenium, followed by synthesis of more sulfhydryl compounds to increase the tolerance to Cd (Chen et al., 2020). The lcd mutants were screened with Cd tolerance; LCD is localized in the cytoplasm and nucleus and is majorly expressed in the vascular tissues of roots and leaves. The content of Cd in grains of the lcd mutant was significantly lower than that in the control when the plants were grown in low Cd-contaminated soil (Shimo et al., 2011). Heat shock transcription factor A4a (HsfA4a) of wheat was screened with Cd tolerance in yeast; the homolog gene of TaHsfA4a in rice is OsHsfA4a, which could also rescue Cd tolerance in yeast. When TaHsfA4a was expressed in rice, it increased the Cd tolerance of rice, whereas the tolerance to Cd was decreased when the expression of OsHsfA4a was downregulated (Shim et al., 2009). Overexpression of the full-length sequence or truncated sequence of OsO3L2 or OsO3L3 can not only reduce Cd absorption in roots and leaves but also significantly reduce Cd accumulation in grains without affecting the content of other metals such as Mg, Fe, Cu, and Zn (Wang et al., 2016, 2019). Furthermore, the auxin influx transporter OsAUX1 is involved in primary root and root hair elongation in response to Cd stress (Yu et al., 2015). The expression of OsPCS1 was induced by Cd in roots and seeds (Das et al., 2017). Both the absence of OsPCS1 and OsPCS2 transcripts in developing seeds significantly reduced the content of Cd in the grains by 51% (Das et al., 2017). Cd/cation exchange gene OsCCX2 is primarily expressed in the xylem region of rice nodes, and OsCCX2 is localized on the plasma membrane. Knockout of OsCCX2 significantly reduced the Cd content in grains; detailed studies showed that knockout of OsCCX2 reduced the Cd transport from roots to shoots (Hao et al., 2018).
Genotypes play an important role in the absorption, transport, and detoxification of Cd in plants. Differences have been known to exist in Cd accumulation in rice germplasm, which lays the foundation for studying the causes of different Cd tolerance (Arao and Ae, 2003; Ueno et al., 2009a; Pinson et al., 2015). In a recent study, Luo et al. used 212 rice accessions to determine Cd content and found that an indicia cultivar Tainan1 (TN1) over accumulated Cd in grains and leaves than cultivar Chunjiang06 (CJ06) (Luo et al., 2018). Further study identified a quantitative trait locus (QTL) mediating Cd accumulation in leaves and named it CAL1 (Cd accumulation in leaf1). CAL1 is primarily expressed in the roots and leaf sheaths. Cd treatment significantly induced the expression of CAL1 in NIL (near isogenic line) -TN1 roots. Cross-section analysis showed that the expression of CAL1 was superior to that in the xylem parenchyma cells and the vascular system of roots. The accumulation of Cd in leaf blades of seedlings and straws of mature plants in NIL-TN1 was higher than that in NIL-CJ06. This result is consistent with the increased Cd concentration observed in the xylem sap of NIL-TN1. However, no significant difference in Cd level was observed in grains between NIL-TN1 and NIL-CJ06. In vitro metal-binding assays showed that CAL1 functions in chelating Cd and facilitating Cd efflux from protoplasts. CAL1 binds Cd in the cytosol, secretes Cd from the xylem parenchyma cells into the xylem vessels, and prevents Cd loading into the phloem, explaining why CAL1 specifically affects the accumulation of Cd in leaves and rice straw but not in grains (Luo et al., 2018). The closest homologous gene to CAL1 in rice is OsCAL2, which is mainly expressed in roots and located in the cell wall (Luo et al., 2020). Moreover, OsCAL2 also showed Cd binding activity, heterologous overexpression of OsCAL2 increased the accumulation of Cd in Arabidopsis shoots, whereas it decreased the concentration of Cd in roots, and overexpression of OsCAL2 in rice increased the accumulation of Cd in straws and seeds (Luo et al., 2020). The other gene responsible for Cd accumulation in rice grains between indica and japonica is OsCd1 (Yan et al., 2019), which belongs to the major facilitator superfamily (MFS). OsCd1 is mainly expressed in the roots and its expression is not induced by Cd; it is located in the plasma membrane and involved in Cd uptake. When OsCd1 is expressed in yeast, it increases the sensitivity of yeast cells to Cd treatment compared with control strains. The difference in Cd accumulation in rice grains between indica and japonica is ascribed to a natural variation in OsCd1 and a missense mutation in Val449Asp. In a near-isogenic line (NIL) assay, the introgression line of OsCd1V449 exhibited significantly reduced Cd accumulation in grains compared to 9,311 backgrounds (Yan et al., 2019).
The variations in Cd concentration in the xylem sap are closely related to Cd accumulation in rice shoots and grain, indicating the transport of Cd from roots to shoots as a key process to mediate Cd homeostasis (Uraguchi et al., 2009). Non-functional alleles of OsHMA3 have been reported in certain indica cultivars with high Cd accumulation (Ueno et al., 2010, 2011; Miyadate et al., 2011). Another study revealed that one of these alleles represent a loss of OsHMA3 function, resulting in decreased vacuolar sequestration of Cd in roots and increased Cd translocation to shoots (Ueno et al., 2010; Miyadate et al., 2011). Its heterologous expression in yeast showed that the 80th amino acid residue of OsHMA3 protein in the high Cd cultivar Anjana Dhan was mutated from Arg to His, resulting in a loss of function (Ueno et al., 2010). In addition, a new loss-of-function allele of OsHMA3 has been found in certain temperate japonica rice varieties with the accumulation of high Cd in shoots and grains (Yan et al., 2016). This allele does not exist in any indica rice varieties among 533 rice varieties (Chen et al., 2014) and the sequencing group of 950 world rice varieties (Huang et al., 2012). Compared to the functional allele of OsHMA3 in Nipponbare, the new allele has a single SNP in the coding region, resulting in a mutation of the 380th amino acid from Ser to Arg. Heterologous expression in yeast showed that the new allele of OsHMA3 haplotype (type II) is nonactive in Cd transport, resulting in the same Cd sensitivity and accumulation phenotypes in yeast as the control and the known nonfunctional allele (type VIII) of Anjana Dhan in yeast. Ser to Arg mutation is expected to alter the charge properties of the OsHMA3 protein without affecting the subcellular localization of OsHMA3 (Yan et al., 2016), which is different, as both the functional (type I) and unfunctional (type VIII) OsHMA3 are mislocated in the endoplasmic reticulum (ER) when expressed in yeast (Ueno et al., 2010). It was also reported that the promoter activity of OsHMA3 differs between 9,311 and PA64s, resulting in differential OsHMA3 expression and Cd accumulation in shoots and grain (Liu et al., 2020). This implies that we can reduce the accumulation of Cd in grains through combine different haplotypes of OsHMA3 and its promoter activity.
Quantitative trait locus (QTL) mapping, a powerful tool to study multivariate genes for complex agronomic traits, has been successfully used to identify loci that control Cd accumulation in rice (Hu et al., 2018). Several QTLs for Cd tolerance have been identified through different genetic populations and phenotypic analysis methods. QTL analysis of Cd accumulation using an F2 population from Anjana Dhan and Nipponbare identified a QTL on chromosome 7 with a significant effect on Cd accumulation, explaining 85.6% of the phenotypic variation in Cd concentration in the shoots of the F2 population (Ueno et al., 2009b). Hu et al. identified certain QTLs related to Cd content in brown rice (CCBR) and Cd content in milled rice (CCMR) using a double haploid (DH) population of Zhongjiadao 17 × D50, of which qCCBR2-1/qCCBR2-2 and qCCBR9-1/qCCBR9-2 were found to be responsible for CCBR, whereas qCCMR5-1/qCCMR5-2 were found to be responsible for CCMR in several fields and pot experiments (Hu et al., 2018). Zhao et al. detected 14 Cd accumulation QTLs in rice grains from 312 rice accessions by a genome-wide association study (GWAS) (Zhao et al., 2018), of which 3 QTLs were first identified in this study, and 4 QTLs were the previously cloned genes (OsNRAMP1, OsNRAMP5, OsHMA3, and LCD) that mediate Cd accumulation in rice (Miyadate et al., 2011; Shimo et al., 2011; Takahashi et al., 2011; Sasaki et al., 2012). The grain Cd accumulation QTL of OsCd1 was identified through the GWAS analysis using 127 rice cultivars (Yan et al., 2019). Natural variations in OsCd1 in the Val449Asp mutation could distinguish the Cd accumulation in rice grains between indica and japonica. The Cd accumulation in polished rice was analyzed using 338 distinct rice accessions under Cd-contaminated soil, and 35 QTLs were identified through GWAS in a 2-year assay, of which 9 QTLs were co-localized with a previously reported gene; OsABCB24 was predicted to be a novel QTL of qCd1-3 (Pan et al., 2020). A total of 119 Cd-mediated growth response (CGR) -QTLs have been discovered recently, of which 55 have been validated by previously described QTLs, and 64 are novel CGR loci. Certain reported genes have been found to function in CGRs (Yu et al., 2021).
With industrialization and urbanization, Cd pollution in soil has become a serious health concern. Solving the problem of soil Cd pollution has become a long-term and arduous task. Cultivating new rice varieties with low Cd, high quality, high yield, and high resistance is one of the effective measures to solve soil Cd pollution and ensure national food security. Under Cd stress, plants regulate the absorption, transport, distribution, and sequestration of Cd by mediating the expression of related genes, thereby balancing the toxicity caused by Cd. However, the limited knowledge of functional genes cannot completely explain the biological processes of Cd homeostasis between tissues and organs and the flow between subcellular organelles in rice. First, although a series of progress has been made regarding the mechanism of Cd absorption, transport, and detoxification in rice, certain genes can be used to remediate Cd-contaminated soil and produce grains with less Cd accumulation. However, these genes are rarely used in popularized varieties. Second, studies on the mechanism of genes involved in Cd stress response are still considerably less and are limited to the uptake and transport of Cd in rice. As well as the upstream regulatory networks of functional genes are still scarce. Third, we need to study the methods to balance different functional genes under different Cd stress to harness the maximum benefit. Plants can induce the expression of several genes under Cd stress; currently, the functions of only a few Cd-induced transporters are known. For example, the expression of AtHMA3 was induced under Cd stress to accelerate its transport Cd to vacuoles (Mendoza-Cózatl et al., 2011), and nitrate transporter AtNRT1.8 was also induced by Cd to balance the adverse effects of Cd on nitrogen absorption and assimilation and improve the resistance to Cd (Boussama et al., 1999). Fourth, rhizosphere microbes play an important role in plants responding to Cd stress; however, it is still unclear how to use rhizosphere microbes to remediate Cd-contaminated soil or produce less Cd accumulation in rice grains. Fifth, certain already identified functional Cd absorption and transport proteins, such as OsNRAMP5, have no substrate specificity. Knockout of OsNRAMP5 is also known to affect the uptake and transport of Mn, thereby reducing the production of rice. Next, we need to study how to uncover the structural basis of functional transporters to balance the uptake of beneficial nutrients and harmful Cd. Sixth, variants of rice germplasm can play an important role in Cd stress, in addition to QTL mapping, we need to combine GWAS and high-throughput transcriptomics to identify more Cd stress response genes and elucidate the underlying molecular mechanism. Seventh, some plants that are super Cd accumulation and tolerant should also be studied, and the mechanism can be applied to the study of cadmium tolerance in rice, this provides a useful supplement to better understand the mechanisms of Cd uptake, transport, and detoxification in plants. We believe these findings will provide a basis for exploring the differences in the characteristics of Cd homeostasis in rice and reducing the environmental and food safety risks and phytoremediation.
HA and MH wrote the first draft of the manuscript and organized the tables and figures. DW and CL reviewed the manuscript. All authors have read and agreed to the published version of the manuscript.
This research was financially supported by the Chinese Postdoctoral Science Foundation (2021M693467), the Natural Science Fund of Education Department of Anhui province (KJ2021A0898), the Talent introduction project in Anhui Science and Technology University (NXYJ202101), and the Natural Science Fund of Anhui Science and Technology University (No. 2021zryb16).
The authors declare that the research was conducted in the absence of any commercial or financial relationships that could be construed as a potential conflict of interest.
All claims expressed in this article are solely those of the authors and do not necessarily represent those of their affiliated organizations, or those of the publisher, the editors and the reviewers. Any product that may be evaluated in this article, or claim that may be made by its manufacturer, is not guaranteed or endorsed by the publisher.
Akesson, A., Barregard, L., Bergdahl, I. A., Nordberg, G. F., Nordberg, M., and Skerfving, S. (2014). Non-renal effects and the risk assessment of environmental cadmium exposure. Environ. Health Perspect. 122, 431–438. doi: 10.1289/ehp.1307110
Arao, T., and Ae, N. (2003). Genotypic variations in cadmium levels of rice grain. Soil Sci. Plant Nutr. 49, 473–479. doi: 10.1080/00380768.2003.10410035
Balestri, M., Ceccarini, A., Forino, L. M. C., Zelko, I., Martinka, M., Lux, A., et al. (2014). Cadmium uptake, localization and stress-induced morphogenic response in the fern Pteris vittata. Planta 239, 1055–1064. doi: 10.1007/s00425-014-2036-z
Bashir, K., Ishimaru, Y., and Nishizawa, N. K. (2012). Molecular mechanisms of zinc uptake and translocation in rice. Plant Soil 361, 189–201. doi: 10.1007/s11104-012-1240-5
Bertin, G., and Averbeck, D. (2006). Cadmium: cellular effects, modifications of biomolecules, modulation of DNA repair and genotoxic consequences. Biochimie 88, 1549–1559. doi: 10.1016/j.biochi.2006.10.001
Boussama, N., Ouariti, O., and Ghorbal, M. H. (1999). Changes in growth and nitrogen assimilation in barley seedlings under cadmium stress. J. Plant Nutr. 22, 731–752. doi: 10.1080/01904169909365668
Branca, J. J. V., Pacini, A., Gulisano, M., Taddei, N., Fiorillo, C., and Becatti, M. (2020). Cadmium-induced cytotoxicity: effects on mitochondrial electron transport chain. Front. Cell Dev. Biol. 8:604377. doi: 10.3389/fcell.2020.604377
Bughio, N., Yamaguchi, H., Nishizawa, N. K., Nakanishi, H., and Mori, S. (2002). Cloning an iron-regulated metal transporter from rice. J. Exp. Bot. 53, 1677–1682. doi: 10.1093/jxb/erf004
Cannino, G., Ferruggia, E., Luparello, C., and Rinaldi, A. M. (2009). Cadmium and mitochondria. Mitochondrion 9, 377–384. doi: 10.1016/j.mito.2009.08.009
Chang, J. D., Huang, S., Yamaji, N., Zhang, W., Ma, J. F., and Zhao, F. J. (2020). OsNRAMP1 transporter contributes to cadmium and manganese uptake in rice. Plant Cell Environ. 43, 2476–2491. doi: 10.1111/pce.13843.
Chen, J., Huang, X. Y., Salt, D. E., and Zhao, F. J. (2020). Mutation in OsCADT1 enhances cadmium tolerance and enriches selenium in rice grain. New Phytol. 226, 838–850. doi: 10.1111/nph.16404
Chen, W. R., Feng, Y., and Chao, Y. E. (2008). Genomic analysis and expression pattern of OsZIP1, OsZIP3, and OsZIP4 in two rice (Oryza sativa L.) genotypes with different zinc efficiency. Russ. J. Plant Physl. 55, 400–409. doi: 10.1134/S1021443708030175
Chen, W., Gao, Y., Xie, W., Gong, L., Lu, K., Wang, W., et al. (2014). Genome-wide association analyses provide genetic and biochemical insights into natural variation in rice metabolism. Nat. Genet. 46, 714–721. doi: 10.1038/ng.3007
Clemens, S. (2006a). Toxic metal accumulation, responses to exposure and mechanisms of tolerance in plants. Biochimie 88, 1707–1719. doi: 10.1016/j.biochi.2006.07.003
Clemens, S. (2006b). Evolution and function of phytochelatin synthases. J. Plant Physiol. 163, 319–332. doi: 10.1016/j.jplph.2005.11.010
Clemens, S., Aarts, M. G., Thomine, S., and Verbruggen, N. (2013). Plant science: the key to preventing slow cadmium poisoning. Trends Plant Sci. 18, 92–99. doi: 10.1016/10.1016/j.tplants.2012.08.003
Clemens, S., Antosiewicz, D. M., Ward, J. M., Schachtman, D. P., and Schroeder, J. I. (1998). The plant cDNA LCT1 mediates the uptake of calcium and cadmium in yeast. Proc. Natl. Acad. Sci. U.S.A. 95, 12043–12048. doi: 10.1073/pnas.95.20.12043
Clemens, S., Palmgren, M. G., and Krämer, U. A. (2002). Long way ahead: understanding and engineering plant metal accumulation. Trends Plant Sci. 7, 309–315. doi: 10.1016/s1360-1385(02)02295-1
Colangelo, E. P., and Guerinot, M. L. (2006). Put the metal to the petal: metal uptake and transport throughout plants. Curr. Opin. Plant Biol. 9, 322–330. doi: 10.1016/j.pbi.2006.03.015
Connolly, E. L., Fett, J. P., and Guerinot, M. L. (2002). Expression of the IRT1 metal transporter is controlled by metals at the levels of transcript and protein accumulation. Plant Cell 14, 1347–1357. doi: 10.1105/tpc.001263
Cui, Y., Wang, M., Yin, X., Xu, G., Song, S., Li, M., et al. (2019). OsMSR3, a small heat shock protein, confers enhanced tolerance to copper stress in Arabidopsis thaliana. Int. J. Mol. Sci. 20:6096. doi: 10.3390/ijms20236096
Curie, C., Cassin, G., Couch, D., Divol, F., Higuchi, K., Jean, M. L., et al. (2009). Metal movement within the plant: contribution of nicotianamine and yellow stripe 1-like transporters. Ann. Bot. 103, 1–11. doi: 10.1093/aob/mcn207
Das, N., Bhattacharya, S., Bhattacharyya, S., and Maiti, M. K. (2017). Identification of alternatively spliced transcripts of rice phytochelatin synthase 2 gene OsPCS2 involved in mitigation of cadmium and arsenic stresses. Plant Mol. Biol. 94, 167–183. doi: 10.1007/s11103-017-0600-1
Ding, Y., Gong, S., Wang, Y., Wang, F., Bao, H., Sun, J., et al. (2018). MicroRNA166 modulates cadmium tolerance and accumulation in rice. Plant Physiol. 177, 1691–1703. doi: 10.1104/pp.18.00485
Eriksson, J. E. (1989). The influence of pH, soil type and time on adsorbtion and uptake by plants of Cd added to the soil. Water Air Soil Pollut. 48, 317–335. doi: 10.1007/BF00283334
Florijn, P. J., and Beusichem, M. L. (1993). Uptake and distribution of cadmium in maize inbred lines. Plant Soil 150, 25–32. doi: 10.1016/j.scitotenv.2020.138972
Fu, S., Lu, Y., Zhang, X., Yang, G., Chao, D., Wang, Z., et al. (2019). The ABC transporter ABCG36 is required for cadmium tolerance in rice. J. Exp. Bot. 70, 5909–5918. doi: 10.1093/jxb/erz335
Garcia, O., Bouige, P., Forestier, C., and Dassa, E. (2004). Inventory and comparative analysis of rice and Arabidopsis ATP-binding cassette (ABC) systems. J. Mol. Biol. 343, 249–265. doi: 10.1016/j.jmb.2004.07.093
Haider, F. U., Liqun, C., Coulter, J. A., Cheema, S. A., Wu, J., Zhang, R., et al. (2021). Cadmium toxicity in plants: impacts and remediation strategies. Ecotoxicol. Environ. Saf. 211:111887. doi: 10.1016/j.ecoenv.2020.111887
Ham, D. J., Moon, J. C., Hwang, S. G., and Jang, C. S. (2013). Molecular characterization of two small heat shock protein genes in rice: their expression patterns, localizations, networks, and heterogeneous overexpressions. Mol. Biol. Rep. 40, 6709–6720. doi: 10.1007/s11033-013-2786-x
Hao, X., Zeng, M., Wang, J., Zeng, Z., Dai, J., Xie, Z., et al. (2018). A node-expressed transporter OsCCX2 is involved in grain cadmium accumulation of rice. Front. Plant Sci. 9:476. doi: 10.3389/fpls.2018.00476
Hu, D. W., Sheng, Z. H., Li, Q. L., Chen, W., Wei, X. J., Xie, L. H., et al. (2018). Identification of QTLs associated with cadmium concentration in rice grains. J. Integr. Agr. 17, 1563–1573. doi: 10.1016/S2095-3119(17)61847-1
Huang, S., Sasaki, A., Yamaji, N., Okada, H., Mitani-Ueno, N., and Ma, J. F. (2020). The ZIP transporter family member OsZIP9 contributes to root zinc uptake in Rice under zinc-limited conditions. Plant Physiol. 183, 1224–1234. doi: 10.1104/pp.20.00125
Huang, X., Zhao, Y., Wei, X., Li, C., Wang, A., Zhao, Q., et al. (2012). Genome-wide association study of flowering time and grain yield traits in a worldwide collection of rice germplasm. Nat. Genet. 44, 32–39. doi: 10.1038/ng.1018
Ishikawa, S., Ishimaru, Y., Igura, M., Kuramata, M., Abe, T., Senoura, T., et al. (2012). Ion-beam irradiation, gene identification, and marker-assisted breeding in the development of low-cadmium rice. Proc. Natl. Acad. Sci. U.S.A. 109, 19166–19171. doi: 10.1073/pnas.1211132109
Ishimaru, Y., Bashir, K., Nakanishi, H., and Nishizawa, N. K. (2012). OsNRAMP5, a major player for constitutive iron and manganese uptake in rice. Plant Signal. Behav. 7, 763–766. doi: 10.4161/psb.20510
Ishimaru, Y., Kim, S., Tsukamoto, T., Oki, H., Kobayashi, T., Watanabe, S., et al. (2007). Mutational reconstructed ferric chelate reductase confers enhanced tolerance in rice to iron deficiency in calcareous soil. Proc. Natl. Acad. Sci. U.S.A. 104, 7373–7378. doi: 10.1073/pnas.061055510
Ismael, M. A., Elyamine, A. M., Moussa, M. G., Cai, M., Zhao, X., and Hu, C. (2019). Cadmium in plants: uptake, toxicity, and its interactions with selenium fertilizers. Metallomics 11, 255–277. doi: 10.1039/c8mt00247a
Jamers, A., Blust, R., De Coen, W., Griffin, J. L., and Jones, O. A. (2013). An omics based assessment of cadmium toxicity in the green alga Chlamydomonas reinhardtii. Aquat. Toxicol. 126, 355–364. doi: 10.1016/j.aquatox.2012.09.007
Jin, Y. H., Clark, A. B., Slebos, R. J., Al-Refai, H., Taylor, J. A., Kunkel, T. A., et al. (2003). Cadmium is a mutagen that acts by inhibiting mismatch repair. Nat. Genet. 34, 326–329. doi: 10.1038/ng1172
Kumagai, S., Suzuki, T., Tezuka, K., Satoh-Nagasawa, N., Takahashi, H., Sakurai, K., et al. (2014). Sakurai functional analysis of the C-terminal region of the vacuolar cadmium-transporting rice OsHMA3. FEBS Lett. 588, 789–794. doi: 10.1016/j.febslet.2014.01.037
Lan, H. X., Wang, Z. F., Wang, Q. H., Wang, M. M., Bao, Y. M., Huang, J., et al. (2013). Characterization of a vacuolar zinc transporter OZT1 in rice (Oryza sativa L.). Mol. Biol. Rep. 40, 1201–1210. doi: 10.1007/s11033-012-2162-2
Lee, S., and An, G. (2009). Over-expression of OsIRT1 leads to increased iron and zinc accumulations in rice. Plant Cell Environ. 32, 408–416. doi: 10.1111/j.1365-3040.2009.01935.x
Lee, S., Kim, Y. Y., Lee, Y., and An, G. (2007). Rice P1B-type heavy-metal ATPase, OsHMA9, is a metal efflux protein. Plant Physiol. 145, 831–842. doi: 10.1104/pp.107.102236
Li, H., Luo, N., Li, Y. W., Cai, Q. Y., Li, H. Y., Mo, C. H., et al. (2017). Cadmium in rice: transport mechanisms, influencing factors, and minimizing measures. Environ. Pollut. 224, 622–630. doi: 10.1016/j.envpol.2017.01.087
Li, S., Zhou, X., Huang, Y., Zhu, L., Zhang, S., Zhao, Y., et al. (2013). Identification and characterization of the zinc-regulated transporters, iron-regulated transporter-like protein (ZIP) gene family inmaize. BMC Plant Biol. 13:114. doi: 10.1186/1471-2229-13-114
Liu, X. S., Feng, S. J., Zhang, B. Q., Wang, M. Q., Cao, H. W., Rono, J. K., et al. (2019). OsZIP1 functions as a metal efflux transporter limiting excess zinc, copper and cadmium accumulation in rice. BMC Plant Biol. 19, 283–216. doi: 10.1186/s12870-019-1899-3
Liu, C. L., Gao, Z. Y., Shang, L. G., Yang, C. H., Ruan, B. P., Zeng, D. L., et al. (2020). Natural variation in the promoter of OsHMA3 contributes to differential grain cadmium accumulation between Indica and japonica rice. J. Integr. Plant Biol. 62, 314–329. doi: 10.1111/jipb.12794
Liu, F., Liu, X. N., Ding, C., and Wu, L. (2015). The dynamic simulation of rice growth parameters under cadmium stress with the assimilation of multi-period spectral indices and crop model. Field Crop Res. 183, 225–234. doi: 10.1016/j.fcr.2015.08.004
Lu, C., Zhang, L., Tang, Z., Huang, X. Y., Ma, J. F., and Zhao, F. J. (2019). Producing cadmium-free Indica rice by overexpressing OsHMA3. Environ. Int. 126, 619–626. doi: 10.1016/j.envint.2019.03.004
Luo, J. S., Huang, J., Zeng, D. L., Peng, J. S., Zhang, G. B., Ma, H. L., et al. (2018). A defensin-like protein drives cadmium efflux and allocation in rice. Nat. Commun. 9:645. doi: 10.1038/s41467-018-03088-0
Luo, J. S., Xiao, Y., Yao, J., Wu, Z., Yang, Y., Ismail, A. M., et al. (2020). Overexpression of a Defensin-like gene CAL2 enhances cadmium accumulation in plants. Front. Plant Sci. 11:217. doi: 10.3389/fpls.2020.00217
Lux, A., Martinka, M., Vaculik, M., and White, P. J. (2011). Root responses to cadmium in the rhizosphere: a review. J. Exp. Bot. 62, 21–37. doi: 10.1093/jxb/erq281
Mann, S. S., and Ritchie, G. S. (1995). Forms of cadmium in sandy soils after amendment with soils of higher fixing capacity. Environ. Pollut. 87, 23–29. doi: 10.1016/s0269-7491(99)80004-9
Martinoia, E., Grill, E., Tommasini, R., Kreuz, K., and Amrhein, N. (1993). ATP-dependent glutathione s-conjugate ‘export’ pump in the vacuolar membrane of plants. Nature 364, 247–249. doi: 10.1038/364247a0
Mench, M., and Martin, E. (1991). Mobilization of cadmium and other metals from two soils by root exudates of Zea mays L., Nicotiana tabacum L. and Nicotiana rustica L. Plant Soil 132, 187–196. doi: 10.1007/BF00010399
Mendoza-Cózatl, D. G., Jobe, T. O., Hauser, F., and Schroeder, J. I. (2011). Long-distance transport, vacuolar sequestration, tolerance, and transcriptional responses induced by cadmium and arsenic. Curr. Opin. Plant Biol. 14, 554–562. doi: 10.1016/j.pbi.2011.07.004
Milner, M. J., Seamon, J., Craft, E., and Kochian, L. V. (2013). Transport properties of members of the ZIP family in plants and their role in Zn and Mn homeostasis. J. Exp. Bot. 64, 369–381. doi: 10.1093/jxb/ers315
Miyadate, H., Adachi, S., Hiraizumi, A., Tezuka, K., Nakazawa, N., Kawamoto, T., et al. (2011). OsHMA3, a P1B-type of ATPase affects root-to-shoot cadmium translocation in rice by mediating efflux into vacuoles. New Phytol. 189, 190–199. doi: 10.1111/j.1469-8137.2010.03459.x
Mohammed, A. S., Kapri, A., and Goel, R. (2011). Heavy metal pollution: source, impact, and remedies. Environ. Pollut. 20, 1–28. doi: 10.1007/978-94-007-1914-9_1
Moons, A. (2003). Ospdr9, which encodes a PDR-type ABC transporter is induced by heavy metals, hypoxic stressand redox perturbations in rice roots. FEBS Lett. 553, 370–376. doi: 10.1016/s0014-5793(03)01060-3
Morel, M., Crouzet, J., Gravot, A., Auroy, P., Leonhardt, N., Vavasseur, A., et al. (2009). AtHMA3, a P(1B)-ATPase allowing cd/Zn/co/Pb aacuolar storage in Arabidopsis. Plant Physiol. 149, 894–904. doi: 10.1104/pp.108.130294
Nakanishi, H., Ogawa, I., Ishimaru, Y., Mori, S., and Nishizawa, N. K. (2006). Iron deficiency enhances cadmium uptake and translocation mediated by the Fe2+ transporters OsIRT1 and OsIRT2 in rice. Soil Sci. Plant Nutr. 52, 464–469. doi: 10.1111/j.1747-0765.2006.00055.x
Nevo, Y., and Nelson, N. (2006). The NRAMP family of metal-ion transporters. BBA-Mol. Cell. Res. 1763, 609–620. doi: 10.1016/j.bbamcr.2006.05.007
Oda, K., Otani, M., Uraguchi, S., Akihiro, T., and Fujiwara, T. (2011). Rice ABCG43 is cd inducible and confers Cd tolerance on yeast. Biosci. Biotech. Bioch. 75, 1211–1213. doi: 10.1271/bbb.110193
Ouariti, O., Gouia, H., and Ghorbal, M. H. (1997). Responses of bean and tomato plants to cadmium: growth mineral nutrition and nitrate reduction. Plant Physiol. Bioch. 114, 345–352. doi: 10.1104/pp.114.1.345
Pan, X., Li, Y., Liu, W., Liu, S., Min, J., Xiong, H., et al. (2020). QTL mapping andcandidate gene analysis of cadmium accumulation in polished rice by genome-wide association study. Sci. Rep. 10:11791. doi: 10.1038/s41598-020-68742-4
Pinson, S. R. M., Tarpley, L., Yan, W. G., Yeater, K., Lahner, B., Yakubova, E., et al. (2015). World-wide genetic diversity for mineral element concentrations in rice grain. Crop Sci. 55, 294–311. doi: 10.2135/cropsci2013.10.0656
Ramegowda, Y., Venkategowda, R., Jagadish, P., Govind, G., Hanumanthareddy, R. R., Makarla, U., et al. (2013). Expression of a rice Zn transporter, OsZIP1, increases Zn concentration in tobacco and finger millet transgenic plants. Plant Biotechnol. Rep. 7, 309–319. doi: 10.1007/s11816-012-0264-x
Ramesh, S. A., Shin, R., Eide, D. J., and Schachtman, D. P. (2003). Differential metal selectivity and gene expression of two zinc transporters from rice. Plant Physiol. 133, 126–134. doi: 10.1104/pp.103.026815
Redondo-Gómez, S., Mateos-Naranjo, E., and Andrades-Moreno, L. (2010). Accumulation and tolerance characteristics of cadmium in a halophytic cd-hyperaccumulator Arthrocnemum macrostachyum. J. Hazard. Mater. 184, 299–307. doi: 10.1016/j.jhazmat.2010.08.036
Rizwan, M., Ali, S., Adrees, M., Rizvi, H., Zia-Ur-Rehman, M., Hannan, F., et al. (2016). Cadmium stress in rice: toxic effects, tolerance mechanisms, and management: a critical review. Environ. Sci. Pollut. Res. Int. 23, 17859–17879. doi: 10.1007/s11356-016-6436-4
Sasaki, A., Yamaji, N., and Ma, J. F. (2014). Overexpression of OsHMA3 enhances Cd tolerance and expression of Zn transporter genes in rice. J. Exp. Bot. 65, 6013–6021. doi: 10.1093/jxb/eru340
Sasaki, A., Yamaji, N., Mitani-Ueno, N., Kashino, M., and Ma, J. F. (2015). A node-localized transporter OsZIP3 is responsible for the preferential distribution of Zn to developing tissues in rice. Plant J. 84, 374–384. doi: 10.1111/tpj.13005
Sasaki, A., Yamaji, N., Yokosho, K., and Ma, J. F. (2012). Nramp5 is a major transporter responsible for manganese and cadmium uptake in rice. Plant Cell 24, 2155–2167. doi: 10.1105/tpc.112.096925
Satoh-Nagasawa, N., Mori, M., Nakazawa, N., Kawamoto, T., Nagato, Y., Sakurai, K., et al. (2012). Mutations in rice (Oryza sativa) heavy metal ATPase 2 (OsHMA2) restrict the translocation of zinc and cadmium. Plant Cell Physiol. 53, 213–224. doi: 10.1093/pcp/pcr166
Shah, K., Kumar, R. G., Verma, S., and Dubey, R. S. (2001). Effect of cadmium on lipid peroxidation, superoxide anion generation and activities of antioxidant enzymes in growing rice seedlings. Plant Sci. 161, 1135–1144. doi: 10.1016/S0168-9452(01)00517-9
Shim, D., Hwang, J. U., Lee, J., Lee, S., Choi, Y., An, G., et al. (2009). Orthologs of the class A4 heat shock transcription factor HsfA4a confer cadmium tolerance in wheat and rice. Plant Cell 21, 4031–4043. doi: 10.1105/tpc.109.066902
Shimo, H., Ishimaru, Y., An, G., Yamakawa, T., Nakanishi, H., and Nishizawa, N. K. (2011). Low cadmium (LCD), anovel gene related to cadmium tolerance and accumulation in rice. J. Exp. Bot. 62, 5727–5734. doi: 10.1093/jxb/err300
Song, W. E., Chen, S. B., Liu, J. F., Chen, L., Song, N. N., Li, N., et al. (2015). Variation of Cd concentration in various rice cultivars and derivation of cadmium toxicity thresholds for paddy soil by species-sensitivity distribution. J. Integr. Agr. 14, 1845–1854. doi: 10.1016/S2095-3119(14)60926-6
Song, Y., Jin, L., and Wang, X. (2017b). Cadmium absorption and transportation pathways in plants. Int. J. Phytoremediation 19, 133–141. doi: 10.1080/15226514.2016.1207598
Song, W. Y., Lee, H. S., Jin, S. R., Ko, D., Martinoia, E., Lee, Y., et al. (2015). Rice PCR1 influences grain weight and Zn accumulation in grains. Plant Cell Environ. 38, 2327–2339. doi: 10.1111/pce.12553
Song, Y., Wang, Y., Mao, W., Sui, H., Yong, L., Yang, D., et al. (2017a). Dietary cadmium exposure assessment among the Chinese population. PLoS One 12:e0177978. doi: 10.1371/journal.pone.0177978
Stephens, B. W., Cook, D. R., and Grusak, M. A. (2011). Characterization of zinc transport by divalent metal transporters of the ZIP family from the model legume Medicago truncatula. Biometals 24, 51–58. doi: 10.1007/s10534-010-9373-6
Takahashi, R., Ishimaru, Y., Senoura, T., Shimo, H., Ishikawa, S., Arao, T., et al. (2011). The OsNRAMP1 iron transporter is involved in Cd accumulation in rice. J. Exp. Bot. 62, 4843–4850. doi: 10.1093/jxb/err136
Takahashi, R., Ishimaru, Y., Shimo, H., Ogo, Y., Senoura, T., Nishizawa, N. K., et al. (2012). The OsHMA2 transporter is involved in root-to-shoot translocation of Zn and cd in rice. Plant Cell Environ. 35, 1948–1957. doi: 10.1111/j.1365-3040.2012.02527.x
Tan, L., Qu, M., Zhu, Y., Peng, C., Wang, J., Gao, D., et al. (2020). ZINC TRANSPORTER5 and ZINC TRANSPORTER9 function synergistically in zinc/cadmium uptake. Plant Physiol. 183, 1235–1249. doi: 10.1104/pp.19.01569
Tan, L., Zhu, Y., Fan, T., Peng, C., Wang, J., Sun, L., et al. (2019). OsZIP7 functions in xylem loading in roots and inter-vascular transfer in nodes to deliver Zn/cd to grain in rice. Biochem. Bioph. Res Co. 512, 112–118. doi: 10.1016/j.bbrc.2019.03.024
Tanakak, K., Fujimakil, S., Fujiwara, T., Yonevama, T., and Hayashi, H. (2007). Quantitative estimation of the contribution of the phloem in cadmium transport to grains in Rice plants. Soil Sci. Plant Nutr. 53, 72–77. doi: 10.1111/j.1747-0765.2007.00116.x
Tang, L., Dong, J., Tan, L., Ji, Z., Li, Y., Sun, Y., et al. (2021). Overexpression of OsLCT2, a low-affinity cation transporter gene, reduces cadmium accumulation in shoots and grains of rice. Rice 14, 89–15. doi: 10.1186/s12284-021-00530-8
Tian, S., Liang, S., Qiao, K., Wang, F., Zhang, Y., and Chai, T. (2019). Co-expression of multiple heavy metal transporters changes the translocation, accumulation, and potential oxidative stress of cd and Zn in rice (Oryza sativa). J. Hazard. Mater. 380:120853. doi: 10.1016/j.jhazmat.2019.120853
Tiong, J., McDonald, G., Genc, Y., Shirley, N., Langridge, P., and Huang, C. Y. (2015). Increased expression of six ZIP family genes by zinc (Zn) deficiency is associated with enhanced uptake and root-to-shoot translocation of Zn in barley (Hordeum vulgare). New Phytol. 207, 1097–1109. doi: 10.1111/nph.13413
Ueno, D., Koyama, E., Kono, I., Ando, T., Yano, M., and Ma, J. F. (2009a). Identification of a novel major quantitative trait locus controlling distribution of cd between roots and shoots in rice. Plant Cell Physiol. 50, 2223–2233. doi: 10.1093/pcp/pcp160
Ueno, D., Kono, I., Yokosho, K., Ando, T., Yano, M., and Ma, J. F. (2009b). A major quantitative trait locus controlling cadmium translocation in rice (Oryza sativa). New Phytol. 182, 644–653. doi: 10.1111/j.1469-8137.2009.02784.x
Ueno, D., Milner, M. J., Yamaji, N., Yokosho, K., Koyama, E., Zambrano, M. C., et al. (2011). Elevated expression of TcHMA3 plays a key role in the extreme Cd tolerance in a cd-hyperaccumulating ecotype of Thlaspi caerulescens. Plant J. 66, 852–862. doi: 10.1111/j.1365-313x.2011.04548.x
Ueno, D., Yamaji, N., Kono, I., Huang, C. F., Ando, T., Yano, M., et al. (2010). Gene limiting cadmium accumulation in rice. Proc. Natl. Acad. Sci. U.S.A. 107, 16500–16505. doi: 10.1073/pnas.1005396107
Uraguchi, S., and Fujiwara, T. (2012). Cadmium transport and tolerance in rice: perspectives for reducing grain cadmium accumulation. Rice 5:5. doi: 10.1186/1939-8433-5-5
Uraguchi, S., Kamiya, T., Sakamoto, T., Kasai, K., Sato, Y., Nagamura, Y., et al. (2011). Low-affinity cation transporter (OsLCT1) regulates cadmium transport into rice grains. Proc. Natl. Acad. Sci. U.S.A. 108, 20959–20964. doi: 10.1073/pnas.1116531109
Uraguchi, S., Mori, S., Kuramata, M., Kawasaki, A., Arao, T., and Ishikawa, S. (2009). Root-to-shoot cd translocation via the xylem is the major process determining shoot and grain cadmium accumulation in Rice. J. Exp. Bot. 60, 2677–2688. doi: 10.1093/jxb/erp119
Verbruggen, N., Hermans, C., and Schat, H. (2009). Mechanisms to cope with arsenic or cadmium excess in plants. Curr. Opin. Plant Biol. 12, 364–372. doi: 10.1016/j.pbi.2009.05.001
Verrier, P. J., Bird, D., Burla, B., Dassa, E., Forestier, C., Geisler, M., et al. (2008). Plant ABC proteins-a unified nomenclature and updated inventory. Trends Plant Sci. 13, 151–159. doi: 10.1016/j.tplants.2008.02.001
Wang, C., Guo, W., Cai, X., Li, R., and Ow, D. W. (2019). Engineering low-cadmium rice through stress-inducible expression of OXS3-family member genes. New Biotechnol. 48, 29–34. doi: 10.1016/j.nbt.2018.04.004
Wang, C., Guo, W., Ye, S., Wei, P., and Ow, D. W. (2016). Reduction of cd in Rice through expression of OXS3-like gene fragments. Mol. Plant 9, 301–304. doi: 10.1016/j.molp.2015.09.006
Wang, X., Song, Y., Ma, Y., Zhuo, R., and Jin, L. (2011). Screening of Cd tolerant genotypes and isolation of metallothionein genes in alfalfa (Medicago sativa L.). Environ. Pollut. 159, 3627–3633. doi: 10.1016/j.envpol.2011.08.001
Williams, L. E., and Mills, R. F. (2005). P1B-ATPases-an ancient family of transition metal pumps with diverse functions in plants. Trends Plant Sci. 10, 491–502. doi: 10.1016/j.tplants.2005.08.008
Xia, J., Yamaji, N., Kasai, T., and Ma, J. (2010). Plasma membrane localized transporter for aluminum in rice. Proc. Natl. Acad. Sci. U.S.A. 107, 18381–18385. doi: 10.1073/pnas.1004949107
Xu, X., Zhao, Y., Zhao, X., Wang, Y., and Deng, W. (2014). Sources of heavy metal pollution in agricultural soils of a rapidly industrializing area in the Yangtze Delta of China. Ecotoxicol. Environ. Saf. 108, 161–167. doi: 10.1016/j.ecoenv.2014.07.001
Yadav, A. K., Shankar, A., Jha, S. K., Kanwar, P., Pandey, A., and Pandey, G. K. (2015). A rice tonoplastic calcium exchanger, OsCCX2 mediates Ca2+/cation transport in yeast. Sci. Rep. 5:17117. doi: 10.1038/srep17117
Yamaji, N., Xia, J., Mitani-Ueno, N., Yokosho, K., and Feng Ma, J. (2013). Preferential delivery of zinc to developing tissues in rice is mediated by P-type heavy metal ATPase OsHMA2. Plant Physiol. 162, 927–939. doi: 10.1104/pp.113.216564
Yan, J., Wang, P., Wang, P., Yang, M., Lian, X., Tang, Z., et al. (2016). A loss-of-function allele of OsHMA3 associated with high cadmium accumulation in shoots and grain of japonica rice cultivars. Plant Cell Environ. 39, 1941–1954. doi: 10.1111/pce.12747
Yan, H., Xu, W., Xie, J., Gao, Y., Wu, L., Sun, L., et al. (2019). Variation of a major facilitator superfamily gene contributes to differential cadmium accumulation between rice subspecies. Nat. Commun. 10:2562. doi: 10.1038/s41467-019-10544-y
Yang, G., Fu, S., Huang, J., Li, L., Long, Y., Wei, Q., et al. (2021). The tonoplast-localized transporter OsABCC9 is involved in cadmium tolerance and accumulation in rice. Plant Sci. 307:110894. doi: 10.1016/j.plantsci.2021.110894
Yang, J., Gao, M. X., Hu, H., Ding, X. M., Lin, H. W., Wang, L., et al. (2016). OsCLT1, a CRT-like transporter 1, is required for glutathione homeostasis and arsenic tolerance in rice. New Phytol. 211, 658–670. doi: 10.1111/nph.13908
Yang, M., Zhang, Y., Zhang, L., Hu, J., Zhang, X., Lu, K., et al. (2014). OsNRAMP5 contributes to manganese translocation and distribution in rice shoots. J. Exp. Bot. 65, 4849–4861. doi: 10.1093/jxb/eru259
Yang, M., Li, Y., Liu, Z., Tian, J., Liang, L., Qiu, Y., et al. (2020). A high activity zinc transporter OsZIP9 mediates zinc uptake in rice. Plant J. 103, 1695–1709. doi: 10.1111/tpj.14855
Ye, X., Ma, Y., and Sun, B. (2012). Influence of soil type and genotype on cd bioavailability and uptake by rice and implications for food safety. J. Environ. Sci. 24, 1647–1654. doi: 10.1016/s1001-0742(11)60982-0
Yu, C., Sun, C., Shen, C., Wang, S., Liu, F., Liu, Y., et al. (2015). The auxin transporter, OsAUX1, is involved in primary root and root hair elongation and in cd stress responses in rice (Oryza sativa L.). Plant J. 83, 818–830. doi: 10.1111/tpj.12929
Yu, J., Liu, C., Lin, H., Zhang, B., Li, X., Yuan, Q., et al. (2021). Loci and natural alleles for cadmium-mediated growth responses revealed by a genome wide association study and transcriptome analysis in rice. BMC Plant Biol. 21:374. doi: 10.1186/s12870-021-03145-9
Yu, H., Wang, J., Fang, W., Yuan, J., and Yang, Z. (2006). Cadmium accumulation in different rice cultivars and screening for pollution-safe cultivars of rice. Sci. Total Environ. 370, 302–309. doi: 10.1016/j.scitotenv.2006.06.013
Yuan, L., Yang, S., Liu, B., Zhang, M., and Wu, K. (2012). Molecular characterization of a rice metal tolerance protein, OsMTP1. Plant Cell Rep. 31, 67–79. doi: 10.1007/s00299-011-1140-9
Zhao, F. J., Ma, Y. B., Zhu, Y. G., Tang, Z., and McGrath, S. P. (2015). Soil contamination in China: current status and mitigation strategies. Environ. Sci. Technol. 49, 750–759. doi: 10.1021/es5047099
Zhao, J., Yang, W., Zhang, S., Yang, T., Liu, Q., Dong, J., et al. (2018). Genome-wide association study and candidate gene analysis of rice cadmium accumulation in grain in a diverse rice collection. Rice 11:61. doi: 10.1186/s12284-018-0254-x
Keywords: cadmium (Cd), translocation, transporter, quantitative trait locus (QTL), rice (Oryza sativa L.), uptake
Citation: Ai H, Wu D, Li C and Hou M (2022) Advances in molecular mechanisms underlying cadmium uptake and translocation in rice. Front. Plant Sci. 13:1003953. doi: 10.3389/fpls.2022.1003953
Received: 27 July 2022; Accepted: 29 August 2022;
Published: 20 September 2022.
Edited by:
Ryoung Shin, RIKEN Center for Sustainable Resource Science (CSRS), Yokohama, JapanReviewed by:
Hiroshi Masuda, Akita Prefectural University, Akita, JapanCopyright © 2022 Ai, Wu, Li and Hou. This is an open-access article distributed under the terms of the Creative Commons Attribution License (CC BY). The use, distribution or reproduction in other forums is permitted, provided the original author(s) and the copyright owner(s) are credited and that the original publication in this journal is cited, in accordance with accepted academic practice. No use, distribution or reproduction is permitted which does not comply with these terms.
*Correspondence: Mengmeng Hou, aG91bWVuZ21lbmdAY2Fhcy5jbg==
Disclaimer: All claims expressed in this article are solely those of the authors and do not necessarily represent those of their affiliated organizations, or those of the publisher, the editors and the reviewers. Any product that may be evaluated in this article or claim that may be made by its manufacturer is not guaranteed or endorsed by the publisher.
Research integrity at Frontiers
Learn more about the work of our research integrity team to safeguard the quality of each article we publish.