- 1Blueberry Breeding and Genomics Lab, Department of Horticultural Sciences, University of Florida, Gainesville, FL, United States
- 2Plant Molecular and Cellular Biology Program, University of Florida, Gainesville, FL, United States
- 3Department of Horticultural Sciences, University of Florida, Gainesville, FL, United States
Branched-chain volatiles (BCVs) constitute an important family of fruit volatile metabolites essential to the characteristic flavor and aroma profiles of many edible fruits. Yet in contrast to other groups of volatile organic compounds important to fruit flavor such as terpenoids, phenylpropanoids, and oxylipins, the molecular biology underlying BCV biosynthesis remains poorly understood. This lack of knowledge is a barrier to efforts aimed at obtaining a more comprehensive understanding of fruit flavor and aroma and the biology underlying these complex phenomena. In this review, we discuss the current state of knowledge regarding fruit BCV biosynthesis from the perspective of molecular biology. We survey the diversity of BCV compounds identified in edible fruits as well as explore various hypotheses concerning their biosynthesis. Insights from branched-chain precursor compound metabolism obtained from non-plant organisms and how they may apply to fruit BCV production are also considered, along with potential avenues for future research that might clarify unresolved questions regarding BCV metabolism in fruits.
Introduction
Volatile organic compounds (VOCs) are essential components of fruit flavor, being necessary for human perception of the distinct flavors produced by the many different types of fruit found in nature (Noble, 1996; Goff and Klee, 2006; El Hadi et al., 2013; Plotto et al., 2017). The importance of VOCs to fruit flavor has prompted researchers to investigate the underlying biosynthetic pathways responsible for their formation. Great progress has been made in understanding the molecular basis of terpenoid (Cheng et al., 2007; Nagegowda, 2010; Tholl, 2015; Abbas et al., 2017), phenylpropanoid (Boatright et al., 2004; Qualley et al., 2012; Lackus et al., 2021), and oxylipin (Mosblech et al., 2009; Scala et al., 2013; Griffiths, 2015; Ameye et al., 2018) volatile biosynthesis in plants. However, much less is known about the molecular correlates underlying the production of branched-chain volatiles (BCVs), a family of compounds encompassing some VOCs that are notable contributors to the flavor of several important fruit crops.
Branched chain volatiles are defined as those volatile organic compounds that contain a branched-chain functional group structurally similar to those of the branched-chain amino acids- valine, leucine, and isoleucine (Figure 1). Due to this structural similarity BCVs were theorized to derive from the metabolism of branched-chain amino acids (BCAAs), a hypothesis supported by numerous feeding experiments (Tressl and Drawert, 1973; Hansen and Poll, 1993; Rowan et al., 1996; Wyllie and Fellman, 2000; Pérez et al., 2002; Matich and Rowan, 2007; Gonda et al., 2010). Yet while thorough work has been done elucidating the biosynthesis and metabolism of branched-chain amino acids in plants (Singh and Shaner, 1995; Taylor et al., 2004; Binder et al., 2007; Binder, 2010; Xing and Last, 2017), the precise enzymatic mechanisms by which BCAA metabolism diverges into BCV biosynthesis remain relatively understudied. Furthermore, recent experimental evidence indicates that under certain circumstances BCV production may occur independently of normal BCAA metabolism (Sugimoto, 2011; Kochevenko et al., 2012; Sugimoto et al., 2021). A more complete understanding of BCV biosynthesis in plants would be of great importance to researchers working to better understand the molecular basis of fruit flavor, and in particular to those who desire to apply such knowledge to the development of novel fruit varieties with improved flavor traits.
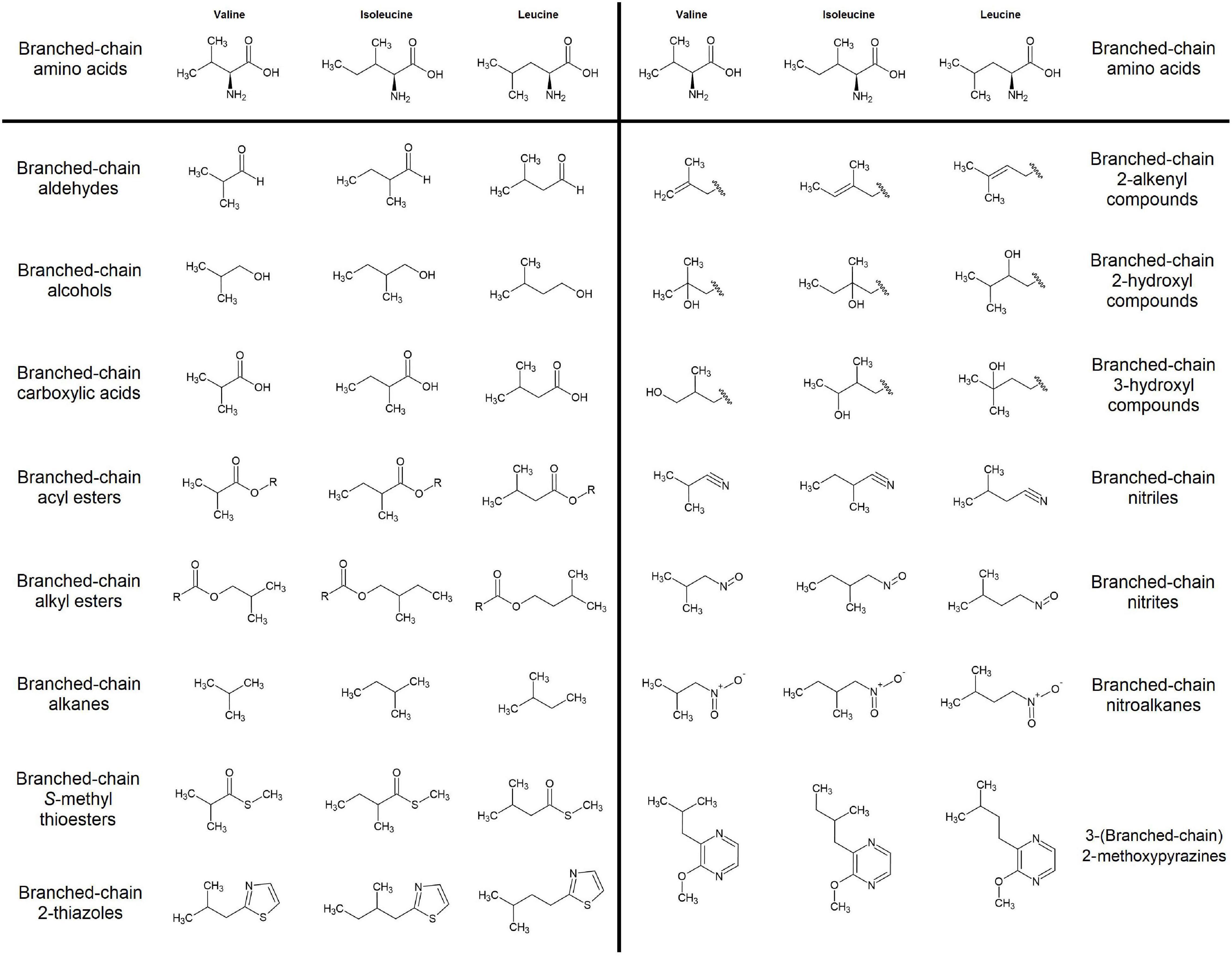
Figure 1. Categories of branched-chain volatile compounds detected in edible fruits. At least one volatile from each family was reported in at least one of the 175 fruit volatile studies examined in this review (see Supplementary Table 1).
This review seeks to collate and evaluate published research regarding the biosynthesis of branched chain volatiles in plants, with an emphasis on these processes as they might occur in fruit crops. While BCV biosynthesis has been touched upon in other reviews on the subject of plant volatiles (Dudareva et al., 2006, 2013; El Hadi et al., 2013), a comprehensive exploration of the state of current knowledge concerning the molecular basis of BCV biosynthesis in plants has yet to be published.
Branched-Chain Volatiles in Fruits
Branched-chain volatiles are ubiquitous components of fruit aroma volatile profiles. An examination of research literature concerning fruit volatile profiles found that 127 unique branched-chain volatile compounds were identified in 106 distinct types of edible fruit across 175 published studies (Table 1). A list of all BCVs reported for a particular fruit by these studies is given in Supplementary Table 1. These fruits include representatives from 22 individual taxonomic orders spanning monocots, eudicots, and magnoliids, suggesting that the production of branched-chain volatiles in fruit tissue is a characteristic with widespread evolutionary utility. It has been proposed that since many fruit volatiles are derived from nutritionally significant compounds, their production in fruit tissue might be a means of signaling nutritional value to seed-dispersing frugivores (Goff and Klee, 2006). Because animals are incapable of biosynthesizing branched-chain amino acids (Hou and Wu, 2018), volatile cues enabling the identification of food sources rich in these compounds could serve as powerful attractants to animal seed-dispersers. This may be one explanation for the ubiquity of branched-chain volatiles in fruits from plants across many divergent evolutionary lineages, though much more research is needed to firmly establish this hypothesis. Conversely BCVs may also find use as defense agents against herbivorous predators, an application associated with certain nitrogen-containing branched-chain VOCs (Irmisch et al., 2013, 2014).

Table 1. List of all branched-chain volatile compounds detected in 106 edible fruits across 175 published studies of fruit volatile content.
An overwhelming majority of the branched-chain volatiles identified in fruits are classified as volatile esters. Out of the 127 distinct BCVs identified in Table 1, 108 are esters. Many of these are conjugate esters derived from a branched-chain structure attached to a compound from a completely different biosynthetic origin. In this way, branched-chain volatiles incorporating structures derived from terpenoid, phenylpropanoid, and oxylipin metabolism are formed- allowing for massive diversity in the number of possible volatiles containing a branched-chain structure. The remaining non-ester BCVs observed in Table 1 include branched-chain alcohols, aldehydes, alkanes, and carboxylic acids. Several BCVs containing more unusual functional groups are also listed, including compounds with nitrile, nitrite, nitro, and thioester functional groups.
Branched-chain volatiles have been recognized as being crucially responsible for the distinctive flavor and aroma properties of many commercially important fruits such as apple, banana, melon, and pineapple, among others (Wyllie et al., 1995; Plotto, 1998; Dixon and Hewett, 2000; Boudhrioua et al., 2003; Tokitomo et al., 2005; Wendakoon et al., 2006). In terms of effect on human sensory perception it has been widely reported that branched-chain volatile esters tend to impart characteristic “fruity” aroma notes, while non-ester branched-chain volatiles induce a broader range of olfactory sensations (Schwab et al., 2008; Sugimoto, 2011; El Hadi et al., 2013; Lytra et al., 2014). Fruity aroma is a characteristic shared with several other non-branched-chain volatile compounds, particularly short- and medium- length straight-chain esters (Schwab et al., 2008; El Hadi et al., 2013). However, it has been observed that ester compounds containing the branched-chain moiety have significantly lower odor thresholds than the corresponding straight-chain counterparts (Takeoka et al., 1995), indicating that BCVs may be more potent stimulators of the “fruity” olfactory sensation. It is important to communicate that the “fruity” aroma notes imparted by BCVs are not always associated with positive consumer responses, and in some fruits are associated with decreased consumer ratings (Goulet et al., 2012).
Current Knowledge Regarding Branched-Chain Volatile Biosynthesis
Because of the importance of branched-chain volatiles to the characteristic flavors of several important fruit crops much research has been done in trying to understand the general biosynthesis pathways that lead to BCV production, though this area remains relatively understudied when compared to the biosynthetic processes that produce other classes of important fruit volatiles such as terpenoids and oxylipins. The body of published experimental evidence regarding this topic points to four possible hypotheses regarding BCV biosynthesis (Figure 2), none of which are mutually exclusive with any of the others. These hypotheses are summarized below.
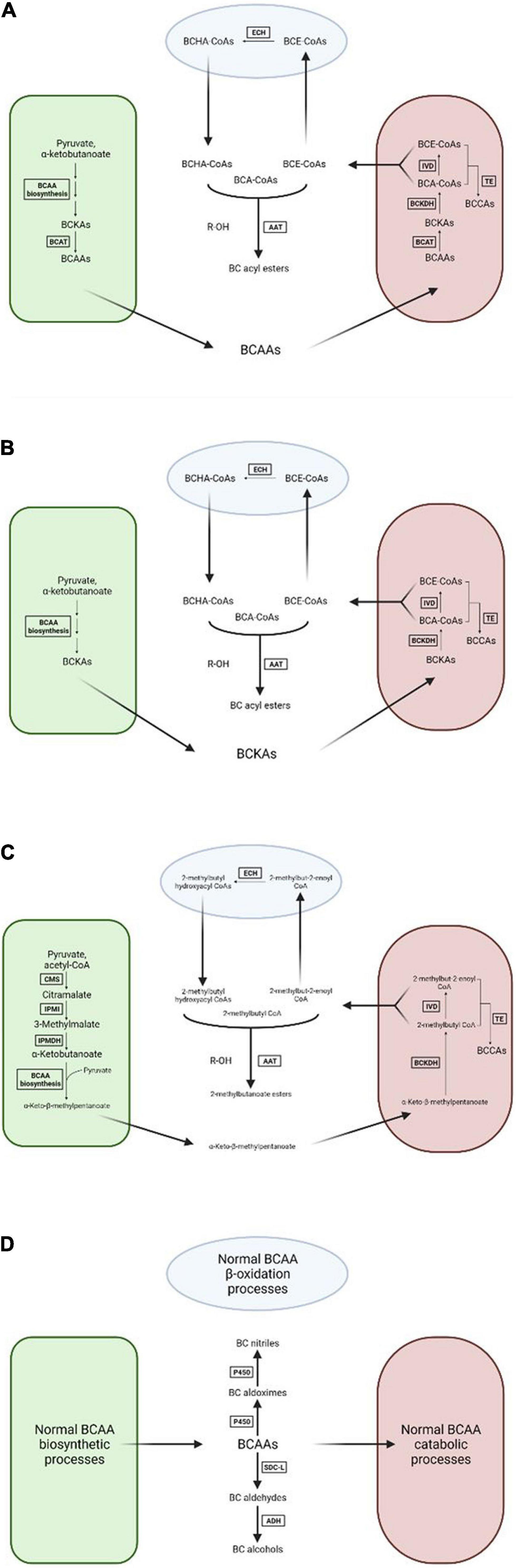
Figure 2. Visual diagrams of the four hypotheses concerning branched-chain volatile biosynthesis in plant cells. (A) Mitochondrial catabolism of branched-chain amino acids. (B) De novo branched-chain α-ketoacid biosynthesis followed by mitochondrial catabolism. (C) Production of 2-methylbutyl volatiles by citramalate synthase. (D) Direct transformation of branched-chain amino acids. BCAA, branched-chain amino acid; BCKA, branched-chain α-ketoacid; BCAT, branched-chain amino acid aminotransferase; BCKDH, branched-chain α-ketoacid dehydrogenase complex; IVD, isovaleryl-CoA dehydrogenase; TE, thioesterase; ECH, enoyl-CoA hydratase; AAT, alcohol acyltransferase; CMS, citramalate synthase; IPMI, isopropylmalate isomerase; IPMDH, isopropylmalate dehydrogenase; P450, cytochrome P450 enzyme; SDC-L, serine-decarboxylase like enzyme; ADH, alcohol dehydrogenase. Green compartment represents the chloroplast, maroon compartment the mitochondrion, light blue compartment the peroxisome, and white background the cytosol. Arrows with faded ends indicate cross-membrane transport.
Mitochondrial Catabolism of Branched-Chain Amino Acids
The oldest and most studied of the four possibilities, this hypothesis posits that BCVs are generated through the catabolic degradation of BCAAs in mitochondria. It has long been known that plants possess the capability to break down excess BCAAs into the energy-rich metabolites acetyl-CoA and propionyl-CoA and that several of the key enzymes involved in this process are localized to mitochondria (Binder, 2010; Hildebrandt et al., 2015). An excellent overview of this pathway as hypothesized to occur in plants is provided in Binder (2010). Briefly, this process begins with the deamination of BCAAs by branched-chain aminotransferases (BCATs) followed by decarboxylation of the resulting branched-chain α-ketoacids (BCKAs) through the branched-chain α-ketoacid dehydrogenase complex (BCKDH). This results in the formation of various branched-chain acyl-CoAs (BCA-CoAs), which in turn are reduced at the α-carbon through the action of isovaleryl-CoA dehydrogenases or functionally similar enzymes. These reduced BCA-CoAs then undergo β-oxidation into acetyl-CoA and propionyl-CoA, though whether these later stages occur in mitochondria or in peroxisomes remains unresolved (Graham and Eastmond, 2002; Kaur et al., 2009).
Since numerous volatile esters are known to be biosynthesized in fruit tissue by alcohol acyltransferase (AAT) enzymes (Beekwilder et al., 2004; El-Sharkawy et al., 2005; Souleyre et al., 2005; Günther et al., 2011; Goulet et al., 2015) and because AATs require an alcohol and an acyl-CoA as substrates, the branched-chain acyl-CoAs produced by mitochondrial BCAA catabolism would naturally be considered likely candidates for conversion to volatile branched-chain acyl esters. Feeding and isotope labeling experiments conducted in apple, banana, and strawberry provide strong evidence that BCAAs can be directly converted to volatile branched-chain acyl esters in fruit tissue (Tressl and Drawert, 1973; Rowan et al., 1996; Wyllie and Fellman, 2000; Pérez et al., 2002). Furthermore, the detection in several fruits of volatile branched-chain acyl esters with double bonds and hydroxyl groups in positions necessary for β-oxidation to proceed indicate that intermediaries of late-stage BCAA catabolism are actively incorporated into volatile fruit compounds. And it has also been demonstrated in hops that a mitochondrial thioesterase is capable of cleaving BCA-CoAs into branched-chain carboxylic acids (Xu et al., 2013), important aroma volatiles detected in many kinds of fruit (Supplementary Table 1). Notably, it was found that when apple and banana fruit tissue was fed with deuterium-labeled BCAAs, deuterated branched-chain carboxylic acid production was observed (Tressl and Drawert, 1973; Rowan et al., 1996). Taken together, these lines of evidence strongly support the hypothesis that fruit BCVs are formed through catabolic breakdown of BCAAs.
However, several difficulties remaining with this hypothesis indicate that it may not be generally applicable across all fruits. Evidence from tomato seems to show that BCAA catabolism may not be primarily responsible for the formation of BCVs in that fruit; when disks of tomato fruit tissue were fed elevated levels of BCAAs, no significant measurable increase in BCV quantity was detected as compared to control (Kochevenko et al., 2012). Furthermore, overexpression of tomato BCAT genes did not result in plants yielding fruits that produced increased levels of BCVs (Kochevenko et al., 2012). Since, BCAT enzymes catalyze the first step in BCAA catabolic breakdown, overexpression of the genes encoding these proteins should theoretically result in higher levels of BCAAs entering the degradation pathway which in turn should yield more substrates for conversion into greater amounts of BCVs- results not observed in the tomato BCAT overexpression experiments. Other difficulties with the BCAA catabolism hypothesis deal with the issue of cellular regulation of BCAA metabolism: since it is known that under ordinary conditions the biosynthesis of BCAAs is tightly regulated through feedback inhibition (Binder, 2010; Galili et al., 2016; Xing and Last, 2017), it must be explained how some fruits can overcome these regulatory barriers to produce the great amounts of BCAAs and corresponding breakdown products needed to support the biosynthesis of massive quantities of BCVs observed in these fruits. By itself, the BCAA catabolism hypothesis is incapable of accounting for this. These difficulties have led to the proposal and investigation of other hypotheses regarding possible alternate routes for BCV biosynthesis.
De novo Branched-Chain α-Ketoacid Biosynthesis Followed by Mitochondrial Catabolism
Based primarily off of research done in tomato, this hypothesis posits that BCAAs are not the main source of carbon used for biosynthesis of fruit BCVs but that instead it is branched-chain α-ketoacids that fill this role. In this hypothesis, the biosynthesis of BCAAs proceeds as normal up until the last step, where instead of conversion to BCAAs by BCAT enzymes branched-chain α-ketoacids produced so far are directly exported to the mitochondria for BCKDH-mediated catabolism into BCVs and associated precursors. This hypothesis has several explanatory advantages. For one, it explains the findings in tomato that feeding excess BCAAs to tomato fruit tissue does not result in elevated BCV volatile production, while feeding excess BCKAs does (Kochevenko et al., 2012). Another advantage is that this hypothesis manages to bypass BCAA-mediated feedback inhibition of upstream biosynthesis enzymes, though inhibition by non-BCAA precursors generated by this pathway may still occur depending on the sensitivities of these enzymes to those compounds.
Much more experimental evidence is needed to conclude whether or not this hypothesized pathway is involved in BCV biosynthesis in certain fruits. In particular, labeling experiments where deuterated BCKAs are fed to fruit tissue and deuterated BCVs are observed but not deuterated BCAAs would provide robust evidence for the validity of this hypothesis. Supporting evidence could include an observation that BCAT transcripts become drastically reduced in fruit tissue as compared to the other upstream BCAA biosynthesis enzymes. These in vitro assays would show that BCKAs do not cause feedback inhibition of the upstream biosynthesis enzyme isoforms present in fruit, or the discovery and characterization of a chloroplast transporter expressed in fruit tissue that preferentially exports BCKAs before they can be converted to BCAAs by chloroplastic BCATs.
It is important to note that this hypothesis may not be mutually exclusive with the BCAA catabolism hypothesis: it may be possible that fruit cells produce elevated levels of BCKAs while also simultaneously catabolizing BCAAs into volatiles. In fact, doing both might actually increase the quantity of carbon shunted into mitochondrial BCKDH-mediated volatile precursor production: catabolizing BCAAs would reduce inhibitory pressure on key BCAA biosynthesis enzymes which would then be free to produce greater quantities of BCKAs that would be directly used to support BCV production. Further investigation is needed to determine if such a scenario indeed occurs in fruit tissue in nature and if so, what quantifiable impact each pathway has on overall BCV production.
Production of 2-Methylbutyl Volatiles by Citramalate Synthase
Work in apple has revealed a third possible route for the biosynthesis of BCVs. Unlike the first two hypothesized pathways, this route does not involve the early steps of classical plant BCAA biosynthesis but instead relies on the enzyme citramalate synthase (CMS), an enzyme previously known to be involved in BCAA biosynthesis only in bacteria (Sugimoto, 2011; Sugimoto et al., 2021). Briefly, in this pathway citramalate synthase condenses one molecule of pyruvate with one molecule of acetyl-CoA to form the dicarboxylic acid citramalate, a molecule of which is then converted to 3-methylmalate by the action of isopropylmalate isomerase (IPMI) enzymes. This compound is then acted upon by isopropylmalate dehydrogenase (IPMDH) enzymes to yield α-ketobutanoate, a known precursor of isoleucine biosynthesis via the established plant pathway. Biosynthesis to α-keto-β-methylpentanoate and isoleucine then proceeds along the conventional plant BCAA biosynthesis pathway. Direct experimental evidence for the activity of this pathway in apple has been obtained through the use of 13C-labeled acetate feeding and in vitro biochemical characterization of CMS and IPMI enzymes expressed in apple fruit tissue (Sugimoto, 2011; Sugimoto et al., 2021). Supporting evidence in the form of measured increases in levels of citramalate and CMS transcripts as apple ripening progressed was also obtained in the same studies.
The primary explanatory advantage of this hypothesis is that it enables the production of massive quantities of 2-methylbutyl volatile compounds in fruit without being subject to the feedback inhibition that large concentrations of isoleucine exert on threonine deaminase (TD), an enzyme that catalyzes the first committed step in isoleucine biosynthesis (Sidorov et al., 1981; Singh and Shaner, 1995; Binder, 2010; Sugimoto et al., 2021). Furthermore, additional evidence in apple indicates that fruit CMS enzymes may also play a role in biosynthesizing straight-chain esters, a class of compound commonly found at high levels in several fruits including apple (Plotto, 1998; El Hadi et al., 2013; Liu et al., 2021; Sugimoto et al., 2021). Nevertheless, this hypothesis alone suffers from a serious limitation: it is unable to account for the high levels of 3-methylbutyl volatiles observed in several fruits as well as elevated levels of 2-methylpropyl volatiles observed in others. Therefore, other hypotheses must be deferred to when considering the biosynthetic mechanisms underlying the formation of 3-methylbutyl and 2-methylpropyl fruit volatiles. The fact that 3-methylbutyl and 2-methylpropyl compounds have been detected in apple alongside high levels of 2-methylbutyl volatiles (Qin et al., 2017; Liu et al., 2021) seems to indicate that at least two separate and distinct BCV biosynthesis pathways can be active at the same time in this fruit.
Direct Transformation of Branched-Chain Amino Acids
The last possible mechanism of BCV biosynthesis is direct conversion of BCAAs into volatile compounds or immediate precursors. Rather than undergoing several steps of degradation through the established BCAA catabolism process before being incorporated into BCVs, this hypothesis proposes that some volatiles are directly transformed through enzymatic action into BCVs or the immediate precursors of such. Evidence from alfalfa and chickpea indicates that plants form branched-chain aldehydes and alcohols through this pathway: in both species, cDNAs for serine decarboxylase-like (SDC-L) enzymes were found that when heterologously expressed in bacteria produced enzymes capable of directly forming branched-chain aldehydes from branched-chain amino acids (Torrens-Spence et al., 2014). Branched-chain alcohols could then be formed through the action of alcohol dehydrogenase (ADH) enzymes acting on these branched-chain aldehydes. Similarly, work done in poplar demonstrated that branched-chain nitrile volatiles can be biosynthesized from BCAAs through the action of two cytochrome P450 enzymes, one that converts BCAAs into branched-chain aldoximes and another that subsequently converts the branched-chain aldoximes into branched-chain nitriles (Irmisch et al., 2013, 2014). Presumably, this pathway may also be involved in the biosynthesis of other nitrogen-containing BCVs reported from various fruits as it does not involve the loss of the original amino acid’s nitrogen atom but permits its refunctionalization into another chemical moiety. Indeed, evidence from grape indicates that branched-chain 2-methoxypyrazine volatiles are formed in just such a manner (Lei et al., 2019). Experiments involving feeding of 15N-labeled BCAAs to tissue from fruits known to produce nitrogenous BCVs would be one way to empirically demonstrate such a role for this pathway. The biosynthesis of both types of branched-chain volatile compound have been shown to be accomplished without the involvement of the mitochondrial BCKDH enzyme complex, a key feature of the previous three hypotheses.
It is interesting to note that this pathway can theoretically provide a possible route for the biosynthesis of branched-chain acyl esters that also does not rely on the activity of the mitochondrial BCKDH complex (Figure 3). In this proposed route, branched-chain aldehydes are generated by a serine decarboxylase-like enzyme as per that characterized by Torrens-Spence et al. (2014). These branched-chain aldehydes would then be converted to branched-chain carboxylic acids by the action of aldehyde dehydrogenases (ALDHs), enzymes known to be found in the genomes of many plants (Brocker et al., 2013; Tola et al., 2021). The resulting branched-chain carboxylic acids could then be turns into the corresponding branched-chain acyl-CoAs by carboxyl-CoA ligases (CCLs), as was demonstrated to occur in hops (Xu et al., 2013). This route is fully compatible with existing data from feeding and labeling experiments that demonstrate the conversion of BCAAs into branched-chain acyl esters. In addition, given that neither of the known BCV-forming SDC-like enzymes are predicted to localize to mitochondria (Torrens-Spence et al., 2014) and that several plant ALDHs are known to be localized to the cytosol (Končitíková et al., 2015; Tola et al., 2021) along with CCLs shown to activate branched-chain substrates (Xu et al., 2013), this proposed pathway solves the difficulty of explaining how BCA-CoAs produced in the mitochondria can cross the mitochondrial membrane into the cytosol where plant AAT enzymes have been shown to be localized (Noichinda et al., 1999a; Zhang et al., 2019b). Furthermore, since prior research indicates that mitochondrial BCKDH-mediated BCAA catabolism is tightly regulated by a variety of factors in plants (Fujiki et al., 2001; Peng et al., 2015) a BCA-CoA production pathway not involving the BCKDH complex would not need to overcome in-built regulatory hurdles to generate large quantities of BCA-CoAs. Several difficulties are still apparent with this hypothetical route to branched-chain acyl esters: the accumulation of high levels of aldehyde compounds is known to be toxic to cells, competition for aldehyde substrates with the ADH enzymes known to produce branched-chain alcohols, and the simple fact that no direct evidence of this alternate route has yet been empirically demonstrated. Experimental evidence such as deuterium-labeled branched-chain aldehydes yielding branched-chain acyl esters with deuterated acyl moieties or the characterization of fruit ALDH enzymes capable of forming branched-chain carboxylic acids from branched-chain aldehydes would go a long way to establish the viability of this proposed pathway to branched-chain acyl ester biosynthesis.
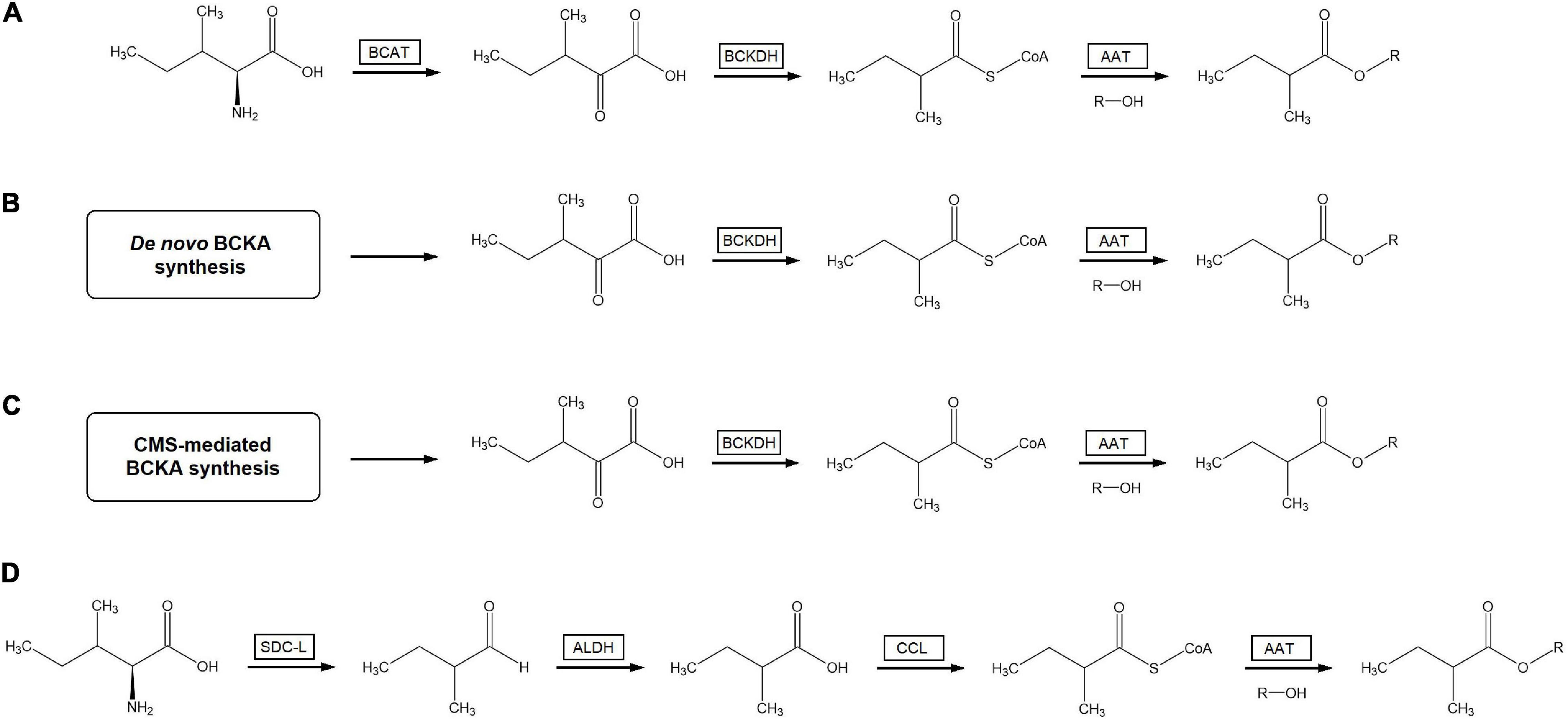
Figure 3. Possible biosynthesis routes to volatile branched-chain acyl esters. (A) Mitochondrial BCAT- and BCKDH-mediated catabolism of free branched-chain amino acids to branched-chain acyl-CoAs followed by esterification with alcohols by AAT enzymes. (B) De novo chloroplast synthesis of branched-chain α-ketoacids followed by BCKDH-mediated catabolism to branched-chain acyl-CoAs and subsequent AAT-mediated esterification with alcohols. (C) CMS-initiated synthesis of α-keto-β-methylpentanoate followed by BCKDH-mediated catabolism to 2-methylbutyl acyl-CoA and subsequent AAT-mediated esterification with alcohols to form 2-methylbutanoate esters. (D) Branched-chain aldehyde synthesis from free branched-chain amino acids via SDC-L, followed by conversion of branched-chain aldehydes to branched-chain carboxylic acids through ALDH enzymes, followed by activation to branched-chain acyl-CoAs by CCL enzymes and subsequent condensation with alcohols via AAT to generate branched-chain acyl esters. The first, second, and fourth routes are capable of generating acyl esters with all three branched-chain structures, while the third route can only generate 2-methylbutanoate esters. The four pathways are illustrated yielding 2-methylbutanoate esters from appropriate precursors for ease of comparison. BCAT, branched-chain amino acid aminotransferase; BCKDH, branched-chain α-ketoacid dehydrogenase complex; AAT, alcohol acyltransferase; BCKA, branched-chain α-ketoacids; SDC-L, serine-decarboxylase like enzyme; ALDH, aldehyde dehydrogenase; CCL, carboxyl-CoA ligase.
Unresolved Questions Concerning Branched-Chain Volatile Biosynthesis
Despite significant progress in understanding the molecular correlates of BCV biosynthesis in plants, much remains to be uncovered. In comparison, the metabolic bases of the biosynthesis of other important classes of fruit volatile such as the terpenoid, oxylipin, and phenylpropanoid families have been quite thoroughly characterized. For a similar level of understanding to be achieved for branched-chain volatiles, several important questions need to be resolved. A few of these unresolved questions regarding BCV biosynthesis most relevant to fruit volatile metabolism are explored below:
How Branched-Chain α-Ketoacid Dehydrogenase Complex-Mediated Branched-Chain Amino Acid Catabolism Is Regulated in Fruit Tissue
As has been discussed in previous sections of this review, branched-chain acyl esters have been shown to be among the most abundant BCVs detected in several important fruits and have also been demonstrated to be key components of many characteristic fruit flavors. The characterization of numerous fruit alcohol acyltransferase enzymes indicates that these volatiles are most likely formed through the condensation of alcohols with branched-chain acyl-CoAs (Beekwilder et al., 2004; El-Sharkawy et al., 2005; Souleyre et al., 2005; Günther et al., 2011). In three of the four hypotheses regarding BCV biosynthesis previously explored in this review, the mitochondrial BCKDH enzyme complex plays the critical role in generating the BCA-CoAs necessary for branched-chain acyl ester biosynthesis. Evidence in plants indicates that the activity of this complex is regulated by several factors (Fujiki et al., 2001; Peng et al., 2015), while work done on the far more studied mammalian BCKDH complex has identified several molecules that directly modulate BCKDH activity. These include a kinase which directly suppresses BCKDH activity and can itself be inhibited by branched-chain α-ketoacids (Paxton and Harris, 1984; Harris et al., 1986, 1997), phosphatase enzymes that reverse the effects of the kinase and promote activity of the BCKDH complex (Lu et al., 2009; Zhou et al., 2012) and even BCA-CoAs themselves, which have been shown to suppress BCKDH activity in vitro (Parker and Randle, 1978).
The overall question relevant to researchers working in the field of fruit aroma volatile metabolism is what role if any do these BCKDH regulatory mechanisms play in the generation of branched-chain acyl esters? If regulatory mechanisms that directly or indirectly suppress BCKDH activity in plants are identified, how are they overcome in fruit tissues to produce the great quantities of BCA-CoAs needed to support large-scale branched-chain acyl ester biosynthesis as observed in fruits such as apple? If plant homologs of the mammalian BCKDH kinase and phosphatase enzymes are found, are their expression levels in fruit negatively or positively correlated with branched-chain acyl ester content? Are subunits of the plant BCKDH complex inhibited by high levels of BCA-CoAs as was demonstrated to occur in the mammalian complex (Parker and Randle, 1978)? The answers to these questions and related ones would shed great insight into an important aspect of fruit BCV production and may even offer potential avenues for altering the BCV content of fruits by manipulating or even bypassing the underlying regulatory mechanisms governing branched-chain acyl ester precursor biosynthesis.
What Biosynthetic Processes Form Sulfur-Containing Branched-Chain Volatiles
In this review’s survey of 175 published studies of the volatile profiles of various fruits only two sulfur-containing BCVs, 2-(2-methylpropyl)-thiazole and S-methyl 3-methylbutanethioate, were found (Table 1). Compared with the 125 other branched-chain compounds reported, this would seem to indicate that sulfur-containing BCVs are of little importance to the study of fruit volatile metabolism. Nevertheless, the detection of one of these compounds [2-(2-methylpropyl)-thiazole] in the economically significant tomato fruit at concentrations far above the minimum odor threshold (Baldwin et al., 2000; Tieman et al., 2012) as well as the importance of sulfur-containing volatile compounds in general to the flavor of numerous tropical fruits (Engel, 1999; Cannon and Ho, 2018) justifies a closer look at the biosynthesis of sulfur-containing BCVs.
The compound 2-(2-methylpropyl)-thiazole, hereafter referred to as 2-isobutylthiazole, is a known enhancer of tomato flavor that finds frequent use in the preparation of artificial condiments (Kazeniac and Hall, 1972; Christiansen et al., 2011). It has been detected in ripe tomato fruits by several studies (Baldwin et al., 2000; Tikunov et al., 2005; Tieman et al., 2012). Very little is known about the biosynthesis of this compound (Paolo et al., 2018). Hierarchically clustered metabolite data from several tomato introgressed lines characterized for volatile content seems to indicate a branched-chain amino acid origin for this compound (Mathieu et al., 2009). It is the precise nature of how this biosynthetic process occurs that remains totally unknown. That plants possess the capacity to biosynthesize the thiazole moiety is well known from studies examining plant biosynthesis of thiamine (Belanger et al., 1995; Goyer, 2010). However, from a biochemistry perspective it is difficult to see how this established pathway could incorporate a branched-chain amino acid. It is far more likely that the biosynthesis of this volatile occurs via a novel mechanism that uses a leucine molecule as a starting point and source for the thiazole’s nitrogen. Feeding 15N-labeled leucine to portions of tomato fruit tissue and examining any generated 2-isobutylthiazole molecules for that radioisotope could confirm or refute that assertion. Far more difficult would be accounting for the sulfur component of the thiazole ring and the two carbons at the four- and five-positions. While experiments using radiolabeled cysteine or methionine could yield some insight into the thiazole ring’s origin, ultimately it may be more feasible to use the abundant genetic resources available for tomato to track down potential biosynthesis enzymes using fine mapping of quantitative trait loci robustly associated with variable levels of this compound.
The other sulfur-containing BCV identified is the compound S-methyl 3-methylbutanethioate, isolated from cantaloupe (Beaulieu and Grimm, 2001). Several sulfur volatiles have been detected in the aroma profiles of ripe melons and are thought to contribute to that fruit’s characteristic aroma (Wyllie and Leach, 1992). Due to this, some investigation into the biosynthesis of sulfur volatiles in general in melon fruits has been conducted. Isotope feeding experiments conducted with 13C5-L-methionine gave evidence that volatile S-methyl thioesters are formed through methionine catabolism (Gonda et al., 2013). This study found that the enzyme L-methionine-γ–lyase (MGL) was capable of cleaving radiolabeled methanethiol from L-methionine and that S-methyl thioesters incorporated a radiolabeled carbon at the methyl group. As it has been shown in strawberry that alcohol acyltransferase can catalyze the formation of straight-chain thioesters by condensing thioalcohols with acyl-CoAs (Noichinda et al., 1999b), it may be likely that branched-chain S-methyl thioesters in fruit arise from AAT-mediated condensation of branched-chain acyl-CoAs with methanethiol derived from the MGL-catalyzed cleavage of methionine (Figure 4). Empirically demonstrating if this process occurs in plants by incubating branched-chain volatile ester forming AAT enzymes with branched-chain acyl-CoAs and thioalcohols would answer an important question regarding the metabolism of volatile branched-chain sulfur compounds.
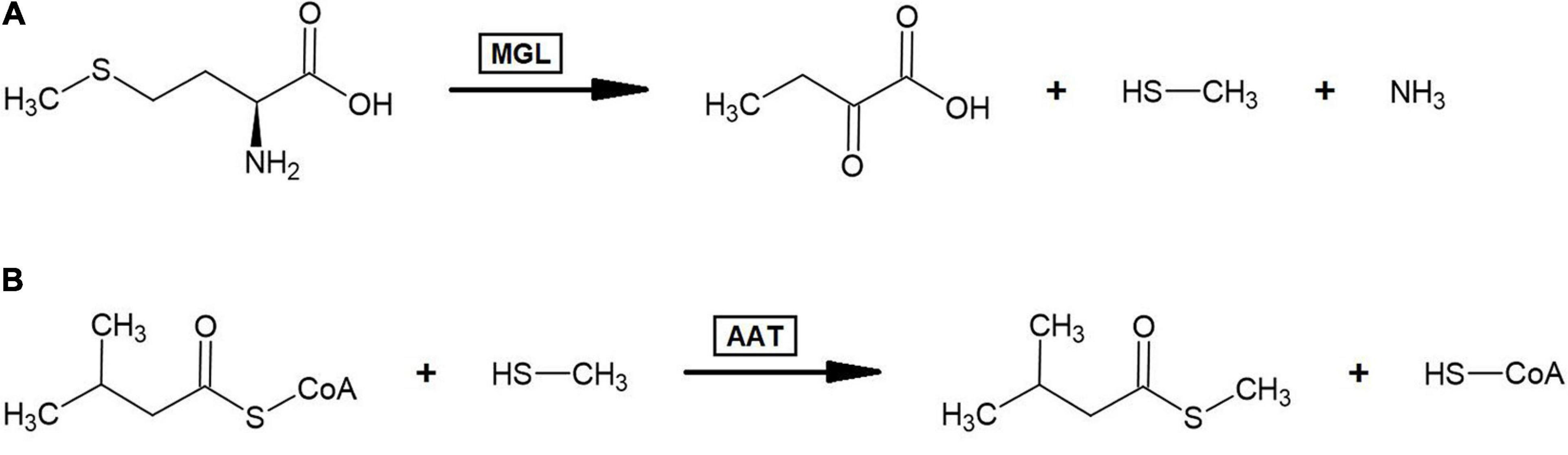
Figure 4. Proposed biosynthesis pathway for S-methyl branched-chain thioester volatiles. (A) Breakdown of L-methionine to α-ketobutanoate, methanethiol, and ammonia by the action of L-methionine-γ–lyase (MGL). (B) Formation of S-methyl branched-chain thioesters via alcohol acyltransferase (AAT) mediated condensation of methanethiol with branched-chain acyl-CoAs. This panel illustrates this process occurring with 3-methylbutyl-CoA and yielding S-methyl 3-methylbutanethioate since that was the only S-methyl branched-chain thioester volatile identified across the 175 fruit volatile studies examined in this review; however, this process could theoretically yield S-methyl 2-methylpropanethioate and S-methyl 2-methylbutanethioate from 2-methylpropyl-CoA and 2-methylbutyl-CoA, respectively.
What Biosynthetic Processes Are Capable of Forming Branched-Chain Alkanes in Fruit
Only one branched-chain alkane volatile was reported from all of the literature examined, the compound being 2-methylbutane and its presence being reported in raspberry (Aprea et al., 2015). Because the presence of this compound was reported below the level required for reliable quantification and because other studies of raspberry aroma failed to detect its presence, it is unlikely that that this compound plays a role in raspberry aroma. Nevertheless, as small to medium size branched alkanes in general are important components of commercial gasoline the biosynthesis of 2-methylbutane in fruit may be of interest to workers researching ways to bioengineer plants capable of producing fuel hydrocarbons. It has been shown that some plants can produce alkanes through the decarbonylation of aldehydes (Cheesbrough and Kolattukudy, 1984; Dennis and Kolattukudy, 1991; Aarts et al., 1995; Schneider-Belhaddad and Kolattukudy, 2000). While this route has been demonstrated to operate primarily on long- and very-long chain fatty acid derivatives in plants (Bernard et al., 2012; Ni et al., 2018), it may be a viable biochemical route to short branched-chain alkanes especially since branched-chain aldehydes are commonly found in several different kinds of plant (Supplementary Table 1). Further research with an emphasis on short branched-chain substrates is needed to confirm if such a pathway is indeed responsible for branched-chain alkane volatile biosynthesis in plants.
Conclusion
While knowledge regarding the biochemical basis of branched-chain volatile metabolism in fruits has advanced significantly in recent years, it is still rather inadequate especially when compared to our detailed understanding of oxylipin, terpenoid, and phenylpropanoid volatile biosynthesis. Much more is known regarding the impact BCVs have on the flavor and aroma qualities of several edible fruits, yet this underlies the importance of obtaining a more thorough understanding of the molecular correlates underlying fruit BCV production. What has been published regarding this topic points to four general hypotheses concerning the mechanisms of BCV volatile biosynthesis in fruits, any or all of which might be operational in vivo. It is clear that properly elucidating which metabolic processes are responsible for fruit BCV biosynthesis will require a great deal of further experimental work, and it may very well be that the precise mechanisms vary from fruit to fruit.
Several questions regarding BCV metabolism remain unresolved, particularly those concerning the biosynthesis of more unusual compounds such as branched-chain alkanes and S-methyl thioesters. Furthermore, the regulation of important parts of several proposed BCV biosynthesis routes remains little known in plants. Whether well-studied regulatory mechanisms known to control similar pathways in mammals are also active in plants is a particularly relevant question, especially if bypassing these mechanisms presents a potential way to modulate BCV content in fruit tissue. Information gained on this aspect of BCV metabolism could prove quite useful to groups researching ways to improve the flavor of certain fruits by manipulating levels of important volatile compounds.
Ultimately, advancing our understanding of BCV metabolism represents a way to further our knowledge of the molecular basis of fruit flavor and aroma. The fact that important progress has been made should not detract from the fact that significant gaps remain regarding our understanding of how specifically these compounds are generated in fruits. Filling these gaps through rigorous experimental work will go a long way to making branched-chain volatiles as well understood as their oxylipin, terpenoid, and phenylpropanoid counterparts.
Author Contributions
LB and PM conceived the idea to develop the manuscript. LB reviewed and wrote the original draft. DT and PM reviewed and helped to create the final version. All authors contributed to the article and approved the submitted version.
Funding
This research was funded by the Blueberry Breeding Program at University of Florida.
Conflict of Interest
The authors declare that the research was conducted in the absence of any commercial or financial relationships that could be construed as a potential conflict of interest.
Publisher’s Note
All claims expressed in this article are solely those of the authors and do not necessarily represent those of their affiliated organizations, or those of the publisher, the editors and the reviewers. Any product that may be evaluated in this article, or claim that may be made by its manufacturer, is not guaranteed or endorsed by the publisher.
Supplementary Material
The Supplementary Material for this article can be found online at: https://www.frontiersin.org/articles/10.3389/fpls.2021.814138/full#supplementary-material
References
Aarts, M. G., Keijzer, C. J., Stiekema, W. J., and Pereira, A. (1995). Molecular characterization of the Cer1 gene of arabidopsis involved in epicuticular wax biosynthesis and pollen fertility. Plant Cell 7, 2115–2127. doi: 10.1105/tpc.7.12.2115
Abbas, F., Ke, Y., Yu, R., Yue, Y., Amanullah, S., Jahangir, M. M., et al. (2017). Volatile terpenoids: multiple functions, biosynthesis, modulation and manipulation by genetic engineering. Planta 246, 803–816. doi: 10.1007/s00425-017-2749-x
Ağalar, H. G., Demirci, B., and Başer, K. H. C. (2014). The Volatile Compounds of Elderberries (Sambucus nigra L.). Nat. Vol. Essent. Oils 1, 51–54.
Alves, G. L., and Franco, M. R. B. (2003). Headspace gas chromatography-mass spectrometry of volatile compounds in murici (Byrsonima crassifolia l. Rich) J. Chromatogr. A. 985, 297–301. doi: 10.1016/S0021-9673(02)01398-5
Amanpour, A., Guclu, G., Kelebek, H., and Selli, S. (2019). Characterization of key aroma compounds in fresh and roasted terebinth fruits using aroma extract dilution analysis and GC–MS-Olfactometry. Microchem. J. 145, 96–104. doi: 10.1016/j.microc.2018.10.024
Ameye, M., Allmann, S., Verwaeren, J., Smagghe, G., Haesaert, G., Schuurink, R. C., et al. (2018). Green leaf volatile production by plants: a meta-analysis. New Phytol. 220, 666–683. doi: 10.1111/nph.14671
Amira, E. A., Guido, F., Behija, S. E., Manel, I., Nesrine, Z., Ali, F., et al. (2011). Chemical and aroma volatile compositions of date palm (Phoenix dactylifera L.) fruits at three maturation stages. Food Chem. 127, 1744–1754. doi: 10.1016/j.foodchem.2011.02.051
Aprea, E., Biasioli, F., and Gasperi, F. (2015). Volatile Compounds of Raspberry Fruit: From Analytical Methods to Biological Role and Sensory Impact. Molecules 20, 2445–2474. doi: 10.3390/molecules20022445
Arena, E., Campisi, S., Fallico, B., Lanza, M. C., and Maccarone, E. (2001). Aroma value of volatile compounds of prickly pear (Opuntia ficus indica (L.) Mill. Cactaceae). Ital. J. Food Sci. 13, 311–319.
Aubert, C., and Bourger, N. (2004). Investigation of Volatiles in Charentais Cantaloupe Melons (Cucumis melo Var. cantalupensis). Characterization of Aroma Constituents in Some Cultivars. J. Agric. Food Chem. 52, 4522–4528. doi: 10.1021/jf049777s
Augusto, F., Valente, A. L. P., dos Santos, Tada, E., and Ruvellino, S. R. (2000). Screening of Brazilian fruit aromas using solid-phase microextraction–gas chromatography–mass spectrometry. J. Chromatogr. A. 873, 117–127. doi: 10.1016/S0021-9673(99)01282-0
Baldwin, E. A., Scott, J. W., Shewmaker, C. K., and Schuch, W. (2000). Flavor trivia and tomato aroma: biochemistry and possible mechanisms for control of important aroma components. HortScience 35, 1013–1022. doi: 10.21273/HORTSCI.35.6.1013
Barrios Guio, J. C., Sinuco Leon, D. C., and Morales Perez, A. L. (2010). Compuestos volátiles libres y enlazados glicosídicamente en la pulpa de la uva Caimarona (Pourouma cecropiifolia Mart.). Acta Amaz. 40, 189–198. doi: 10.1590/S0044-59672010000100024
Beaulieu, J. C., and Grimm, C. C. (2001). Identification of Volatile Compounds in Cantaloupe at Various Developmental Stages Using Solid Phase Microextraction. J. Agric. Food Chem. 49, 1345–1352. doi: 10.1021/jf0005768
Beekwilder, J., Alvarez-Huerta, M., Neef, E., Verstappen, F. W. A., Bouwmeester, H. J., and Aharoni, A. (2004). Functional characterization of enzymes forming volatile esters from strawberry and banana. Plant Physiol. 135, 1865–1878. doi: 10.1104/pp.104.042580
Belanger, F. C., Leustek, T., Chu, B. Y., and Kriz, A. L. (1995). Evidence for the thiamine biosynthetic pathway in higher-plant plastids and its developmental regulation. Plant Mol. Biol. 29, 809–821. doi: 10.1007/bf00041170
Belgis, M. B., Wijaya, C. H., Apriyantono, A., Kusbiantoro, B., and Yuliana, N. D. (2017). Volatile and aroma characterization of several lai (Durio kutejensis) and durian (Durio zibenthinus) cultivars grown in Indonesia. Sci Hortic. 220, 291–298. doi: 10.1016/j.scienta.2017.03.041
Belo, R. F. C., Augusti, R., Lopes, P. S. N., and Junqueira, R. G. (2013). Characterization and classifcation of pequi trees (Caryocar brasiliense Camb.) based on the profle of volatile constituents using headspace solid-phase microextraction - gas chromatography - mass spectrometry and multivariate analysis. Food Sci. Technol. 33, 116–124. doi: 10.1590/S0101-20612013000500018
Bernard, A., Domerguem, F., Pascal, S., Jetter, R., Renne, C., Faure, J. D., et al. (2012). Reconstitution of plant alkane biosynthesis in yeast demonstrates that Arabidopsis ECERIFERSUM1 and ECERIFERUM3 are core components of a very-long-chain alkane synthesis complex. Plant Cell 24, 3106–3118. doi: 10.1105/tpc.112.099796
Besada, C., Sanchez, G., Gil, R., Granell, A., and Salvador, A. (2017). Volatile metabolite profiling reveals the changes in the volatile compounds of new spontaneously generated loquat cultivars. Food Res. Int. 100, 234–243. doi: 10.1016/j.foodres.2017.06.068
Binder, S. (2010). Branched-chain amino acid metabolism in Arabidopsis thaliana. Arabidopsis Book 8:e0137. doi: 10.1199/tab.0137
Binder, S., Knill, T., and Schuster, J. (2007). Branched-chain amino acid metabolism in higher plants. Physiol. Plant. 129, 68–78. doi: 10.1111/j.1399-3054.2006.00800.x
Boatright, J., Negre, F., Chen, X. L., Kish, C. M., Wood, B., Peel, G., et al. (2004). Understanding in vivo benzenoid metabolism in petunia petal tissue. Plant Physiol. 135, 1993–2011. doi: 10.1104/pp.104.045468
Borges, E. C., and Rezende, C. M. (2000). Main aroma constituents of genipap (Genipa americana) and bacuri (Platonia sculenta). J. Essential Oil Res. 12, 71–74. doi: 10.1080/10412905.2000.9712046
Boudhrioua, N., Giampaoli, P., and Bonazzi, C. (2003). Changes in aromatic components of banana during ripening and air-drying. LWT 36, 633–642. doi: 10.1016/S0023-6438(03)00083-5
Boulanger, R., and Crouzet, J. (2000). Free and bound flavour components of Amazonian fruits: 2. cupuaçu volatile compounds. Flavour Fragr. J. 15, 251–257. doi: 10.1002/1099-1026(200007/08)15:4<251::AID-FFJ905<3.0.CO;2-2
Boulanger, R., Chassagne, D., and Crouzet, J. (1999). Free and bound flavour components of amazonian fruits. 1: Bacuri. Flavour Fragr. J. 14, 303–311. doi: 10.1002/(SICI)1099-1026(199909/10)14:5<303::AID-FFJ834<3.0.CO;2-C
Brocker, C., Vasiliou, M., Carpenter, S., Carpenter, C., Zhang, Y., Wang, X., et al. (2013). Aldehyde dehydrogenase (ALDH) superfamily in plants: gene nomenclature and comparative genomics. Planta 237, 189–210. doi: 10.1007/s00425-012-1749-0
Cannon, R. J., and Ho, C. (2018). Volatile Sulfur Compounds in Tropical Fruits. J. Food Drug Anal. 26, 445–468. doi: 10.1016/j.jfda.2018.01.014
Carasek, E., and Pawliszyn, J. (2006). Screening of Tropical Fruit Volatile Compounds Using Solid-Phase Microextraction (SPME) Fibers and Internally Cooled SPME Fiber. J. Agric. Food Chem. 54, 8688–8696. doi: 10.1021/jf0613942
Ceva-Antunes, P. M. N., Bizzo, H. R., Alves, S. M., and Antunes, O. A. C. (2003). Analysis of volatile compounds of Taperebá (Spondias mombin L.) and Cajá (Spondias mombin L.) by simultaneous distillation and extraction (SDE) and solid phase microextraction (SPME). J. Agric. Food Chem. 51, 1387–1392. doi: 10.1021/jf025873m
Ceva-Antunes, P. M. N., Bizzo, H. R., Silva, A. S., Carvalho, C. P. S., and Antunes, O. A. C. (2006). Analysis of Volatile Composition of Siriguela (Spondias Purpurea L.) By Solid Phase Microextraction (SPME). LWT-Food Sci. Technol. 39, 436–442. doi: 10.1016/j.lwt.2005.02.007
Chai, Q., Liu, W., Wang, L., Yang, C., Wang, Y., Fang, J., et al. (2012). Volatiles of plums evaluated by HS-SPME with GC–MS at the germplasm level. Food Chem. 130, 432–440. doi: 10.1016/j.foodchem.2011.05.127
Cheesbrough, T. M., and Kolattukudy, P. E. (1984). Alkane biosynthesis by decarbonylation of aldehydes catalyzed by a particulate preparation from Pisum sativum. Proc. Natl. Acad. Sci. U.S.A. 81, 6613–6617. doi: 10.1073/pnas.81.21.6613
Chen, L., Zhang, X., Jin, Q., Yang, L., Li, J., and Chen, F. (2015). Free and Bound Volatile Chemicals in Mulberry (Morus atropurpurea Roxb.). J. Food Sci. 80, C975–C982. doi: 10.1111/1750-3841.12840
Chen, Q. Q., Song, J. X., Bi, J. F., Meng, X. J., and Wu, X. Y. (2018). Characterization of volatile profile from ten different varieties of Chinese jujubes by HS-SPME/GC–MS coupled with E-nose. Food Res. Int. 105, 605–615. doi: 10.1016/j.foodres.2017.11.054
Chen, X., Quek, S. W., Fedrizzi, B., and Kilmartin, P. A. (2020). Characterization of free and glycosidically bound volatile compounds from tamarillo (Solanum betaceum Cav.) with considerations on hydrolysis strategies and incubation time. LWT 124:109178. doi: 10.1016/j.lwt.2020.109178
Cheng, A.-X., Lou, Y.-G., Mao, Y.-B., Lu, S., Wang, L.-J., and Chen, X.-Y. (2007). Plant terpenoids: biosynthesis and ecological functions. J. Integr. Plant Biol. 49, 179–186. doi: 10.1111/j.1744-7909.2007.00395.x
Cheng, H., Chen, J., Li, X., Pan, J., Xue, S. J., Liu, D., et al. (2015). Differentiation of the volatile profiles of Chinese bayberry cultivars during storage by HS-SPME–GC/MS combined with principal component analysis. Postharvest Biol. Technol. 100, 59–72. doi: 10.1016/j.postharvbio.2014.09.003
Cheng, K., Peng, B., and Yuan, F. (2020). Volatile composition of eight blueberry cultivars and their relationship with sensory attributes. Flavour Fragr. J. 35, 443–453. doi: 10.1002/ffj.3583
Cheong, K. W., Tan, C. P., Mirhosseini, H., Hamid, N. S. A., Osman, A., and Basri, M. (2010). Equilibrium headspace analysis of volatile flavour compounds extracted from soursop (Annona muricata) using solid phase microextraction. Food Res. Int. 43, 1267–1276. doi: 10.1016/j.foodres.2010.03.001
Choi, J. Y., Lee, S. M., Lee, H. J., and Kim, Y.-S. (2018). Characterization of aroma-active compounds in Chinese quince (Pseudocydonia sinensis Schneid) by aroma dilution analyses. Food Res. Int. 105, 828–835. doi: 10.1016/j.foodres.2017.12.015
Christiansen, K. F., Olsen, E., Vegarud, G., Langsrud, T., Lea, P., Haugen, J. E., et al. (2011). Flavor release of the tomato flavor enhancer, 2-isobutylthiazole, from whey protein stabilized model dressings. Food Sci. Technol. Int. 17, 143–154. doi: 10.1177/1082013210381935
Conde-Martínez, N., Sinuco, D. C., and Osorio, C. (2014). Chemical studies on curuba (Passiflora mollissima (Kunth) L. H. Bailey) fruit flavour. Food Chem. 15, 356–363. doi: 10.1016/j.foodchem.2014.02.056
Corpas, E. J., Taborda, G., Tapasco, O. A., and Ortiz, A. (2016). Comparison between extraction methods to obtain volátiles from lulo (Solatium quitoense) pulp. Rev. Colomb. Quim. 45, 12–21. doi: 10.15446/rev.colomb.quim.v45n3.61359
Cozzolino, R., De Giulio, B., Petriccione, M., Martignetti, A., Malorni, L., Zampella, L., et al. (2020). Comparative analysis of volatile metabolites, quality and sensory attributes of Actinidia Chinensis fruit. Food Chem. 316:126340. doi: 10.1016/j.foodchem.2020.126340
da Rocha, R. F. J., da Silva, Araújo, I. M., de Freitas, S. M., dos Santos, and Garruti, D. (2017). Optimization of headspace solid phase micro-extraction of volatile compounds from papaya fruit assisted by GC–olfactometry. J. Food Sci. Technol. 54, 4042–4050. doi: 10.1007/s13197-017-2871-6
da Silva, A. P. G., Spricigo, P. C., Purgatto, E., de Alencar, S. M., and Jacomino, A. P. (2019a). Volatile Compounds Determined by SPME-GC, Bioactive Compounds, In Vitro Antioxidant Capacity and Physicochemical Characteristics of Four Native Fruits from South America. Plant Foods Hum. Nutr. 74, 358–363. doi: 10.1007/s11130-019-00745-7
da Silva, A. P. G., Spricigo, P. C., Purgatto, E., de Alencar, S. M., Sartori, A. P., and Jacomino, A. P. (2019b). Chemical composition, nutritional value and bioactive compounds in six uvaia accessions. Food Chem. 294, 547–556. doi: 10.1016/j.foodchem.2019.04.121
D’Agostino, M. F., Sanz, J., Sanz, M. L., Giuffrè, A. M., Sicari, V., and Soria, A. C. (2015). Optimization of a Solid-Phase Microextraction method for the Gas Chromatography–Mass Spectrometry analysis of blackberry (Rubus ulmifolius Schott) fruit volatiles. Food Chem. 178, 10–17. doi: 10.1016/j.foodchem.2015.01.010
de Araújo, F. F., de Paulo Farias, D., Neri-Numa, I. A., Dias-Audibert, F. L., Delafiori, J., de Souza, F. G., et al. (2021). Chemical characterization of Eugenia stipitata: A native fruit from the Amazon rich in nutrients and source of bioactive compounds. Food Res. Int. 139:109904. doi: 10.1016/j.foodres.2020.109904
de Santana, K. L., de Sousa Galvão, M., de Jesus, M. S., Nogueira, J. P., and Narain, N. (2017). HS-SPME optimization and extraction of volatile compounds from soursop (Annona muricata L.) pulp with emphasis on their characteristic impact compounds. Ciênc. Tecnol. Aliment. Campinas 37, 250–260. doi: 10.1590/1678-457X.20916
de Sousa Galvão, M., Narain, N., Madruga, M. S., and Santos, M. S. P. (2010). Volatile compounds in Umbu (Spondias tuberosa Arruda Câmara) fruits during maturation. Acta Hortic. 864, 509–517. doi: 10.17660/ActaHortic.2010.864.68
de Souza, M. P., Bataglion, G. A., da Silva, F. M. A., de Almeida, R. A., Paz, W. H. P., Nobre, T. A., et al. (2016). Phenolic and aroma compositions of pitomba fruit (Talisia esculenta Radlk.) assessed by LC–MS/MS and HS-SPME/GC–MS. Food Res. Int. 83, 87–94. doi: 10.1016/j.foodres.2016.01.031
Dennis, M. W., and Kolattukudy, P. E. (1991). Alkane biosynthesis by decarbonylation of aldehyde catalyzed by a microsomal preparation from Botryococcus braunii. Arch. Biochem. Biophys. 287, 268–275. doi: 10.1016/0003-9861(91)90478-2
Dixon, J., and Hewett, E. W. (2000). Factors affecting apple aroma/flavour volatile concentration: A Review. N. Z. J. Crop Hortic. Sci. 28, 155–173. doi: 10.1080/01140671.2000.9514136
Dong, J., Zhang, Y., Tang, X., Jin, W., and Han, Z. (2013). Differences in volatile ester composition between Fragaria × ananassa and F. vesca and implications for strawberry aroma patterns. Sci. Hortic. 150, 47–53. doi: 10.1016/j.scienta.2012.11.001
dos Santos, da Silva, M., Alves-Santos, A. M., dos Santos, I. D., Wagner, R., Naves, M. M. V., et al. (2020). A new population of pequi (Caryocar spp.) developed by Brazilian indigenous people has agro-industrial and nutraceutical advantages. Eur. Food Res. Technol. 246, 1715–1724. doi: 10.1007/s00217-020-03525-9
Du, X., Finn, C. E., and Qian, M. C. (2010). Volatile composition and odour-activity value of thornless ‘Black Diamond’ and ‘Marion’ blackberries. Food Chem. 119, 1127–1134. doi: 10.1016/j.foodchem.2009.08.024
Du, X., Plotto, A., Song, M., Olmstead, J., and Rouseff, R. (2011). Volatile Composition of Four Southern Highbush Blueberry Cultivars and Effect of Growing Location and Harvest Date. J. Agric. Food Chem. 59, 8347–8357. doi: 10.1021/jf201184m
Dudareva, N., Klempien, A., Muhlemann, J. K., and Kaplan, I. (2013). Biosynthesis, function and metabolic engineering of plant volatile organic compounds. New Phytol. 198, 16–32. doi: 10.1111/nph.12145
Dudareva, N., Negre, F., Nagegowda, D. A., and Orlova, I. (2006). Plant volatiles: recent advances and future perspectives. Crit. Rev. Plant Sci. 25, 417–440. doi: 10.1080/07352680600899973
El Hadi, M. A., Zhang, F. J., Wu, F. F., Zhou, C. H., and Tao, J. (2013). Advances in fruit aroma volatile research. Molecules 18, 8200–8229. doi: 10.3390/molecules18078200
Elizalde-González, M. P., and Segura-Rivera, E. J. (2018). Volatile compounds in different parts of the fruit Psidium guajava L. cv. “Media China” identified at distinct phenological stages using HS-SPME-GC-QTOF/MS. Phytochem. Anal. 29, 649–660. doi: 10.1002/pca.2778
El-Sharkawy, I., Manriquez, D., Flores, F. B., Regad, F., Bouzayen, M., Latché, A., et al. (2005). Functional characterization of a melon alcohol acyl-transferase gene family involved in the biosynthesis of ester volatiles. Identification of the crucial role of a threonine residue for enzyme activity. Plant Mol. Biol. 59, 345–362. doi: 10.1007/s11103-005-8884-y
Engel, K. H. (1999). “The Importance of Sulfur-Containing Compounds to Fruit Flavors,” in Flavor Chemistry, eds R. Teranishi, E. L. Wick, and I. Hornstein (Boston, MA: Springer), 265–273. doi: 10.1007/978-1-4615-4693-1_23
Fang, H., Chen, J., Tian, Y., Liu, Y., Li, H., and Cheng, H. (2020). Chemometrics characterization of volatile changes in processed bayberry juice versus intact fruit during storage by headspace solid-phase micro-extraction combined with GC-MS. J. Food Process. Preserv. 44:e14444. doi: 10.1111/jfpp.14444
Faria, J. V., Valido, V. H., Weider, H. P., Paz, da Silva, F. M. A., de Souza, A. D. L., et al. (2021). Comparative evaluation of chemical composition and biological activities of tropical fruits consumed in Manaus, central Amazonia, Brazil. Food Res. Int. 139:109836. doi: 10.1016/j.foodres.2020.109836
Farneti, B., Khomenko, I., Grisenti, M., Ajelli, M., and Betta, E. (2017). Exploring Blueberry Aroma Complexity by Chromatographic and Direct-Injection Spectrometric Techniques. Front. Plant Sci. 8:617. doi: 10.3389/fpls.2017.00617
Feng, S., Huang, M. Y., Crane, J. H., and Wang, Y. (2018). Characterization of key aroma-active compounds in lychee (Litchi chinensis Sonn.). J. Food Drug Anal. 26, 497–503. doi: 10.1016/j.jfda.2017.07.013
Ferreira, D. D. F., Garruti, D. S., Barin, J. S., Cichoski, A. J., and Wagner, R. (2016). Characterization of odor-active compounds in gabiroba fruits (Campomanesia xanthocarpa O. Berg). J. Food Qual. 39, 90–97. doi: 10.3390/metabo10050197
Ferreira, L., Perestrelo, R., and Câmara, J. S. (2009). Comparative analysis of the volatile fraction from Annona cherimola Mill. cultivars by solid-phase microextraction and gas chromatography–quadrupole mass spectrometry detection. Talanta 77, 1087–1096. doi: 10.1016/j.talanta.2008.08.011
Fraga, S. R. G., and Rezende, C. M. (2001). The Aroma of Brazilian Ambarella Fruit (Spondias cytherea Sonnerat). J. Essent. Oil Res. 13, 252–255. doi: 10.1080/10412905.2001.9699686
Franco, M. R. B., and Shibamoto, T. (2000). Volatile Composition of Some Brazilian Fruits: Umbu-caja (Spondias citherea), Camu-camu (Myrciaria dubia), Araça-boi (Eugenia stipitata), and Cupuaçu (Theobroma grandiflorum). J. Agric. Food Chem. 48, 1263–1265. doi: 10.1021/jf9900074
Fraternale, D., Ricci, D., Flamini, G., and Giomaro, G. (2011). Volatiles Profile of Red Apple from Marche Region (Italy). Rec. Nat. Prod. 5, 202–207.
Fredes, A., Sales, C., Barreda, M., Valcárcel, M., Roselló, S., and Beltrán, J. (2016). Quantification of prominent volatile compounds responsible for muskmelon and watermelon aroma by purge and trap extraction followed by gas chromatography–mass spectrometry determination. Food Chem. 190, 689–700. doi: 10.1016/j.foodchem.2015.06.011
Freitas, T. P., Taver, I. B., Spricigo, P. C., do Amaral, L. B., Purgatto, E., and Jacomino, A. P. (2020). Volatile Compounds and Physicochemical Quality of Four Jabuticabas (Plinia sp.). Molecules 25:4543. doi: 10.3390/molecules25194543
Fujiki, Y., Ito, M., Nishida, I., and Watanabe, A. (2001). Leucine and its keto acid enhance the coordinated expression of genes for branched-chain amino acid catabolism in Arabidopsis under sugar starvation. FEBS Lett. 499, 161–165. doi: 10.1016/s0014-5793(01)02536-4
Galili, G., Amir, R., and Fernie, A. R. (2016). The regulation of essential amino acid synthesis and accumulation in plants. Annu. Rev. Plant Biol. 67, 153–178. doi: 10.1105/tpc.17.00186
Gilbert, J. L., Guthart, M. J., Gezan, S. A., de Carvalho, M. P., Schwieterman, M. L., Colquhoun, T. A., et al. (2015). Identifying Breeding Priorities for Blueberry Flavor Using Biochemical, Sensory, and Genotype by Environment Analyses. PLoS One 10:e0138494. doi: 10.1371/journal.pone.0138494
Goff, S. A., and Klee, H. J. (2006). Plant volatile compounds: sensory cues for health and nutritional value? Science 311, 815–819. doi: 10.1126/science.1112614
Gonda, I., Bar, E., Portnoy, V., Lev, S., Burger, J., Schaffer, A. A., et al. (2010). Branched-chain and aromatic amino acid catabolism into aroma volatiles in Cucumis melo L. fruit. J. Exp. Bot. 61, 1111–1123. doi: 10.1093/jxb/erp390
Gonda, I., Lev, S., Bar, E., Sikron, N., Portnoy, V., Davidovich-Rikanati, R., et al. (2013). Catabolism of L-methionine in the formation of sulfur and other volatiles in melon (Cucumis melo L.) fruit. Plant J. 74, 458–472. doi: 10.1111/tpj.12149
Goulet, C., Kamiyoshihara, Y., Lam, N. B., Richard, T., Taylor, M. G., Tieman, D. M., et al. (2015). Divergence in the enzymatic activities of a Tomato and Solanum pennellii alcohol acyltransferase impacts fruit volatile ester composition. Mol. Plant 8, 153–162. doi: 10.1016/j.molp.2014.11.007
Goulet, C., Mageroy, M. H., Lam, N. B., Floystad, A., Tieman, D. M., and Klee, H. J. (2012). Role of an esterase in flavor volatile variation within the tomato clade. Proc. Natl. Acad. Sci. U. S. A. 109, 19009–19014. doi: 10.1073/pnas.1216515109
Goyer, A. (2010). Thiamine in plants: aspects of its metabolism and functions. Phytochemistry 71, 1615–1624. doi: 10.1016/j.phytochem.2010.06.022
Graham, I. A., and Eastmond, P. J. (2002). Pathways of straight and branched chain fatty acid catabolism in higher plants. Prog. Lipid Res. 41, 156–181. doi: 10.1016/S0163-7827(01)00022-4
Grande-Tovar, D. C., Johannes, D.-G., Puerta, L. F., Rodríguez, G. C., Sacchetti, G., Paparella, A., et al. (2019). Bioactive micro-constituents of ackee arilli (Blighia sapida K.D. Koenig). An. Acad. Bras. Ciênc. 91, 1–15. doi: 10.1590/0001-3765201920180140
Griffiths, G. (2015). Biosynthesis and analysis of plant oxylipins. Free Radic. Res. 49, 565–582. doi: 10.3109/10715762.2014.1000318
Grimm, J. E., and Steinhaus, M. (2019). Characterization of the Major Odor-Active Compounds in Jackfruit Pulp. J. Agric. Food Chem. 67, 5838–5846. doi: 10.1021/acs.jafc.9b01445
Grimm, J. E., and Steinhaus, M. (2020). Characterization of the Major Odorants in Cempedak—Differences to Jackfruit. J. Agric. Food Chem. 68, 258–266. doi: 10.1021/acs.jafc.9b06564
Güler, Z., and Gül, E. (2017). Volatile organic compounds in the aril juices and seeds from selected five pomegranate (Punica granatum L.) cultivars. Int. J. Food Prop. 20, 281–293. doi: 10.1080/10942912.2016.1155057
Günther, C. S., Chervin, C., Marsh, K. B., Newcomb, R. D., and Souleyre, E. J. F. (2011). Characterisation of two alcohol acyltransferases from kiwifruit (Actinidia spp.) reveals distinct substrate preferences. Phytochemistry 72, 700–710. doi: 10.1016/j.phytochem.2011.02.026
Hansen, K., and Poll, L. (1993). Conversion of L-isoleucine into 2-methylbut-2-enyl esters in apples. LWT. 26, 178–180. doi: 10.1006/fstl.1993.1036
Harb, J., Bisharat, R., and Streif, J. (2008). Changes in volatile constituents of blackcurrants (Ribes nigrum L. cv. ‘Titania’) following controlled atmosphere storage. Postharvest Biol. Technol. 47, 271–279. doi: 10.1016/j.postharvbio.2007.08.007
Harris, R. A., Hawes, J. W., Popov, K. M., Zhao, Y., Shimomura, Y., Sato, J., et al. (1997). Studies on the regulation of the mitochondrial α-ketoacid dehydrogenase complexes and their kinases. Adv. Enzyme Regul. 37, 271–293. doi: 10.1016/s0065-2571(96)00009-x
Harris, R. A., Paxton, R., Powell, S. M., Goodwin, G. W., Kuntz, M. J., and Han, A. C. (1986). Regulation of branched-chain alpha-ketoacid dehydrogenase complex by covalent modification. Adv. Enz. Reg. 25, 219–237. doi: 10.1016/0065-2571(86)90016-6
Hayaloglu, A. A., and Demir, N. (2016). Phenolic Compounds, Volatiles, and Sensory Characteristics of Twelve Sweet Cherry (Prunus avium L.) Cultivars Grown in Turkey. J. Food Sci. 81, C7–C18. doi: 10.1111/1750-3841.13175
Hildebrandt, T. M., Nunes Nesi, A., Araújo, W. L., and Braun, H.-P. (2015). Amino acid catabolism in plants. Mol. Plant 8, 1563–1579. doi: 10.1016/j.molp.2015.09.005
Hou, Y., and Wu, G. (2018). Nutritionally Essential Amino Acids. Adv. Nutr. 9, 849–851. doi: 10.1093/advances/nmy054
Irmisch, S., McCormick, A. C., Boeckler, G. A., Schmidt, A., Reichelt, M., Schneider, B., et al. (2013). Two herbivore-induced cytochrome P450 Enzymes CYP79D6 and CYP79D7 catalyze the formation of volatile aldoximes involved in poplar defense. Plant Cell 25, 4737–4754. doi: 10.1105/tpc.113.118265
Irmisch, S., McCormick, A. C., Günther, J., Schmidt, A., Boeckler, G. A., Gershenzon, J., et al. (2014). Herbivore-induced poplar cytochrome P450 enzymes of the CYP71 family convert aldoximes to nitriles which repel a generalist caterpillar. Plant J. 80, 1095–1107. doi: 10.1111/tpj.12711
Irwandi, J., Che Man, Y. B., Selamat, J., Ahmad, F., and Sugisawa, H. (2008). Retention of volatile components of durian fruit leather during processing and storage. J. Food Process. Pres. 32, 740–750. doi: 10.1111/j.1745-4549.2008.00211.x
Iwaoka, W., Hagi, Y., Umano, K., and Shibamoto, T. (1994). Volatile Chemicals Identified in Fresh and Cooked Breadfruit. J. Agric. Food Chem. 42, 975–976. doi: 10.1021/jf00040a026
Janzantti, N. S., and Monteiro, M. (2017). HS–GC–MS–O analysis and sensory acceptance of passion fruit during maturation. J. Food Sci. Technol. 54, 2594–2601. doi: 10.1007/s13197-017-2671-z
Jayarathna, P. L. I., Jayawardena, J. A. E. C., and Vanniarachchy, M. P. G. (2020). Identification of Physical, Chemical Properties and Flavor Profile of Spondias dulcis in Three Maturity Stages. Int. J. Adv. Res. Sci. Eng. 5, 208–211.
Joulain, D., Casazza, A., Laurent, R., Portier, D., Guillamon, N., Pandya, R., et al. (2004). Volatile Flavor Constituents of Fruits from Southern Africa: Mobola Plum (Parinari curatellifolia). J. Agric. Food Chem. 52, 2322–2325. doi: 10.1021/jf030702i
Jung, K. (2018). Analysis and sensory evaluation of volatile constituents of blackcurrant (Ribes nigrum L.) and redcurrant (Ribes rubrum L.) fruits. [PhD dissertation]. Munich: Technical University of Munich.
Kamatou, G. P. P., Viljoen, A. M., Õzek, T., and Başer, K. H. C. (2008). Head-space volatiles of Gethyllis afra and G. ciliaris fruits (“kukumakranka”). S. Afr. J. Bot. 74, 768–770. doi: 10.1016/j.sajb.2008.07.002
Kang, W., Li, Y., Xu, Y., Jiang, W., and Tao, Y. (2012). Characterization of aroma compounds in Chinese bayberry (Myrica rubra Sieb. et Zucc.) by Gas Chromatography Mass Spectrometry (GC-MS) and Olfactometry (GC-O). J. Food Sci. 77, C1030–C1035. doi: 10.1111/j.1750-3841.2012.02747.x
Kaur, N., Reumann, S., and Hu, J. (2009). Peroxisome biogenesis and function. Arabidopsis Book 7:e0123. doi: 10.1199/tab.0123
Kazeniac, S. J., and Hall, R. M. (1972). 2-Alkylthiazoles as Tomato Product Enhancers. U.S. Patent No 3,660,112. Washington, DC: U.S. Patent and Trademark Office.
Kochevenko, A., Araujo, W. L., Maloney, G. S., Tieman, D. M., Do, P. T., and Taylor, M. G. (2012). Catabolism of branched chain amino acids supports respiration but not volatile synthesis in tomato fruits. Mol. Plant. 5, 366–375. doi: 10.1093/mp/ssr108
Končitíková, R., Vigouroux, A., Kopečná, M., Andree, T., Bartoš, J., and Šebela, M. (2015). Role and structural characterization of plant aldehyde dehydrogenases from family 2 and family 7. Biochem. J. 468, 109–123. doi: 10.3390/genes12010051
Kozioł, M. J., and Macía, M. J. (1998). Chemical composition, nutritional evaluation, and economic prospects of Spondias purpurea (Anacardiaceae). Econ. Botany 52, 373–380. doi: 10.1007/BF02862067
Kraujalytë, V., Leitner, E., and Venskutonis, P. R. (2013). Characterization of Aronia melanocarpa Volatiles by Headspace-Solid-Phase Microextraction (HS-SPME), Simultaneous Distillation/Extraction (SDE), and Gas Chromatography-Olfactometry (GC-O) Methods. J. Agric. Food Chem. 61, 4728–4736. doi: 10.1021/jf400152x
Lackus, N. D., Schmidt, A., Gershenzon, J., and Köllner, T. G. (2021). A peroxisomal β-oxidative pathway contributes to the formation of C6–C1 aromatic volatiles in poplar. Plant Physiol. 186, 891–909. doi: 10.1093/plphys/kiab111
Lasekan, O., Khatib, A., Juhari, H., Patiram, P., and Lasekan, A. (2013). Headspace solid-phase microextraction gas chromatography-mass spectrometry determination of volatile compounds in different varieties of African star apple fruit (Chrysophillum albidum). Food Chem. 141, 2089–2097. doi: 10.1016/j.foodchem.2013.05.081
Leffingwell, J. C., Alford, E. D., and Leffingwell, D. (2015). Identification of the Volatile Constituents of Raw Pumpkin (Cucurbita pepo L.) by Dynamic Headspace Analyses. Leffingwell Rep. 7, 1–14.
Legua, P., Domenech, A., Martínez, J. J., Sánchez-Rodríguez, L., Hernández, F., Carbonell-Barrachina, A. A., et al. (2017). Bioactive and Volatile Compounds in Sweet Cherry Cultivars. J. Food Nutr. Res. 5, 844–851. doi: 10.12691/jfnr-5-11-8
Lei, Y., Xie, S., Chen, H., Guan, X., and Zhang, Z. (2019). Behavior of 3-isobutyl-2-methoxypyrazine biosynthesis related to proposed precursor and intermediate in wine grape. Food Chem. 277, 609–616. doi: 10.1016/j.foodchem.2018.10.121
Leite Neta, M. T. S., de Jesus, M. S., da Silva, J. L. A., Araujo, H. C. S., Sandes, R. D. D., Shanmugam, S., et al. (2019). Effect of spray drying on bioactive and volatile compounds in soursop (Annona muricata) fruit pulp. Food Res. Int. 124, 70–77. doi: 10.1016/j.foodres.2018.09.039
Li, C., Xin, M., Li, L., He, X., Yi, P., Tang, Y., et al. (2021). Characterization of the aromatic profile of purple passion fruit (Passiflora edulis Sims) during ripening by HS-SPME-GC/MS and RNA sequencing. Food Chem. 355:129685. doi: 10.1016/j.foodchem.2021.129685
Li, J.-X., Schieberle, P., and Steinhaus, M. (2012). Characterization of the major odor-active compounds in Thai durian (Durio zibethinus L. ‘Monthong’) by aroma extract dilution analysis and headspace gas chromatography–olfactometry. J. Agric. Food Chem. 60, 11253–11262. doi: 10.1021/jf303881k
Lim, S.-H., Nam, H., and Baek, H.-H. (2016). Aroma Characteristics of Acai Berry. Korean J. Food Sci. Technol. 48, 122–127. doi: 10.9721/KJFST.2016.48.2.122
Lindhorst, A. C., and Steinhaus, M. (2016). Aroma-active compounds in the fruit of the hardy kiwi (Actinidia arguta) cultivars Ananasnaya, Bojnice, and Dumbarton Oaks: differences to common kiwifruit (Actinidia deliciosa “Hayward”). Eur. Food Res. Technol. 242, 967–975. doi: 10.1007/s00217-015-2603-y
Liu, X., Hao, N., Feng, R., Meng, Z., Li, Y., and Zhao, Z. (2021). Transcriptome and metabolite profiling analyses provide insight into volatile compounds of the apple cultivar ‘Ruixue’ and its parents during fruit development. BMC Plant Biol. 21:231. doi: 10.1186/s12870-021-03032-3
Lu, G., Sun, H., She, P., Youn, J. Y., Warburton, S., Ping, P., et al. (2009). Protein phosphatase 2Cm is a critical regulator of branched-chain amino acid catabolism in mice and cultured cells. J. Clin. Invest. 119, 1678–1687. doi: 10.1172/jci38151
Lu, J., Li, H., Quan, J., An, W., Zhao, J., and Xi, W. (2017). Identification of characteristic aroma volatiles of Ningxia goji berries (Lycium barbarum L.) and their developmental changes. Int. J. Food Prop. 20, S214–S227. doi: 10.1080/10942912.2017.1295254
Lytra, G., Tempere, S., de Revel, G., and Barbe, J.-C. (2014). Distribution and Organoleptic Impact of Ethyl 2-Methylbutanoate Enantiomers in Wine. J. Agric. Food Chem. 62, 5005–5010. doi: 10.1021/jf500670z
Mahattanatawee, K., Goodner, K. L., and Baldwin, E. A. (2005). Volatile constituents and character impact compounds of selected Florida’s tropical fruit. Proc. Fla. State Hort. Soc. 118, 414–418.
Maia, J. G. S., Andrade, E. H. A., and Silva, M. H. L. (2008). Aroma volatiles of pequi fruit (Caryocar brasiliense Camb.). J. Food Compos. Anal. 21, 574–576. doi: 10.1016/j.jfca.2008.05.006
Maia, J. G. S., Andrade, E. H. A., and Zoghbi, M. G. B. (2003). Volatiles from fruits of Pouteria Pariry (Ducke) Baehni and P. caimito (Ruiz and Pavon.) Rdlkl. J. Essent. Oil-Bear. Plants 6, 127–129. doi: 10.1080/0972-060x.2003.10643339
Maia, J. G. S., Andrade, E. H. A., and Zoghbi, M. G. B. (2004). Aroma volatiles from two fruit varieties of jackfruit (Artocarpus heterophyllus Lam.). Food Chem. 85, 195–197. doi: 10.1016/S0308-8146(03)00292-9
Majcher, M. A., Scheibe, M., and Jelén, H. H. (2020). Identification of Odor Active Compounds in Physalis peruviana L. Molecules 25:245. doi: 10.3390/molecules25020245
Márquez, C. J., Jimenez, A. M., Osorio, C., and Cartagena, J. R. (2011). Volatile compounds during ripening of Colombian soursop (Annona muricata L. cv. Elita). Vitae 18, 245–250.
Marsol-Vall, A., Kortesniemi, M., Karhu, S. T., Kallio, H., and Yang, B. (2018). Profiles of volatile compounds in blackcurrant (Ribes nigrum) cultivars with a special focus on the influence of growth latitude and weather conditions. J. Agric. Food Chem. 66, 7485–7495. doi: 10.1021/acs.jafc.8b02070
Martín, D. A., and Osorio, C. (2019). Identification of aroma-active volatile compounds in Pouteria sapota fruit by aroma extraction dilution analyses (AEDA). Quím. Nova 42, 1–4. doi: 10.21577/0100-4042.20170369
Mathieu, S., Cin, V. D., Fei, Z., Li, H., Bliss, P., Taylor, M. G., et al. (2009). Flavour compounds in tomato fruits: identification of loci and potential pathways affecting volatile composition. J. Exp. Bot. 60, 325–337. doi: 10.1093/jxb/ern294
Matich, A., and Rowan, D. (2007). Pathway analysis of branched-chain ester biosynthesis in apple using deuterium labeling and enantioselective gas chromatography–mass spectrometry. J. Agric. Food Chem. 55, 2727–2735. doi: 10.1021/jf063018n
Misran, A., Padmanabhan, P., Sullivan, J. A., Khanizadeh, S., and Paliyath, G. (2015). Composition of phenolics and volatiles in strawberry cultivars and influence of preharvest hexanal treatment on their profiles. Can. J. Plant Sci. 95, 115–126. doi: 10.4141/CJPS-2014-245
Mohd Ali, M., Hashim, N., Abd Aziz, S., and Lasekan, O. (2020). Pineapple (Ananas comosus): A comprehensive review of nutritional values, volatile compounds, health benefits, and potential food products. Food Res. Int. 137:109675. doi: 10.1016/j.foodres.2020.109675
Monteiro, S. S., Ribeiro, S. R., Soquetta, M. B., Pires, F. J., Wagner, R., and Rosa, C. S. (2018). Evaluation of the chemical, sensory and volatile composition of sapota-do-Solimões pulp at diferent ripening stages. Food Res. Int. 109, 159–167. doi: 10.1016/j.foodres.2018.04.033
Montero-Calderón, M., Rojas-Graü, M. A., and Martín-Belloso, O. (2010). Aroma Profile and Volatiles Odor Activity Along Gold Cultivar Pineapple Flesh. J. Food Sci. 75, S506–S512. doi: 10.1111/j.1750-3841.2010.01831.x
Mosblech, A., Feussner, I., and Heilmann, I. (2009). Oxylipins: structurally diverse metabolites from fatty acid oxidation. Plant Physiol. Bioch. 47, 511–517. doi: 10.1016/j.plaphy.2008.12.011
Nagegowda, D. A. (2010). Plant volatile terpenoid metabolism: biosynthetic genes, transcriptional regulation and subcellular compartmentation. FEBS Lett. 584, 2965–2973. doi: 10.1016/j.febslet.2010.05.045
Narain, N., Almeida, J. N., Galvão, M. S., Madruga, M. S., and Brito, E. S. (2004). Compostos voláteis dos frutos de maracujá (Passiflora edulis forma Flavicarpa) e de cajá (Spondias mombin L.) obtidos pela técnica de headspace dinâmico. Ciênc. Tecnol. Aliment. 24, 212–216. doi: 10.1590/S0101-20612004000200009
Narain, N., de Sousa Galvão, M., da, S., Ferreira, D., and Navarro, D. M. A. F. (2010). Identification of Volatile Compounds in Mangaba (Hancornia speciosa Gomes) Fruit – a Preliminary Study. Acta Hortic. 864, 289–294. doi: 10.17660/ActaHortic.2010.864.38
Ni, E., Zhou, L., Li, J., Jiang, D., Wang, Z., Zheng, S., et al. (2018). OsCER1 plays a pivotal role in very-long-chain alkane biosynthesis and affects plastid development and programmed cell death of tapetum in rice (Oryza sativa L.). Front. Plant Sci. 9:1217. doi: 10.3389/fpls.2018.01217
Noble, A. C. (1996). Taste-aroma interactions. Trends Food Sci. Technol. 7, 439–444. doi: 10.1016/S0924-2244(96)10044-3
Nogueira, J. M. F., Fernandes, P. J. P., and Nascimento, A. M. D. (2003). Composition of Volatiles of Banana Cultivars from Madeira Island. Phytochem. Anal. 14, 87–90. doi: 10.1002/pca.691
Nogueira, J. P., Pires, de Siqueira, A. C., Dutra Sandes, R. D., de Sousa Galvão, M., Santos Leite, et al. (2018). An insight into key volatile compounds in acerola (Malpighia emarginata DC.) pulp based on their odour activity values and chemometric evaluation. Anal. Methods 10, 5851–5866. doi: 10.1039/c8ay01427b
Noichinda, S., Ueda, Y., Imahori, Y., and Chachin, K. (1999a). Subcellular Localization of Alcohol Acetyltransferase in Strawberry Fruit. Food Sci. Technol. Res 5, 239–242. doi: 10.3136/fstr.5.239
Noichinda, S., Ueda, Y., Imahori, Y., and Chachin, K. (1999b). Thioester Production and Thioalcohol Specificity of Alcohol Acetyltransferase in Strawberry Fruit. Food Sci. Technol. Res 5, 99–103. doi: 10.3136/fstr.5.99
Oliveira, A. L., Lopes, R. B., Cabral, F. A., and Eberlin, M. N. (2006). Volatile compounds from pitanga fruit (Eugenia uniflora L.). Food Chem. 99, 1–5. doi: 10.1016/j.foodchem.2005.07.012
Oliveira, I., de Pinho, P. G., Malheiro, R., Baptista, P., and Pereira, J. A. (2011). Volatile profile of Arbutus unedo L. fruits through ripening stage. Food Chem. 128, 667–673. doi: 10.1016/j.foodchem.2011.03.084
Ong, B. T., Nazimah, S. A. H., Tan, C. P., Mirhosseini, H., Osman, A., Mat Hashim, D., et al. (2008). Analysis of volatile compounds in five jackfruit (Artocarpus heterophyllus L.) cultivars using solid-phase microextraction (SPME) and gas chromatography-time-of-flight mass spectrometry (GC-TOFMS). J. Food Compos. Anal. 21, 416–422. doi: 10.1016/j.jfca.2008.03.002
Ong, P. K., Acree, T. E., and Lavin, E. H. (1998). Characterization of volatiles in rambutan fruit (Nephelium lappaceum L.). J. Agric. Food Chem. 46, 611–615. doi: 10.1021/jf970665t
Ong, P. K., and Acree, T. E. (1998). Gas chromatography/olfactory analysis of lychee (Litchi chinesis Sonn.). J. Agric. Food Chem. 46, 2282–2286. doi: 10.1021/jf9801318
Osorio, C., Alarcon, M., Moreno, C., Bonilla, A., Barrios, J., Garzón, C., et al. (2006). Characterization of Odor-Active Volatiles in Champa (Campomanesia lineatifolia R. & P.). J. Agric. Food Chem. 54, 509–516. doi: 10.1021/jf052098c
Oz, A. T., Baktemur, G., Paydas Kargi, S., and Kafkas, E. (2016). Volatile Compounds of Strawberry Varieties. Chem. Nat. Compd. 52, 507–509. doi: 10.1007/s10600-016-1690-8
Paolo, D., Bianchi, G., Scalzo, R. L., Morelli, C. F., Rabuffetti, M., and Speranza, G. (2018). The Chemistry behind Tomato Quality. Nat. Prod. Commun. 13, 1225–1232. doi: 10.1177/1934578X1801300927
Parker, P. J., and Randle, P. J. (1978). Partial purification and properties of branched-chain 2-oxo acid dehydrogenase of ox liver. Biochem. J. 171, 751–757. doi: 10.1042/bj1710751
Parliament, T. H., and Smith, A. J. (1999). Volatile Components of Amelanchier arborea Nutt. (Shadberry) Fruit. J. Essent. Oil Res. 11, 606–608. doi: 10.1080/10412905.1999.9701222
Paxton, R., and Harris, R. A. (1984). Regulation of branched-chain α-ketoacid dehydrogenase kinase. Arch. Biochem. Biophys. 231, 48–57. doi: 10.1016/0003-9861(84)90361-8
Peng, C., Uygun, S., Shiu, S. H., and Last, R. L. (2015). The impact of the branched-chain ketoacid dehydrogenase complex on amino acid homeostasis in Arabidopsis. Plant Physiol. 169, 1807–1820. doi: 10.1104/pp.15.00461
Pérez, A. G., Olias, R., Luaces, P., and Sanz, C. (2002). Biosynthesis of Strawberry Aroma Compounds through Amino Acid Metabolism. J. Agric. Food Chem. 50, 4037–4042. doi: 10.1021/jf011465r
Pino, J. A. (2010). Volatile Compounds from Fruits of Pouteria campechiana (Kunth) Baehni. J. Essent. Oil-Bear. Plants 13, 326–330. doi: 10.1080/0972060X.2010.10643829
Pino, J. A. (2014). Odour-active compounds in papaya fruit cv. Red Maradol. Food Chem. 146, 120–126. doi: 10.1016/j.foodchem.2013.09.031
Pino, J. A., and Bent, L. (2013). Odour-active compounds in guava (Psidium guajava L. cv. Red Suprema). J. Sci. Food Agric. 93, 3114–3120. doi: 10.1002/jsfa.6153
Pino, J. A., and Marbot, R. (2001). Volatile Flavor Constituents of Acerola (Malpighia emarginata DC.) Fruit. J. Agric. Food Chem. 49, 5880–5882. doi: 10.1021/jf010270g
Pino, J. A., and Quijano, C. E. (2007). Volatile compounds of arazá fruit (Eugenia stipitata McVaught). Rev. CENIC, Cienc. Quím. 38, 363–366.
Pino, J. A., and Quijano, C. E. (2012). Study of the volatile compounds from plum (Prunus domestica L. cv. Horvin) and estimation of their contribution to the fruit aroma. Ciênc. Tecnol. Aliment. Campinas 32, 76–83. doi: 10.1590/S0101-20612012005000006
Pino, J. A., and Roncal, E. (2016). Characterisation of odour-active compounds in cherimoya (Annona cherimola Mill.) fruit. Flavour Fragr. J. 31, 143–148. doi: 10.1002/ffj.3292
Pino, J. A., Cuevas-Glory, L. F., Marbot, R., and Fuentes, V. (2008). Volatile compounds of grosella (Phyllanthus acidus [L.] Skeels) fruit. Rev. CENIC, Cienc. Quím. 39, 3–5.
Pino, J. A., Marbot, R., and Aguero, J. (2003a). Volatile components of sapodilla fruit (Manilkara achras L.). J. Essent. Oil Res. 15, 374–375. doi: 10.1080/10412905.2003.9698614
Pino, J. A., Marbot, R., and Vazquez, C. (2002). Characterization of volatiles in Loquat fruit (Eriobotrya japonica Lindl.). Rev. CENIC, Cienc. Quím. 33, 115–119.
Pino, J. A., Márquez, E., Quijano, C. E., and Castro, D. (2010). Volatile compounds in noni (Morinda citrifolia L.) at two ripening stages. Ciênc. Tecnol. Aliment., Campinas 30, 183–187. doi: 10.1590/S0101-20612010000100028
Pino, J. A., Mesa, J., Muñoz, Y., Pilar Marti, M., and Marbot, R. (2005). Volatile Components from Mango (Mangifera indica L.) Cultivars. J. Agric. Food Chem. 53, 2213–2223. doi: 10.1021/jf0402633
Pino, J., Marbot, R., Rosado, A., and Vazquez, C. (2003b). Volatile constituents of fruits of Garcinia dulcis Kurz. from Cuba. Flavour Fragr. J. 18, 271–274. doi: 10.1002/ffj.1187
Pintea, L., Dulf, F. V., Bunea, A., Socaci, S. A., Pop, E. A., Oprita, V.-A., et al. (2020). Carotenoids, Fatty Acids, and Volatile Compounds in Apricot Cultivars from Romania-A Chemometric Approach. Antioxidants 9:562. doi: 10.3390/antiox9070562
Pinto, A. B., Guedes, C. M., Moreira, R. T. A., and De Maria, C. A. B. (2006). Volatile constituent from headspace and aqueous solution of genipap (Genipa americana) fruit isolated by the solid-phase extraction method. Flavour Fragr. J. 21, 488–491. doi: 10.1002/ffj.1623
Plagemann I, Krings, I, Berger, R. G., and Maróstica, M. R. Jr. (2012). Volatile constituents of jaboticaba (Myrciaria jabuticaba (Vell.) O. Berg) fruits. J. Essent. Oil Res. 24, 45–51. doi: 10.1080/10412905.2012.645651
Plotto, A. (1998). Instrumental and sensory analysis of ‘Gala’ apple (Malus domestica, Borkh) aroma. [PhD dissertation]. Corvallis (OR): Oregon State University.
Plotto, A., Bai, J., and Baldwin, E. (2017). “Fruits,” in Springer Handbook of Odor, ed. A. Buettner (Switzerland: Springer International Publishing), 27–28.
Qian, M. C., and Wang, Y. (2005). Seasonal Variation of Volatile Composition and Odor Activity Value of‘Marion’(Rubus spp. hyb) and ‘Thornless Evergreen’ (R. laciniatus L.) Blackberries. J. Food Sci. 70, C13–C20. doi: 10.1111/j.1365-2621.2005.tb09013.x
Qin, G., Tao, S., Cao, Y., Wu, J., Zhang, H., Huang, W., et al. (2012). Evaluation of the volatile profile of 33 Pyrus ussuriensis cultivars by HS-SPME with GC-MS. Food Chem. 134, 2367–2382. doi: 10.1016/j.foodchem.2012.04.053
Qin, L., Wei, Q.-P., Kang, W.-H., Zhang, Q., Sun, J., and Liu, S.-Z. (2017). Comparison of Volatile Compounds in ‘Fuji’ Apples in the Different Regions in China. Food Sci. Technol. 23, 79–89. doi: 10.3136/fstr.23.79
Qualley, A. V., Widhalm, J. R., Adebesin, F., Kish, C. M., and Dudareva, N. (2012). Completion of the core beta-oxidative pathway of benzoic acid biosynthesis in plants. Proc. Natl. Acad. Sci. U.S.A. 109, 16383–16388. doi: 10.1073/pnas.1211001109
Quijano, C. E., and Pino, J. A. (2006). Changes in volatile constituents during the ripening of cocona (Solanum sessiliflorum Dunal) fruit. Rev. CENIC, Cienc. quím. 37, 133–136.
Quijano, C. E., and Pino, J. A. (2007). Analysis of Volatile Compounds of camu-camu (Myrciaria dubia (HBK) Mcvaugh) Fruit Isolated by Different Methods. J. Essent. Oil Res. 19, 527–533. doi: 10.1080/10412905.2007.9699323
Quijano-Célis, C. E., Echeverri-Gil, D., Ruiz, Y., and Pino, J. A. (2013). Volatiles from Syzygium paniculatum Fruit. Nat. Prod. Commun. 8, 129–130. doi: 10.1177/1934578X1300800131
Ramadan, M. M., El-Ghorab, A. H., and Ghanem, K. Z. (2015). Volatile compounds, antioxidants, and anticancer activities of Cape gooseberry fruit (Physalis peruviana L.): An in-vitro study. J. Arab Soc. Med. Res. 10, 56–64. doi: 10.4103/1687-4293.175556
Rezende, C. M., and Fraga, S. R. G. (2003). Chemical and aroma determination of the pulp and seeds of murici (Byrsonima crassifolia L.). J. Braz. Chem. Soc. 14, 425–428. doi: 10.1590/S0103-50532003000300014
Rohloff, J., Nestby, R., Nes, A., and Martinussen, I. (2009). Volatile profiles of European blueberry: few major players, but complex aroma patterns. Latv. J. Agron. 12, 98–103.
Rowan, D. D., Lane, H. P., Allen, J. M., Fielder, S., and Hunt, M. B. (1996). Biosynthesis of 2-methylbutyl, 2-methyl-2-butenyl, and 2-methylbutanoate esters in red delicious and granny smith apples using deuterium-labeled substrates. J. Agric. Food Chem. 44, 3276–3285. doi: 10.1021/jf9508209
Ruse, K., Sabovics, M., Rakcejeva, T., Dukalska, L., Galoburda, R., and Berzina, L. (2012). The effect of drying conditions on the presence of volatile compounds in cranberries. Int. J. Agric. Biosyst. Eng. 6, 163–169. doi: 10.5281/zenodo.1086205
Russo, F., Caporaso, N., Paduano, A., and Sacchi, R. (2017). Characterisation of volatile compounds in Cilento (Italy) figs (Ficus carica L.) cv. Dottato as affected by the drying process. Int. J. Food Prop. 20, S1366–S1376. doi: 10.1080/10942912.2017.1344991
Sampaio, T. S., and Nogueira, P. C. L. (2006). Volatile components of mangaba fruit (Hancornia speciosa Gomes) at three stages of maturity. Food Chem. 95, 606–610. doi: 10.1016/j.foodchem.2005.01.038
Sanabria, G. G. R., Garcia, A. J. C., Lima, A. W. O., Alejandra, B.-M. M., and Narain, N. (2018). HS-SPME-GC-MS detection of volatile compounds in Myrciaria jabuticaba Fruit. Sci. Agropecu. 9, 319–327. doi: 10.17268/sci.agropecu.2018.03.03
Sánchez, G., Besada, C., Badenes, M. L., Monforte, A. J., and Granell, A. (2012). A Non-Targeted Approach Unravels the Volatile Network in Peach Fruit. PLoS One 7:e38992. doi: 10.1371/journal.pone.0038992
Santos, G. B. M., Dionísio, A. P., Magalhães, H. C. R., Abreu, F. A. P., Lira, S. M., Lima, A. C. V., et al. (2020). Effects of processing on the chemical, physicochemical, enzymatic, and volatile metabolic composition of pitaya (Hylocereus polyrhizus (F.A.C. Weber) Britton & Rose). Food Res. Int. 127, 108710–108711. doi: 10.1016/j.foodres.2019.108710
Scala, A., Allmann, S., Mirabella, R., Haring, M. A., and Schuurink, R. C. (2013). Green Leaf Volatiles: a plant’s multifunctional weapon against herbivores and pathogens. Int. J. Mol. Sci. 14, 17781–17811. doi: 10.3390/ijms140917781
Scheuermann, E., Seguel, I., Montenegro, A., Bustos, R. O., Hormazabal, E., and Quiroz, A. (2008). Evolution of aroma compounds of murtilla fruits (Ugni molinae Turcz) during storage. J. Sci. Food Agric. 88, 485–492. doi: 10.1002/jsfa.3111
Schneider-Belhaddad, F., and Kolattukudy, P. E. (2000). Solubilization, partial purification, and characterization of a fatty aldehyde decarbonylase from a higher plant, Pisum sativum. Arch. Biochem. Biophys. 377, 341–349. doi: 10.1006/abbi.2000.1798
Schwab, W., Davidovich-Rikanati, R., and Lewinsohn, E. (2008). Biosynthesis of plant-derived flavor compounds. Plant J. 54, 712–732. doi: 10.1111/j.1365-313X.2008.03446.x
Shiota, H. (1993). New Esteric Components in the Volatiles of Banana Fruit (Musa sapientum L.). J. Agric. Food Chem. 41, 2056–2062. doi: 10.1021/jf00035a046
Shoko, T., Apostolides, Z., and Monjerezi, Saka, J. D. K. (2013). Volatile constituents of fruit pulp of Strychnos cocculoides (Baker) growing in Malawi using solid phase microextraction. S. Afr. J. Bot. 84, 11–12. doi: 10.1016/j.sajb.2012.09.001
Sidorov, V., Menczel, L., and Maliga, P. (1981). Isoleucine-requiring Nicotiana plant deficient in threonine deaminase. Nature 294, 87–88. doi: 10.1038/294087A0
Silva, M. R., Bueno, G. H., Araújo, R. L. B., Lacerda, I. C. A., Freitas, L. G., Morais, H. A., et al. (2019). Evaluation of the Influence of Extraction Conditions on the Isolation and Identification of Volatile Compounds from Cagaita (Eugenia dysenterica) Using HS-SPME/GC-MS. J. Braz. Chem. Soc. 30, 379–387. doi: 10.21577/0103-5053.20180187
Singh, B. K., and Shaner, D. L. (1995). Biosynthesis of branched chain amino acids: from test tube to field. Plant Cell 7, 935–944. doi: 10.2307/3870048
Sosa-Moguel, O., Pino, J. A., Sauri-Duch, E., and Cuevas-Glory, L. (2018). Characterization of odor-active compounds in three varieties of ciruela (Spondias purpurea L.) fruit. Int. J. Food Prop. 21, 1008–1016. doi: 10.1080/10942912.2018.1479858
Souleyre, E. J. F., Greenwood, D. R., Friel, E. N., Karunairetnam, S., and Newcomb, R. D. (2005). An alcohol acyl transferase from apple (cv. Royal Gala), MpAAT1, produces esters involved in apple fruit flavor. FEBS J. 272, 3132–3144. doi: 10.1111/j.1742-4658.2005.04732.x
Sugimoto, N. (2011). Branched-Chain Ester Biosynthesis in Ripening Apple Fruit. [PhD dissertation]. East Lansing (MI): Michigan State University.
Sugimoto, N., Engelgau, P., Jones, A. D., Song, J., and Beaudry, R. (2021). Citramalate synthase yields a biosynthetic pathway for isoleucine and straight- and branched-chain ester formation in ripening apple fruit. Proc. Natl. Acad. Sci. U.S.A. 118:e2009988118. doi: 10.1073/pnas.2009988118
Sun, S. Y., Jiang, W. G., and Zhao, Y. P. (2010). Characterization of the aroma-active compounds in five sweet cherry cultivars grown in Yantai (China). Flavour Fragr. J. 25, 206–213. doi: 10.1002/ffj.1994
Takeoka, G. R., Buttery, R. G., Turnbaugh, J. G., and Benson, M. (1995). Odor thresholds of various branched esters. LWT 28, 153–156. doi: 10.1016/S0023-6438(95)80028-X
Tateo, F., and Bononi, M. (2010). Headspace-SPME Analysis of Volatiles from Quince Whole Fruits. J. Essent. Oil Res. 22, 416–418. doi: 10.1080/10412905.2010.9700360
Taylor, N. L., Heazlewood, J. L., Day, D. A., and Millar, A. H. (2004). Lipoic Acid-Dependent oxidative catabolism of α-keto acids in mitochondria provides evidence for branched-chain amino acid catabolism in Arabidopsis. Plant Physiol. 134, 838–848. doi: 10.1104/pp.103.035675
Tholl, D. (2015). Biosynthesis and biological functions of terpenoids in plants. Adv. Biochem. Eng. Biotechnol. 148, 63–106. doi: 10.1007/10_2014_295
Tieman, D., Bliss, P., McIntyre, L. M., Blandon-Ubeda, A., Bies, D., Odabasi, A. Z., et al. (2012). The chemical interactions underlying tomato flavor preferences. Curr. Biol. 22, 1035–1039. doi: 10.1016/j.cub.2012.04.016
Tietel, Z., Porat, R., Weiss, K., and Ulrich, D. (2011). Identification of aroma-active compounds in fresh and stored ‘Mor’ mandarins. Int. J. Food Sci. Technol. 46, 2225–2231. doi: 10.1111/j.1365-2621.2011.02740.x
Tikunov, Y., Lommen, A., Ric de Vos, C. H., Verhoeven, H. A., Bino, R. J., Hall, R. D., et al. (2005). A novel approach for non-targeted data analysis for metabolomics: large-scale profiling of tomato fruit volatiles. Plant. Physiol. 139, 1125–1137. doi: 10.1104/pp.105.068130
Tokitomo, Y., Steinhaus, M., Bütner, A., and Schieberle, P. (2005). Odor-active constituents in fresh pineapple (Ananas comosus [L.] Merr.) by quantitative and sensory evaluation. Biosci. Biotechnol. Biochem. 69, 1323–1330. doi: 10.1271/bbb.69.1323
Tola, A. J., Jaballi, A., Germain, H., and Missihoun, T. D. (2021). Recent Development on Plant Aldehyde Dehydrogenase Enzymes and Their Functions in Plant Development and Stress Signaling. Genes 12:51. doi: 10.3390/genes12010051
Torrens-Spence, M. P., von Guggenberg, R., Lazear, M., Ding, H., and Li, J. (2014). Diverse functional evolution of serine decarboxylases: identification of two novel acetaldehyde synthases that uses hydrophobic amino acids as substrates. BMC Plant Biol. 14:247. doi: 10.1186/s12870-014-0247-x
Tressl, R., and Drawert, F. (1973). Biogenesis of banana volatiles. J. Agric. Food Chem. 21, 560–565. doi: 10.1021/jf60188a031
Uekane, T. M., Nicolotti, L., Griglione, A., Bizzo, H. R., Rubiolo, P., Bicchi, C., et al. (2017). Studies on the volatile fraction composition of three native Amazonian-Brazilian fruits: Murici (Byrsonima crassifolia L., Malpighiaceae), Bacuri (Platonia insignis M., Clusiaceae), and sapodilla (Manilkara sapota L., Sapotaceae). Food Chem. 219, 13–22. doi: 10.1016/j.foodchem.2016.09.098
Ulrich, D., Komes, D., Olbricht, K., and Hoberg, E. (2007). Diversity of aroma patterns in wild and cultivated Fragaria accessions. Genet. Resour. Crop Evol. 54, 1185–1196. doi: 10.1007/s10722-006-9009-4
Vendramini, A. L., and Trugo, L. C. (2000). Chemical composition of acerola fruit (Malpighia punicifolia L.) at three stages of maturity. Food Chem. 71, 195–198. doi: 10.1016/S0308-8146(00)00152-7
Viljanen, K., Heinio, R.-L., Juvonen, R., Kosso, T., and Puupponen-Pimia, R. (2014). Relation of sensory perception with chemical composition of bioprocessed lingonberry. Food Chem. 157, 148–156. doi: 10.1016/j.foodchem.2014.02.030
Viljoen, A. M., Kamatou, G. P. P., and Başer, K. H. C. (2008). Head-space volatiles of marula (Sclerocarya birrea subsp. caffra). S. Afr. J. Bot. 74, 325–326. doi: 10.1016/j.sajb.2007.10.005
Wall, M. M., Miller, S., and Siderhurst, M. S. (2018). Volatile changes in Hawaiian noni fruit, Morinda citrifolia L., during ripening and fermentation. J. Sci. Food Agric. 98, 3391–3399. doi: 10.1002/jsfa.8850
Wang, C., Zhang, W., Li, H., Mao, J., Guo, C., Ding, R., et al. (2019). Analysis of volatile compounds in pears by HS-SPME-GC×GC-TOFMS. Molecules 24:1795. doi: 10.3390/molecules24091795
Wendakoon, S. K., Ueda, Y., Imahori, Y., and Ishimaru, M. (2006). Effect of short-term anaerobic conditions on the production of volatiles, activity of alcohol acetyltransferase and other quality traits of ripened bananas. J. Sci. Food Agric. 86, 1475–1480. doi: 10.1002/jsfa.2518
Werkhoff, P., Güntert, M., Krammer, G., Sommer, H., and Kaulen, J. (1998). Vacuum Headspace Method in Aroma Research: Flavor Chemistry of Yellow Passion Fruits. J. Agric. Food Chem. 46, 1076–1093. doi: 10.1021/jf970655s
Wijaya, C. H., Ulrich, D., Lestari, R., Schippel, K., and Ebert, G. (2005). Identification of potent odorants in different cultivars of snake fruit [Salacca zalacca (Gaert.) Voss] using gas chromatography-olfactometry. J. Agric. Food Chem. 53, 1637–1641. doi: 10.1021/jf048950h
Wong, K. C., and Loi, H. K. (1996b). Volatile Constituents of Bouea macrophylla Griff. Fruit. J. Essent. Oil Res. 8, 99–100. doi: 10.1080/10942912.2016.1218892
Wong, K. C., Chee, S. G., and Er, C. C. (1996a). Volatile constituents of the fruits of Muntingia calabura L. J. Essent. Oil Res. 8, 423–426. doi: 10.1080/10412905.1996.9700655
Wong, K. C., Wong, S. N., Loi, H. K., and Lim, C. L. (1996c). Volatile constituents from the fruits of four edible Sapindaceae: Rambutan (Nephelium lappaceum L.), pulasan (N. ramboutan-ake (Labill.) Leenh.), longan (Dimocarpus longan Lour.), and mata kucing (D. longan ssp. malesianus Leenh.). Flavour Fragr. J. 11, 223–229. doi: 10.1002/(SICI)1099-1026(199607)11:4<223::AID-FFJ579<3.0.CO;2-B
Wong, K. C., Wong, S. W., Siew, S. S., and Tie, D. Y. (1994). Volatile Constituents of the Fruits of Lansium domesticum Correa (Duku and Langsat) and Baccaurea motleyana (Muell. Arg.) Muell. Arg. (Rambai). Flavour Fragr. J. 9, 319–324. doi: 10.1002/ffj.2730090608
Wu, Y., Duan, S., Zhao, L., Gao, Z., Luo, M., Song, S., et al. (2016). Aroma characterization based on aromatic series analysis in table grapes. Sci. Rep. 6:31116. doi: 10.1038/srep31116
Wu, Y., Pan, Q., Qu, W., and Duan, C. (2009). Comparison of volatile profiles of nine litchi (Litchi chinensis Sonn.) cultivars from Southern China. J. Agric. Food Chem. 57, 9676–9681. doi: 10.1021/jf902144c
Wyllie, S. G., and Fellman, J. K. (2000). Formation of volatile branched chain esters in bananas (Musa sapientum L.). J. Agric. Food Chem. 48, 3493–3496. doi: 10.1021/jf0001841
Wyllie, S. G., and Leach, D. N. (1992). Sulfur-containing compounds in the aroma volatiles of melons (Cucumis melo). J. Agric. Food Chem. 40, 253–256. doi: 10.1021/jf00014a017
Wyllie, S. G., Leach, D. N., Wang, Y., and Shewfelt, R. L. (1995). “Key aroma compounds in melons: Their development and cultivar dependence,” in Fruit Flavors: Biogenesis, Characterization, and Authentication, eds R. L. Rouseff and M. M. Leahy (Washington, DC: American Chemical Society), 248–257. doi: 10.1021/bk-1995-0596.ch022
Xi, W., Zheng, H., Zhang, Q., and Li, W. (2016). Profiling Taste and Aroma Compound Metabolism during Apricot Fruit Development and Ripening. Int. J. Mol. Sci. 17:998. doi: 10.3390/ijms17070998
Xiao, Z., Wu, Q., Niu, Y., Wu, M., Zhu, J., Zhou, X., et al. (2017). Characterization of the key aroma compounds in five varieties of mandarins by gas chromatography-olfactometry, odor activity values, aroma recombination, and omission analysis. J. Agric. Food Chem. 65, 8392–8401. doi: 10.1021/acs.jafc.7b02703
Xing, A., and Last, R. L. (2017). A regulatory hierarchy of the Arabidopsis branched-chain amino acid metabolic network. Plant Cell 29, 1480–1499. doi: 10.1105/tpc.17.00186
Xu, H., Zhang, F., Liu, B., Huhman, D. V., Sumner, L. W., Dixon, R. A., et al. (2013). Characterization of the formation of branched short-chain fatty acid:CoAs for bitter acid biosynthesis in hop glandular trichomes. Mol. Plant 6, 1301–1317. doi: 10.1093/mp/sst004
Yang, C., Wang, Y., Wu, B., Fang, J., and Li, S. (2011). Volatile compounds evolution of three table grapes with different flavour during and after maturation. Food Chem. 128, 823–830. doi: 10.1016/j.foodchem.2010.11.029
Yang, L., Liu, J., Wang, X., Wang, X., Ren, F., Zhang, Q., et al. (2019). Characterization of Volatile Component Changes in Jujube Fruits during Cold Storage by Using Headspace-Gas Chromatography-Ion Mobility Spectrometry. Molecules. 24:3904. doi: 10.3390/molecules24213904
Yilmaztekin, M. (2014). Analysis of volatile components of cape gooseberry (Physalis peruviana L.) grown in Turkey by HS-SPME and GC-MS. Sci. World J. 2014:796097. doi: 10.1155/2014/796097
Zhang, C. Y., Zhang, Q., Zhong, C. H., and Guo, M. Q. (2016). Analysis of volatile compounds responsible for kiwifruit aroma by desiccated headspace gas chromatography-mass spectrometry. J. Chromatogr. A. 1440, 255–259. doi: 10.1016/j.chroma.2016.02.056
Zhang, C. Y., Zhang, Q., Zhong, C. H., and Guo, M. Q. (2019a). Volatile fingerprints and biomarkers of three representative kiwifruit cultivars obtained by headspace solid-phase microextraction gas chromatography mass spectrometry and chemometrics. Food Chem. 271, 211–215. doi: 10.1016/j.foodchem.2018.07.169
Zhang, T., Bao, F., Yang, Y., Hu, L., Ding, A., Wang, J., et al. (2019b). A comparative analysis of floral scent compounds in intraspecific cultivars of Prunus mume with different corolla colours. Molecules 25:145. doi: 10.3390/molecules25010145
Zhao, Y., Zhan, P., Tian, H.-L., Wang, P., Lu, C., Tian, P., et al. (2021). Insights into the Aroma Profile in Three Kiwifruit Varieties by HS-SPME-GC-MS and GC-IMS Coupled with DSA. Food Anal. Methods 14, 1033–1042. doi: 10.1007/s12161-020-01952-8
Zheng, L.-Y., Sun, G.-M., Liu, Y.-G., Lv, L.-L., Yang, W.-X., Zhao, W.-F., et al. (2012). Aroma Volatile Compounds from Two Fresh Pineapple Varieties in China. Int. J. Mol. Sci. 13, 7383–7392. doi: 10.3390/ijms13067383
Zhou, M., Lu, G., Gao, C., Wang, Y., and Sun, H. (2012). Tissue-specific and nutrient regulation of the branched-chain α-keto acid dehydrogenase phosphatase, protein phosphatase 2Cm (PP2Cm). J. Biol. Chem. 287, 23397–23406. doi: 10.1074/jbc.m112.351031
Zhu, J.-C., Chen, F., Wang, L.-Y., Niu, Y.-W., Chen, H.-X., Wang, H.-L., et al. (2016). Characterization of the Key Aroma Volatile Compounds in Cranberry (Vaccinium Macrocarpon Ait.) Using Gas Chromatography–Olfactometry (GC-O) and odor activity value (OAV). J. Agric. Food Chem. 64, 4990–4999. doi: 10.1021/acs.jafc.6b01150
Zhu, J.-C., Wang, L.-Y., Xiao, Z.-B., and Niu, Y.-W. (2018). Characterization of the key aroma compounds in mulberry fruits by application of gas chromatography–olfactometry (GC-O), odor activity value (OAV), gas chromatography-mass spectrometry (GC–MS) and flame photometric detection (FPD). Food Chem. 245, 775–785. doi: 10.1016/j.foodchem.2017.11.112
Keywords: branched-chain, volatiles, fruit, aroma, flavor, biosynthesis, metabolism
Citation: Bizzio LN, Tieman D and Munoz PR (2022) Branched-Chain Volatiles in Fruit: A Molecular Perspective. Front. Plant Sci. 12:814138. doi: 10.3389/fpls.2021.814138
Received: 12 November 2021; Accepted: 23 December 2021;
Published: 27 January 2022.
Edited by:
Itay Maoz, Agricultural Research Organization (ARO), IsraelReviewed by:
Jun Song, Agriculture and Agri-Food Canada (AAFC), CanadaNatasha Spadafora, University of Calabria, Italy
Copyright © 2022 Bizzio, Tieman and Munoz. This is an open-access article distributed under the terms of the Creative Commons Attribution License (CC BY). The use, distribution or reproduction in other forums is permitted, provided the original author(s) and the copyright owner(s) are credited and that the original publication in this journal is cited, in accordance with accepted academic practice. No use, distribution or reproduction is permitted which does not comply with these terms.
*Correspondence: Patricio R. Munoz, cC5tdW5vekB1ZmwuZWR1