- 1Graduate School of Horticulture, Chiba University, Matsudo, Japan
- 2Plant Molecular Research Center, Chiba University, Chiba, Japan
This study aimed to evaluate short-duration (24 h) UV-B irradiation as a preharvest abiotic stressor in canola plants. Moreover, we quantified the expression levels of genes related to bioactive compounds synthesis in response to UV-B radiation. Canola seedlings were cultivated in a plant factory under artificial light (200 μmol m–2 s–1 photosynthetic photon flux density; white LED lamps; 16 h on/8 h off), 25°C/20°C daytime/nighttime air temperature, and 70% relative humidity. Eighteen days after sowing, the seedlings were subjected to supplemental UV-B treatment. The control plants received no UV-B irradiation. The plants were exposed to 3, 5, or 7 W m–2 UV-B irradiation. There were no significant differences in shoot fresh weight between the UV-B-irradiated and control plants. With increasing UV-B irradiation intensity and exposure time, the H2O2 content gradually increased, the expression levels of genes related to photosynthesis downregulated, and phenylpropanoid and flavonoid production, and also total phenolic, flavonoid, antioxidant, and anthocyanin concentrations were significantly enhanced. The genes related to secondary metabolite biosynthesis were immediately upregulated after UV-B irradiation. The relative gene expression patterns identified using qRT-PCR corroborated the variations in gene expression that were revealed using microarray analysis. The time point at which the genes were induced varied with the gene location along the biosynthetic pathway. To the best of our knowledge, this is the first study to demonstrate a temporal difference between the accumulation of antioxidants and the induction of genes related to the synthesis of this compound in UV-B-treated canola plants. Our results demonstrated that short-term UV-B irradiation could augment antioxidant biosynthesis in canola without sacrificing crop yield or quality.
Introduction
Phytochemicals are naturally occurring, bioactive, and non-nutrient compounds in plants (Visioli et al., 2011). They include polyphenols, terpenoids, alkaloids, carotenoids, aromatic glucosinolates, among others. Some of these promote human health, as they are anti-inflammatory, anticancer, antioxidant, and so on (Reddy et al., 2003; Scalbert et al., 2011). Research interest in foods containing functional antioxidant phytochemicals has recently grown. The quantity and quality of phytochemicals may be improved through various environmental controls. Plant factories and vertical farms can precisely regulate the ambient environment and are suitable as production systems for crops rich in phytochemicals (Goto, 2011).
Plants control a wide range of physiological processes and use UV radiation as an environmental signal. The three types of UV radiation are UV-C (100–280 nm), UV-B (280–315 nm), and UV-A (315–400 nm) (Madronich et al., 1998). However, only UV-A and UV-B radiations reach the surface of the Earth, as UV-C is absorbed mainly by the tropospheric ozone layer. As UV-B irradiation range is highly energetic and has a short wavelength, it can generate excessive reactive oxygen species (ROS) in plants exposed to high levels of it. Excessive ROS can damage DNA, proteins, membranes, and the photosynthetic apparatus. Hence, they can adversely affect plant growth and development (Tevini et al., 1981; Flint and Caldwell, 1984; Tevini and Steinmüller, 1987; Jenkins, 2009). In contrast, low-level UV-B irradiation promotes morphological responses in plants, such as leaf growth, stomatal differentiation, and the inhibition of hypocotyl elongation. Therefore, the morphophysiological responses of plants to UV irradiation vary with intensity and exposure duration. However, even at the same UV irradiation intensity and exposure time plant responses vary with species, genotype, resistance, sensitivity, leaf thickness, and other factors (Rozema et al., 1997; Kataria et al., 2014; Lee and Oh, 2015). According to a previous report (Sytar et al., 2018), the sensitivity of lettuce to UV was different depending on the species, and the content of secondary metabolites (total phenol, flavonoid, anthocyanin, and phenolic acids) also varied when different lettuce species were exposed to the same UV conditions.
Plants may adapt to augmented UV-B irradiation by increasing secondary metabolite production. UV-B-responsive genes are induced via UV resistance locus 8 (UVR8)-dependent UV-B signaling pathway, and promote the accumulation of phenolic compounds, such as hydroxycinnamic, ferulic, caffeic, and sinapic acids and flavonoid compounds, such as luteolin, kaempferol, and quercetin (Jenkins, 2009; Wargent et al., 2009; Neugart et al., 2014; Escobar et al., 2017). The overall responses of plants to UV-B irradiation are governed by acclimatization mechanisms, such as the accumulation of compounds that absorb UV-B radiation, and they protect the photosynthetic apparatus from injury (Allen et al., 1998; Chang et al., 2009). The long-term effects of UV-B irradiation on plants have been extensively investigated, and the findings of these studies helped predict the consequences of increasing UV exposure. Nevertheless, few studies have focused on the impact of short-term UV-B exposure on plants. As the photosynthesis process is sensitive to UV-B irradiation, most plant species are affected by it and their growth may be impaired in response to prolonged UV exposure. Therefore, research is being conducted on increasing the phytochemical content of plants without inhibiting their growth. It was recently discovered that short-term (several days) UV-B irradiation might serve as a preharvest treatment to obtain plant products rich in antioxidants (Pandey and Pandey-Rai, 2014; Inostroza-Blancheteau et al., 2016). Studies on the effects of short-term UV-B irradiation may help elucidate UV-induced signaling pathways and trends in genes and/or parameters that immediately respond to UV irradiation.
Previous research showed that upregulation of the expression of genes related to bioactive secondary metabolites synthesis triggered by UV-B exposure may vary with duration. Inostroza-Blancheteau et al. (2016) found that PAL, CHS, and F3’H expression levels were upregulated within 6 h of UV-B exposure. Elevated total phenolic content was observed in highbush blueberry leaves (Vaccinium corymbosum L. cv. Bluegold) exposed to UV-B for 24 h. In addition, Neugart and Bumke-Vogt (2021) investigated the effects on major genes expression of the phenylpropanoid pathway, contents of the flavonoid groups, and hydroxycinnamic acid derivatives after short-term UV-B irradiation and before the harvest of various Brassica species (B. rapa, B. nigra, B. oleracea, B. juncea, B. napus, and B. carinata). The response during the acclimation period after the UV-B irradiation (2 and 24 h) was investigated, but the response immediately after the UV-B irradiation was not confirmed. If we conduct a study to check the changes in gene expression and the content of bioactive compounds immediately after UV exposure, it is expected that the temporal differences between gene expression and the synthesis of bioactive compounds in response to UV irradiation are elucidated.
Hence, this study aimed to identify UV-B treatment conditions conducive to the accumulation of bioactive compounds without inhibiting plant growth. Furthermore, this study was performed to confirm the expression patterns of photosynthesis and secondary metabolites biosynthesis related genes and the increasing patterns of bioactive compounds according to UV-B exposure time in canola plants.
Materials and Methods
Plant Materials and Cultivation Conditions
The experiments were conducted at Chiba University, Japan, in a closed plant production system with multilayer cultivation shelves. Canola (Brassica napus L. cv. Kizakino-natane) was the plant material. It was used as a model for research on the growth, gene expression, and accumulation of bioactive compounds in a Brassica leaf vegetable cultivated under various environmental conditions (Goto et al., 2020; Son et al., 2020). The seeds were germinated on Kimtowels (NIPPONPAPER CRECIA Co. Ltd., Tokyo, Japan). One day after sowing (DAS), the germinated seeds were transplanted to M-size polyurethane sponges (M Hydroponics Laboratory Co. Inc., Aichi, Japan). The seedlings were then transplanted to 18.6-L hydroponic containers (San Box No. 26B; SANKO Co. Ltd., Tokyo, Japan) under white LED lamps (LDL40S-N/19/21; Panasonic Corp., Osaka, Japan) and cultivated until 18 DAS. A quarter-strength Otsuka A formulation (OAT house A treatment; OAT Agrio Co. Ltd., Tokyo, Japan) was the nutrient solution used in all the experiments. The pH and electrical conductivity of the nutrient solution were ∼6.4-6.5 and ∼1.0-1.1 dS m–1, respectively. The environmental conditions were 200 μmol m–2 s–1 photosynthetic photon flux density, 16-h light/8-h dark, 25°C/20°C daytime/nighttime air temperature, 70% RH, and 1,000 μmol mol–1 CO2.
UV-B Treatment
The 18-DAS seedlings were subjected to UV-B irradiation at three intensities, including relatively low (intensity 3 W m–2; daily dose 259.2 kJ m–2), medium (intensity 5 W m–2; daily dose 432 kJ m–2), and high (intensity 7 W m–2; daily dose 604.8 kJ m–2) (Figure 1A). The UV-B energy levels used in this study were determined through preliminary experiments. When UV-B levels of 3, 5, and 7 W m–2 were applied to 18-DAS canola plants for 3 days, changes in gene expression of PAL, CHS, rbcL, and rbcS, and morphological changes such as a decrease in leaf area and increase in specific leaf weight (leaf thickness) were observed in leaves in response to UV-B levels (data not shown). Therefore, the three levels of UV-B used in our study were thought to be effective treatments for inducing both gene expression and bioactive compounds synthesis in canola plants for only 1 day. This study was conducted to confirm the initial response by short-term UV-B irradiation for 1 day. Each UV-B irradiation intensity was regulated by covering the UV-B lamp with Al foil. UV-B irradiation was applied to the canola plants for 24 h. The UV-B irradiation source was a UV-B lamp (TL20W/01 RS; Philips, Hamburg, Germany). Figure 1A shows the spectral radiant flux of the UV-B lamp. The UV-B lamp spectrum was measured with a spectroradiometer (USR-45D; Ushio Inc., Tokyo, Japan). Ten samples were performed during 24 h. The duration of the UV-B treatments and the sampling intervals are shown in Figure 1B. Shoot fresh weights were determined immediately before and after 24 h UV-B treatment.
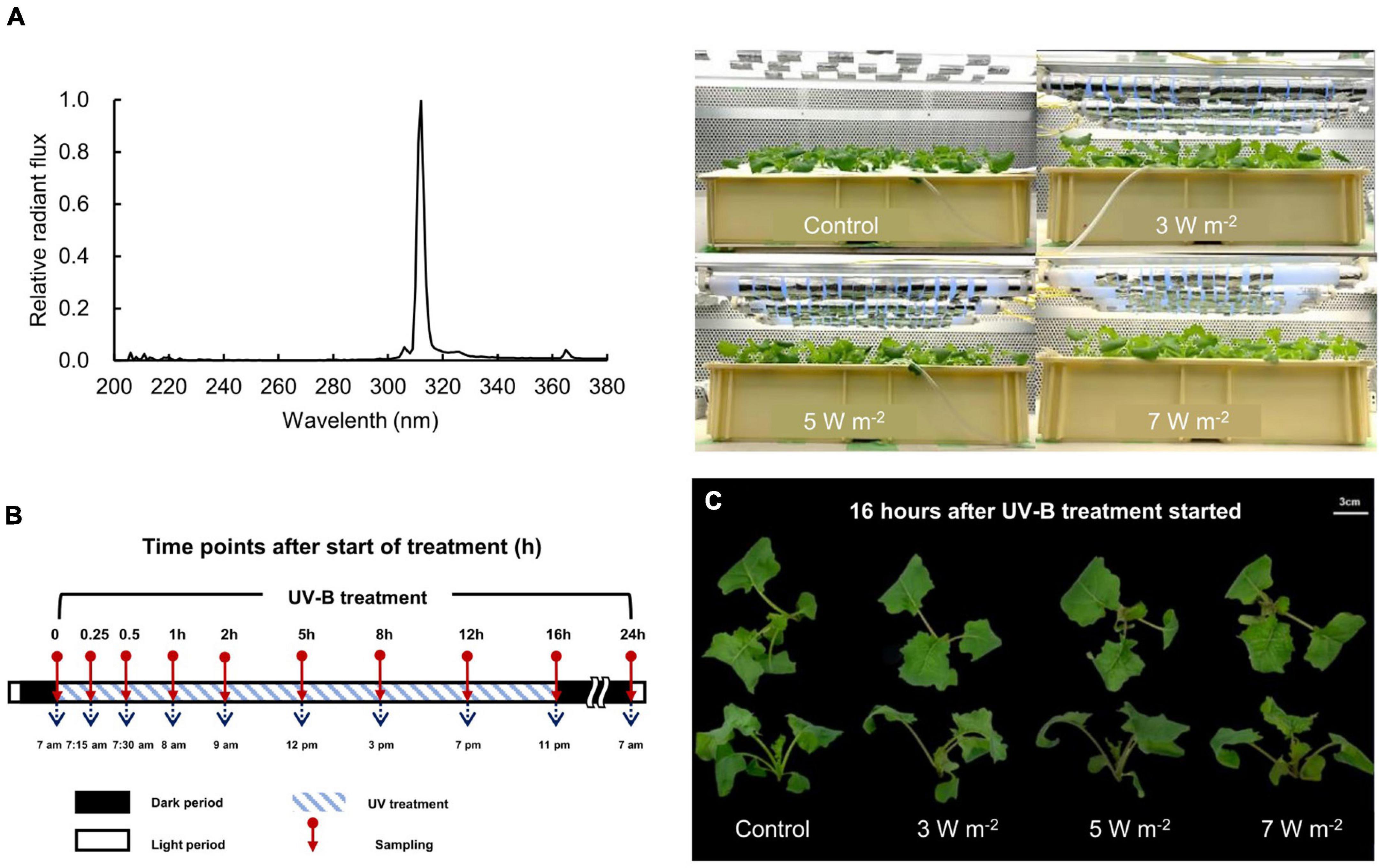
Figure 1. A spectral radiant flux of UV-B lamp (TL20W/01 RS; Philips, Hamburg, Germany) measured with a spectroradiometer (USR-45D; Ushio Inc., Tokyo, Japan) and photographs of canola plants under UV-B lamps (A), UV-B sampling and exposure times (B), and canola plants after 16 h UV-B treatment (C). UV-B irradiation intensity was set to 3, 5, and 7 W m–2 by overlapping Al foil on the lamp tube. Sampling was conducted at 0, 0.25, 0.5, 1, 2, 5, 8, 12, 16, and 24 h to investigate time-dependent responses to UV-B irradiation. UV-B irradiation intensities at top of the cultivation panel were set to 3, 5, and 7 W m–2. White bar = 3 cm.
Total Phenolic Concentration and Antioxidant Capacity Determination
Canola leaf samples were dried under vacuum in a freezer (FDU-1110; Tokyo Rikakikai Co. Ltd., Tokyo, Japan) at −45°C for 24 h and pulverized in an MM400 ball mill (Retsch GmbH, Haan, Germany) at 20 Hz for 2 min. Dried powder (0.01 g) was extracted with 1 mL of 80% (v/v) acetone. The third leaves of each canola plant that were subjected to different UV-B irradiation intensities were used in the analysis. The UV-B irradiation times were 0, 0.25, 0.5, 1, 2, 5, 8, 12, 16, and 24 h. Total phenolics and antioxidants were extracted from ∼200 mg fresh leaf sample with 80% (v/v) acetone according to the methods of Miller and Rice-Evans (1996) and Ainsworth and Gillespie (2007), respectively. The total phenolic concentration and the antioxidant capacity were determined with a spectrophotometer (V-750; JASCO Corp., Tokyo, Japan) at 765 nm and 730 nm, respectively. Results were expressed as milligrams gallic acid equivalents (GAE) per gram of fresh weight for the total phenolic concentration (GAE mg g–1 FW). To determine the antioxidant capacity, acetone extracts were diluted 10-fold, and the results were expressed as millimoles trolox-equivalents per gram fresh weight (TEAC mM g–1 FW).
Flavonoid Concentration Determination
The third leaves of each canola plant were exposed to UV-B irradiation for 0, 0.25, 0.5, 1, 2, 5, 8, 12, 16, or 24 h and were used for this analysis. Approximately, 200 mg of leaf tissue was dried under vacuum in a freezer (FDU-1110; Tokyo Rikakikai Co. Ltd.) at −45°C for 24 h and pulverized in an MM400 ball mill (Retsch GmbH) at 20 Hz for 2 min. About 7 mg dried powder was extracted with 1 ml of 70% (v/v) ethanol and the extracts were sonicated at 30 Hz for 6 min, incubated in the dark at 4°C overnight, and centrifuged at 13,000 × g for 2 min at room temperature (20–25°C). The extract (150 μl) was then added to a mixture of 750 μl distilled water plus 45 μl of 5% (w/v) NaNO2. The solution was then vortexed and maintained in the dark at room temperature (20–25°C) for 6 min. Then, 90 μl of 10% (w/v) AlCl3 was added to the solution, and the mixture was incubated for 5 min. Then, 300 μl of 1 M NaOH and 165 μl of distilled water were added to the solution. The optical density of the reaction mixture was measured in a spectrophotometer (V-750; JASCO Corp.) at 510 nm. The flavonoid concentration was expressed as milligrams catechin equivalents per gram dry weight (mg catechin/g DW).
Anthocyanin Concentration Determination
Approximately, 400-500 mg fresh second leaf tissue was collected from plants subjected to UV-B irradiation for 0.25, 0.5, 1, 2, 5, 8, 12, 16, or 24 h. The leaf tissue was stored at −80°C until the analysis. The anthocyanins were analyzed according to the method of Mancinelli and Schwartz (1984) with certain modifications. The plant tissues were extracted overnight at 4°C in 400 μl of 1% (v/v) HCl in methanol. Two hundred microliters of distilled water plus 500 μl chloroform were added to the extracts and the mixtures were centrifuged at 13,000 × g for 2 min at room temperature (20–25°C). The chloroform layer was separated, 400 μl of the top layer was transferred to a fresh microtube, and 600 μl of 1% (v/v) HCl in methanol was added to it. The anthocyanin concentrations were calculated using the following formula:
where, the factor 0.25 compensates for the contribution of the chlorophylls to A530. Cyanidin-3-glucoside was a reference standard.
Hydrogen Peroxide Content Determination
The hydrogen peroxide content of the canola plants was determined according to the method of Velikova et al. (2000). Fresh leaf tissue (0.2 g) was ground twice in the MM400 ball mill (Retsch GmbH) at 30 Hz for 1 min each time. Then 2 mL of 0.1% (w/v) trichloroacetic acid (TCA) was added to the microtube containing the leaf sample. The extract was centrifuged at 12,000 × g for 15 min at room temperature (20–25°C). The supernatant (0.5 mL) was added to 0.5 mL of 10 mM potassium phosphate buffer (pH 7.0) plus 1 ml of 1 M KI. The absorbance was measured in a spectrophotometer (V-750; JASCO Corp.) at 390 nm. H2O2 in the leaf extracts was estimated via an equation used to determine standard H2O2 concentrations. The H2O2 was expressed as micromoles H2O2 equivalents per gram fresh weight (μmol H2O2/g FW).
Gene Expression Quantification
The third leaves were sampled at 0, 0.25, 0.5, 1, 2, 5, 8, 12, 16, and 24 h UV-B irradiation to investigate time-dependent changes in gene expression. Approximately, 100-150 mg fresh leaf sample was collected and stored at −80°C until the analysis. The RNeasy Plant Mini Kit (Qiagen N.V., Venlo, Netherlands) was used to extract total RNA. The oligonucleotide primers used in the experiments were constructed according to information obtained from the GenBank database (Supplementary Table 1). Complementary DNA (cDNA) was synthesized with a PrimeScript RT Reagent Kit (Perfect Real Time; Takara Bio Inc., Kusatsu, Shiga, Japan) in a GeneAmp PCR System 9700 (Thermo Fisher Scientific, Waltham, MA, United States). The PCR was performed in a Thermal Cycler Dice Real Time System (TP970; Takara Bio Inc.) set to 37°C for 15 min followed by incubation at 85°C for 5 s, termination of the reaction, and cooling at 4°C. TB Green Premix ex Taq (Tli RNaseH Plus; Takara Bio Inc.) was used for the PCR. The PCR conditions for the amplification were 95°C for 5 s (hold), 40 cycles of 95°C for 5 s→60°C for 30 s (two-step PCR), one cycle of 95°C for 15 s→60°C for 30 s→95°C for 15 s (dissociation). The following mRNAs were amplified: phenylalanine ammonia-lyase (PAL), cinnamic acid 4-hydroxylase (C4H), 4-coumaroyl-CoA ligase (4CL), ferulate 5-hydroxylase (F5H), chalcone synthase (CHS), chalcone isomerase (CHI), flavanone 3-hydroxylase (F3H), flavonoid 3′-hydroxylase (F3′H), flavonol synthase (FLS), dihydroflavonol 4-reductase (DFR), anthocyanidin synthase (ANS), constitutively photomorphogenic (COP1), elongated hypocotyl 5 (HY5), light-harvesting complex II chlorophyll a/b-binding protein gene (Lhcb), ribulose-1,5-bisphosphate carboxylase/oxygenase large subunit (rbcL), and ribulose-1,5-bisphosphate carboxylase/oxygenase small subunit (rbcS). The mRNA expression levels were normalized against that of the actin (ACT) reference gene. Relative gene expression was calculated using the log2 treatment:control ratio.
Microarray Analysis
To explore genome-wide expression changes, samples were selected from plants exposed to 5 W m–2 UV-B irradiation for 0.5, 2, 8, 16, and 24 h. Total RNA (1–5 μg) was isolated from each sample with an Agilent Quick Amp Labeling Kit (Agilent Technologies, Palo Alto, CA, United States) and used in the microarray analysis. After fragmentation, the cDNA (1.65 μg) was hybridized using Agilent microarray protocols. The hybridized probes were scanned with an Agilent G4900DA SG12494263. The ratios of normalized fluorescence values were obtained by calculating the log2 treatment:control expression ratios.
The differentially expressed genes (DEGs) were used to evaluate differential gene expression of 5 W m–2 UV-B irradiation. To analyze reliable data, the noise was excluded (signal evaluation). Flag values of microarray analyzed with Agilent software are as follows: [0] signal was not detected, [1] signal detected but difficult to evaluate, and [2] signal detected. Results of variations in gene expression only involved data with flag values [2]. After data signal evaluation, gene ontology (GO) terms were retrieved and data with confirmed GO function were used for DEGs analysis. Gene differences between those values were calculated based on the Log2 ratio. The result was shown as the number of upregulated (Log2 fold change ≥ 1) and downregulated genes (Log2 fold change ≤ −1).
Statistical Analysis
The means were subjected to one-way ANOVA in SPSS v. 24 (IBM Corp., Armonk, NY, United States). There were four biological replicates and one plant per replicate. The displayed data are the means and ±SE per treatment. The means were compared using the Tukey–Kramer test. Treatment means were considered significantly different at p < 0.05.
Results
Growth Characteristics After UV-B Exposure
After 16 h UV-B irradiation exposure, the stems of the canola plants turned red. Stem redness intensified with UV-B irradiation intensity (Figure 1C). At higher UV-B irradiation intensity levels, the fresh weight of the canola plant decreased after 24 h. However, none of the UV-B treatments (3, 5, or 7 W m–2) notably affected canola growth relative to the control after 24 h (Supplementary Figure 1).
Total Phenolic, Flavonoid, and Anthocyanin Concentrations, and Antioxidant Capacity in Response to UV-B
The total phenolic concentration and antioxidant capacity of the canola leaves varied with UV-B exposure time and irradiation level (Figure 2). The total phenolic concentration increased 2 h after the onset of UV-B irradiation and continuously increased over time (Figure 2A). The plants subjected to 3, 5, and 7 W m–2 UV-B irradiation for 16 h showed a 1.43-, 1.53-, and 1.66-fold increase, respectively, in total phenolic concentrations compared with the control plants. The total phenolic concentration increased with UV-B irradiation intensity, showing a 1.82-fold increase in the plants subjected to 7 W m–2 UV-B irradiation for 24 h compared with the control. After 24 h, the plants exposed to 7 W m–2 UV-B irradiation presented a 1.75-fold increase in antioxidant capacity compared with the control plants (Figure 2B).
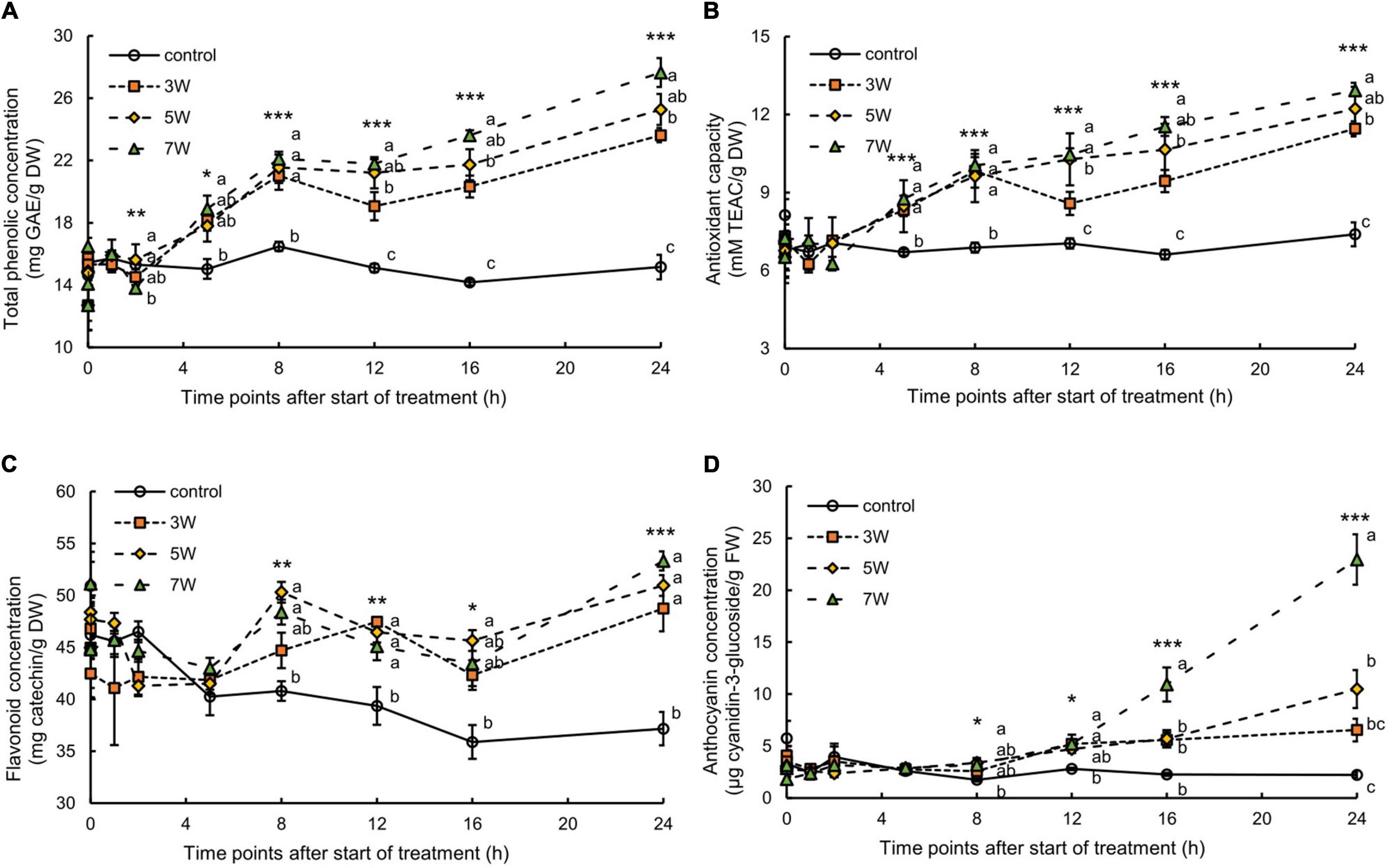
Figure 2. Time courses of total phenolic concentration (A), antioxidant capacity (B), flavonoid concentration (C), and anthocyanin concentration (D) in canola subjected to 3, 5, and 7 W m–2 UV-B irradiation intensity. Third (total phenolic and antioxidant capacity and flavonoid) and second (anthocyanin) leaves from the bottom of each canola plant were subjected to various UV-B irradiation intensities. Vertical bars indicate SE (n = 4). Different letters (a, b, and c) indicate a significant difference using Tukey–Kramer test (*p < 0.05, **p < 0.01, and ***p < 0.001, respectively).
The total flavonoid concentration in the canola leaves varied with UV-B irradiation intensity and exposure time (Figure 2C). The total flavonoid concentration increased 8 h after the onset of UV-B irradiation and continuously increased. They reached their maxima after 24 h UV-B irradiation but did not significantly differ among UV-B irradiation intensity levels.
The plants subjected to 3, 5, and 7 W m–2 UV-B irradiation showed 2.59-, 2.63-, and 5.15-fold increase, respectively, in anthocyanin concentrations after 16 h of the treatment compared with the control plants (Figure 2D). The plants exposed to 7 W m–2 UV-B irradiation for 24 h showed an 11.21-fold increase in anthocyanin concentrations compared with the control plants. The stems and leaves of the canola plants turned red after 16 h UV-B exposure.
Hydrogen Peroxide Production in Response to UV-B
In this work, the association between H2O2 content and plant UV-B exposure was like that for anthocyanin (Figure 3). The H2O2 content significantly increased 8 h after the onset of UV-B irradiation and continuously increased. The plants subjected to 7 W m–2 UV-B irradiation for 24 h showed maximum H2O2 content, representing a 4.97-fold increase compared with the control plants.
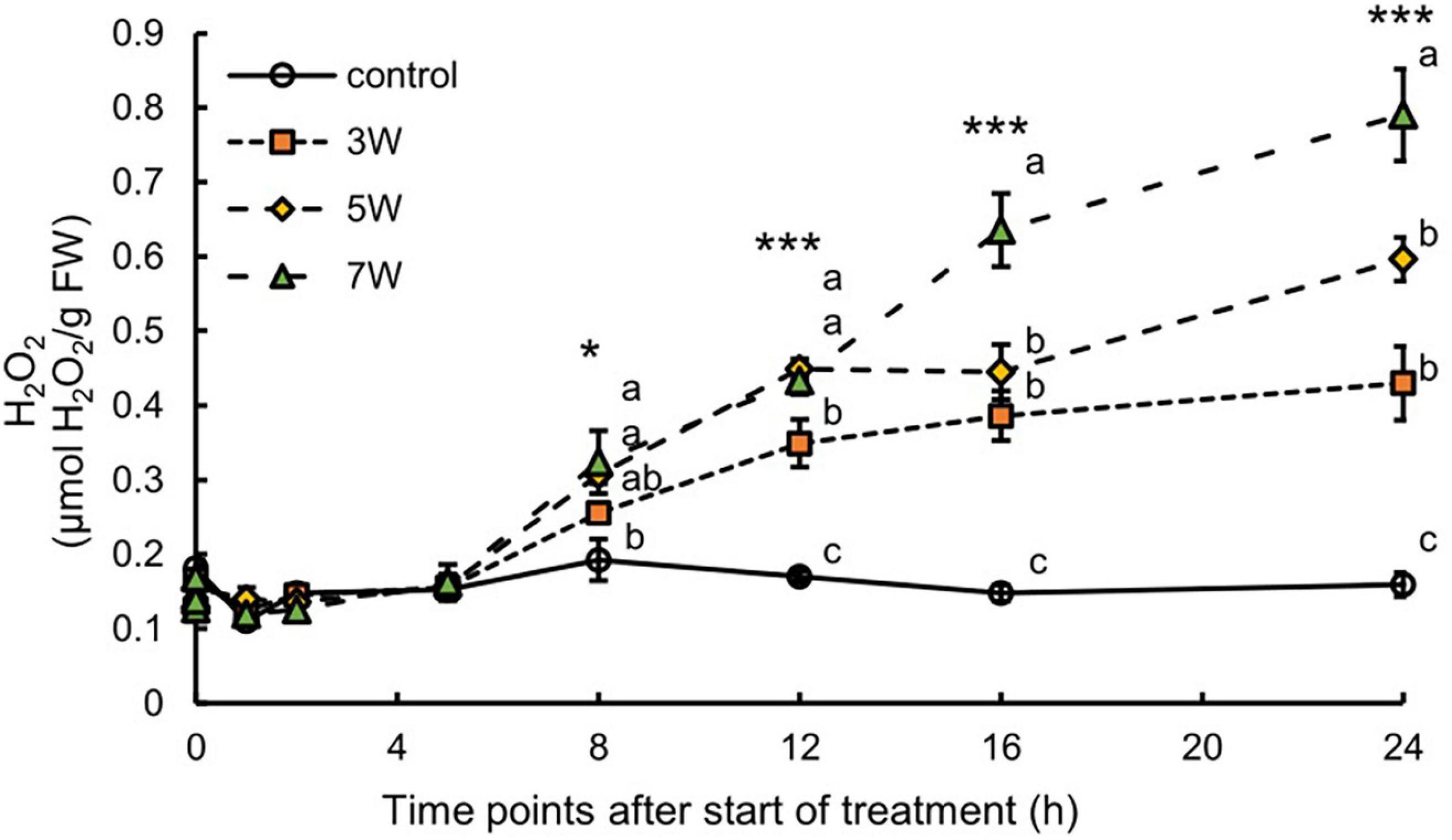
Figure 3. Time courses of H2O2 content in canola subjected to 3, 5, and 7 W m–2 UV-B irradiation intensity. Second leaves from the bottom of each canola plant were subjected to various UV-B irradiation intensities. Vertical bars indicate SE (n = 4). Different letters (a, b, and c) indicate a significant difference using Tukey–Kramer test (*p < 0.05, **p < 0.01, and ***p < 0.001, respectively).
Expression of Genes Related to UVR8-Dependent UV-B Signaling Pathway
The expression levels of COP1 and HY5 were significantly induced by UV-B irradiation (Figure 4). The levels of COP1 and HY5 were upregulated after the onset of UV-B irradiation and reached maxima at 2 h; thereafter, COP1 and HY5 levels were substantially downregulated.
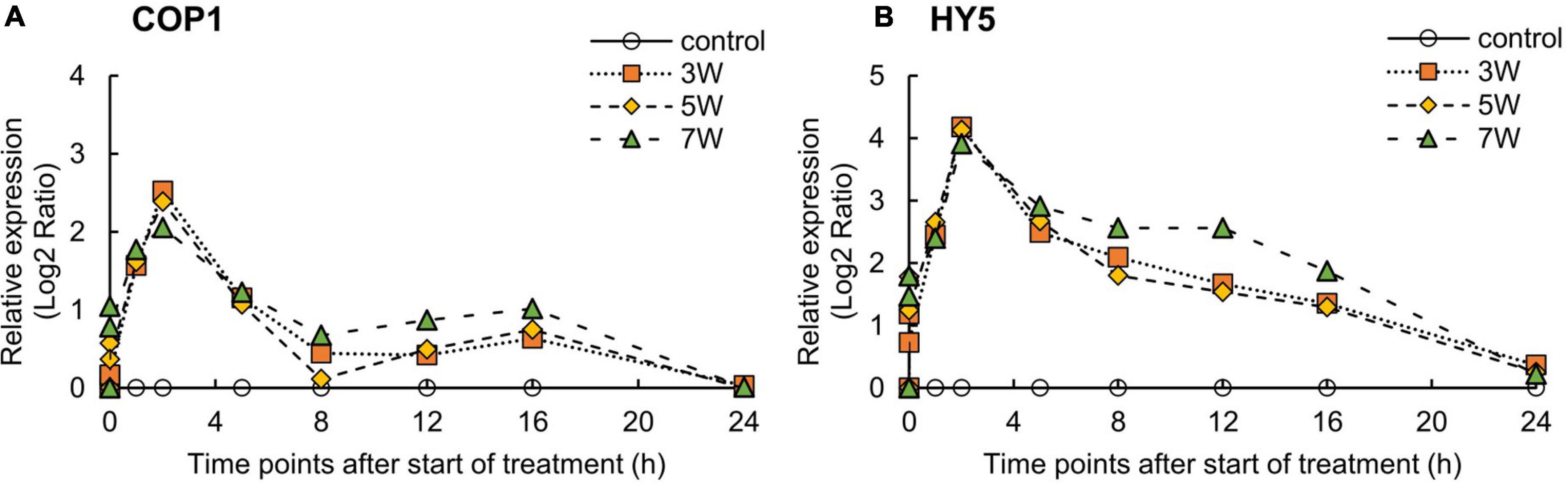
Figure 4. Time courses of COP1 (A) and HY5 (B) mRNA expression in canola plants. Vertical bars indicate SE (n = 4). Third leaves from the bottom of each canola plant were subjected to various UV-B irradiation intensities. UV-B irradiation intensities at the top of the cultivation panel were set to 3, 5, and 7 W m– 2. Line graphs indicate log2 fold changes (treatment:control) in gene expression levels.
Expression of UV-B-Responsive Genes Related to Phenylpropanoid and Flavonoid Biosynthesis
To identify DEGs from microarray data, genes were determined to be differentially expressed in the UV-B samples compared with the control samples (Supplementary Figure 2). GO annotation was used to functionally analyze the canola plant. Approximately, 2,500 gene transcripts were assessed after the signal evaluation was applied during 24 h of UV-B exposure (Supplementary Figure 2). Differential gene expression analysis showed a total of 291, 398, 197, 192, and 305 at 0.5, 2, 8, 16, and 24 h of UV-B exposure, respectively. Among them, 188 and 181 upregulated genes showed significantly highest fold changes at 0.5 and 2 h of UV irradiation. The number of downregulated genes was 217, showing the highest fold change at 2 h of UV irradiation.
Table 1 shows the results of the microarray analyses of the variations in the expression levels of the genes related to phenylpropanoid and flavonoid biosynthesis, respectively. The relative gene expression patterns identified using RT-PCR corroborated the variations in gene expression revealed by microarray analysis. PAL was continuously expressed between 2 h and 16 h UV-B irradiation. The expression levels of C3′H and CCoAOMT were significantly upregulated after 2 h UV-B exposure. F5H expression was upregulated after 8 h UV-B treatment. The downstream SGT expression was upregulated throughout the UV-B irradiation period. The expression levels of all flavonoid genes, except FLS and ANS, continuously varied between 2 and 16 h of UV-B treatment (Table 1).
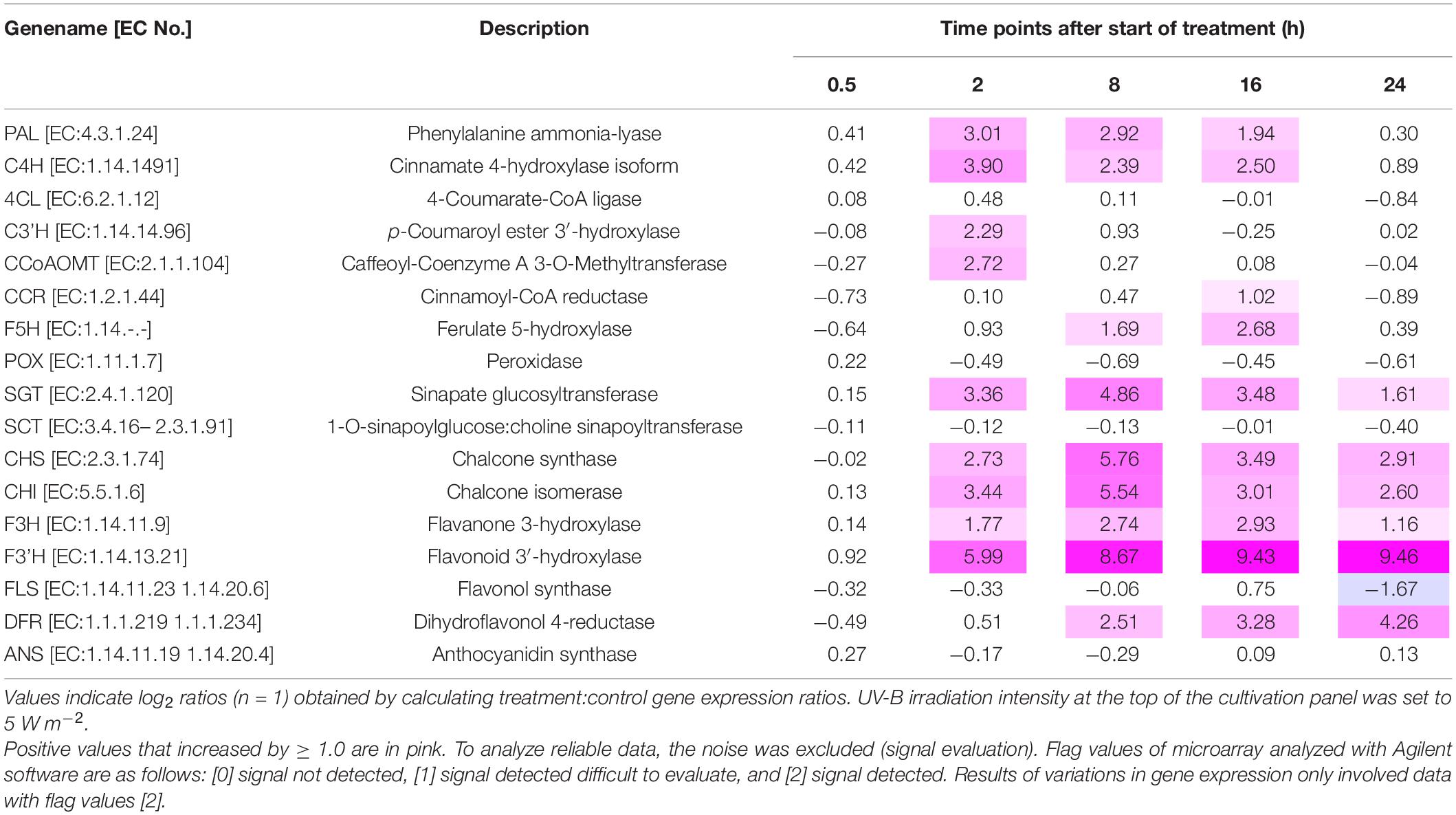
Table 1. Gene expression variation related to phenylpropanoid and flavonoid biosynthesis in canola subjected to UV-B irradiation.
The expression levels of the genes encoding secondary metabolites and the shikimate pathway were analyzed (Supplementary Figure 3). Relative gene expression varied with UV-B irradiation intensity and exposure time. The PAL and C4H expression levels reached maxima after 2 h UV-B treatment (Figure 5). PAL and C4H encode the first and second key enzymes in the phenylpropanoid pathway. In the plants subjected to 7 W m–2 UV-B irradiation, PAL and C4H expression levels were downregulated at a slower rate than in the plants subjected to the other UV-B irradiation intensity levels. The trend in 4CL expression in response to UV-B irradiation resembled those for PAL, C4H, and F5H expression, which peaked after 5 h UV-B treatment and increased once again after 16 h UV-B exposure. The plants exposed to 7 W m–2 UV-B irradiation maintained high F5H expression levels between 5 and 12 h UV-B exposure. CHS expression reached maxima at 5 h (3 W m–2) and 8 h (5 and 7 W m–2) UV-B treatment and decreased thereafter. CHI expression reached a peak after 2 h at all the UV-B irradiation intensity levels and decreased thereafter. F3H expression reached a peak at 5 h after UV-B treatment and decreased thereafter. F3’H expression reached a maximum after 5 h UV-B irradiation and remained at high levels between 5 and 12 h UV-B treatment. FLS expression reached peaks at 2 h (5 and 7 W m–2) and 5 h (3 W m–2) UV-B exposure and decreased thereafter. DFR expression reached a maximum at 5 h UV-B exposure and decreased to a minimum after 16 h. In the plants subjected to 5 and 7 W m–2 UV-B irradiation, DFR expression reached maxima after 5 h UV-B treatment and decreased thereafter. ANS expression showed a trend similar to that of DFR only until 5 h UV-B treatment. At all UV-B irradiation intensities, ANS expression was dramatically downregulated after 8 h and was upregulated thereafter.
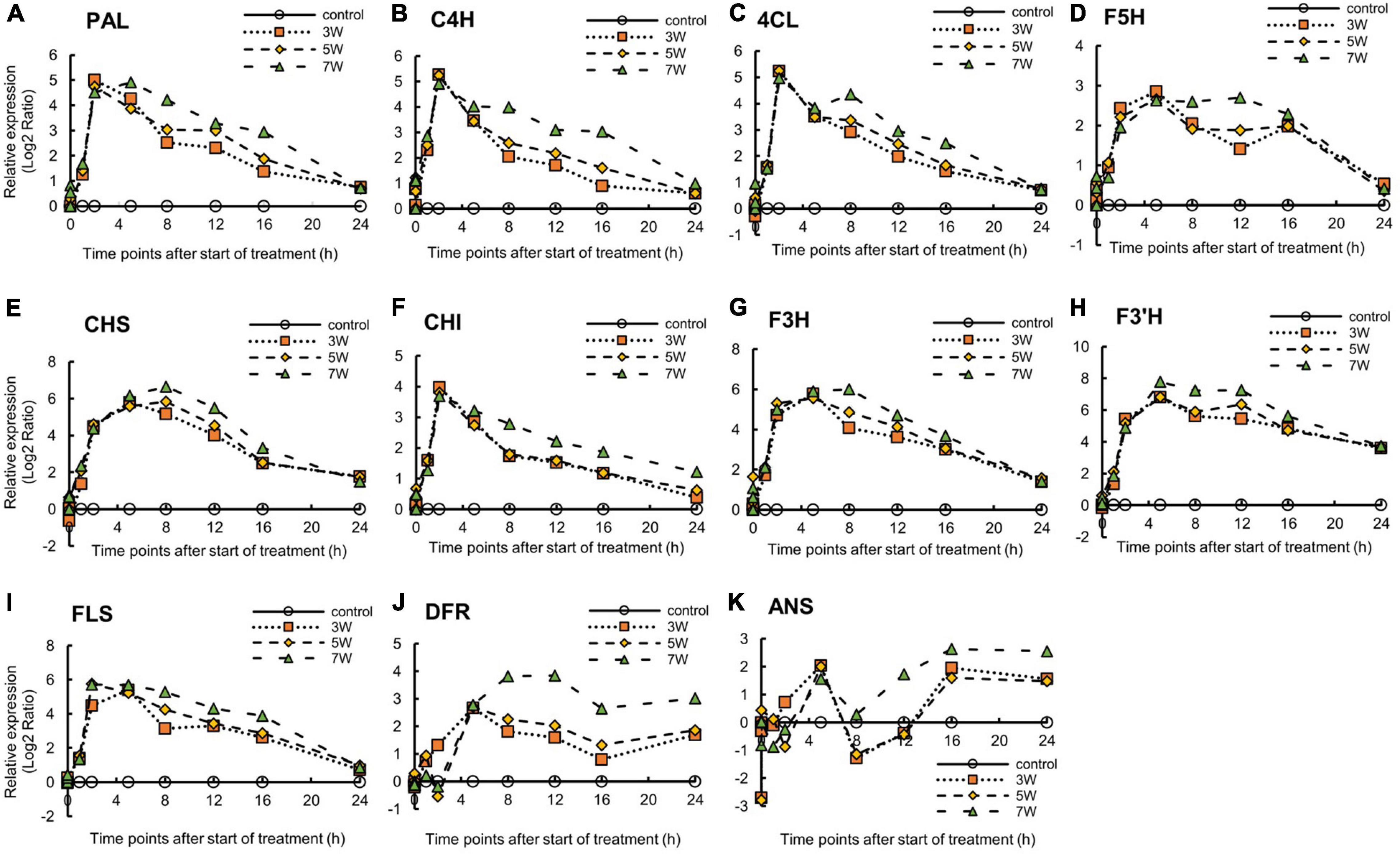
Figure 5. Time courses of PAL (A), C4H (B), 4CL (C), F5H (D), CHS (E), CHI (F), F3H (G), F3’H (H), FLS (I), DFR (J), and ANS (K) mRNA expression in canola (n = 4). Third leaves from the bottom of each canola plant were subjected to various UV-B irradiation intensities. UV-B irradiation intensities at top of the cultivation panel were set to 3, 5, and 7 W m–2. Line graphs indicate log2 fold changes (treatment:control) in gene expression levels.
Expression of Genes Related to Photosynthesis in Response to UV-B Radiation
Figure 6 shows the log2 ratios of the expression levels of the photosynthesis-related genes, namely Lhcb1, rbcL, and rbcS, within 24 h of UV-B irradiation. Relative Lhcb1 expression was reduced under all UV-B treatments. However, the timing of the decrease in Lhcb1 expression varied with UV-B irradiation intensity. Nevertheless, all UV-B irradiation intensities lowered gene photosynthesis-related gene expression after 8 h UV-B treatment. Then, Lhcb1 expression was upregulated after 16 h UV-B exposure.
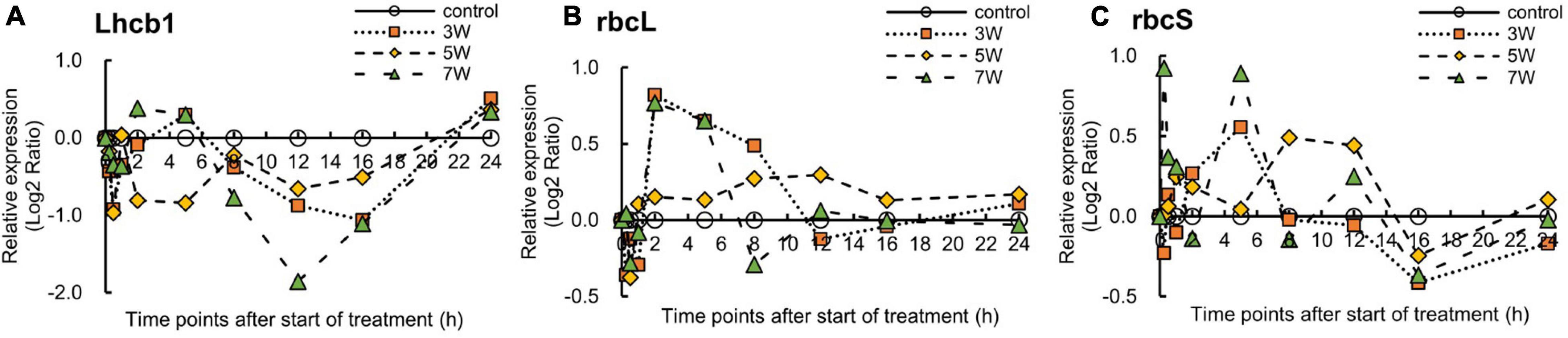
Figure 6. Time courses of (A) Lhcb1, (B) rbcL, and (C) rbcS mRNA expression in canola (n = 4). Third leaves from the bottom of each canola plant were subjected to various UV-B irradiation intensities. UV-B irradiation intensities at top of the cultivation panel were set to 3, 5, and 7 W m–2. Line graphs indicate log2 fold changes (treatment:control) in gene expression levels.
The rbcL expression levels varied with UV-B irradiation intensity. For 3 and 7 W m–2 UV-B irradiation treatments, rbcL expression was rapidly upregulated after 2 h, downregulated after 8 h, and showed expression levels similar to those of the control thereafter.
The rbcS expression levels also varied with UV-B irradiation intensity. For 3 and 7 W m–2 treatments, rbcS expression was rapidly upregulated after 4 h and downregulated after 8 h. Under the 5 W m–2 UV-B treatment, however, rbcS expression was immediately upregulated at 8 h and downregulated thereafter. Nevertheless, the variations in the expression levels did not significantly differ among photosynthesis-related genes (data not shown).
Discussion
Effect of UV-B Irradiation on Growth
The growth of the canola plants was not significantly affected by short-term (24 h) UV-B treatment (Supplementary Figure 1). Ravindran et al. (2010) reported that UV-B exposure for 2 days (20 W m–2, 2 h per day; daily dose 144 kJ m–2) did not affect the growth parameters (shoot length, fresh weight, dry weight, or leaf area) of Indigofera tinctoria, whereas 4-day UV-B irradiation reduced growth. When tobacco was subjected to various UV-B irradiation doses (0, 37, 740, 1,480, and 2,960 J m–2) for 4 days, cell proliferation significantly declined. Cell death was also significantly induced after 4 days of UV-B irradiation at all intensities (Takahashi et al., 2015). These results indicate that exposure to 3, 5, and 7 W m–2 UV-B irradiation for 24 h did not adversely affect canola plant growth. These treatments were not intense enough to cause permanent oxidative damage to the plants. However, long-term UV-B treatment may adversely affect plant growth, and the responses to it may vary with plant species, developmental stage, and leaf thickness (Piluzza and Bullitta, 2011; Wang et al., 2018).
Effects of UV-B Irradiation on Bioactive Compounds and Hydrogen Peroxide
High-intensity UV light can damage DNA, proteins, and the photosynthetic apparatus, including the chloroplasts. These injuries can adversely affect plant growth and development (Diffey, 1991). However, low-intensity UV irradiation may induce various protective mechanisms in plants, including the accumulation of low-molecular-weight compounds, such as antioxidants that suppress oxidative damage and maintain redox homeostasis (Bharti and Khurana, 1997). UV-B irradiation can induce the biosynthesis of phenylpropanoids and flavonoids that absorb UV (Jenkins, 2009; Robson et al., 2015). In the leaf epidermis, phenolics reduce oxidative damage and protect the photosynthetic apparatus by inhibiting the penetration of UV-B irradiation into the inner photosynthetic layers (Agati and Tattini, 2010).
Here, short-term UV-B irradiation at 3, 5, and 7 W m–2 activated secondary metabolite biosynthesis pathways and promoted antioxidant accumulation in canola plants (Figures 2, 3). The concentrations of secondary metabolites, such as total phenolics, antioxidants, total flavonoids, anthocyanins, and H2O2, significantly increased in response to all UV-B exposure levels (Figures 2, 3). These results suggested that the stems and leaves of canola plants turned red because the concentration of anthocyanins was significantly increased by UV-B irradiation; thus, anthocyanin expression may be associated with higher UV-B irradiation levels (Figure 2D). Furthermore, the results of total phenolic and antioxidant capacity showed almost similar trends, which agreed with results of previous studies that showed a positive linear relation between total phenolic content and antioxidant capacity (ABTS and DPPH) (Javanmardi et al., 2003; Pennycooke et al., 2005; Piluzza and Bullitta, 2011).
Based on the homeostasis between ROS and antioxidants, ROS is continuously generated in different cellular compartments as by-products of various metabolic pathways, such as plant respiration and photosynthesis, even under normal conditions (Berni et al., 2019). A constant adjustment of ROS concentration is achieved by non-enzymatic secondary metabolites such as CAT, APX, GPX, and GR; therefore, plants have a normal metabolism (Kapoor et al., 2015). However, when plants were subjected to a stressful environment (biotic and abiotic), an imbalance between ROS and antioxidants occurs, resulting in a rapid increase in ROS concentration and inducing irreversible oxidative processes such as cell death by “oxidative burst” (Sharma et al., 2012). However, the appropriate concentration of ROS acts as a signaling molecule participating in important developmental and physiological processes and responds to highly generated ROS by generating secondary metabolites such as polyphenol, terpene, and vitamins (Sharma et al., 2012; Xia et al., 2015). In particular, H2O2 plays a central role in signaling pathways because of its relatively long lifetime and can freely diffuse across membranes through aquaporins (Bienert et al., 2007; Møller et al., 2007). Moreover, at the appropriate levels, ROS may act as signaling molecules in various intracellular processes and induce antioxidant biosynthesis.
In this study, the concentration of H2O2 showed a tendency to increase as energy increased, but all UV treatments did not significantly affect plant growth and morphological changes. Therefore, the amount of ROS generated was not high enough to inhibit canola growth and was within the appropriate range. The observed increases in the bioactive compound content at 3, 5, and 7 W m–2 UV-B irradiation indicated that these radiation levels were within a suitable range to stimulate antioxidant biosynthesis in canola. Previous studies reported that the UV levels they used increased the bioactive compound content in plants (Lois, 1994). Arabidopsis plants were exposed to various levels of UV-B irradiation intensity (0.10, 0.15, 0.24, 0.37, and 0.8 W m–2) for 30 h and flavonoid accumulation was the highest at 0.15 W m–2 UV-B irradiation (Lois, 1994). However, exposure to high UV levels may actually decrease the bioactive compound content by impairing cellular function. Similar results were obtained for sweet basil leaves exposed to various levels of UV-B irradiation intensity (0, 2.3, 3.6, and 4.8 W m–2) (Ghasemzadeh et al., 2016). The concentrations of total phenolics, total flavonoids, and individual flavonoids, and phenolic acids were the highest at 3.6 W m–2 UV-B irradiation but significantly declined at 4.8 W m–2 UV-B irradiation. In Medicago sativa, antioxidant and flavonoid compounds significantly increased in response to lower UV-B irradiation levels (17.35 μW cm–2 d–1), whereas higher UV-B irradiation levels caused severe damage and adversely affected growth and development (Gao et al., 2019). The foregoing results suggest that, below certain UV exposure thresholds, the content of bioactive compounds, such as flavonoids, may be proportional to the UV dose. In this study, there is a possibility that 7 W m–2 UV-B irradiation was the optimal UV-B exposure level, whereas 10 W m–2 reduced the bioactive compound content in canola.
Effects of UV-B Irradiation on Expression of the Secondary Metabolites Biosynthesis-Related Genes
The expression of the genes encoding antistress and antioxidant compounds is regulated by the UVR8 pathway (Hideg et al., 2013). The cytoplasmic UVR8 photoreceptor is an active dimer and it becomes a monomer during UV-B absorption. UV-B-induced UVR8 photoreceptor monomers directly reacted with E3 ubiquitin ligase “constitutively photomorphogenic 1” (COP1) and promoted “elongated hypocotyl 5” (HY5) transcription in Arabidopsis nuclei (Brown et al., 2005; Favory et al., 2009; Wu et al., 2012). In Arabidopsis, the accumulation of HY5 transcripts promoted flavonoid biosynthesis by activating chalcone synthase (CHS) and prevented UV-B absorption by epidermal tissue (Bharti and Khurana, 1997; Kliebenstein et al., 2002; Brown and Jenkins, 2008). Photomorphogenic responses, such as an increase in leaf thickness, axillary branching, and the induction of UV-absorbing compounds, are mediated by the activation of UV-B photoreceptor UV “resistance locus 8” (UVR8) in response to low-to-moderate UV-B irradiation levels (0.1-21 μmol m–2 s–1 and 1.77-1.07 W m–2) (Hectors et al., 2007; Brown and Jenkins, 2008; Rizzini et al., 2011). COP1 and HY5 transcription were induced in Arabidopsis by low UV-B flux rates (0.1 μmol m–2 s–1) (Brown and Jenkins, 2008). In this study, the expression levels of COP1 and HY5 were upregulated immediately after UV-B exposure and attained the highest expression levels after 2 h. UV-B-absorbing compounds were also affected by UVR8 pathway activation and accumulated in the canola plants (Figure 2). However, high UV-B irradiation levels may induce ROS biosynthesis, which damages DNA, proteins, membranes, and so on, and impedes plant growth and development (Brown and Jenkins, 2008; Hideg et al., 2013). Here, the UV-exposed plants were not harmed, and their bioactive compound content had significantly increased. These results suggest that the canola plants were subjected to only low-to-moderate levels of UV-B irradiation.
The genes PAL, C4H, 4CL, F5H, CHS, CHI, F3H, F3’H, FLS, DFR, and ANS are key genes in the phenylpropanoid and flavonoid biosynthesis pathways. PAL leads to the main bifurcation in phenylpropanoid metabolism and is an upstream gene. F3’H, DFR, and ANS are downstream genes in flavonoid biosynthesis. The times to peak expression differed among genes; genes with downregulated expression required a long time to reach maximum expression (Figure 5); however, with variable gene expression (Table 1), the trends in upregulation were like those encoding phenylpropanoids (PAL, C4H, and F5H) and flavonoids (CHS, CHI, F3H, F3’H, and DFR) according to qRT-PCR (Figure 5). When Chrysanthemum morifolium was exposed to UV-B irradiation for different durations, HY5 expression was rapidly upregulated after 1 h, whereas the expression levels of CHS, CHI, F3H, and FLS increased after 6 h (Yang et al., 2018). Similar trends were reported in a previous study analyzing CHS, CHI, F3H, DFR, and ANS expression in radish sprouts (Su et al., 2016). CHS, CHI, and F3H are located upstream in the flavonoid biosynthesis pathway, and their expression levels were upregulated after 12 h UV-B exposure. However, the expression levels of downstream DFR and ANS were upregulated after 24 h UV-B irradiation. Moreover, the expression levels of phenylpropanoid and flavonoid-related genes were consistently upregulated at 7 W m–2 UV-B irradiation. At 7 W m–2 UV-B irradiation, gene expression levels decreased at a slower rate than at 3 and 5 W m–2 UV-B irradiation. Therefore, 7 W m–2 UV-B irradiation stimulated gene expression more effectively than 3 or 5 W m–2, regardless of the duration of UV-B exposure.
Microarray analysis was used for the whole-genome exploration of gene expression profiles in canola plants. Quantitative RT-PCR results supported the microarray data. In the microarray analysis, many DEGs, down- or upregulated, were observed during 24 h of UV-B irradiation (Supplementary Figure 2). These genes identified in this study have been implicated in secondary metabolite syntheses (Table 1). The DEGs were significantly upregulated at the 0.5 and 2 h of UV-B exposure relative to the other time point. The significantly upregulated DEGs with unknown genes were from the 0.5 and 2 h of UV-B irradiation. These results suggested that DEGs were involved in the complex molecular mechanisms necessary for resistance to UV irradiation in canola plants. In addition, even in the quantitative RT-PCR results (Figures 4, 5), genes of the UVR8 pathway and the upper group of the secondary metabolite rapidly increased after 2 h of UV exposure. The DEGs results indicated that numerous genes in UV-B treated plants have undergone molecular biological changes on 0.5 and 2 h of UV-B irradiation. Even at 0.5 h, the number of upregulated genes was large. It is possible that unanalyzed (or unconfirmed) genes were expressed and ultimately led to upregulation.
The time points at which the expression levels of genes were upregulated varied with plant species. UV-B irradiation induced genes related to phenylpropanoid and flavonoid biosynthesis, which increased polyphenol antioxidant content. The results of the gene expression analyses were consistent with those for the characteristics of the bioactive compounds (Figure 2). The observed increases in the bioactive compound content in plants subjected to 3, 5, and 7 W m–2 UV-B irradiation indicated that all UV-B exposure levels were within a range suitable to stimulate antioxidant phenolic compound biosynthesis in canola. All UV-B treatments increased the production of these bioactive compounds by upregulating the expression levels of several key genes in the biosynthetic pathways for these compounds. However, there were significant time lags between the observed increases in the levels of these compounds and upregulation of the expression levels of genes encoding them. The expression levels of PAL, C4H, and 4CL were dramatically increased after 2 h UV-B irradiation. The relative expression of F5H reached a peak after 5 h UV-B irradiation (Figure 5). The total phenolic concentration coincided with the peaks in F5H expression and significantly increased, starting at 5 h UV-B irradiation (Figure 2A). The flavonoid biosynthesis genes (CHS, F3H, F3’H, and FLS) were induced in response to HY5 transcript accumulation following UV-B irradiation, and their peak expression levels occurred after 5-8 h UV-B irradiation. The expression levels of ANS and DFR reached their maxima at 12-16 h UV-B treatment. According to a previous report, some genes involved in the biosynthesis of the phenylpropanoid pathway are not expressed continuously when exposed to UV-B irradiation, but genes are expressed rapidly for a short period and then return to the basal level (Höll et al., 2019; Meyer et al., 2021). Our results also showed a tendency to decrease to the basal level after each gene expression peaked.
Total flavonoid concentration and relative anthocyanin content increased at 5-8 h and 12-16 h UV-B irradiation, respectively (Figure 2). Regardless of the time points at which the expression levels of the gene reached their maxima, the bioactive compound content continued to rise. These reactions may have been defense responses in anticipation of future exposure to the same abiotic stress. If UV irradiation is interrupted, the plant may use its “memory capacity” to continue increasing the bioactive compound content (Bruce et al., 2007). Time differences between gene expression and bioactive compound biosynthesis were reported for wheat leaves under drought stress (Ma et al., 2014). Their CHS, CHI, F3H, FLS, DFR, and ANS expression levels reached their maxima at 12-16 h after UV. Timely application of short-term, moderate-intensity UV-B irradiation to leafy vegetables 1-2 days preharvest might enhance antioxidant phytochemical production without yield or quality loss in horticultural crops raised in plant factories and vertical farms under artificial light. Thus, bioactive compound biosynthesis might have continued to increase in canola even after the 24-h UV-B treatment.
Effects of UV-B Irradiation on Expression of the Photosynthetic Metabolites Biosynthesis-Related Genes
The photosynthesis-related genes Lhcb1, rbcL, and rbcS were differentially expressed throughout the entire UV-B irradiation period (Figure 6). The Lhcb1, rbcL, and rbcS expression levels in pea plants were reduced by UV-B treatment (Strid et al., 1994). Lhcb1 encodes the chlorophyll a/b-binding protein of the photosystem (PS) - light-harvesting antenna complex and maintains the photosynthetic apparatus (Andersson et al., 2003). Lhcb1 also modulates stomatal movement and promotes plant stress tolerance (Xu et al., 2012). In this study, Lhcb1 expression was rapidly downregulated under all UV-B treatments after 8 h and upregulated after 24 h UV-B treatment (Figure 6A). These results suggest that short-term UV-B exposure had a negative effect on the photosynthetic machinery. Kalbina and Strid (2006) reported that Lhcb1 mRNA expression was reduced in Arabidopsis by short-term UV-B irradiation. O2⋅– and H2O2 are secondary messengers involved in the downregulation of the expression of photosynthetic genes, such as Lhcb (Kalbina and Strid, 2006). Hence, the generated ROS might have downregulated Lhcb1 expression in this study.
The CO2 fixation is achieved through photosynthesis, and the enzyme Rubisco is involved in this complex process. It was reported that this highly sensitive mechanism may be inhibited by UV-B irradiation (Fedina et al., 2010). The rbcS (Rubisco small subunit) and rbcL (Rubisco large subunit) proteins are susceptible to degradation. However, the expression levels of rbcS and rbcL are upregulated to compensate for the damage caused to their protein products by oxidative stress (Xiong et al., 2010). Here, rbcS expression was upregulated after 2 h and downregulated after 8 h UV-B treatment (Figure 6C); however, rbcL expression was upregulated after 4 h and downregulated after 16 h UV-B treatment. The times of peak expression differed slightly between rbcS and rbcL. Nevertheless, the rbcS and rbcL protein levels were drastically downregulated by UV-B treatment. It is therefore possible that rbcS and rbcL mRNA levels were rapidly upregulated to regenerate the lost photosynthetic proteins (Xiong et al., 2010). Enhanced gene transcription might compensate for ROS-mediated protein damage/loss and help maintain the photosynthetic machinery subjected to UV-B irradiation. Increased rbcS and rbcL transcription have also been reported to cause drought and salinity stress, which promote oxidative stress (Lilley et al., 1996; Fu et al., 2007). Thus, rbcS and rbcL expression levels were rapidly upregulated to compensate for the decreases in photosynthetic protein content caused by UV-B exposure. Nevertheless, prolonged UV-B exposure might delay or even suppress upregulation of rbcS and rbcL expression.
In canola, short-term UV-B irradiation downregulated the expression of genes implicated in photosynthesis but did not adversely affect plant growth (Supplementary Figure 1). However, if UV-B irradiation continues for > 2 days, plant growth would significantly decrease.
Conclusion
This study demonstrated that the genes regulating secondary metabolite biosynthesis in canola were affected by UV-B irradiation intensity and duration. UV-B irradiation for ≤ 24 h was merely a mild stressor for canola and did not damage its photosynthetic machinery or hinder its growth. To minimize UV-B-induced damage, phenylpropanoid and flavonoid biosynthesis were rapidly activated, and antioxidant phytochemicals accumulated. The expression levels of the genes governing targeted secondary metabolic pathways significantly differed with UV-B exposure duration. There were also temporal differences between gene expression and bioactive compound accumulation. The concentrations of all antioxidants increased in response to the peak expression levels of the genes regulating the phenylpropanoid and flavonoid pathways. The plants subjected to 7 W m–2 UV-B irradiation showed 1. 82-, 1. 75-, and 11.21-fold increase in total phenolic, flavonoid, and anthocyanin concentrations, respectively, compared with the unexposed controls because of the inherent “memory mechanism” of the plant. To the best of our knowledge, this work is the first to demonstrate a temporal difference between the accumulation of antioxidants and the induction of the genes encoding them in UV-B-treated canola plants. Based on the discoveries in this work, timely application of short-term, moderate-intensity UV-B irradiation to canola and other leafy vegetables at 1-2 days preharvest might enhance the biosynthesis of health-promoting antioxidants without compromising crop yield or quality.
As biosynthesis and accumulation of bioactive compounds are regulated by complex temporal and spatial patterns, more in-depth studies are needed on temporal differences between gene expression and target bioactive compounds’ accumulation. Short-term UV irradiation could be a widely used technique for controlling the activation timing of target compounds in plant factories and vertical farms.
Data Availability Statement
The datasets presented in this study can be found in online repositories. The names of the repository/repositories and accession number(s) can be found at: https://www.ebi.ac.uk/arrayexpress/E-MTAB-11034.
Author Contributions
J-HL and SS: performance of experiments, sample collection, and analyses of chemical data. J-HL: writing – original draft preparation. EG: writing – review and editing, conceptualization, experimental design, supervision, and funding acquisition. All authors read and agreed to the final version of the manuscript.
Funding
This work was supported by the Ministry of Economy, Trade, and Industry of Japan in the form of a grant-in-aid from the project “Development of Fundamental Technologies for the Production of High-Value Materials Using Transgenic Plants.”
Conflict of Interest
The authors declare that the research was conducted in the absence of any commercial or financial relationships that could be construed as a potential conflict of interest.
Publisher’s Note
All claims expressed in this article are solely those of the authors and do not necessarily represent those of their affiliated organizations, or those of the publisher, the editors and the reviewers. Any product that may be evaluated in this article, or claim that may be made by its manufacturer, is not guaranteed or endorsed by the publisher.
Supplementary Material
The Supplementary Material for this article can be found online at: https://www.frontiersin.org/articles/10.3389/fpls.2021.786555/full#supplementary-material
References
Agati, G., and Tattini, M. (2010). Multiple functional roles of flavonoids in photoprotection. New Phytol. 186, 786–793. doi: 10.1111/j.1469-8137.2010.03269.x
Ainsworth, E. A., and Gillespie, K. M. (2007). Estimation of total phenolic content and other oxidation substrates in plant tissues using folin–ciocalteu reagent. Nat. Protoc. 2, 875–877. doi: 10.1038/nprot.2007.102
Allen, D. J., Nogués, S., and Baker, N. R. (1998). Ozone depletion and increased UV-B radiation: is there a real threat to photosynthesis? J. Exp. Bot. 49, 1775–1788. doi: 10.1093/jxb/49.328.1775
Andersson, J., Wentworth, M., Walters, R. G., Howard, C. A., Ruban, A. V., Horton, P., et al. (2003). Absence of the Lhcb1 and Lhcb2 proteins of the light-harvesting complex of the photosystem II—effects on photosynthesis, grana stacking and fitness. Plant J. 35, 350–361. doi: 10.1046/j.1365-313X.2003.01811.x
Berni, R., Luyckx, M., Xu, X., Legay, S., Sergeant, K., Hausman, J. F., et al. (2019). Reactive oxygen species and heavy metal stress in plants: impact on the cell wall and secondary metabolism. Environ. Exp. Bot. 161, 98–106. doi: 10.1016/j.envexpbot.2018.10.017
Bharti, A. K., and Khurana, J. P. (1997). Mutants of Arabidopsis as tools to understand the regulation of phenylpropanoid pathway and UVB protection mechanisms. Photochem. Photobiol. 65, 765–776. doi: 10.1111/j.1751-1097.1997.tb01923.x
Bienert, G. P., Møller, A. L., Kristiansen, K. A., Schulz, A., Møller, I. M., Schjoerring, J. K., et al. (2007). Specific aquaporins facilitate the diffusion of hydrogen peroxide across membranes. J. Biol. Chem. 282, 1183–1192. doi: 10.1074/jbc.M603761200
Brown, B. A., and Jenkins, G. I. (2008). UV-B signaling pathways with different fluence-rate response profiles are distinguished in mature Arabidopsis leaf tissue by requirement for UVR8, HY5, and HYH. Plant Physiol. 146, 576–588. doi: 10.1104/pp.107.108456
Brown, B. A., Cloix, C., Jiang, G. H., Kaiserli, E., Herzyk, P., Kliebenstein, D. J., et al. (2005). A UV-B-specific signaling component orchestrates plant UV protection. Proc. Natl Acad. Sci. U.S.A. 102, 18225–18230. doi: 10.1073/pnas.0507187102
Bruce, J. A. T., Matthes, C. M., Napier, A. J., and Pickett, A. J. (2007). Stressful ‘memories’ of plants: evidence and possible mechanisms. Plant Sci. 173, 603–608. doi: 10.1016/j.plantsci.2007.09.002
Chang, X., Alderson, P. G., and Wright, C. J. (2009). Enhanced UV-B radiation alters basil (Ocimum basilicum L.) growth and stimulates the synthesis of volatile oils. J. Hortic. For. 1, 27–031.
Diffey, B. L. (1991). Solar ultraviolet radiation effects on biological systems. Phys. Med. Biol. 36, 299–328. doi: 10.1088/0031-9155/36/3/001
Escobar, A. L., Alberdi, M., Acevedo, P., Machado, M., Nunes-Nesi, A., Inostroza-Blancheteau, C., et al. (2017). Distinct physiological and metabolic reprogramming by highbush blueberry (Vaccinium corymbosum) cultivars revealed during long-term UV-B radiation. Physiol. Plant. 160, 46–64. doi: 10.1111/ppl.12536
Favory, J. J., Stec, A., Gruber, H., Rizzini, L., Oravecz, A., Funk, M., et al. (2009). Interaction of COP1 and UVR8 regulates UV-B-induced photomorphogenesis and stress acclimation in Arabidopsis. EMBO J. 28, 591–601. doi: 10.1038/emboj.2009.4
Fedina, I., Hidema, J., Velitchkova, M., Georgieva, K., and Nedeva, D. (2010). UV-B induced stress responses in three rice cultivars. Biol. Plant. 54, 571–574. doi: 10.1007/s10535-010-0102-3
Flint, S. D., and Caldwell, M. M. (1984). Partial inhibition of in vitro pollen germination by simulated solar ultraviolet-B radiation. Ecology 65, 792–795. doi: 10.2307/1938051
Fu, B. Y., Xiong, J. H., Zhu, L. H., Zhao, X. Q., Xu, H. X., Gao, Y. M., et al. (2007). Identification of functional candidate genes for drought tolerance in rice. Mol. Genet. Genomics 278, 599–609. doi: 10.1007/s00438-007-0276-3
Gao, L., Liu, Y., Wang, X., Li, Y., and Han, R. (2019). Lower levels of UV-B light trigger the adaptive responses by inducing plant antioxidant metabolism and flavonoid biosynthesis in Medicago sativa seedlings. Funct. Plant Biol. 46, 896–906. doi: 10.1071/FP19007
Ghasemzadeh, A., Ashkani, S., Baghdadi, A., Pazoki, A., Jaafar, H. Z., and Rahmat, A. (2016). Improvement in flavonoids and phenolic acids production and pharmaceutical quality of sweet basil (Ocimum basilicum L.) by ultraviolet-B irradiation. Molecules 21:1203. doi: 10.3390/molecules21091203
Goto, E. (2011). Production of pharmaceutical materials using genetically modified plants grown under artificial lighting. Acta Hortic. 907, 45–52. doi: 10.17660/ActaHortic.2011.907.3
Goto, E., Ide, M., Saito, Y., and Hikosaka, S. (2020). Enhancement of gene expression related to phytochemical accumulation in plant leaves via exposure to environmental stresses in a plant factory. Acta Hortic. 1296, 265–272. doi: 10.17660/ActaHortic.2020.1296.34
Hectors, K., Prinsen, E., De Coen, W., Jansen, M. A. K., and Guisez, Y. (2007). Arabidopsis thaliana plants acclimated to low dose rates of ultraviolet B radiation show specific changes in morphology and gene expression in the absence of stress symptoms. New Phytol. 175, 255–270. doi: 10.1111/j.1469-8137.2007.02092.x
Hideg, E., Jansen, M. A., and Strid, A. (2013). UV-B exposure, ROS, and stress: inseparable companions or loosely linked associates? Trends Plant Sci. 18, 107–115. doi: 10.1016/j.tplants.2012.09.003
Höll, J., Lindner, S., Walter, H., Joshi, D., Poschet, G., Pfleger, S., et al. (2019). Impact of pulsed UV-B stress exposure on plant performance: how recovery periods stimulate secondary metabolism while reducing adaptive growth attenuation. Plant Cell Environ. 42, 801–814. doi: 10.1111/pce.13409
Inostroza-Blancheteau, C., Acevedo, P., Loyola, R., Arce-Johnson, P., Alberdi, M., and Reyes-Díaz, M. (2016). Short-term UV-B radiation affects photosynthetic performance and antioxidant gene expression in highbush blueberry leaves. Plant Physiol. Biochem. 107, 301–309. doi: 10.1016/j.plaphy.2016.06.019
Javanmardi, J., Stushnoff, C., Locke, E., and Vivanco, J. M. (2003). Antioxidant activity and total phenolic content of Iranian Ocimum accessions. Food Chem. 83, 547–550. doi: 10.1016/S0308-8146(03)00151-1
Jenkins, G. I. (2009). Signal transduction in responses to UV-B radiation. Annu. Rev. Plant Biol. 60, 407–431. doi: 10.1146/annurev.arplant.59.032607.092953
Kalbina, I., and Strid, A. (2006). The role of NADPH oxidase and MAP kinase phosphatase in UV-B-dependent gene expression in Arabidopsis. Plant Cell Environ. 29, 1783–1793. doi: 10.1111/j.1365-3040.2006.01555.x
Kapoor, D., Sharma, R., Handa, N., Kaur, H., Rattan, A., Yadav, P., et al. (2015). Redox homeostasis in plants under abiotic stress: role of electron carriers, energy metabolism mediators and proteinaceous thiols. Front. Environ. Sci. 3:13. doi: 10.3389/fenvs.2015.00013
Kataria, S., Jajoo, A., and Guruprasad, K. N. (2014). Impact of increasing ultraviolet-B (UV-B) radiation on photosynthetic processes. J. Photochem. Photobiol. B 137, 55–66. doi: 10.1016/j.jphotobiol.2014.02.004
Kliebenstein, D. J., Lim, J. E., Landry, L. G., and Last, R. L. (2002). Arabidopsis UVR8 regulates ultraviolet-B signal transduction and tolerance and contains sequence similarity to human Regulator of Chromatin Condensation 1. Plant Physiol. 130, 234–243. doi: 10.1104/pp.005041
Lee, J.-H., and Oh, M.-M. (2015). Short-term low temperature increases phenolic antioxidant levels in kale. Hortic. Environ. Biotechnol. 56, 588–596. doi: 10.1007/s13580-015-0056-7
Lilley, J. M., Ludlow, M. M., McCouch, S. R., and O’Toole, J. C. (1996). Locating QTL for osmotic adjustment and dehydration tolerance in rice. J. Exp. Bot. 47, 1427–1436. doi: 10.1093/jxb/47.9.1427
Lois, R. (1994). Accumulation of UV-absorbing flavonoids induced by UV-B radiation in Arabidopsis thaliana L. Planta 194, 498–503. doi: 10.1007/BF00714462
Ma, D., Sun, D., Wang, C., Li, Y., and Guo, T. (2014). Expression of flavonoid biosynthesis genes and accumulation of flavonoid in wheat leaves in response to drought stress. Plant Physiol. Biochem. 80, 60–66. doi: 10.1016/j.plaphy.2014.03.024
Madronich, S., McKenzie, R. L., Björn, L. O., and Caldwell, M. M. (1998). Changes in biologically active ultraviolet radiation reaching the Earth’s surface. J. Photochem. Photobiol. B 46, 5–19. doi: 10.1016/S1011-1344(98)00182-1
Mancinelli, A. L., and Schwartz, O. M. (1984). The photoregulation of anthocyanin synthesis IX. The photosensitivity of the response in dark and light-grown tomato seedlings. Plant Cell Physiol. 25, 93–105.
Meyer, P., Van de Poel, B., and De Coninck, B. (2021). UV-B light and its application potential to reduce disease and pest incidence in crops. Hortic. Res. 8, 1–20. doi: 10.1038/s41438-021-00629-5
Miller, N. J., and Rice-Evans, C. A. (1996). Spectrophotometric determination of antioxidant activity. Redox Rep. 2, 161–171. doi: 10.1080/13510002.1996.11747044
Møller, I. M., Jensen, P. E., and Hansson, A. (2007). Oxidative modifications to cellular components in plants. Annu. Rev. Plant. Biol. 58, 459–481. doi: 10.1146/annurev.arplant.58.032806.103946
Neugart, S., and Bumke-Vogt, C. (2021). Flavonoid glycosides in Brassica species respond to UV-B depending on exposure time and adaptation time. Molecules 26:494. doi: 10.3390/molecules26020494
Neugart, S., Fiol, M., Schreiner, M., Rohn, S., Zrenner, R., Kroh, L. W., et al. (2014). Interaction of moderate UV-B exposure and temperature on the formation of structurally different flavonol glycosides and hydroxycinnamic acid derivatives in kale (Brassica oleracea var sabellica). J. Agric. Food Chem. 62, 4054–4062. doi: 10.1021/jf4054066
Pandey, N., and Pandey-Rai, S. (2014). Short term UV-B radiation-mediated transcriptional responses and altered secondary metabolism of in vitro propagated plantlets of Artemisia annua L. Plant Cell Tissue Organ Cult. 116, 371–385. doi: 10.1007/s11240-013-0413-0
Pennycooke, J. C., Cox, S., and Stushnoff, C. (2005). Relationship of cold acclimation, total phenolic content and antioxidant capacity with chilling tolerance in petunia (Petunia× hybrida). Environ. Exp. Bot. 53, 225–232. doi: 10.1016/j.envexpbot.2004.04.002
Piluzza, G., and Bullitta, S. (2011). Correlations between phenolic content and antioxidant properties in twenty-four plant species of traditional ethnoveterinary use in the Mediterranean area. Pharm. Biol. 49, 240–247. doi: 10.3109/13880209.2010.501083
Ravindran, K. C., Indrajith, A., Pratheesh, P. V., Sanjiviraja, K., and Balakrishnan, V. (2010). Effect of ultraviolet-B radiation on biochemical and antioxidant defence system in Indigofera tinctoria L. seedlings. Int. J. Eng. Sci. Technol. 2, 226–232. doi: 10.4314/ijest.v2i5.60154
Reddy, L., Odhav, B., and Bhoola, K. D. (2003). Natural products for cancer prevention: a global perspective. Pharmacol. Ther. 99, 1–13. doi: 10.1016/S0163-7258(03)00042-1
Rizzini, L., Favory, J. J., Cloix, C., Faggionato, D., O’Hara, A., Kaiserli, E., et al. (2011). Perception of UV-B by the Arabidopsis UVR8 protein. Science 332, 103–106. doi: 10.1126/science.1200660
Robson, T. M., Klem, K., Urban, O., and Jansen, M. A. (2015). Re-interpreting plant morphological responses to UV-B radiation. Plant Cell Environ. 38, 856–866. doi: 10.1111/pce.12374
Rozema, J., Chardonnens, A., Tosserams, M., Hafkenscheid, R., and Bruijnzeel, S. (1997). Leaf thickness and UV-B absorbing pigments of plants in relation to an elevational gradient along the Blue Mountains. Jamaica. Plant Ecol. 128, 151–159. doi: 10.1023/A:1009719109153
Scalbert, A., Andres-Lacueva, C., Arita, M., Kroon, P., Manach, C., Urpi-Sarda, M., et al. (2011). Databases on food phytochemicals and their health-promoting effects. J. Agric. Food Chem. 59, 4331–4348. doi: 10.1021/jf200591d
Sharma, P., Jha, A. B., Dubey, R. S., and Pessarakli, M. (2012). Reactive oxygen species, oxidative damage, and antioxidative defense mechanism in plants under stressful conditions. J. Bot. 26. doi: 10.1155/2012/217037
Son, K.-H., Ide, M., and Goto, E. (2020). Growth characteristics and phytochemicals of canola (Brassica napus) grown under UV radiation and low root zone temperature in a controlled environment. Hortic. Environ. Biotechnol. 61, 267–277. doi: 10.1007/s13580-019-00219-4
Strid, A., Chow, W. S., and Anderson, J. M. (1994). UV-B damage and protection at the molecular level in plants. Photosynth. Res. 39, 475–489. doi: 10.1007/BF00014600
Su, N., Lu, Y., Wu, Q., Liu, Y., Xia, Y., Xia, K., et al. (2016). UV-B-induced anthocyanin accumulation in hypocotyls of radish sprouts continues in the dark after irradiation. J. Sci. Food Agric. 96, 886–892. doi: 10.1002/jsfa.7161
Sytar, O., Zivcak, M., Bruckova, K., Brestic, M., Hemmerich, I., Rauh, C., et al. (2018). Shift in accumulation of flavonoids and phenolic acids in lettuce attributable to changes in ultraviolet radiation and temperature. Sci. Hortic. 239, 193–204. doi: 10.1016/j.scienta.2018.05.020
Takahashi, S., Kojo, K. H., Kutsuna, N., Endo, M., Toki, S., Isoda, H., et al. (2015). Differential responses to high- and low-dose ultraviolet-B stress in tobacco Bright Yellow-2 cells. Front. Plant Sci. 6:254. doi: 10.3389/fpls.2015.00254
Tevini, M., and Steinmüller, D. (1987). Influence of light, UV-B radiation, and herbicides on wax biosynthesis of cucumber seedling. J. Plant Physiol. 131, 111–121. doi: 10.1016/S0176-1617(87)80272-9
Tevini, M., Iwanzik, W., and Thoma, U. (1981). Some effects of enhanced UV-B irradiation on the growth and composition of plants. Planta 153, 388–394. doi: 10.1007/BF00384258
Velikova, V., Yordanov, I., and Edreva, A. (2000). Oxidative stress and some antioxidant systems in acid rain-treated bean plants: protective role of exogenous polyamines. Plant Sci. 151, 59–66. doi: 10.1016/S0168-9452(99)00197-1
Visioli, F., De La Lastra, C. A., Andres-Lacueva, C., Aviram, M., Calhau, C., Cassano, A., et al. (2011). Polyphenols and human health: a prospectus. Crit. Rev. Food Sci. Nutr. 51, 524–546. doi: 10.1080/10408391003698677
Wang, Y., Meng, G., Chen, S., Chen, Y., Jiang, J., and Wang, Y. P. (2018). Correlation analysis of phenolic contents and antioxidation in yellow-and black-seeded Brassica napus. Molecules 23:1815. doi: 10.3390/molecules23071815
Wargent, J. J., Moore, J. P., Roland-Ennos, A., and Paul, N. D. (2009). Ultraviolet radiation as a limiting factor in leaf expansion and development. Photochem. Photobiol. 85, 279–286. doi: 10.1111/j.1751-1097.2008.00433.x
Wu, D., Hu, Q., Yan, Z., Chen, W., Yan, C. Y., Huang, X., et al. (2012). Structural basis of ultraviolet-B perception by UVR8. Nature 484, 214–219. doi: 10.1038/nature10931
Xia, X. J., Zhou, Y. H., Shi, K., Zhou, J., Foyer, C. H., and Yu, J. Q. (2015). Interplay between reactive oxygen species and hormones in the control of plant development and stress tolerance. J. Exp. Bot. 66, 2839–2856. doi: 10.1093/jxb/erv089
Xiong, J. H., Fu, B. Y., Xu, H. X., and Li, Y. S. (2010). Proteomic analysis of PEG-simulated drought stress responsive proteins of rice leaves using a pyramiding rice line at the seedling stage. Bot. Stud. 51, 137–145.
Xu, Y. H., Liu, R., Yan, L., Liu, Z. Q., Jiang, S. C., Shen, Y. Y., et al. (2012). Light-harvesting chlorophyll a/b-binding proteins are required for stomatal response to abscisic acid in Arabidopsis. J. Exp. Bot. 63, 1095–1106. doi: 10.1093/jxb/err315
Keywords: antioxidant capacity, bioactive compounds, environmental stress, phytochemical, microarray
Citation: Lee J-H, Shibata S and Goto E (2021) Time-Course of Changes in Photosynthesis and Secondary Metabolites in Canola (Brassica napus) Under Different UV-B Irradiation Levels in a Plant Factory With Artificial Light. Front. Plant Sci. 12:786555. doi: 10.3389/fpls.2021.786555
Received: 30 September 2021; Accepted: 08 November 2021;
Published: 22 December 2021.
Edited by:
Carl-Otto Ottosen, Aarhus University, DenmarkReviewed by:
Rodrigo Loyola, Instituto de Investigaciones Agropecuarias (INIA), ChileOksana Sytar, Taras Shevchenko National University of Kyiv, Ukraine
Copyright © 2021 Lee, Shibata and Goto. This is an open-access article distributed under the terms of the Creative Commons Attribution License (CC BY). The use, distribution or reproduction in other forums is permitted, provided the original author(s) and the copyright owner(s) are credited and that the original publication in this journal is cited, in accordance with accepted academic practice. No use, distribution or reproduction is permitted which does not comply with these terms.
*Correspondence: Eiji Goto, Z290b0BmYWN1bHR5LmNoaWJhLXUuanA=