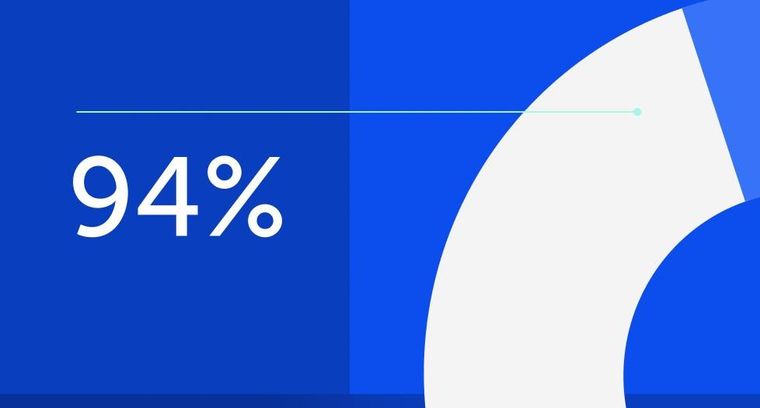
94% of researchers rate our articles as excellent or good
Learn more about the work of our research integrity team to safeguard the quality of each article we publish.
Find out more
ORIGINAL RESEARCH article
Front. Plant Sci., 11 January 2022
Sec. Plant Physiology
Volume 12 - 2021 | https://doi.org/10.3389/fpls.2021.774582
Sugar is an important carbon source and contributes significantly to the improvement of plant growth and fruit flavor quality. Sugar transport through the tonoplast is important for intracellular homeostasis and metabolic balance in plant cells. There are four tonoplast sugar transporters (FvTST1-4) in strawberry genome. The qRT-PCR results indicated that FvTST1 has a differential expression pattern in different tissues and developmental stages, and exhibited highest expression level in mature fruits. The yeast complementation assay showed that FvTST1 can mediate the uptake of different sugars, such as fructose, glucose, sucrose, and mannose. Subcellular localization analyses revealed that FvTST1 was mainly targeted to the tonoplast. Transient expression of FvTST1 in strawberry fruits enhanced both fruit ripening and sugar accumulation. Furthermore, FvTST1-transformed tomato plants exhibited higher sucrose and auxin content, enhanced seed germination and vegetative growth, higher photosynthetic rate, early flowering, and bore fruit; fructose and glucose levels were higher in transgenic fruits than those in the control. Transcriptomic analysis indicated that the auxin signaling pathway was highly enriched pathway in up-regulated Gene-ontology terms. In transgenic plants, genes encoding transcription factors, such as phytochrome-interacting factors PIF1, -3, and -4, as well as their potential target genes, were also induced. Collectively, the results show that FvTST1 enhances plant growth and fruit ripening by modulating endogenous sugars, and highlight the biological significance of this gene for future breeding purposes.
Sugars play pivotal roles in plant growth and development. They are a carbon source and signal molecules regulating multiple gene networks (Ruan, 2014). Sugars are synthesized in photosynthetic leaves (source) and eventually transported to nonphotosynthetic tissues (sink) such as those in roots and fruits, where they are used as a carbon source for plant growth and fruit development (Tognetti et al., 2013; Julius et al., 2017). Sucrose (Suc), monosaccharide fructose (Fru), and glucose (Glu) are important for improving fruit quality, because they act as primary nutrients and sweetening compounds (Patrick et al., 2013). Although the sugar content of a fruit is primarily dependent on leaf (source) input, the key in regulating sugar accumulation lies within the fruit (Ruan and Patrick, 1995).
Vacuoles account for nearly 90% of entire cellular volume in mature fruits and store more than 70% of total sugar (Shiratake and Martinoia, 2007). Plant cell activity to accumulate sugar is regulated by sugar flow in the cytoplasm and the function of sugar transporters in the tonoplast (Ruan, 2014; Ren et al., 2018; Zhu et al., 2021). The tonoplast consists of a group of transporters, including SUTs/SUCs (sucrose transporters) (Schneider et al., 2012), SWEETs (Klemens et al., 2013; Eom et al., 2015), VGTs (vacuolar glucose transporters) (Aluri and Büttner, 2007), and TSTs (Wormit et al., 2006), and it encodes proteins consistent with sugar influx and efflux into plant vacuoles.
Recently, many reports have demonstrated that the TST family of sugar transporters is important for sugar accumulation in plants. For instance, PpTST1 expression tendency is associated with accumulation of sugar in fruits, and its TRV-mediated suppression inhibited sucrose and hexose content in peach fruits (Peng et al., 2020). In pear, six PbTMTs were reported, of which PbTMT4 was highly expressed in the fruit. Moreover, the heterologous expression of PbTMT4 in tomato significantly enhanced Glu and Fru content in tomato fruits (Cheng R. et al., 2018), suggesting that PbTMT4 likely transports hexoses. CmTST2 expression was higher in sugar-rich varieties and was lower in low-sugar varieties in melon, and its overexpression dramatically increased sugar content in strawberry and cucumber fruits (Cheng J. et al., 2018). MdTST1 expression was higher during apple fruit development, indicating its functional correlation with sugar influx to vacuoles during the ripening of the fruit (Wei et al., 2014). In grape fruits, VvTST1 and VvTST2 were significantly expressed TST isoforms closely associated with hexose accumulation (Afoufa-Bastien et al., 2010; Çakir and Giachino, 2012). The overexpression of Arabidopsis TSTs (AtTST1 and -2, formerly named as TMT1 and -2) facilitates sugar influx into plant vacuoles (Wormit et al., 2006; Schulz et al., 2011). Sugar signaling plays a crucial role in plant growth (Mishra et al., 2021) and enhances the ripening of fruits (Jia et al., 2013; Durán-Soria et al., 2020). For example, exogenous sucrose treatment promoted fruit coloration, ripening, and postharvest process of strawberry and tomato fruits (Jia et al., 2013; Li et al., 2016). Recent research documented that water melon TST (ClTST2) overexpression is associated with sugar accumulation, and that it is controlled by a sugar-induced transcription factor (SUSIWM1) (Ren et al., 2018). The sugar-inducible transcription factor SUSIWM1 induces ClTST2 transcript level by binding to the SURE element in the promoter region, thereby enhancing sugar influx into the vacuole. Intriguingly, in potato, the SURE element in a gene promoter was regulated by sucrose, which suggests that during fruit ripening, storage of sugars in the vacuole is mediated by TSTs and regulated by sucrose (Grierson et al., 1994). Sucrose crosstalk with plant hormones has also been documented, such as the transcription factors PIF, which has recently been identified to be crucially implicated in sucrose signaling (Lilley et al., 2012), directly induces the transcript level of expansins (EXPs) and XYLOGLUCAN ENDOTRANSGLUCOSYLASE/HYDROLASE PROTEINs (XTHs). Many plant hormones, including auxin, brassinosteroid (BR), ethylene (ET), and gibberellic acid (GA) (Kohnen et al., 2016; Ivakov et al., 2017), are involved in this process.
The study reports the expression of FvTSTs in the strawberry fruit, and vegetative as well as reproductive tissues, and determined the highest expression level of FvTST1 in mature fruits. The transport function of FvTST1 was analyzed in hxt-null yeast mutant. Moreover, FvTST1 was overexpressed in strawberry fruits and tomato plants to elucidate its physiological role. An RNA-sequences analysis was performed to examine significant transcriptional changes between transgenic and non-transgenic tomato plants.
Strawberry seeds (Fragaria vesca L.) were grown in a mixture of peat, vermiculite, and perlite (volume ratio of 3:2:1) by regular fertilization. Fruits were harvested in different developmental stages and were grouped as previously reported (Jia et al., 2013); small green (about 6 days after flowering (DAF), big green (13 DAF), degreening (18 DAF), white (22 DAF), initiated redness (26 DAF), partially red (29 DAF), and fully red (33DAF); and were stored at –80°C for qPCR analyses. The vegetative tissues including root, stem, leaf bud, first leaf, second leaf, and mature leaf, as well as reproductive tissues such as flower buds, initiated opening, partial opening, and fully opened flowers, were also collected and frozen on-site in liquid nitrogen, then stored at −80°C until use.
Total RNA was extracted from specific tissues using RNAprep Pure Kit (Takara, Beijing, China) (for polysaccharide- and polyphenolic-rich plants), following the user manual from the manufacturer. Nanodrop 2000 Spectrophotometer (Thermo Fisher Scientific, Wilmington, DE, United States) and 1% agarose gel electrophoresis were used to determine RNA quality and concentration. The extracted RNA was digested with DNase I, and PrimeScript™ RT Master Mix (Takara, Beijing, China) was used to synthesize cDNA.
The open reading frame of the cDNA was amplified with gene-specific primers (Supplementary Table 2) based on FvTST1 (Accession XM_004300064) available at NCBI. The whole cDNA of FvTST1 was then amplified with mix super fidelity polymerase (Vazyme Biotech Co., Ltd.), under thermal cycling conditions of 95°C denaturation for 5 min, followed by 30 cycles of 95°C for 30 s, 60°C for 30 s, 72°C for 2 min, and a final extension of 72°C for 10 min. A single band of expected size 2217 bp was purified from gel and sub cloned into pEASY vector (TransGen Biotech, Beijing, China).
In this study, qPCR was conducted for FvTSTs in different tissues of strawberry, and for validation of some genes between transgenic and non-transgenic lines (control). We designed the primers with the Primer Premier 5.0 software (Premier Biosoft, Palo Alto, CA, United States) (Supplementary Table 2). The 18S ribosomal RNA (18S rRNA) (accession number KF668233.1) was used for transcript level normalization. PrimeScriptTM RT Kit (Perfect Real Time) (Takara, Beijing, China) was used for the preparation of the qPCR reaction, and the CFX96 platform (Bio RAD, Shanghai, China)1 was used to perform the reaction. The 2–ΔΔCT method was used for the calculation of the transcript levels of the genes.
The Sugar transport function was elucidated in yeast mutant strain (EBY.VW4000) (Wieczorke et al., 1999) using lithium acetate transformation with pYES2.0 vector containing the FvTST1 gene or with empty vector (Gietz et al., 1992). FvTST1 was amplified using a pair of primers with restriction sites Sac I and Xba I at their 5′ ends (Supplementary Table 3). To get pYE2.0-FvTST1, the resulting DNA fragment was cleaved with Sac I and Xba I, and was connected into pYES2.0. The pYES2.0-FvTST1-transformed yeast strain (EBY.VW4000) and transformants were cultured and selected as reported before (Li et al., 2017). For complementation assay of sugar, yeast cells were inoculated in a liquid medium with 2% maltose as a carbon source, and were incubated overnight at 30°C to an optical density (OD600) of 1. The yeast cells were then diluted serially with (10-, 100-, and 1,000-fold), and a 4-μl dilution was cultured in the medium containing yeast nitrogen base without uracil with 2% maltose, fructose, glucose, sucrose, and mannose as carbon sources. Wild-type yeast was used as positive control, while an empty vector and EBY.VW.4000 were used as negative controls. All the plates were placed at 30°C for 2 days and were documented.
The FvTST1 gene fused with GFP was cloned into the binary vector pBI121. The CaMV35s-FvTST1-GFP fusion construct, tonoplast marker CaMV35s- γTIP-mCherry (CD3-975), and plasma membrane marker CaMV35s-PIP2A-mCherry (CD3-1007) (Nelson et al., 2007) were verified by sequencing, and then transformed into Agrobacterium. The Agrobacterium was inoculated in an LB medium and incubated overnight at 28°C in a shaker at 200 rpm. The bacterial culture was collected and then suspended in a buffer containing MES; 10 mM (pH 5.6), MgCl2 10 mM, and acetosyringone 200 mM to an OD600 of 1. The mixture was kept at room temperature for 1–3 h and was infiltrated into the lower surface of Nicotiana benthamiana leaves with a 1-ml syringe. After 3 days, the infiltrated leaves were put on water glass slides for microscopic examination. Fluorescence was detected in leaf cells using a TCS-SP8 laser-scanning confocal microscope (Leica, Mannheim, Germany). The fluorescence detection wavelengths of GFP were 488 and 500–530 nm, and the fluorescence detection wavelengths of mCherry were 514 and 560–620 nm.
The CDS of FvTST1 was sub-cloned into the binary vector pBI121 employing Xba I and Sac I. The promoter of the TOMATO PROLINE RICH PROTEIN (TPRP-F1) gene (X61395.1) (Salts et al., 1991) was then placed 5′ upstream of the FvTST1 CDS in pBI121-FvTST1 using a pair of one-step recombinant cloning primers (Supplementary Table 2) employing Hind III and Xba I to get the plant expression construct pBI121-PTPRP–F1::FvTST1.
FvTST1 and its counterpart empty vector pBI121 were infiltrated into strawberry fruits according to a previous report (Jia et al., 2013). An LB liquid medium with antibiotics was used for the inoculation of Agrobacterium and incubated overnight at 28°C to an optical density (OD600) of 1. The culture was collected and then suspended in the buffer (10 mM MgCl2, 10 mMMes, pH5.6, and 200 mM acetosyringone) to an OD600 = 0.8, and placed at room temperature for 2 h before infiltration into the fruit. Fruits of strawberry attached to the plant in degreening stage (18 DAF) were injected using a sterile 1-ml hypodermic syringe.
To generate the FvTST1 transgenic tomato, Agrobacterium-mediated leaf disk transformation of tomato [Solanum lycopersicum cv. Ailsa Craig+ (AC+)] was performed as reported previously (Sun et al., 2006). Cotyledons of 2-week-old seedlings were pre-cultured in an MS medium supplemented with 0.1% Gamborg’s vitamins, 1 μg/ml 6-BA, and 40 ng/ml of IAA for 3 days. The cotyledons were then immersed for 15 min in an Agrobacterium culture (OD600 = 0.1–0.2) with the liquid MS medium. The cotyledons were dried and then co-cultured for 2 days in an MS medium containing 0.1% Gamborg’s vitamins, 0.1 μg/ml Kinetin, 0.2 μg/ml 2, 4-D, and 1.5 μg/ml acetosyringone under dark conditions. After co-cultivation, the cotyledons were transformed into a regeneration and selection MS medium (0.1% Gamborg’s vitamin, 10 μg/ml 6-BA, 0.4 μg/ml IAA, 0.5 mg/ml carbenicillin, and 50 mg/ml kanamycin). After every 3 weeks, the medium was refreshed until shooting. Two-to-three-centimeter newly developed shoots were then transformed into a rooting MS medium (0.1% Gamborg’s vitamins, 4 μg/ml IAA, 0.5 mg/ml carbenicillin, and 50 mg/ml kanamycin) until true roots developed. The presence of FvTST1 in positive regenerants from kanamycin selection was verified by PCR and sequencing.
The T1 seeds from T0 transgenic tomato plants from each independent transgenic line were geminated in a 1/2 MS medium containing kanamycin, and the non-transgenic seeds were grown in a 1/2 MS medium without kanamycin and transferred to a growth chamber, which was set at 25°C/22°C with relative humidity of 65%, a photoperiod of 16 h and a photon flux of about 200 μmoles⋅m–2⋅s–1. Seven-day-old (4 DAG) transgenic and control seedlings were collected, frozen in liquid nitrogen, and stored at –80°C until use. The total RNA from independent transgenic and control lines was extracted. RNA-seq was performed with a commercial RNA-seq agent (OE Biotech, Shanghai, China), and with Trimmomatic; the raw data (raw reads) were analyzed (Bolger et al., 2014). To obtain the clean reads, poly-n and low-quality reads were removed. Clean reads were mapped to reference genomes of Solanum lycopersicum cultivar “Heinz 1706,” available at the Sol Genome network ITAG v2, using HISAT2 (Kim et al., 2015). Cufflinks were used for normalization of exonic reads (Trapnell et al., 2012), which were reported as FPKM, and the number of gene reads was counted using htseq-count (Anders and Huber, 2012; Anders et al., 2015). DESeq R package functions, estimate size factors, and nbinomTest were used to find DEGs (Anders and Huber, 2012). A p value < 0.05 and a fold change > 2 or < 0.5 have been set as the significant differential expression thresholds for the selection of induced or suppressed genes. The DEGs were analyzed for GO and KEGG pathway enrichment using the R package (Kanehisa et al., 2008), which was based on hypergeometric distribution.
The contents of Fru, Glu, and Suc, in the strawberry fruit, tomato seedlings, and fruits were determined using an LC-20A high-performance liquid chromatography system (Shimadzu Company, Kyoto, Japan) with a 5-μm XB-NH2 column (4.6 mm × 250 mm; Shanghai Welch Materials, China) and a RID20A detector. To develop a standard curve, authentic standards of different sugars were purchased from Sigma (St Louis, MO, United States). Soluble sugars, Fru, Glu, and Suc were extracted according to previous reports (Castonguay et al., 1995). Plant tissues weighing 0.5 g were ground with liquid nitrogen, and soluble sugars were extracted using 10 ml of distilled water at 100°C for 1 h. The samples were centrifuged at 13,000 g for 10 min, and the supernatant was transferred to a new 10-ml tube and brought to 10 ml with distilled water. A 1-ml sample was filtered before HPLC analysis through a 0.22-μm filter membrane. The mobile phase contained acetonitrile/water with a volume-to-volume ratio of 75:25 v/v, and flow rate was adjusted to 1 ml/min with an injection volume of 10 μl.
The IAA authentic internal standard (BCCC6104) was purchased from Sigma-Aldrich (St. Louis, MO, United States. IAA was extracted following previous reports (Pan et al., 2008). Seedlings weighing 0.3 g were ground to fine powder with liquid nitrogen. Extraction buffer (isopropanol–HCl, 2: 0.002 v/v) with 2 ml was added to the powder and incubated for 30 min at 4°C and centrifuged at 13,000 g for 5 min, and repeated twice. After centrifugation, the lower organic phase was transferred to a 10-mL tube and evaporated in a constant stream of nitrogen. The samples were kept in the dark before being resuspended in a solution of 150 μl methanol and 0.1% formic acid. The samples were filtered with a 0.45-μm microfilter, and the reversed phase column (C18 ZORBAX 300SB, 3 μm; 4.6 mm × 9 mm × 150 mm; Agilent, Sta. Clara, CA, United States) was used for sample injection. The mobile phase contained methanol as solvent A, water with 0.1% formic acid was used as solvent B, and column temperature was adjusted to 30 °C. The product was purified using a solvent gradient, which began with volume adjustment to 20% methanol for 2 min, and was linearly increased to 80% methanol for 14 min, after equilibration for 5 min, and then reverted to the volume of 20% methanol for 0.1 min. A hybrid triple quadruple mass spectrometer (SCIEX 6500 QTrap; Applied Biosystems, Foster City, CA, United States) was employed for quantification analysis. System voltage was set to 4.5 kV. Source temperature was set to 500°C. Nebulizer and curtain drying gas pressure was adjusted to 75.15 and 65 psi, respectively.
The net photosynthesis rates of cotyledons of (4 DAG) seedlings were measured with CIRAS-3 (PP Systems, Amesbury, MA, United States). The analyzers act as absorption meters to measure infrared absorption. The optical bench is temperature-controlled and pressure-compensated to provide accurate CO2 and H2O measurement. Net photosynthesis (A) was determined from the difference between the CO2 concentration entering (Cin) and exiting (Cout) the cuvette. IRGA CO2 reading was corrected for water vapor, temperature, and atmospheric pressure. Since humidity dilutes the air leaving the cuvette (Cout), it was compensated using the following equation: net photosynthesis (A) = (Cin × W) – [Cout × (W+E)], where (A) is net photosynthesis, (W) is mass flow of air per unit leaf area into the cuvette, and (E) is transpiration rate.
The experiment was conducted using a completely random method. For statistical analysis, the SPSS software version 19.00 (SPSS, Chicago, IL, United States) was used. The mean values of transgenic lines and wild type lines were compared by independent t-test or one-way ANOVA test. The data was expressed as the mean ± standard deviation of three replicates.
Previously, four putative TST genes, FvTST1-4, were identified in the strawberry genome (Jiu et al., 2018; Liu et al., 2020). The phylogenetic tree revealed that FvTSTs share significant homology with ArabidopsisAtTST1, -2, -3 (Supplementary Figure 1). To understand the possible role of FvTSTs, the expression profile of these genes was analyzed in different tissues by qRT-PCR analysis (Figure 1). Expression analyses of FvTSTs indicated that they were differentially expressed in all the examined tissues in different developmental stages. Among them, FvTST1 was strongly expressed in the second leaf; however, it was weakly expressed or unnoticeable in other tissues, including young fruits, but its level was significantly increased in fully red fruits. Moreover, FvTST2 and FvTST3 had almost the same weak expression tendencies during plant growth and fruit development. On the contrary, FvTST4 expression was detected in the root, stem, and leaf, and was weakly expressed in flower buds, partially open flowers, fully open flower, and small green fruits, while higher expression level was detected in partially red fruits, and then decreased in fully red fruits. Overall, the results highlight that FvTST1 is a highly expressed TST gene during strawberry fruit development, and suggest that it might contribute to sugar accumulation in fruits.
Figure 1. Transcript profile of FvTSTs in different organs of strawberry in different developmental stages by quantitative real-time polymerase chain reaction (qRT-PCR). The data represent mean ± standard deviation of three biological replicates. To normalize the relative expression level, 18S rRNA was used as an internal control.
The expression profile of genes is used as a useful tool for the selection of candidate genes; however, further evaluation is necessary. In a widely used heterologous system, the transport function of many sugar transporters has been characterized (Lemoine, 2000). The hxt-null strain EBY.VW4000 (Wieczorke et al., 1999; Conde et al., 2007) has been widely used for studying plant sugar transporters. The knockout of hexose transporters in this strain prevents it from uptaking hexoses, thus allowing the assessment of the gene introduced. FvTST1 was expressed in EBY.VW4000 hxt-null yeast mutant cells. Selection of the transformant was carried out in the medium containing maltose without uracil as a carbon source, and was confirmed by PCR (Supplementary Figure 2). The pYES2.0–FvTST1 transformant in the yeast strain was then plated in a medium containing 2% maltose, fructose, glucose, sucrose, and mannose as key carbon sources. The positive control was wild-type yeast, and the negative control was an empty vector and EBY.VW.4000. The serial dilution (10-, 100-, and 1,000-fold) of yeast strain demonstrated that the EBY.VW4000 transformant with pYES2.0–FvTST1 and wild-type yeast can grow in all the media including the sucrose-containing medium, and that the empty vector and EBY.VW.4000 can only grow in the presence of maltose (Figure 2). This result confirms that FvTST1 is a functional tonoplast sugar transporter.
Figure 2. Sugar transport function analysis of FvTST1 in yeast mutant strain EBY.VW.4000. Yeast cells were diluted serially (10-, 100-, and 1,000-fold) and were spotted in a minimal solid medium for 2 days at 30°C, with 2% maltose, fructose, glucose, sucrose, and mannose as sources of carbon. The wild-type yeast was used as a positive control, and EBY.VW.4000 and empty vector were used as negative controls.
For subcellular localization of FvTST1, we established a construct encoding FvTST1-GFP driven by a 35S promoter, and was co-expressed wit hCaMV35s-γTIP-mCherry (tonoplast marker, CD3-975) and CaMV35s-PIP2A-mCherry (plasma membrane marker, CD3-1007) (Nelson et al., 2007), in Nicotiana benthamiana leaves. Confocal microscopy images show that the GFP signal from FvTST1 overlapped with the mCherry signal of the tonoplast marker, and a merged yellow signal was observed in whole cells, whereas in zoom-in cells, two GFP lines and two mCherry lines from the tonoplast marker were observed and indicated by white arrows in zoom-in cells (Figure 3A). We further analyzed the co-localization of FvTST1 with the plasma membrane marker. Two linear GFP signals (white arrows), a single mCherry signal, and a weak merged yellow signal were observed in the zoom-in cells of the plasma membrane marker (Figure 3B). These data show that FvTST1 is mainly located in the tonoplast.
Figure 3. Subcellular localization of FvTST1-GFP fusion protein in Nicotiana benthamiana. (A) Co-localization of FvTST1-GFP with Gamma TIP-mCherry (CD3-975 tonoplast, TP, marker) in N. benthamiana leaves. (B) Co-localization of FvTST1-GFP and PIP2A-mCherry (CD3-1007; plasma membrane, PM, marker) in N. benthamiana leaves. The dashed box indicates the enlarged portion and highlights localization differences between TP and PM.
To elucidate the possible role of FvTST1 during fruit development, the construct with FvTST1 and pBI121 empty vectors (control) were injected in the degreening stage (18 DAF) of strawberry fruits, while the fruits were still attached to the plants. Seven days after injection, we observed that the fruits injected with FvTST1 turned red, and that the fruits with the pBI121 empty vector did not turn red (Figure 4A). We analyzed the expression level of FvTST1 in these two groups and found that FvTST1 exhibited higher expression in the FvTST1-injected fruits than in the control (Figure 4B). The sugar concentration in these two groups was assessed, and the content of Fru, Glu, and Suc was significantly induced in the FvTST1-overexpressed fruits than in the control (Figure 4C). These findings demonstrated that FvTST1 overexpression in the strawberry fruits enhanced fruit ripening and sugar accumulation.
Figure 4. Transient expression of FvTST1 in strawberry fruit. (A) Phenotypes of FvTST1-overexpressed strawberry fruit. (B) qRT PCR analyses of FvTST1-overexpressed fruit and control. (C) Sugar content (fructose, glucose, and sucrose) in FvTST1-overexpressed fruits. The values represent mean ± standard deviation of three biological replicates. The SPSS software (v19.0) was used for one-way analysis of variance (ANOVA) (**p < 0.01).
To further elucidate the role of FvTST1 in plant growth and fruit development, the FvTST1 overexpression construct (Figure 5A) was overexpressed in the tomato plant. Transgene presence in the transgenic plants was analyzed by PCR (Figure 5B), and a high level of transgene transcript was detected in all the independent transgenic lines, but not detected in the non-transgenic (control) line (Figure 5C). In particular, the transgenic plant shows enhanced vegetative growth (Figures 5D,E), flowers, and bears fruits (Figures 5F,G) significantly earlier than control. Transgenic plant height was significantly higher than that of the control (Figure 5H). Moreover, to understand the phenotypic alteration between transgenic plants and control, the seedlings were characterized further. The root and hypocotyl length of “transgenic seedlings” was significantly longer (p < 0.05) than that of control (Figures 5I,J). Moreover, higher net photosynthetic rates were observed in some of the transgenic lines (T4, T5, and T6) than in the control (Figure 5K). Transgenic seeds were heavier than the control (Figure 5L), and they germinated 2 days earlier than the control. FvTST1 transcript level was significantly increased in the root, hypocotyl, and cotyledons of the transgenic plants, but it was not detected in the control plants (Figure 5M).
Figure 5. Phenotypic alteration of FvTST1 transgenic tomato (Solanum lycopersicum cv. Ailsa Craig+ (AC+). (A) PTPRP–F1::FvTST1-pBI121 plants expression construct. (B) PCR detection of FvTST1 in transgenic plants. (C) Transcript levels of FvTST1 in transgenic and control plants. (D) Phenotypic differences among 7-day-old (4 DAG) transgenic plants (T) compared to control (CK) (Scale bar = 5 cm). (E) Enhanced growth of 38-day-old transgenic plant compared to control (Scale bar = 20 cm). (F) Phenotypes of 51-day-old transgenic plants and control (Scale bar = 20 cm). (G) Phenotypes of 95-day-old transgenic and control plants (Scale bar = 30 cm). (H) Plant height differences between transgenic plant and control. (I) Root growth of the 7-day-old (4 DAG) transgenic plants compared to control. (J) Hypocotyl growth of 7-day-old (4 DAG) transgenic plants compared to control. (K) Net Photosynthesis rate (L) Higher seed weight of transgenic tomato compared to control plants. (M) Transcript level analysis of FvTST1 in root (R), hypocotyl (H) and cotyledon (C). (CK refers to control and “T” stands for transgenic, and number represents independent transgenic line). The data were shown as mean ± standard deviation of three biological replicates. The SPSS software (v19.0) was used for one-way ANOVA (*p < 0.05; **p < 0.01; ***p < 0.001).
Soluble sugars (Fru, Glu, and Suc) were measured in seedlings and mature fruits of the transgenic and control plants (Figure 6). Our results showed that Suc content was significantly higher in the transgenic seedlings than in the controls, but that there were no obvious changes in Fru and Glu content (Figure 6A). However, when the transgenic plant mature fruits were compared to the controls, the Fru and Glu contents were significantly increased, but no changes in Suc content were observed (Figure 6B).
Figure 6. Analysis of sugars content in transgenic and control plants. (A) Sugar content in 7-day-old (4 DAGs) transgenic plants and control plants. (B) Sugar content in mature fruit of transgenic and non-transgenic plants. Values are mean ± standard deviation of three replicates. Statistical analysis was performed with the SPSS software (v19.0); one-way ANOVA followed by Dennett’s multiple comparisons was performed (**p < 0.01; ***p < 0.001).
RNA-seq analysis was performed to reveal the transcriptional changes in the transgenic plants. Clean reads ranged from 46.35 to 49.29 M, total mapped reads were 97%, and Q30 of the raw bases was 94% (Supplementary Table 1), suggesting that the transcriptome data were reliable. A total of 25,934 genes were expressed in the 7-day old (4 DAG) tomato seedlings. Among the DEGs, using fold change > 2 and a Q-value < 0.05, a total of 1,142 genes were up-regulated and 746 genes were down-regulated (Supplementary Figure 3A). According to GO analysis, the down-regulated genes had 514 enriched (q < 0.05) GO terms, whereas the up-regulated genes had 427 enriched (q < 0.05) GO terms.
Gene ontology (GO) was further studied, and the top 10 GO terms of biological process (BP), cellular component (CC), and molecular function (MF) for up-regulated and down-regulated genes were analyzed (Supplementary Figures 3B,C). In the up-regulated biological process, “the auxin signaling pathway,” “auxin polar transport,” and “regulation of growth” were highly enriched terms (Supplementary Figure 3B). Apoplast, membrane system, and cell wall were enhanced, which was consistent with enhanced seedling growth (Supplementary Figure 3B). PSI was the most affected pathway in BP, CC, and MF (Supplementary Figure 3C) in the down-regulated genes. Low-affinity nitrate transmembrane transport was found to be down-regulated (Supplementary Figure 3B), suggesting alteration of nitrate/carbon balance. The genes selected during this study are available at https://solgenomics.net/ while the name and accession numbers are provided (Supplementary Table S3).
Phytochrome-interacting factors (PIFs) are a group of negative regulators of photomorphogenesis (Toledo-Ortiz et al., 2010; Kim k. et al., 2016) and are induced by sucrose (Lilley et al., 2012). In this study PIF1, -3, and -4 were significantly up-regulated, and were confirmed by qPCR analysis (Figure 7A). PHYTOCHROME B (PHYB), which is suppressed by PIFs (Jang et al., 2010), was found down-regulated with a fold change of 0.62. Many genes that were directly induced by PIFs were significantly enhanced in this study, such as BZR1, GA2ox2, TAA1, and IAA29 (Toledo-Ortiz et al., 2010), and their expression was also confirmed by qPCR (Figure 7B). PSY, another target of PIF1 (Toledo-Ortiz et al., 2010), was also found to be down-regulated. PIFs have the ability to bind to G-box, E-box, and ACE cis-elements (Zhang et al., 2013; Kim J. et al., 2016), and the existence of G-box, E-box, and ACE was checked across the 2kb segment upstream of the translation start site (ATG) for GA2ox2, BZR1, and TAA1. The results showed that 5, 8, and 18 cis-elements existed in the promoters of TAA1, BZR1, and GA2ox2, respectively (Supplementary Figure 4A). Moreover, sequences of the 2kb segment upstream of the ATG of all 1,888 DEGs from the tomato genome were examined for cis-element analysis. The results revealed that most of the promoters of the examined DEGs contained E-boxes and ACE. The promoters of the genes containing G-boxes accounted for about 1/4 to 1/3 of the total number of the affected genes (Supplementary Figure 4B).
Figure 7. Transcriptional alterations of PIFs, their putative target genes and hypocotyl and root growth-related DEGs. (A) Expression level of PIF1, -3, -4 in transgenic plants and control. (B) Expression of PIF putative direct target genes GA2ox2, BZR1 and TAA1, and IAA29. (C) Transcript levels of expansins. (D) Transcript level of XTHs. (E) Transcript level of SAUR19. The data were shown as mean ± standard deviation of three biological replicates, and 18srRNA was used as internal control for the normalization of expression level.
In addition, PIFs and auxin both regulate EXPs and XTHs, which are important for hypocotyl elongation and root growth. In this study, 6 EXPs and 10 XTHs were up-regulated, except XTH8, XTH32, and EXPA1 (Figures 7C,D). Besides, the transcription of the gene SAUR19 was up-regulated (Figure 7E), which has been reported previously for hypocotyl growth stimulation indirectly regulated by PIFs (Leivar and Monte, 2014).
Interestingly, a total of 54 DEGs were obtained in the “auxin-activated signaling pathway,” with 45 up-regulated and 9 down-regulated, including SAUR9/11/20/21/24/26/32/50/63/64, and WAG2. Regarding “Auxin polar transport,” which is vital for root growth (Sabatini et al., 1999; Grieneisen et al., 2007; Gutierrez et al., 2012), linked DEGs were all up-regulated (Figure 8A). Consistently, a significantly higher auxin concentration was found in the 7-day old (4DAG) transgenic seedlings compared to the control (Figure 8B). It was worth noting that 33 DEGs out of 45 “growth regulation” genes were members of the auxin signaling pathway, indicating the pivotal role of auxin in regulating transgenic plant growth. Expression alterations of SAUR19/21/24/63/64, WAG2, were further confirmed by qPCR analysis (Figure 8C). These findings strongly suggest that PIF1, -3, -4 and/or auxin signaling pathways are involved in enhancement of root growth and hypocotyl elongation.
Figure 8. Auxin signaling pathway was affected in the PTPRPF1::FvTST1 lines (A) DEGs related to “Regulation of growth,” “Auxin-activated signaling pathway,” and “Auxin polar transport.” (B) Auxin concentration in transgenic seedlings and control. (C) qPCR verification of several genes involved in auxin signaling pathway. The dot represents the twelve genes that are responsible for growth enhancement, but not the members of auxin signaling pathway. Values are means ± standard deviation of three biological replicates. The SPSS software (v19.0) was used for one-way ANOVA (*p < 0.05; **p < 0.01).
High sugars accumulate in many fleshy fruits, such as apple, grape, tomato, and strawberry. In fruit cells, the vacuole is the major compartment for sugar storage in fruit cells, accounting for more than 90% of total cell volume (Shiratake and Martinoia, 2007; Hedrich et al., 2015). The tonoplast comprises many sugar transporters including SWEETs (Eom et al., 2015), SUCs/SUTs (sucrose transporters) (Schneider et al., 2012), VGTs (Aluri and Büttner, 2007), and TSTs (Wormit et al., 2006). Tonoplast sugar transporters (Wormit et al., 2006) are the most important regulators that contribute to sugar influx into the vacuole. Several TSTs have been reported in diverse plant species that influenced plant growth and fruit quality, including Arabidopsis (Wormit et al., 2006), grape (Afoufa-Bastien et al., 2010; Çakir and Giachino, 2012), apple (Wei et al., 2014), melon (Cheng J. et al., 2018), watermelon (Ren et al., 2018), and pear (Cheng R. et al., 2018). However the understanding of the TST function in different plant species is underestimated. The findings of this study provide insights into the role FvTST1 in sugar accumulation in fruit and plant growth enhancement.
There are 4 TSTs in the strawberry genome. In particular, FvTST1 shares significant homology with previously identified sugar transporters such as AtTST1 (Wormit et al., 2006), VvTST1 (Afoufa-Bastien et al., 2010), and MdTST1 (Wei et al., 2014). The expression levels of these genes have been analyzed in different tissues (Figure 1). FvTST1 was highly expressed during strawberry fruit development, and its expression pattern was consistent with fruit development and sugar accumulation, warranting speculations that FvTST1 may play a role in strawberry fruit sugar accumulation. These assertions coincide with previous findings (Wei et al., 2014; Cheng J. et al., 2018).
In addition, to identify the function of FvTST1 as a sugar transporter, we overexpressed FvTST1 in hexose-deficient yeast (EBY.VW4000) (Wieczorke et al., 1999). The yeast expressing gene FvTST1 can grow in the medium containing, fructose, glucose, sucrose, and mannose, but the hexose-deficient yeast (EBY.VW4000) and empty vector did not grow (Figure 2), which revealed that FvTST1 is a functional sugar transporter. Our results are consistent with previous findings that were observed for the plasma membrane transporter and tonoplast transporter (Vignault et al., 2005; Cheng R. et al., 2018). In our study, FvTST1 transported sucrose, and our findings are concomitant with that of ArabidopsisAtTMT1,-2-transfected cells (Wormit et al., 2006; Schulz et al., 2011), which are located into the tonoplast, and have hexose and sucrose import activity. Furthermore, we have analyzed the subcellular localization of FvTST1 in N. benthamiana leaves (Figure 3), indicating that FvTST1-GFP fluorescence is mainly observed in the tonoplast, which coincides with previous studies such as on AtTST1 (Wormit et al., 2006) and CmTST2 (Cheng J. et al., 2018).
In addition, to elucidate the physiological function and substrate specificity of FvTST1 in vivo, we transiently overexpressed FvTST1 in the strawberry fruit. The fruits overexpressing FvTST1 gene matured faster, and exhibited higher sugar content than the control (Figure 4), confirming the function of FvTST1 as a sugar transporter, and promoting strawberry fruit maturation. In our study, the gene FvTST1-overexpressed fruits showed early maturity, and this alteration could be related to the involvement of sucrose signaling, because in previous studies it was documented that the content of sucrose in the cytosol has signaling functions regulating strawberry fruit maturation (Jia et al., 2013). The overexpression of FaSUT1, a sucrose transporter in the plasma membrane of strawberry, enhanced sucrose content and fruit ripening, but the down regulation of this gene reduced sucrose content and ripening of fruit (Jia et al., 2013).
To assess the role of FvTST1 in plant and fruit development, we observed the phenotypic differences between FvTST1-overexpressing tomato plants and control. The overexpression of FvTST1 exhibited visible phenotypes, such as fast vegetative growth, early flowering, and enhanced fruit ripening (Figure 5), as those observed for tomato plants overexpressing PbTMT4 (Cheng R. et al., 2018). Particularly, in FvTST1-overexpressed transgenic seedlings and fruits, the content of sucrose and hexose were significantly elevated (Figure 6), respectively. It has been reported that the overexpression of MdTST1 and MdTST2 can increase soluble sugar content in apple callus, and that MdTST1 and MdTST2 RNAi suppression significantly reduced soluble sugars (Zhu et al., 2021). Previous studies have shown that the overexpression of AtTST1, -2-altered sugar in subcellular compartments enhanced seed biomass and early plant development (Wingenter et al., 2010; Schulz et al., 2011). However, in Arabidopsis, the overexpression of AtTST1 has no effect on the abundance of soluble sugars in transgenic seedlings (Wingenter et al., 2010). Nevertheless, in this study, relative to the non-transgenic tomato seedlings, the transgenic tomato seedlings exhibited a higher level of sucrose but not hexose (Fru or Glu). These findings suggest that TST genes are conserved in many plant species, and that they are likely to have functional differences in different plants species and e1ven distinct tissues.
To understand further the underlying mechanism of FvTST1 during plant growth and development, RNA sequencing analysis was performed. Auxin activation pathway genes were found to be significantly enriched, indicating that the auxin signaling pathway is essential for seedling development. Previous research studies have indicated that Suc can induce seedling growth through auxin-mediated signaling pathways (Mishra et al., 2009; Lilley et al., 2012). The sucrose-induced auxin signaling pathway involves IAA29, YUC8 and the transcription factor phytochrome-interacting factors (PIFs) (Lilley et al., 2012). In our study, FvTST1 overexpression enhanced sucrose content in transgenic seedlings and found that PIF1, –3, and –4, were significantly up regulated, which coincides with previous findings (Lilley et al., 2012). In addition to many up-regulated genes, BZR1 and GA2ox2, TAA1, and IAA29 regulated by PIFs are crucial for the BR, GA, and auxin signaling pathways (Leivar and Monte, 2014), and sucrose-induced seedling growth (Lilley et al., 2012).
The present data demonstrated that FvTST1 was highly expressed predominantly in fully red fruits, and highlighted its functional association with fruit ripening. Furthermore, FvTST1 transports fructose, glucose, sucrose, and mannose in the hxt-null mutant, and its overexpression in strawberry fruit, tomato plant, and localization experiments support its function as a tonoplast sugar transporter, as well as its importance for sugar accumulation in fruits. Moreover, RNA-seq analyses unravel the mechanism of fast growth and development, indicating that the up-regulation of auxin signaling pathway-linked genes was the most significant transcriptional change. The transcript level of PIF1, -3, and -4 was induced in the transgenic seedlings, which highlighted the role of the sucrose signaling pathway. Collectively, our results suggest that FvTST1 is a consideration target for manipulating early fruit bearing plants and fruits with sweet flavor.
The original contributions presented in the study are included in the article/Supplementary Material, further inquiries can be directed to the corresponding author.
AR generated the transgenic plants and performed the main experiments, analyzed the transcriptomic data, and prepared the manuscript draft. HR critically reviewed the manuscript. YW conceived the project and finalized the manuscript. All authors contributed to the article and approved the submitted version.
This study was supported by the National Natural Science Foundation of China (31770734 and 31972989) and National Key Research and Development Program of China (2018YFD1000601).
The authors declare that the research was conducted in the absence of any commercial or financial relationships that could be construed as a potential conflict of interest.
All claims expressed in this article are solely those of the authors and do not necessarily represent those of their affiliated organizations, or those of the publisher, the editors and the reviewers. Any product that may be evaluated in this article, or claim that may be made by its manufacturer, is not guaranteed or endorsed by the publisher.
Special thanks to Yongsheng Liu for the gift of TPRP-F1 promoter and seeds of (Solanum lycopersicum) cv. AC+. We are also grateful to Professor Shu Wei for providing good suggestions during experiments and mass data collection.
The Supplementary Material for this article can be found online at: https://www.frontiersin.org/articles/10.3389/fpls.2021.774582/full#supplementary-material
Afoufa-Bastien, D., Medici, A., Jeauffre, J., Coutos-Thévenot, P., Lemoine, R., Atanassova, R., et al. (2010). The Vitis vinifera sugar transporter gene family: phylogenetic overview and macroarray expression profiling. BMC Plant Biol. 10:245. doi: 10.1186/1471-2229-10-245
Aluri, S., and Büttner, M. (2007). Identification and functional expression of the Arabidopsis thaliana vacuolar glucose transporter 1 and its role in seed germination and flowering. Proc. Natl. Acad. Sci. U.S.A. 104, 2537–2542. doi: 10.1073/pnas.0610278104
Anders, S., and Huber, W. (2012). Differential Expression of RNA-Seq Data At The Gene Level. Heidelberg: European Molecular Biology Laboratory, 1–23.
Anders, S., Pyl, P. T., and Huber, W. (2015). HTSeq–a Python framework to work with high-throughput sequencing data. Bioinformatics (Oxford, England) 31, 166–169. doi: 10.1093/bioinformatics/btu638
Bolger, A. M., Lohse, M., and Usadel, B. (2014). Trimmomatic: a flexible trimmer for Illumina sequence data. Bioinformatics 30, 2114–2120. doi: 10.1093/bioinformatics/btu170
Çakir, B., and Giachino, R. R. (2012). VvTMT2 encodes a putative tonoplast monosaccharide transporter expressed during grape berry (Vitis vinifera cv. Sultanine) ripening. Plant Omics 5:576. doi: 10.1093/pcp/pcz130
Castonguay, Y., Nadeau, P., Lechasseur, P., and Chouinard, L. (1995). Differential accumulation of carbohydrates in alfalfa cultivars of contrasting winterhardiness. Crop Sci. 35, 509–516. doi: 10.2135/cropsci1995.0011183X003500020038x
Cheng, J., Wen, S., Xiao, S., Lu, B., Ma, M., and Bie, Z. (2018). Overexpression of the tonoplast sugar transporter CmTST2 in melon fruit increases sugar accumulation. J. Exp. Bot. 69, 511–523. doi: 10.1093/jxb/erx440
Cheng, R., Cheng, Y., Lü, J., Chen, J., Wang, Y., Zhang, S., et al. (2018). The gene PbTMT4 from pear (Pyrus bretschneideri) mediates vacuolar sugar transport and strongly affects sugar accumulation in fruit. Physiol. Plant. 3, 307–319. doi: 10.1111/ppl.12742
Conde, C., Agasse, A., Silva, P., Lemoine, R., Delrot, S., Tavares, R., et al. (2007). OeMST2 encodes a monosaccharide transporter expressed throughout olive fruit maturation. Plant Cell Physiol. 48, 1299–1308. doi: 10.1093/pcp/pcm096
Durán-Soria, S., Pott, D. M., Osorio, S., and Vallarino, J. G. (2020). Sugar signaling during fruit ripening. Front. Plant Sci. 11:564917. doi: 10.3389/fpls.2020.564917
Eom, J. S., Chen, L. Q., Sosso, D., Julius, B. T., Lin, I. W., Qu, X. Q., et al. (2015). SWEETs, transporters for intracellular and intercellular sugar translocation. Curr. Opin. Plant Biol. 25, 53–62. doi: 10.1016/j.pbi.2015.04.005
Gietz, D., St Jean, A., Woods, R. A., and Schiestl, R. H. (1992). Improved method for high efficiency transformation of intact yeast cells. Nucleic Acids Res. 20:1425. doi: 10.1093/nar/20.6.1425
Grieneisen, V. A., Xu, J., Marée, A. F. M., Hogeweg, P., and Scheres, B. (2007). Auxin transport is sufficient to generate a maximum and gradient guiding root growth. Nature 449, 1008–1013. doi: 10.1038/nature06215
Grierson, C., Du, J. S., de Torres Zabala, M., Beggs, K., Smith, C., Holdsworth, M., et al. (1994). Separate cis sequences and trans factors direct metabolic and developmental regulation of a potato tuber storage protein gene. Plant J. 5, 815–826. doi: 10.1046/j.1365-313x.1994.5060815.x
Gutierrez, L., Mongelard, G., Floková, K., Pacurar, D. I., Novák, O., Staswick, P., et al. (2012). Auxin controls Arabidopsis adventitious root initiation by regulating jasmonic acid homeostasis. Plant cell 24, 2515–2527. doi: 10.1105/tpc.112.099119
Hedrich, R., Sauer, N., and Neuhaus, H. E. (2015). Sugar transport across the plant vacuolar membrane: nature and regulation of carrier proteins. Curr. Opin. Plant Biol. 25, 63–70. doi: 10.1016/j.pbi.2015.04.008
Ivakov, A., Flis, A., Apelt, F., Fünfgeld, M., Scherer, U., Stitt, M., et al. (2017). Cellulose synthesis and cell expansion are regulated by different mechanisms in growing Arabidopsis hypocotyls. Plant Cell 29, 1305–1315. doi: 10.1105/tpc.16.00782
Jang, I.-C., Henriques, R., Seo, H. S., Nagatani, A., and Chua, N.-H. (2010). Arabidopsis phytochrome interacting factor proteins promote phytochrome B polyubiquitination by COP1 E3 ligase in the nucleus. Plant Cell 22, 2370–2383. doi: 10.1105/tpc.109.072520
Jia, H., Wang, Y., Sun, M., Li, B., Han, Y., Zhao, Y., et al. (2013). Sucrose functions as a signal involved in the regulation of strawberry fruit development and ripening. New Phytol. 198, 453–465. doi: 10.1111/nph.12176
Jiu, S., Haider, M. S., Kurjogi, M. M., Zhang, K., Zhu, X., and Fang, J. (2018). Genome-wide characterization and expression analysis of sugar transporter family genes in woodland strawberry. Plant Genome 11, 1–16. doi: 10.3835/plantgenome2017.11.0103.Received
Julius, B. T., Leach, K. A., Tran, T. M., Mertz, R. A., and Braun, D. M. (2017). Sugar transporters in plants: new insights and discoveries. Plant Cell Physiol. 58, 1442–1460. doi: 10.1093/pcp/pcx090
Kanehisa, M., Araki, M., Goto, S., Hattori, M., Hirakawa, M., Itoh, M., et al. (2008). KEGG for linking genomes to life and the environment. Nucleic Acids Res. 36, 480–484. doi: 10.1093/nar/gkm882
Kim, D., Langmead, B., Salzberg, S. L., and Salzberg, S. L. (2015). HISAT: a fast spliced aligner with low memory requirements Daehwan HHS Public Access. Nat. Methods 12, 357–360. doi: 10.1038/nmeth.3317.HISAT
Kim, J., Kang, H., Park, J., Kim, W., Yoo, J., Lee, N., et al. (2016). PIF1-interacting transcription factors and their binding sequence elements determine the in vivo targeting sites of PIF1. Plant Cell 28, 1388–1405. doi: 10.1105/tpc.16.00125
Kim, K., Jeong, J., Kim, J., Lee, N., Kim, M. E., Lee, S., et al. (2016). PIF1 regulates plastid development by repressing photosynthetic genes in the endodermis. Mol. Plant 9, 1415–1427. doi: 10.1016/j.molp.2016.08.007
Klemens, P. A., Patzke, K., Deitmer, J., Spinner, L., Le Hir, R., Bellini, C., et al. (2013). Overexpression of the vacuolar sugar carrier AtSWEET16 modifies germination, growth, and stress tolerance in Arabidopsis. Plant Physiol. 163, 1338–1352. doi: 10.1104/pp.113.224972
Kohnen, M. V., Schmid-Siegert, E., Trevisan, M., Petrolati, L. A., Sénéchal, F., Müller-Moulé, P., et al. (2016). Neighbor detection induces organ-specific transcriptomes, revealing patterns underlying hypocotyl-specific growth. Plant Cell 28, 2889–2904. doi: 10.1105/tpc.16.00463
Leivar, P., and Monte, E. (2014). PIFs: systems integrators in plant development. Plant Cell 26, 56–78. doi: 10.1105/tpc.113.120857
Lemoine, R. (2000). Sucrose transporters in plants: update on function and structure. Biochim. Biophys. Acta 1465, 246–262. doi: 10.1016/s0005-2736(00)00142-5
Li, D., Mou, W., Wang, Y., Li, L., Mao, L., Ying, T., et al. (2016). Exogenous sucrose treatment accelerates postharvest tomato fruit ripening through the influence on its metabolism and enhancing ethylene biosynthesis and signaling. Acta Physiol. Plant 38:225. doi: 10.1007/s11738-016-2240-5
Li, Y., Wang, Y., Zhang, H., Zhang, Q., Zhai, H., Liu, Q., et al. (2017). The plasma membrane-localized sucrose transporter IbSWEET10 contributes to the resistance of sweet potato to Fusarium oxysporum. Front. Plant Sci. 8:197. doi: 10.3389/fpls.2017.00197
Lilley, J. L. S., Gee, C. W., Sairanen, I., Ljung, K., and Nemhauser, J. L. (2012). An endogenous carbon-sensing pathway triggers increased auxin flux and hypocotyl elongation. Plant Physiol. 160, 2261–2270. doi: 10.1104/pp.112.205575
Liu, H. T., Ji, Y., Liu, Y., Tian, S. H., Gao, Q. H., Zou, X. H., et al. (2020). The sugar transporter system of strawberry: genome-wide identification and expression correlation with fruit soluble sugar-related traits in a Fragaria × ananassa germplasm collection. Hortic. Res. 7:132. doi: 10.1038/s41438-020-00359-0
Mishra, B. S., Sharma, M., and Laxmi, A. (2021). Role of sugar and auxin crosstalk in plant growth and development. Physiol. Plant. doi: 10.1111/ppl.13546
Mishra, B. S., Singh, M., Aggrawal, P., and Laxmi, A. (2009). Glucose and auxin signaling interaction in controlling Arabidopsis thaliana seedlings root growth and development. PLoS One 4:e4502. doi: 10.1371/journal.pone.0004502
Nelson, B. K., Cai, X., and Nebenführ, A. (2007). A multicolored set of in vivo organelle markers for co-localization studies in Arabidopsis and other plants. Plant J. 51, 1126–1136. doi: 10.1111/j.1365-313X.2007.03212.x
Pan, X., Welti, R., and Wang, X. (2008). Simultaneous quantification of major phytohormones and related compounds in crude plant extracts by liquid chromatography-electrospray tandem mass spectrometry. Phytochemistry 69, 1773–1781. doi: 10.1016/j.phytochem.2008.02.008
Patrick, J. W., Botha, F. C., and Birch, R. G. (2013). Metabolic engineering of sugars and simple sugar derivatives in plants. Plant Biotechnol. J. 11, 142–156. doi: 10.1111/pbi.12002
Peng, Q., Wang, L., Ogutu, C., Liu, J., Liu, L., Mollah, M., et al. (2020). Functional analysis reveals the regulatory role of PpTST1 Encoding tonoplast sugar transporter in sugar accumulation of peach fruit. Int. J. Mol. Sci. 21:1112. doi: 10.3390/ijms21031112
Ren, Y., Guo, S., Zhang, J., He, H., Sun, H., Tian, S., et al. (2018). A tonoplast sugar transporter underlies a sugar accumulation QTL in watermelon. Plant Physiol. 176, 836–850. doi: 10.1104/pp.17.01290
Ruan, Y.-L. L. (2014). Sucrose metabolism: gateway to diverse carbon use and sugar signaling. Annu. Rev. Plant Biol. 65, 33–67. doi: 10.1146/annurev-arplant-050213-040251
Ruan, Y.-L., and Patrick, J. W. (1995). The cellular pathway of postphloem sugar transport in developing tomato fruit. Planta 196, 434–444.
Sabatini, S., Beis, D., Wolkenfelt, H., Murfett, J., Guilfoyle, T., Malamy, J., et al. (1999). An auxin-dependent distal organizer of pattern and polarity in the arabidopsis root. Cell 99, 463–472.
Salts, Y., Wachs, R., Gruissem, W., and Barg, R. (1991). Sequence coding for a novel proline-rich protein preferentially expressed in young tomato fruit. Plant Mol. Biol. 17, 149–150. doi: 10.1007/BF00036818
Schneider, S., Hulpke, S., Schulz, A., Yaron, I., Höll, J., Imlau, A., et al. (2012). Vacuoles release sucrose via tonoplast-localised SUC4-type transporters. Plant Biol. (Stuttgart, Germany) 14, 325–336. doi: 10.1111/j.1438-8677.2011.00506.x
Schulz, A., Beyhl, D., Marten, I., Wormit, A., Neuhaus, E., Poschet, G., et al. (2011). Proton-driven sucrose symport and antiport are provided by the vacuolar transporters SUC4 and TMT1/2. Plant J. 68, 129–136. doi: 10.1111/j.1365-313X.2011.04672.x
Shiratake, K., and Martinoia, E. (2007). Transporters in fruit vacuoles. Plant Biotechnol. 24, 127–133.
Sun, H.-J. J., Uchii, S., Watanabe, S., and Ezura, H. (2006). A highly efficient transformation protocol for Micro-Tom, a model cultivar for tomato functional genomics. Plant Cell Physiol. 47, 426–431. doi: 10.1093/pcp/pci251
Tognetti, J. A., Pontis, H. G., and Martínez-Noël, G. M. (2013). Sucrose signaling in plants: a world yet to be explored. Plant Signal. Behav. 8:e23316. doi: 10.4161/psb.23316
Toledo-Ortiz, G., Huq, E., and Rodríguez-Concepción, M. (2010). Direct regulation of phytoene synthase gene expression and carotenoid biosynthesis by phytochrome-interacting factors. Proc. Natl. Acad. Sci. U.S.A. 107, 11626–11631. doi: 10.1073/pnas.0914428107
Trapnell, C., Roberts, A., Goff, L., Pertea, G., Kim, D., Kelley, D. R., et al. (2012). Differential gene and transcript expression analysis of RNA-seq experiments with TopHat and Cufflinks. Nat. Protocols 7, 562–578. doi: 10.1038/nprot.2012.016
Vignault, C., Vachaud, M., Cakir, B., Glissant, D., Dédaldéchamp, F., Büttner, M., et al. (2005). VvHT1 encodes a monosaccharide transporter expressed in the conducting complex of the grape berry phloem. J. Exp. Bot. 56, 1409–1418. doi: 10.1093/jxb/eri142
Wei, X., Liu, F., Chen, C., Ma, F., and Li, M. (2014). The Malus domestica sugar transporter gene family: identifications based on genome and expression profiling related to the accumulation of fruit sugars. Front. Plant Sci. 5:569. doi: 10.3389/fpls.2014.00569
Wieczorke, R., Krampe, S., Weierstall, T., Freidel, K., Hollenberg, C. P., and Boles, E. (1999). Concurrent knock-out of at least 20 transporter genes is required to block uptake of hexoses in Saccharomyces cerevisiae. FEBS Lett. 464, 123–128. doi: 10.1016/s0014-5793(99)01698-1
Wingenter, K., Schulz, A., Wormit, A., Wic, S., Trentmann, O., Hoermiller, I. I., et al. (2010). Increased activity of the vacuolar monosaccharide transporter TMT1 alters cellular sugar partitioning, sugar signaling, and seed yield in Arabidopsis. Plant Physiol. 154, 665–677. doi: 10.1104/pp.110.162040
Wormit, A., Trentmann, O., Feifer, I., Lohr, C., Tjaden, J., Meyer, S., et al. (2006). Molecular identification and physiological characterization of a novel monosaccharide transporter from arabidopsis involved in vacuolar sugar transport. Plant Cell 18, 3476–3490. doi: 10.1105/tpc.106.047290
Zhang, Y., Mayba, O., Pfeiffer, A., Shi, H., Tepperman, J. M., Speed, T. P., et al. (2013). A quartet of PIF bHLH factors provides a transcriptionally centered signaling hub that regulates seedling morphogenesis through differential expression-patterning of shared target genes in Arabidopsis. PLoS Genet. 9:e1003244. doi: 10.1371/journal.pgen.1003244
Keywords: FvTST1, sugar, fruit ripening, PIFs, auxin signaling pathway, growth regulation
Citation: Rashid A, Ruan H and Wang Y (2022) The Gene FvTST1 From Strawberry Modulates Endogenous Sugars Enhancing Plant Growth and Fruit Ripening. Front. Plant Sci. 12:774582. doi: 10.3389/fpls.2021.774582
Received: 12 September 2021; Accepted: 10 December 2021;
Published: 11 January 2022.
Edited by:
Karl H. Hasenstein, University of Louisiana at Lafayette, United StatesReviewed by:
Woei-Jiun Guo, National Cheng Kung University, TaiwanCopyright © 2022 Rashid, Ruan and Wang. This is an open-access article distributed under the terms of the Creative Commons Attribution License (CC BY). The use, distribution or reproduction in other forums is permitted, provided the original author(s) and the copyright owner(s) are credited and that the original publication in this journal is cited, in accordance with accepted academic practice. No use, distribution or reproduction is permitted which does not comply with these terms.
*Correspondence: Yunsheng Wang, d2FuZ3l1bnNoZW5nQGFoYXUuZWR1LmNu
Disclaimer: All claims expressed in this article are solely those of the authors and do not necessarily represent those of their affiliated organizations, or those of the publisher, the editors and the reviewers. Any product that may be evaluated in this article or claim that may be made by its manufacturer is not guaranteed or endorsed by the publisher.
Research integrity at Frontiers
Learn more about the work of our research integrity team to safeguard the quality of each article we publish.