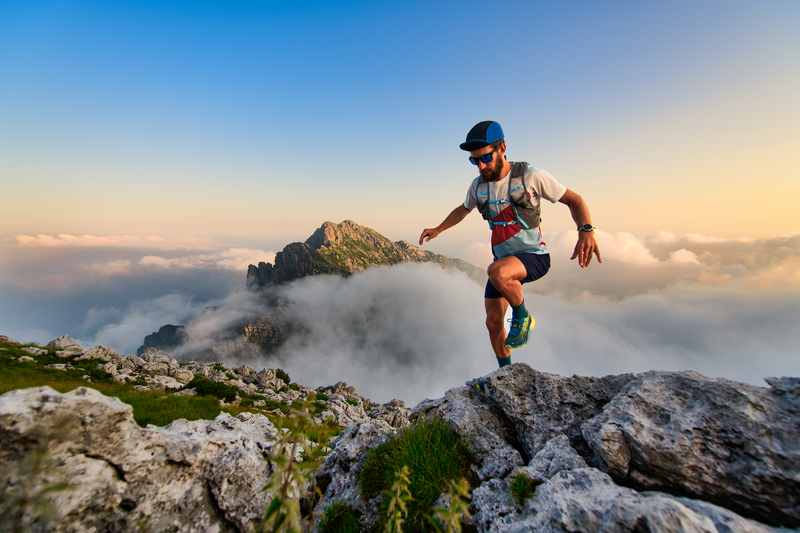
95% of researchers rate our articles as excellent or good
Learn more about the work of our research integrity team to safeguard the quality of each article we publish.
Find out more
ORIGINAL RESEARCH article
Front. Plant Sci. , 11 January 2022
Sec. Plant Biotechnology
Volume 12 - 2021 | https://doi.org/10.3389/fpls.2021.769907
This article is part of the Research Topic Targeted Genome Editing for Crop Improvement View all 20 articles
Sweet orange (Citrus sinensis) is the most economically important species for the citrus industry. However, it is susceptible to many diseases including citrus bacterial canker caused by Xanthomonas citri subsp. citri (Xcc) that triggers devastating effects on citrus production. Conventional breeding has not met the challenge to improve disease resistance of sweet orange due to the long juvenility and other limitations. CRISPR-mediated genome editing has shown promising potentials for genetic improvements of plants. Generation of biallelic/homozygous mutants remains difficult for sweet orange due to low transformation rate, existence of heterozygous alleles for target genes, and low biallelic editing efficacy using the CRISPR technology. Here, we report improvements in the CRISPR/Cas9 system for citrus gene editing. Based on the improvements we made previously [dicot codon optimized Cas9, tRNA for multiplexing, a modified sgRNA scaffold with high efficiency, citrus U6 (CsU6) to drive sgRNA expression], we further improved our CRISPR/Cas9 system by choosing superior promoters [Cestrum yellow leaf curling virus (CmYLCV) or Citrus sinensis ubiquitin (CsUbi) promoter] to drive Cas9 and optimizing culture temperature. This system was able to generate a biallelic mutation rate of up to 89% for Carrizo citrange and 79% for Hamlin sweet orange. Consequently, this system was used to generate canker-resistant Hamlin sweet orange by mutating the effector binding element (EBE) of canker susceptibility gene CsLOB1, which is required for causing canker symptoms by Xcc. Six biallelic Hamlin sweet orange mutant lines in the EBE were generated. The biallelic mutants are resistant to Xcc. Biallelic mutation of the EBE region abolishes the induction of CsLOB1 by Xcc. This study represents a significant improvement in sweet orange gene editing efficacy and generating disease-resistant varieties via CRISPR-mediated genome editing. This improvement in citrus genome editing makes genetic studies and manipulations of sweet orange more feasible.
Citrus is one of the most important fruit crops worldwide because of its delightful flavor and scent, as well as its health properties. Sweet orange (Citrus sinensis) is the most economically important species for the citrus industry. However, citrus production is facing many biotic (e.g., diseases and insects) and abiotic challenges (e.g., drought, flooding, acidity, salinity, heat, cold, drought, and nutrient deficits) (Li, 2009). Among them, citrus diseases such as citrus canker caused by Xanthomonas citri subsp. citri (Xcc) (Ference et al., 2018) and Huanglongbing (HLB, also known as citrus greening) caused by Candidatus Liberibacter asiaticus (Bové, 2006; Wang, 2019; Yuan et al., 2020) are causing devastating effects on the citrus industry. Most elite citrus varieties, including sweet orange and grapefruit varieties, are susceptible to canker and HLB disease. Genetic improvements of citrus via conventional approaches are challenging. Most citrus varieties result from human selection of natural mutations or natural hybridization rather than artificial hybridization. Hybridization-based breeding for citrus is hindered by long juvenile stage (3–10 years), a highly heterozygous nature, heterozygous nature of nucellar seedlings (clones of the female parent), and male or female sterility (Omura and Shimada, 2016). It has been suggested that CRISPR-mediated genome editing will revolutionize the genetic improvements of citrus and other tree crops (Dutt et al., 2020; Wheatley and Yang, 2020).
Citrus genome editing mediated by the CRISPR technology was first reported in 2014 (Jia and Wang, 2014a,b). The first biallelic mutant of citrus (Carrizo citrange, a rootstock variety) was reported in 2017 (Zhang et al., 2017a). The first biallelic/homozygous mutations of citrus scion varieties [Pummelo (Citrus maxima)] were reported in 2020 (Jia and Wang, 2020). It is noteworthy that Pummelo is highly homozygous (Wu et al., 2018) and relatively easy to generate biallelic/homozygous mutants compared to other citrus species. However, most elite citrus varieties including sweet orange are highly heterozygous and biallelic/homozygous mutants have not been reported to date or showed very low editing efficiency (Jia et al., 2017a,2019a; Peng et al., 2017). We aimed to further improve the CRISPR/Cas system to make genome editing of elite citrus varieties more achievable and improve the disease resistance of sweet orange against canker. Utilization of resistant varieties is the most efficient and eco-friendly approach to control diseases. However, most commercial citrus varieties including sweet orange are susceptible to citrus canker (Favaro et al., 2020; Ference et al., 2020).
Improvement of genome editing efficiency of plants has been conducted via multiple approaches including improvements of the expression of Cas proteins and sgRNA using different promoters. Several promoters for driving Cas9 expression have been tested, such as 35S promoter, ubiquitin promoter (Castel et al., 2019), cell division-specific Yao promoter (Yan et al., 2015), egg cell-specific EC1.2/DD45 (Wang et al., 2015), and RPS5a promoter expressing at early embryonic stage (Tsutsui and Higashiyama, 2017; Ordon et al., 2020). Among them, the ubiquitin promoter and RPS5a promoter have significantly improved genome editing efficacy compared to the commonly used 35S promoter in Arabidopsis, tomato, rice, or maize (Stavolone et al., 2003; Cermak et al., 2017; Tsutsui and Higashiyama, 2017; Castel et al., 2019). Endogenous polymerase III promoters (U3 or U6) improve the expression of sgRNAs (Sun et al., 2015; Qi et al., 2018). In addition, other approaches including codon optimization of the Cas proteins (Li et al., 2014) and temperature optimization (Xiang et al., 2017) have shown promises to improve the efficacy of genome editing.
Xanthomonas citri subsp. citri causes the characteristic hypertrophy and hyperplasia symptoms on citrus tissues via secretion of PthA4, a transcriptional activator-like (TAL) effector, through the type III secretion system (Swarup et al., 1991; Yan and Wang, 2012; An et al., 2020). PthA4 enters the nucleus and activates the expression of the canker susceptibility gene LATERAL ORGAN BOUNDARIES 1 (LOB1) via binding to the effector binding element (EBE) in the promoter region using its central nearly identical tandem repeats of 33–34 amino acids (Hu et al., 2014). The specific recognition between nucleotides and the repeat is determined by the 12th and 13th amino acids of each repeat, known as the “repeat-variable diresidue” (RVD) (Moscou and Bogdanove, 2009; Römer et al., 2009). Mutation of the EBE regions has been used to generate disease resistance crops against TAL effector-dependent pathogens such as bacterial blight of rice (Li et al., 2012; Oliva et al., 2019). In our previous study, genome modified Duncan grapefruit of the coding region of LOB1 demonstrates canker resistance (Jia et al., 2017b). Mutation of both alleles of the EBE region of LOB1 is required to abolish its induction by PthA4 and generate canker-resistant citrus plants (Jia et al., 2016, 2021; Jia and Wang, 2020). However, biallelic/homozygous mutation of the EBE region for elite varieties such as sweet orange is yet to be achieved. Most importantly, highly efficient generation of biallelic/homozygous mutation in elite varieties such as sweet orange and grapefruit has not been reported yet.
In this study, we improved the CRISPR/Cas9 system, which was used to generate six biallelic CsLOB1 EBE mutant lines of Hamlin sweet orange. All biallelic CsLOB1 EBE mutant plants (100%) are resistant against Xcc, representing a milestone in canker resistance development for elite citrus varieties. In addition, this improved CRISPR/Cas9 system efficiently (100%) edits the tobacco genome. Hence, this improved CRISPR/Cas9 system may be applied to other dicots besides citrus and tobacco.
First, we sought to improve the CRISPR/Cas9-mediated genome editing efficiency in citrus using the phytoene desaturase (PDS) gene as a target. The PDS gene was chosen for editing because of the obvious albino phenotype caused by homozygous or biallelic knockout mutation of the PDS gene (Zhang et al., 2017a; Huang et al., 2020). The albino phenotype serves as the functional editing (null mutation) readout. We first edited Carrizo citrange, a hybrid between C. sinensis “Washington” sweet orange and Poncirus trifoliata, which can be relatively easily transformed (compared to sweet orange) and edited based on our previous experience (Jia et al., 2017a; Huang et al., 2020). A green fluorescence (GFP) visual maker was included in the constructs to further confirm successful plant transformation in addition to antibiotic marker selection. Inspired by previous reports showing that increased culture temperature can increase editing efficacy (LeBlanc et al., 2018; Malzahn et al., 2019; Milner et al., 2020), we sought to test if we can increase editing efficacy by increasing culture temperature. The editing efficiency was higher at 30°C for both gene editing in protoplasts (Supplementary Figure 1A and Supplementary Table 4) and in Agrobacterium-mediated transformed epicotyl tissue than that at room temperature (Supplementary Figure 1).
Previously, we improved the CRISPR/Cas9 system by codon optimizing the Cas9 gene, identifying citrus U6 (CsU6) promoter, using an improved gRNA scaffold, and inclusion of tRNA for multiplexing (Xie et al., 2015; Huang et al., 2020). Here we aimed to select a superior promoter for driving Cas9 expression. The 320 bp core region of the 35S promoter in the CRISPR/Cas9 construct was replaced with either the Citrus sinensis ubiquitin (CsUbi) or Cestrum yellow leaf curling virus (CmYLCV) promoter (Supplementary Data 1). The CsUbi promoter (Cs4g11190) was selected through mining of citrus RNAseq data (Ribeiro et al., 2021). Cs4g11190 is highly expressed in various stages of leaf development (Supplementary Table 1). The promoter region of CsUbi (Cs4g11190) was amplified from the citrus genome. The CmYLCV promoter (Stavolone et al., 2003) is a strong promoter and functions in both monocots and dicots. The upstream 538 bp regulatory region containing the enhancer elements of 35S was kept and fused with candidate promoters (Figure 1A) to enhance Cas9 expression and/or promote early expression of Cas9 in first dividing cells (Ow et al., 1987; Benfey et al., 1990). The editing efficacy of 35S, CsUbi, and CmYLCV driving Cas9 expression at 30°C was compared (Figure 1A). The albino phenotype was used as a readout for null mutations (Figure 1B). The 35S promoter produced 50% albino plants; the CsUbi promoter and CmYLCV promoter generated 80 and 89% albino plants, respectively (Figure 1C and Supplementary Figure 2), suggesting that CsUbi and CmYLCV promoters are superior to 35S for gene editing in citrus.
Figure 1. Optimal promoters for driving Cas9 expression to improve gene editing efficacy in citrus. (A) 35S promoter, citrus ubiquitin promoter (CsUbi), and Cestrum yellow leaf curling virus (CmYLCV) promoter for driving Cas9 expression in the CRISPR constructs. (B) A representative picture showing albino phenotype, caused by null mutation of the PDS gene with CmYLCV promoter construct. The construct contains GFP as visual marker. (C) Summary of editing efficacy from three constructs shown in (A).
In all transformation events, we observed chimeric albino phenotype. It is common to observe chimera phenotypes during plant transformation of explants, such as epicotyledons, leaves, and stems. The chimera rate for the 35S, CsUbi, and CmYLCV was 25, 12, and 5.6%, respectively (Figure 1C). These results suggest that CsUbi and CmYLCV promoters drive an early expression of the Cas9 in the first transformed cells compared to 35S promoter. All constructs contained GFP. Not surprisingly, GFP florescence was observed to colocalize with albino phenotype. Therefore, for all the transformation experiments, only transformants with evenly distributed GFP florescence all over the plantlets were kept for further analyses.
The CmYLCV promoter is a strong constitutive promoter for heterologous gene expression in Arabidopsis thaliana and Nicotiana tabacum and in a wide variety of crops including Lycopersicon esculentum, Zea mays, and Oryza sativa (Stavolone et al., 2003). We hypothesized that the improved CRISPR/Cas9 system driven by CmYLCV works in other plant species, especially dicots. To test this hypothesis, we tested the construct in tobacco (N. tabacum), an allotetraploid species, considering its amenability to stable transformation. There are two PDS homologs, PDS-A and PDS-B in tobacco genome. Two gRNAs were designed in the conserved regions between the two homologs (Figure 2A). We obtained a total of 11 transformants with GFP. The transformants with green fluorescence all showed albino phenotype (Figure 2B and Supplementary Figure 3), indicating that we achieved tetra-allelic mutations for PDS-A and PDS-B in all positive transformants (Figure 2C). This result demonstrates that the improved CRISPR/Cas9 system achieves a 100% null mutation efficacy for PDS genes in tobacco. This result suggests that our improved CRISPR/Cas9 system may be able to efficiently edit genomes of other dicots, besides citrus and tobacco.
Figure 2. The improved CRISPR/Cas9 system works efficiently for genome editing of tobacco (Nicotiana tabacum). (A) Gene structures of two NtPDS homologs from allotetraploid Nicotiana tabacum. Two gRNAs conserved in both NtPDS-A and NtPDS-B genes as indicated were designed for genome editing. (B) A representative picture shows the albino phenotype in genome modified Nicotiana tabacum. The construct contains GFP as visual marker. (C) Summary of editing efficacy.
Sweet orange is a hybrid between pummelo (C. maxima) and mandarin (Citrus reticulata), and most genes have two different alleles. Here, we used the improved CRISPR/Cas9 system that contains a codon optimized Cas9, tRNA for multiplexing (Xie et al., 2015; Huang et al., 2020), CsU6 promoter (Huang et al., 2020), improved sgRNA scaffold (Dang et al., 2015), and the CmYLCV promoter to edit the EBE in the promoter region of the canker susceptibility gene CsLOB1 (Hu et al., 2014). The EBE region of sweet orange contains two different types/alleles. We aimed to edit both alleles to create null mutations.
Two different gRNAs for both type I and type II EBEs were designed. A third gRNA targeting a region upstream of the translation start codon ATG was also included (Figure 3A). The final construct contains these three gRNAs. Such a design aimed to create not only small indels, but also longer deletion mutations. We first transformed citrus protoplasts to confirm the functionality of the construct. As expected, the construct not only produced indel mutations, but also generated relatively long deletion mutations (Figures 3B–D).
Figure 3. CsLOB1 editing in Hamlin sweet orange protoplasts. (A) CsLOB1 promoter contains two different types. The EBE region was highlighted with yellow; regions chosen for gRNAs were underlined; PAM was shown in red. The final CRISPR constructs contains three gRNAs as indicated. (B) PCR products generated by two primers spanning the EBE and last gRNA. The PCR product with an arrow was amplified from the deletion mutants. (C) Sanger sequencing results of the PCR product with the arrow in (B). Twenty clones were sequenced. m1, 11 clones; m2, 5 clones; m3, 4 clones. (D) Sanger sequencing results of the PCR products marked by * in (B) that were cloned for colony sequencing. Ninety-four clones were sequenced. m4, 2 clones; m5, 2 clones; m6, 1 clone; WT, 89 clones.
Next, we performed a stable transformation of Hamlin sweet orange epicotyls. A total of 16 positive transformants were obtained. Two lines died during regeneration. Finally, we obtained 14 transgenic plants. We genotyped these 14 seedlings. Among them, 11 plants contained biallelic mutations in the EBE region (Figure 4 and Supplementary Figure 4), representing a 78.6% biallelic mutation rate (Supplementary Table 2). The three heterozygous mutants contained both wild-type and edited EBEs. Subsequently, we micro-grafted these seedlings onto the rootstock variety Carrizo citrange. A total of six biallelic mutants and two heterozygous mutants were successfully grafted on the rootstock, whereas the rest did not survive.
Figure 4. Highly efficient generation of biallelic mutant citrus plants for Hamlin sweet orange. Genotyping of biallelic mutants. Mutations of the EBE region is the focus of the genotyping. The EBE region was highlighted with yellow; regions chosen for gRNAs were underlined; PAM was indicated in red; nucleotide G in blue is a SNP only in one allele, but not in another allele.
We hypothesized that the biallelic mutation of the EBE region of CsLOB1 disrupts its binding to Xcc TAL effector PthA4, thus Xcc is unable to induce canker susceptibility gene CsLOB1 for symptom development (Swarup et al., 1992; Hu et al., 2014). To test this hypothesis, wild-type Hamlin sweet orange plants, one heterozygous mutant line (line #11) and six biallelic mutant lines (line #1, 2, 3, 4, 5, 15) of Hamlin sweet orange were tested for resistance against Xcc strains (Figure 5). For each genotype, one half of the leaf was inoculated with wild-type Xcc, and another half was inoculated with Xcc pthA4:Tn5 mutant carrying the designer TALE dLOB2, which activates the expression of LOB2 (Teper et al., 2020). LOB2 is a LOB1 homolog that causes canker symptoms when artificially induced, such as in the presence of dLOB2 (Zhang et al., 2017b; Teper et al., 2020). Xcc pthA4:Tn5 dLOB2 was included as a control. The onset of canker symptoms by Xcc pthA4:Tn5 dLOB2 on the same leave can exclude the possibility that lack of canker symptoms for biallelic mutants is owing to leaf age. At 8 dpi, all wild-type Hamlin leaves developed canker lesions for both wild-type Xcc and dLOB2 carrying Xcc pthA4:Tn5. No canker symptoms were observed for the biallelic mutant lines (line #1, 2, 3, 4, 5, and 15) when inoculated with wild-type Xcc. On the contrary, canker symptoms were observed on the other half of the leaves inoculated with Xcc pthA4:Tn5 dLOB2. The heterozygous mutant line #11 showed canker disease symptoms for both wild-type Xcc and dLOB2 carrying Xcc pthA4:Tn5 (Figure 5). Taken together, the present results demonstrate that the biallelic Hamlin sweet orange mutant lines are resistant to Xcc.
Figure 5. Biallelic mutants of the EBE region of CsLOB1 of Hamlin sweet orange are resistant to Xcc. Xcc inoculation assay was conducted using fully expanded young leaves. Line #11 is a heterozygous mutant; other lines are biallelic mutants. For each line, left half of the tested leaf was syringe inoculated with wild-type Xcc (108 CFU/ml); the right half of the tested leaf was inoculated with Xcc pthA4:Tn5 carrying designer TAL effector dLOB2 (108 CFU/ml). Pictures were taken at 8 days post inoculation.
Next, we tested whether biallelic mutations of the EBE region of CsLOB1 abolish its induction by Xcc using reverse transcription-quantitative PCR (RT-qPCR). The expression level of CsLOB1 in wild-type Hamlin sweet orange was dramatically induced when inoculated with Xcc, which is consistent with previous findings (Hu et al., 2014; Teper et al., 2020). The induction of CsLOB1 was obliterated in the biallelic mutant line Ham1 (Figure 6A). Xcc pthA4:Tn5 dLOB2 induced the expression of LOB2 (Figures 6B,C), which is consistent with canker symptoms induced by Xcc pthA4:Tn5 dLOB2 on both wild-type and biallelic mutant lines (Figure 5) and with our previous result (Teper et al., 2020).
Figure 6. Induction of CsLOB1 by Xcc is abolished in a biallelic mutant line of Hamlin sweet orange. (A) RT-qPCR analyses of CsLOB1 relative expression in leaf samples collected at 48 h post inoculation with indicated treatments. Mock, mock inoculation with buffer; Xcc, inoculation with wild-type Xcc (108 CFU/ml); dLOB2, inoculation with Xcc pthA4:Tn5 carrying designer TAL effector dLOB2 (108 CFU/ml). Each treatment has three biological repeats. Ham1, a representative biallelic mutant line #1 (aka Ham1). All expression levels were normalized to the WT mock. The GAPDH gene was used as an endogenous control. (B) RT-qPCR analysis of LOB2 relative expression of leaf samples collected at 48 h post inoculation with indicated treatment. All expression levels were normalized to the Ham1 mock. The GAPDH gene was used as an endogenous control. (C) Finding promoter binding site for TAL effectors by using Target Finder (https://tale-nt.cac.cornell.edu/node/add/talef-off). Designer TAL effector dLOB2 shows affinity to the LOB2 promoter, but not the LOB1 promoter.
To investigate whether this improved CRISPR/Cas9 can introduce off-target mutations, we amplified and sequenced 20 potential off-target sites with 4 or fewer mismatches within the protospacers in all 6 canker-resistant Hamlin mutant lines. No potential off-targets containing less than 4 mismatches were found. Hence, it is very likely that there are no off-targets. The sequencing results showed that no mutations at potential off-target sites were detected (Supplementary Table 5).
In this study, we improved CRISPR/Cas9 system to achieve high efficacy for biallelic editing in citrus. The improved system contains a dicot codon-optimized Cas9, tRNA for multiplexing, an improved sgRNA scaffold with high efficiency (Dang et al., 2015), CsU6 to drive sgRNA expression, and superior promoters (CmYLCV or CsUbi promoter). The dicot codon-optimized Cas9 can work efficiently in tomato (Cermak et al., 2017), citrus, and N. benthamiana. The improved sgRNA scaffold contains a shorter T-stretch to avoid early termination and 5 nt extensions of the tetraloop for stability (Dang et al., 2015). Importantly, this improved CRISPR/Cas9 system has significantly increased the biallelic/homozygous mutation rates. For Carrizo citrange, the CsUbi and CmYLCV driven constructs achieved null mutation rates of 80 and 89%, respectively, via epicotyl transformation. In addition, the CmYLCV construct attained a 79% biallelic mutation rate in Hamlin sweet orange even under restrained gRNA options (EBE region). The difference in editing efficacy may also result from the differential transformation efficacy in different citrus genotypes. The transformation efficacy was reported to be 20.6% for Carrizo citrange (Pena et al., 1995), but ranged from 0.88 to 23.8 for sweet orange varieties (Poles et al., 2020) and was 2.5% for Hamlin sweet orange (Fávero et al., 2012). Previously, the biallelic mutation rate was reported to be 5.3% for grapefruit (Jia et al., 2021), 44.4% for Carrizo citrange (11.1% homozygous mutation rate) (Huang et al., 2020), and 22.7–55% for Carrizo citrange (Zhang et al., 2017a). Thus, this improved CRISPR/Cas9 system we reported here represents a significant improvement compared to previous studies. In addition, this new system uses a citrus U6 promoter and a CmYLCV promoter to drive sgRNA or Cas9 protein, respectively. It has been recommended to use endogenous promoters to drive Cas proteins or sgRNA to improve efficacy or to address gene regulation concerns. It was reported that U6 promoters and two ubiquitin promoters identified in grapevine significantly increase the editing efficiency in grape by improving the expression of sgRNA and Cas9, respectively (Ren et al., 2021). Similarly, endogenous promoters for U6 and ubiquitin were successfully used for genome editing of maize and yielded mutation efficiencies ranging from 48.5 to 97.1% (Qi et al., 2018). The improved CRISPR/Cas9 system developed in this study can be used not only for editing the genome of citrus, but also for other plant species. We successfully edited tobacco PDS genes (100% null mutation) with the CmYLCV-driven construct. We anticipate that the modified CRISPR/Cas9 system we developed is efficient in genome editing of other plant species, especially dicots.
The CmYLCV promoter and CsUbi promoter driving the expression of Cas9 have significantly improved the citrus editing efficacy compared to that of the 35S promoter. It has been reported that strong and constitutive promoters isolated from the plant housekeeping genes such as ubiquitin or actin can achieve higher mutation rates in various monocot and dicot species (Hassan et al., 2021). The CmYLCV promoter was reported to function in both dicots and monocots and shows higher or comparable activities than the 35S promoter in driving the heterozygous expression (Stavolone et al., 2003). The CmYLCV promoter may drive early expression of the Cas9 in the first transformed cells as suggested by the fewest chimera observed. Similar to the 35S promoter, the CmYLCV promoter is an RNA polymerase II promoter. The CmYLCV promoter demonstrates similar efficacy as the Ubiquitin 2 promoter of switchgrass in transformation, reaching about 80 and 100% of protoplasts after 24 and 48-h, respectively, post transformation (Weiss et al., 2020). In addition to driving the expression of Cas proteins, the CmYLCV promoter has also been successfully used to drive the sgRNA array via Csy4-mediated processing or gRNA–tRNA array (Cermak et al., 2017).
We successfully obtained multiple biallelic canker-resistant Hamlin sweet orange mutant lines against Xcc, which is a TAL effector-dependent pathogen, via editing the EBE region of canker susceptibility gene LOB1 (Hu et al., 2014). TAL effectors are critical virulence factors for most Xanthomonas pathogens (Boch and Bonas, 2010; Perez-Quintero and Szurek, 2019; An et al., 2020). TAL effectors are known to be subject to repeated recombination that allows them to recognize new targets or overcome the resistance resulting from mismatches between the TAL effectors and the EBE regions. It was reported that Xcc is able to overcome 2–5 mismatches between the repeat region of PthA4 and the EBE of CsLOB1, but is unable to overcome the resistance when the mismatch is equal or more than 7 (Teper and Wang, 2021). However, the previous study was conducted via an accelerated evolutionary approach that includes injection with high titers of Xcc than normally encountered in natural settings. In addition, the TALEs were cloned into pBBR1MCS5, a medium copy number vector (approximately 30 copies), whereas naturally occurring PthA4 is three copies in each bacterial cell (Teper and Wang, 2021). It is anticipated that the chance for Xcc to overcome the mismatch between PthA4 and the EBE region in natural settings is much lower than that observed via the accelerated evolutionary study (Teper and Wang, 2021). Additionally, the genome modified lines will enable us to investigate whether Xcc can overcome the resistance resulting from EBE mutations in natural settings in future studies. The biallelic mutants also can serve as a diagnostic tool to monitor emerging Xcc strains that target other susceptibility genes such as LOB2 and LOB3 (Zhang et al., 2017b; Teper et al., 2020). A similar strategy has been developed for Xoo/rice pathosystem (Eom et al., 2019).
Of note, the canker-resistant sweet orange lines contain the CRISPR/Cas construct, which is considered as transgenic and might not be suitable to be used for citrus production. Nevertheless, successful generation of canker-resistant sweet orange represents a milestone in the ultimate utilization of canker-resistant citrus varieties based on mutations of the coding or the EBE region of the canker susceptibility gene LOB1. Generation of canker-resistant citrus varieties will overcome the significant issues in canker management using copper-based products (Zhang et al., 2003), antibiotics (Hu and Wang, 2016; Li et al., 2019, 2020), and biocontrol bacteria. Such an approach has been successfully used to generate disease-resistant rice varieties (Oliva et al., 2019). For rice, the CRISPR/Cas construct can be easily removed by progeny segregation or back-crossing, which is not practical for citrus. This is because citrus has long juvenile stage. In addition, back-crossing leads to the loss of the elite quality of parental varieties. In future studies, we will aim to generate non-transgenic genome-modified canker-resistant citrus varieties via transient expression of the CRISPR/Cas construct. Such a method has been successfully used to generate non-transgenic genome-edited crops (Zhang et al., 2016; Chen et al., 2018; Lin et al., 2018; Veillet et al., 2019). Development of methods using in vitro assembled CRISPR/Cas9/gRNA ribonucleoprotein (Cas9/gRNA RNP) complexes for transient expression of the CRISPR/Cas9 system in citrus may also be an attractive future option given that this approach has proven successful in producing other crop plants that contain precisely edited genes and that are non-transgenic (Woo et al., 2015; Svitashev et al., 2016; Zhang et al., 2021). In addition, newly emerging DNA delivery technologies such as nanoparticles (Demirer et al., 2019; Lv et al., 2020) might help deliver our highly efficient constructs to achieve non-transgenic genome editing.
The seeds of Carrizo citrange were purchased from Lyn Citrus Seeds (Arvin, CA, United States). The seeds of sweet orange Hamlin were harvested from mature fruits in the groves of the Citrus Research and Education Center, University of Florida.
To test the performance of different promoters for driving Cas9, we replaced the 320 bp core region of the 35S promoter in construct pC-CsU6-tRNA-3PDS, which was reported previously (Huang et al., 2020), with promoters used in this study. Specifically, the 35S promoter sequence was digested with BspEI and SalI from pC-CsU6-tRNA-3PDS. CsUbi or CmYLCV (Supplementary Data 1) was PCR amplified and inserted into the same site to replace the 35S promoter. PC-CsUbi-PDS and PC-CmYLCV-PDS constructs were obtained and confirmed by Sanger sequencing. To make the CsLOB1 EBE CRISPR construct, primers LOBPro3-F1/R1 and LOBPro3-F2/R2 (Supplementary Table 3) were used to make multiplex constructs using the transient vector pXH1-CsU6-tRNA via Golden gate cloning as described previously (Huang et al., 2020). Three gRNAs were included: one targeting type I EBE region of CsLOB1, one targeting type II EBE region of CsLOB1 (Jia et al., 2016, 2017b; Jia and Wang, 2020), and one targeting a region upstream of ATG. The construct PXH1-LOB1-Pro3 was confirmed by Sanger sequencing. The CmYLCV promoter sequence (Supplementary Data 1) was cut from PC-CmYLCV-PDS with SphI + SalI and inserted in the same site of PXH1-LOB1-Pro3 to replace the 35S promoter to make the transient expression construct PXH1-CmYLCV-LOB1-Pro3. This construct was used for protoplast transfection.
For stable transformation, the cassette was cut from PXH1-CmYLCV-LOB1-Pro3 with FspI and XbaI and inserted into the ZraI/XbaI site of the binary vector PCXH2, which carries the GFP expression cassette to make the final construct PCXH2-CmYLCV-LOB1-Pro3. This construct, after transforming into Agrobacterium strain EHA105, was used for Agrobacterium-mediated citrus epicotyl transformation.
For tobacco NtPDS gene editing, we also used the same tRNA-based multiplex vector as described above. The Cas9 is driven by CmYLCV promoter, and the gRNAs for NtPDS are driven by CsU6 promoter. Two gRNAs were designed (gRNA1: GAGATTGTTATTGCTGGTGC and gRNA2: GCTGCATGGAAAGATGATGA) based on the conserved regions between the two PDS homologs in the genome of allotetraploid species N. tabacum. The final CRISPR construct was transformed into Agrobacterium strain EHA105, which was used for stable transformation of tobacco leaf discs.
Transformation of CRISPR constructs into embryogenic citrus protoplasts was performed as described previously (Huang et al., 2020).
Agrobacterium-mediated transformation of citrus epicotyl was performed by following the protocol described previously (Jia et al., 2019b; Huang et al., 2020). After co-cultivation of epicotyls with Agrobacterium, the transformed epicotyls were incubated in regeneration medium at 30°C or at room temperature in the dark for 2 weeks before culturing under light at room temperature. For Carrizo PDS gene editing, two independent experiments were performed.
Since the transformation constructs carry the GFP expression cassette, positive transformants were selected under GFP filter in addition to antibiotic selection (Kanamycin). Citrus genomic DNA was extracted using the CTAB (cetyltrimethylammonium bromide) method (Pandey and Wang, 2019). Positive transformants were further confirmed by Cas9 amplification (primers in Supplementary Table 3). Detection of editing in the EBE region of CsLOB1 promoter was performed by amplifying the target region (primers in Supplementary Table 3) with high fidelity DNA polymerase Q5 (New England Biolabs, Ipswich, MA, United States), followed by cloning of PCR products and sequencing.
Xanthomonas citri subsp. citri wild-type strain 306 and dLOB2 containing Xcc pthA4:Tn5 (Yan and Wang, 2012; Hu et al., 2016; Teper et al., 2020) were suspended in 20 mM MgCl2 at 108 CFU/ml. The bacteria suspensions were syringe-infiltrated into young fully expanded leaves of wild-type sweet orange Hamlin sweet orange plants or mutant plants (Teper and Wang, 2021). Inoculated plants were kept in a temperature-controlled (28°C) glasshouse with high humidity. Pictures were taken 8 days post inoculation for disease resistance evaluation.
Leaves of wild-type and a biallelic mutant line #1 (hereafter named Ham1) with and without Xcc inoculation were sampled at 48 h post inoculation. Total RNA was extracted using the TRIzol reagent (Invitrogen, Carlsbad, CA, United States) following the manufacturer’s manual. Extracted RNA was further treated with RQ1 RNase-Free DNase (Promega, Madison, WI, United States) to remove genomic DNA contamination, if any. The treated RNA was further purified with RNeasy Mini Kit (QIAGEN, Germantown, MD, United States). One microgram of RNA from each sample was used for cDNA synthesis by using QuantiTect Reverse Transcription Kit (QIAGEN). Quantitative PCR was performed using SYBR Green PCR Master Mix in QuantStudio 3 Real-Time PCR System (Applied Biosystems, Foster City, CA, United States). The citrus house-keeping gene GAPDH was used as an endogenous control. Quantification of gene expression was calculated by following the comparative Ct method (Pfaffl, 2001). Primers for RT-qPCR are listed in Supplementary Table 2.
To analyze potential off-targets, we obtained the putative off-targets using a web-based software.1 The potential off-target sites with four or fewer mismatches were chosen for analysis. Genomic DNA from LOB1 EBE-edited Hamlin transgenic lines was used as template, and the primers listed in Supplementary Table 3 were used to amplify the fragments spanning the off-targets. Finally, the PCR products were subjected to Sanger sequencing to identify off-target.
The original contributions presented in the study are included in the article/Supplementary Material, further inquiries can be directed to the corresponding author.
XH and NW designed and organized the study. XH performed the experiments. YW contributed to off-target experiments and grafting. XH and NW prepared the manuscript.
This research was supported by the USDA National Institute of Food and Agriculture grants #2018-70016-27412, #2016-70016-24833, and #2019-70016-29796, USDA-NIFA Plant Biotic Interactions Program 2017-67013-26527, Florida Citrus Initiative, and Florida Citrus Research and Development Foundation.
The authors declare that the research was conducted in the absence of any commercial or financial relationships that could be construed as a potential conflict of interest.
All claims expressed in this article are solely those of the authors and do not necessarily represent those of their affiliated organizations, or those of the publisher, the editors and the reviewers. Any product that may be evaluated in this article, or claim that may be made by its manufacturer, is not guaranteed or endorsed by the publisher.
The Supplementary Material for this article can be found online at: https://www.frontiersin.org/articles/10.3389/fpls.2021.769907/full#supplementary-material
An, S. Q., Potnis, N., Dow, M., Vorhölter, F. J., He, Y. Q., Becker, A., et al. (2020). Mechanistic insights into host adaptation, virulence and epidemiology of the phytopathogen Xanthomonas. FEMS Microbiol. Rev. 44, 1–32. doi: 10.1093/femsre/fuz024
Benfey, P. N., Ren, L., and Chua, N. H. (1990). Tissue-specific expression from CaMV 35S enhancer subdomains in early stages of plant development. EMBO J. 9, 1677–1684.
Boch, J., and Bonas, U. (2010). Xanthomonas AvrBs3 family-type III effectors: discovery and function. Annu. Rev. Phytopathol. 48, 419–436. doi: 10.1146/annurev-phyto-080508-081936
Bové, J. M. (2006). Huanglongbing: a destructive, newly-emerging, century-old disease of citrus. J. Plant Path. 2006, 7–37.
Castel, B., Tomlinson, L., Locci, F., Yang, Y., and Jones, J. D. G. (2019). Optimization of T-DNA architecture for Cas9-mediated mutagenesis in Arabidopsis. PLoS One 14:0204778. doi: 10.1371/journal.pone.0204778
Cermak, T., Curtin, S. J., Gil-Humanes, J., Cegan, R., Kono, T. J. Y., Konecna, E., et al. (2017). A Multipurpose Toolkit to Enable Advanced Genome Engineering in Plants. Plant Cell 29, 1196–1217. doi: 10.1105/tpc.16.00922
Chen, L. Z., Li, W., Katin-Grazzini, L., Ding, J., Gu, X. B., Li, Y. J., et al. (2018). A method for the production and expedient screening of CRISPR/Cas9-mediated non-transgenic mutant plants. Horticult. Res. 5:4. doi: 10.1038/s41438-018-0023-4
Dang, Y., Jia, G. X., Choi, J., Ma, H. M., Anaya, E., Ye, C. T., et al. (2015). Optimizing sgRNA structure to improve CRISPR-Cas9 knockout efficiency. Genome Biol. 16:3. doi: 10.1186/s13059-015-0846-3
Demirer, G. S., Zhang, H., Goh, N. S., Gonzalez-Grandio, E., and Landry, M. P. (2019). Carbon nanotube-mediated DNA delivery without transgene integration in intact plants. Nat. Protoc. 14, 2954–2971. doi: 10.1038/s41596-019-0208-9
Dutt, M., El-Mohtar, C. A., and Wang, N. (2020). “Biotechnological Approaches for the Resistance to Citrus Diseases,” in The Citrus Genome, eds A. Gentile, S. La Malfa, and Z. Deng (Cham: Springer International Publishing), 245–257.
Eom, J. S., Luo, D. P., Atienza-Grande, G., Yang, J., Ji, C. H., Luu, V. T., et al. (2019). Diagnostic kit for rice blight resistance. Nat. Biotechnol. 37:1372. doi: 10.1038/s41587-019-0268-y
Favaro, M. A., Molina, M. C., Roeschlin, R. A., Gadea, J., Gariglio, N., and Marano, M. R. (2020). Different Responses in Mandarin Cultivars Uncover a Role of Cuticular Waxes in the Resistance to Citrus Canker. Phytopathology 110, 1791–1801. doi: 10.1094/PHYTO-02-20-0053-R
Fávero, P., de Assis Alves Mourão Filho, F., Stipp, L. C. L., and Mendes, B. M. J. (2012). Genetic transformation of three sweet orange cultivars from explants of adult plants. Acta Physiol. Plant. 34, 471–477. doi: 10.1007/s11738-011-0843-4
Ference, C. M., Gochez, A. M., Behlau, F., Wang, N., Graham, J. H., and Jones, J. B. (2018). Recent advances in the understanding of Xanthomonas citri ssp. citri pathogenesis and citrus canker disease management. Mol. Plant Pathol. 19, 1302–1318. doi: 10.1111/mpp.12638
Ference, C. M., Manthey, J. A., Narciso, J. A., Jones, J. B., and Baldwin, E. A. (2020). Detection of Phenylpropanoids in Citrus Leaves Produced in Response to Xanthomonas citri subsp. citri. Phytopathology 110, 287–296. doi: 10.1094/phyto-06-19-0219-r
Hassan, M. M., Zhang, Y., Yuan, G., De, K., Chen, J. G., Muchero, W., et al. (2021). Construct design for CRISPR/Cas-based genome editing in plants. Trends Plant. Sci. 26, 1133–1152.
Hu, J., and Wang, N. (2016). Evaluation of the Spatiotemporal Dynamics of Oxytetracycline and Its Control Effect against Citrus Huanglongbing via Trunk Injection. Phytopathology 106, 1495–1503.
Hu, Y., Duan, S., Zhang, Y., Shantharaj, D., Jones, J. B., and Wang, N. (2016). Temporal Transcription Profiling of Sweet Orange in Response to PthA4-Mediated Xanthomonas citri subsp. citri Infection. Phytopathology 106, 442–451. doi: 10.1094/PHYTO-09-15-0201-R
Hu, Y., Zhang, J. L., Jia, H. G., Sosso, D., Li, T., Frommer, W. B., et al. (2014). Lateral organ boundaries 1 is a disease susceptibility gene for citrus bacterial canker disease. Proc. Natl. Acad. Sci. U S A. 111, E521–E529. doi: 10.1073/pnas.1313271111
Huang, X., Wang, Y., Xu, J., and Wang, N. (2020). Development of multiplex genome editing toolkits for citrus with high efficacy in biallelic and homozygous mutations. Plant Mol. Biol. 104, 297–307. doi: 10.1007/s11103-020-01043-6
Jia, H., and Wang, N. (2014a). Targeted Genome Editing of Sweet Orange Using Cas9/sgRNA. PLoS One 9:e93806. doi: 10.1371/journal.pone.0093806
Jia, H., and Wang, N. (2014b). Xcc-facilitated agroinfiltration of citrus leaves: a tool for rapid functional analysis of transgenes in citrus leaves. Plant Cell Rep. 33, 1993–2001. doi: 10.1007/s00299-014-1673-9
Jia, H., and Wang, N. (2020). Generation of homozygous canker-resistant citrus in the T0 generation using CRISPR-SpCas9p. Plant Biotechnol. J. 18, 1990–1992. doi: 10.1111/pbi.13375
Jia, H., Omar, A., Orbovic, V., and Wang, N. (2021). Biallelic editing of the LOB1 promoter via CRISPR/Cas9 creates canker-resistant ‘Duncan’ grapefruit. Phytopathology[Preprint]. doi: 10.1094/PHYTO-04-21-0144-R
Jia, H., Orbovic, V., and Wang, N. (2019a). CRISPR-LbCas12a-mediated modification of citrus. Plant Biotechnol. J. 17, 1928–1937. doi: 10.1111/pbi.13109
Jia, H., Orbovic, V., Jones, J. B., and Wang, N. (2016). Modification of the PthA4 effector binding elements in Type I CsLOB1 promoter using Cas9/sgRNA to produce transgenic Duncan grapefruit alleviating XccΔpthA4:dCsLOB1.3 infection. Plant Biotechnol. J. 14, 1291–1301. doi: 10.1111/pbi.12495
Jia, H., Xu, J., Orbović, V., Zhang, Y., and Wang, N. (2017a). Editing Citrus Genome via SaCas9/sgRNA System. Front. Plant Sci. 8:2135. doi: 10.3389/fpls.2017.02135
Jia, H., Zhang, Y., Orbović, V., Xu, J., White, F. F., Jones, J. B., et al. (2017b). Genome editing of the disease susceptibility gene CsLOB1 in citrus confers resistance to citrus canker. Plant Biotechnol. J. 15, 817–823. doi: 10.1111/pbi.12677
Jia, H., Zou, X., Orbovic, V., and Wang, N. (2019b). “Genome Editing in Citrus Tree with CRISPR/Cas9,” in Plant Genome Editing with CRISPR Systems, Vol. 1917, ed. Y. Qi (New York, NY: Humana Press), doi: 10.1007/978-1-4939-8991-1_17
LeBlanc, C., Zhang, F., Mendez, J., Lozano, Y., Chatpar, K., Irish, V., et al. (2018). Increased efficiency of targeted mutagenesis by CRISPR/Cas9 in plants using heat stress. Plant J. 93, 377–386. doi: 10.1111/tpj.13782
Li, H. (2009). Citrus tree abiotic and biotic stress and implication of simulation and modeling tools in tree management. J. Sustainable For. 3, 66–78.
Li, J. F., Zhang, D., and Sheen, J. (2014). Cas9-based genome editing in Arabidopsis and tobacco. Methods Enzymol. 546, 459–472. doi: 10.1016/B978-0-12-801185-0.00022-2
Li, J., Kolbasov, V., Lee, D., Pang, Z., Yixiao, H., Collins, N., et al. (2020). Residue dynamics of streptomycin in citrus delivered by foliar spray and trunk injection and effect on ‘Candidatus Liberibacter asiaticus’ titer. Phytopathology 111, 1095–1103.
Li, J., Pang, Z., Duan, S., Lee, D., Kolbasov, V., and Wang, N. (2019). The in planta effective concentration of oxytetracycline against Candidatus Liberibacter asiaticus for suppression of citrus Huanglongbing. Phytopathology 109, 2046–2054.
Li, T., Liu, B., Spalding, M. H., Weeks, D. P., and Yang, B. (2012). High-efficiency TALEN-based gene editing produces disease-resistant rice. Nat. Biotechnol. 30, 390–392. doi: 10.1038/nbt.2199
Lin, C. S., Hsu, C. T., Yang, L. H., Lee, L. Y., Fu, J. Y., Cheng, Q. W., et al. (2018). Application of protoplast technology to CRISPR/Cas9 mutagenesis: from single-cell mutation detection to mutant plant regeneration. Plant Biotechnol. J. 16, 1295–1310. doi: 10.1111/pbi.12870
Lv, Z. Y., Jiang, R., Chen, J. F., and Chen, W. S. (2020). Nanoparticle-mediated gene transformation strategies for plant genetic engineering. Plant J. 104, 880–891. doi: 10.1111/tpj.14973
Malzahn, A., Tang, X., Lee, K., Ren, Q., Sretenovic, S., Zhang, Y., et al. (2019). Application of CRISPR-Cas12a temperature sensitivity for improved genome editing in rice, maize, and Arabidopsis. BMC Biol. 17:9. doi: 10.1186/s12915-019-0629-5
Milner, M., Craze, M., Hope, M., and Wallington, E. (2020). Turning Up the Temperature on CRISPR: Increased Temperature Can Improve the Editing Efficiency of Wheat Using CRISPR/Cas9. Front. Plant Sci. 11:583374. doi: 10.3389/fpls.2020.583374
Moscou, M. J., and Bogdanove, A. J. (2009). A simple cipher governs DNA recognition by TAL effectors. Science 326:1501. doi: 10.1126/science.1178817
Oliva, R., Ji, C., Atienza-Grande, G., Huguet-Tapia, J. C., Perez-Quintero, A., Li, T., et al. (2019). Broad-spectrum resistance to bacterial blight in rice using genome editing. Nat. Biotechnol. 37, 1344–1350. doi: 10.1038/s41587-019-0267-z
Omura, M., and Shimada, T. (2016). Citrus breeding, genetics and genomics in Japan. Breed. Sci. 66, 3–17.
Ordon, J., Bressan, M., Kretschmer, C., Dall’Osto, L., Marillonnet, S., Bassi, R., et al. (2020). Optimized Cas9 expression systems for highly efficient Arabidopsis genome editing facilitate isolation of complex alleles in a single generation. Funct. Integrat. Genomics 20, 151–162. doi: 10.1007/s10142-019-00665-4
Ow, D. W., Jacobs, J. D., and Howell, S. H. (1987). Functional regions of the cauliflower mosaic virus 35S RNA promoter determined by use of the firefly luciferase gene as a reporter of promoter activity. Proc. Natl. Acad. Sci. U S A. 84, 4870–4874. doi: 10.1073/pnas.84.14.4870
Pandey, S. S., and Wang, N. (2019). Targeted Early Detection of Citrus Huanglongbing Causal Agent ‘Candidatus Liberibacter asiaticus’ Before Symptom Expression. Phytopathology 109, 952–959. doi: 10.1094/phyto-11-18-0432-r
Pena, L., Cervera, M., Juárez, J., Ortega, C., Pina, J., Durán-Vila, N., et al. (1995). High efficiency Agrobacterium-mediated transformation and regeneration of citrus. Plant Sci. 104, 183–191. doi: 10.1016/0168-9452(94)04021-8
Peng, A. H., Chen, S. C., Lei, T. G., Xu, L. Z., He, Y. R., Wu, L., et al. (2017). Engineering canker-resistant plants through CRISPR/Cas9-targeted editing of the susceptibility gene CsLOB1 promoter in citrus. Plant Biotechnol. J. 15, 1509–1519. doi: 10.1111/pbi.12733
Perez-Quintero, A. L., and Szurek, B. (2019). A Decade Decoded: Spies and Hackers in the History of TAL Effectors Research. Annu. Rev. Phytopathol. 57, 459–481. doi: 10.1146/annurev-phyto-082718-100026
Pfaffl, M. W. (2001). A new mathematical model for relative quantification in real-time RT-PCR. Nucleic Acids Res. 29:e45. doi: 10.1093/nar/29.9.e45
Poles, L., Licciardello, C., Distefano, G., Nicolosi, E., Gentile, A., and La Malfa, S. (2020). Recent Advances of In Vitro Culture for the Application of New Breeding Techniques in Citrus. Plants 9:lants9080938. doi: 10.3390/plants9080938
Qi, X., Dong, L., Liu, C., Mao, L., Liu, F., Zhang, X., et al. (2018). Systematic identification of endogenous RNA polymerase III promoters for efficient RNA guide-based genome editing technologies in maize. Crop J. 6, 314–320. doi: 10.1016/j.cj.2018.02.005
Ren, C., Liu, Y., Guo, Y., Duan, W., Fan, P., Li, S., et al. (2021). Optimizing the CRISPR/Cas9 system for genome editing in grape by using grape promoters. Horticult. Res. 8:52. doi: 10.1038/s41438-021-00489-z
Ribeiro, C., Xu, J., Teper, D., Lee, D., and Wang, N. (2021). The transcriptome landscapes of citrus leaf in different developmental stages. Plant Mol. Biol. 106, 349–366.
Römer, P., Strauss, T., Hahn, S., Scholze, H., Morbitzer, R., Grau, J., et al. (2009). Recognition of AvrBs3-like proteins is mediated by specific binding to promoters of matching pepper Bs3 alleles. Plant Physiol. 150, 1697–1712. doi: 10.1104/pp.109.139931
Stavolone, L., Kononova, M., Pauli, S., Ragozzino, A., de Haan, P., Milligan, S., et al. (2003). Cestrum yellow leaf curling virus (CmYLCV) promoter: a new strong constitutive promoter for heterologous gene expression in a wide variety of crops. Plant Mol. Biol. 53, 703–713. doi: 10.1023/B:PLAN.0000019110.95420.bb
Sun, X., Hu, Z., Chen, R., Jiang, Q., Song, G., Zhang, H., et al. (2015). Targeted mutagenesis in soybean using the CRISPR-Cas9 system. Sci. Rep. 5:10342. doi: 10.1038/srep10342
Svitashev, S., Schwartz, C., Lenderts, B., Young, J., and Cigan, A. (2016). Genome editing in maize directed by CRISPR–Cas9 ribonucleoprotein complexes. Nat. Commun. 7:13274.
Swarup, S., Defeyter, R., Brlansky, R., and Gabriel, D. (1991). A pathogenicity locus from Xanthomonas Citri enables strains from several pathovars of Xanthomonas Campestris to elicit canker like lesions on citrus. Phytopathology 81, 802–809.
Swarup, S., Yang, Y., Kingsley, M., and Gabriel, D. (1992). An Xanthomonas citri pathogenicity gene, pthA, pleiotropically encodes gratuitous avirulence on nonhosts. Mol. Plant Microbe Interact. 5, 204–213.
Teper, D., and Wang, N. (2021). Consequences of adaptation of TAL effectors on host susceptibility to Xanthomonas. PLoS Genet. 17:e1009310. doi: 10.1371/journal.pgen.1009310
Teper, D., Xu, J., Li, J. Y., and Wang, N. (2020). The immunity of Meiwa kumquat against Xanthomonas citri is associated with a known susceptibility gene induced by a transcription activator-like effector. PLoS Pathog. 16:1008886. doi: 10.1371/journal.ppat.1008886
Tsutsui, H., and Higashiyama, T. (2017). pKAMA-ITACHI Vectors for Highly Efficient CRISPR/Cas9-Mediated Gene Knockout in Arabidopsis thaliana. Plant Cell Physiol. 58, 46–56. doi: 10.1093/pcp/pcw191
Veillet, F., Perrot, L., Chauvin, L., Kermarrec, M. P., Guyon-Debast, A., Chauvin, J. E., et al. (2019). Transgene-Free Genome Editing in Tomato and Potato Plants Using Agrobacterium-Mediated Delivery of a CRISPR/Cas9 Cytidine Base Editor. Int. J. Mol. Sci. 20:ijms20020402. doi: 10.3390/ijms20020402
Wang, N. (2019). The Citrus Huanglongbing Crisis and Potential Solutions. Mol. Plant 12, 607–609. doi: 10.1016/j.molp.2019.03.008
Wang, Z. P., Xing, H. L., Dong, L., Zhang, H. Y., Han, C. Y., Wang, X. C., et al. (2015). Egg cell-specific promoter-controlled CRISPR/Cas9 efficiently generates homozygous mutants for multiple target genes in Arabidopsis in a single generation. Genome Biol. 16:144. doi: 10.1186/s13059-015-0715-0
Weiss, T., Wang, C. F., Kang, X. J., Zhao, H., Gamo, M. E., Starker, C. G., et al. (2020). Optimization of multiplexed CRISPR/Cas9 system for highly efficient genome editing in Setaria viridis. Plant J. 104, 828–838. doi: 10.1111/tpj.14949
Wheatley, M., and Yang, Y. (2020). Versatile Applications of the CRISPR/Cas Toolkit in Plant Pathology and Disease Management. Phytopathology 111, 1080–1090. doi: 10.1094/PHYTO-08-20-0322-IA
Woo, J., Kim, J., Kwon, S., et al. (2015). DNA-free genome editing in plants with preassembled CRISPR-Cas9 ribonucleoproteins. Nat. Biotechnol. 33, 1162–1164.
Wu, G. A., Terol, J., Ibanez, V., López-García, A., Pérez-Román, E., Borredá, C., et al. (2018). Genomics of the origin and evolution of Citrus. Nature 554, 311–316. doi: 10.1038/nature25447
Xiang, G., Zhang, X., An, C., Cheng, C., and Wang, H. (2017). Temperature effect on CRISPR-Cas9 mediated genome editing. J. Genet. Genomics 44, 199–205. doi: 10.1016/j.jgg.2017.03.004
Xie, K., Minkenberg, B., and Yang, Y. (2015). Boosting CRISPR/Cas9 multiplex editing capability with the endogenous tRNA-processing system. Proc. Natl. Acad. Sci. U S A. 2015, 3570–3575.
Yan, L. H., Wei, S. W., Wu, Y. R., Hu, R. L., Li, H. J., Yang, W. C., et al. (2015). High-Efficiency Genome Editing in Arabidopsis Using YAO Promoter-Driven CRISPR/Cas9 System. Mol. Plant 8, 1820–1823. doi: 10.1016/j.molp.2015.10.004
Yan, Q., and Wang, N. (2012). High-throughput screening and analysis of genes of Xanthomonas citri subsp. citri involved in citrus canker symptom development. Mol. Plant Microbe Interact. 25, 69–84. doi: 10.1094/MPMI-05-11-0121
Yuan, X., Chen, C., Bassanezi, R., Wu, F., Feng, Z., Shi, D., et al. (2020). Region-wide comprehensive implementation of roguing infected trees, tree replacement, and insecticide applications successfully controls citrus HLB. Phytopathology 111, 1361–1368.
Zhang, F., LeBlanc, C., Irish, V. F., and Jacob, Y. (2017a). Rapid and efficient CRISPR/Cas9 gene editing in Citrus using the YAO promoter. Plant Cell Rep. 36, 1883–1887.
Zhang, J. L., Huguet-Tapia, J. C., Hu, Y., Jones, J., Wang, N., Liu, S. Z., et al. (2017b). Homologues of CsLOB1 in citrus function as disease susceptibility genes in citrus canker. Mol. Plant Pathol. 18, 798–810. doi: 10.1111/mpp.12441
Zhang, M., He, Z., Calvert, D. V., Stoffella, P. J., and Yang, X. (2003). Surface runoff losses of copper and zinc in sandy soils. J. Environ. Qual. 32, 909–915.
Zhang, Y., Iaffaldano, B., and Qi, Y. (2021). CRISPR ribonucleoprotein-mediated genetic engineering in plants. Plant Commun. 2:100168. doi: 10.1016/j.xplc.2021.100168
Keywords: CRISPR/Cas9, citrus, canker, HLB, CsLOB1, susceptibility gene, EBE, transcription activator-like effector
Citation: Huang X, Wang Y and Wang N (2022) Highly Efficient Generation of Canker-Resistant Sweet Orange Enabled by an Improved CRISPR/Cas9 System. Front. Plant Sci. 12:769907. doi: 10.3389/fpls.2021.769907
Received: 02 September 2021; Accepted: 09 December 2021;
Published: 11 January 2022.
Edited by:
Maria Raffaella Ercolano, University of Naples Federico II, ItalyReviewed by:
Md Mahmudul Hassan, Patuakhali Science and Technology University, BangladeshCopyright © 2022 Huang, Wang and Wang. This is an open-access article distributed under the terms of the Creative Commons Attribution License (CC BY). The use, distribution or reproduction in other forums is permitted, provided the original author(s) and the copyright owner(s) are credited and that the original publication in this journal is cited, in accordance with accepted academic practice. No use, distribution or reproduction is permitted which does not comply with these terms.
*Correspondence: Nian Wang, bmlhbndhbmdAdWZsLmVkdQ==
†ORCID: Xiaoen Huang, orcid.org/0000-0002-7514-1663; Nian Wang, orcid.org/0000-0001-7743-0728
Disclaimer: All claims expressed in this article are solely those of the authors and do not necessarily represent those of their affiliated organizations, or those of the publisher, the editors and the reviewers. Any product that may be evaluated in this article or claim that may be made by its manufacturer is not guaranteed or endorsed by the publisher.
Research integrity at Frontiers
Learn more about the work of our research integrity team to safeguard the quality of each article we publish.