Introduction
Stomata are the main gateways for the entry of microbial pathogens into leaves (Melotto et al., 2008). However, some try to use hydathodes (Hugouvieux et al., 1998) or breach the cuticle (Grimmer et al., 2012). Stomatal closure, therefore, is an effective measure to restrict pathogen entry and provide the plants an innate immunity (Melotto et al., 2008, 2017; Sawinski et al., 2013; Bharath et al., 2021). Stomata open when the guard cells are turgid and close when guard cells are flaccid (Willmer and Fricker, 1996). Whenever plants are exposed to stress, the guard cells sense and respond by a series of steps that include the production of ROS and NO followed by a rise in Ca2+ and the modulation of ion channels. These events promote the efflux of cations and anions from guard cells. As a result, guard cells lose turgor leading to stomatal closure (Arnaud and Hwang, 2015; Agurla et al., 2018; Saito and Uozumi, 2019; Hsu et al., 2021).
The reopening of stomata is usually slower than the closure, ensuring that the leaves conserve water for an extended period. For example, abscisic acid (ABA)-induced stomatal closure in the epidermis took about 30 min (and in leaf 3 h). In contrast, recovery took 1–6 days, implying short-term and long-term effects on stomata (Liang and Zhang, 1999). Recently, we pointed out that the stomatal closure by ABA was an essential component of plant adaptation to stress factors (Bharath et al., 2021). This article proposes that the initial stomatal closure response triggers many defensive strategies to fight the pathogens. We describe the follow-up of events limiting pathogen spread and emphasize stomata's role in ensuring plants' long-term adaptation against microbes.
Stomatal Closure: An Immediate Barrier of Microbial Entry
Stomatal closure was a typical response against microbial attack (Arnaud and Hwang, 2015; Melotto et al., 2017; Agurla et al., 2018). The process of stomatal closure is initiated by sensing the abiotic (e.g., drought, chilling, and UV-B) or biotic stress (pathogens and insects) components (Agurla et al., 2018). Most microbial pathogens produce pathogen-or microbe-or damage-associated molecular patterns (PAMPs/MAMPs/DAMPs), perceived by pattern recognition receptors (PRRs) present on the plant plasma membrane. Upon perception, plants activate a defense response called pattern-triggered immunity (PTI). When pathogens attempt to overcome PTI, plants trigger effector-triggered immunity (Cui et al., 2015; Nguyen et al., 2021). Bacterial elicitors that trigger stomatal closure include flagellin22 (flg22), lipopolysaccharide, and other elicitor peptides, such as, elf26 (Melotto et al., 2008, 2017; Arnaud and Hwang, 2015). Fungal elicitors, such as, chitin oligosaccharide and chitosan, also induced defense responses in plants (Ye et al., 2020).
Guard cells perceive hormones (e.g., ABA) or elicitors (flg22) by their respective receptors. Upon binding to ABA or flg22, the receptor kinases (e.g., open stomata 1 or botrytis-induced kinase 1) activate RbohD/F and stimulate reactive oxygen species (ROS) production during stomatal closure. However, the role of RBOHD in resistance against pathogens, particularly during pre-invasive stage is not clear. The elevated ROS, in turn, trigger a rise in nitric oxide (NO) and Ca2+. The interaction of these secondary messengers (ROS/NO/Ca2+) regulates the downstream components in guard cells. Both NO and Ca2+ (via Ca2+-dependent protein kinases) promote the ion efflux by activating K+ out, SLAC1, and SLAH3 channels and at the same time inhibit the K-influx channel (Arnaud and Hwang, 2015; Agurla et al., 2018; Kohli et al., 2019; Sun et al., 2019). Similarly, ROS and Ca2+ activate Ca2+ influx and increase cytosolic Ca2+ levels (Klüsener et al., 2002). The elevated ROS, NO, Ca2+ and H2S provide an extended pathogen resistance (Gahir et al., 2020; Liu and Xue, 2021). Cytosolic pH is another secondary messenger that preceded the production of ROS and NO in guard cells, but the exact mechanism is ambiguous (Gonugunta et al., 2009; Bharath et al., 2021). It is necessary to study if such changes in pHcyt can modulate the pathogen resistance as well.
Stomatal Closure Associated With the Modulation of Plant Hormones
Stomatal closure during drought or microbial infection was associated with an increase in plant hormones. Salicylic acid (SA), ABA, methyl jasmonate (MJ), and ethylene (ET) accumulate when microbes attack plants. The concerted action of these hormones causes stomatal closure and induces systemic resistance (Gimenez-Ibanez et al., 2016; van Butselaar and Van den Ackerveken, 2020; Bharath et al., 2021). The modulated hormonal status provides long-term protection to plants against biotic and abiotic stress (Described below).
Discussion
Closure Triggers a Network of Long-Term Events to Ensure the Protection
Stomatal closure in response to microbial infection is an immediate physical measure to prevent microbial entry. However, such closure has long-term effects, such as, a marked decrease in the intercellular CO2 of leaves, a reduction in photosynthetic carbon assimilation, and an elevation in photo respiratory activity. The reduction in transpiration can cause mineral deficiency in leaves. We describe below the consequences of these events and a few associated components.
Decrease in Photosynthesis and Increase in Photorespiration and Peroxisomal Population
When stomata close, the intercellular CO2 is lowered, and transpiration decreased, raising the leaf temperature. Both these factors enhance photorespiration. The increase in photorespiration occurred under conditions of biotic (microbial infection) or abiotic stress (drought) (Lal et al., 1996; Pascual et al., 2010; Voss et al., 2013; Vo et al., 2021). Even fluctuations in transpiration triggered an increase in photorespiration (Furutani et al., 2020). The enhanced photorespiration and the associated rise in H2O2 could confer disease resistance (Taler et al., 2004; Kubo, 2013; Sørhagen et al., 2013). Further, glycolate, glyoxylate, and glycine, being pathway intermediates, accumulate. Glycolate and glyoxylate are toxic to living cells and can double up as antimicrobial compounds. Glycine is the precursor of glutathione, an essential anti-oxidant in plant cells. Photo respiratory enzymes/metabolites mediated the plant defense during tomato-Pseudomonas syringae interactions (Ahammed et al., 2018). Thus, photo respiratory metabolism could help to resist pathogens.
The enhanced photorespiration was often associated with an increase in the peroxisomal population in leaf cells (Chen et al., 2016). Peroxisomal ROS could protect against plant pathogens (Sørhagen et al., 2013). Besides ROS, other components of peroxisomes, namely NO, Ca2+, and polyamines (PA), upregulated the genes involved in SA signaling and PA catabolism, reinforcing plant defense responses (Chen et al., 2016; Wang et al., 2019).
Stomatal Closure Lowers Leaf Sugars
Stomatal closure, whether due to pathogen attack or drought, causes reduced CO2 assimilation and decreased carbon partitioning into sucrose and starch (Wang et al., 2016; Haider et al., 2017). The pathogens required sugars for growth and infection (Solomon et al., 2003; Scharte et al., 2005; Chang et al., 2017). If sufficient sucrose is not available, the extent of proliferation would be restricted (Huai et al., 2020). Therefore, the deficiency in sugar availability lead to decreased fungal growth (Bezrutczyk et al., 2018).
Reduced Transpiration Creates Mineral Deficiency
Transpiration is a prerequisite for long-distance transport of minerals (Ruiz and Romero, 2002). A deficiency of minerals would occur when stomata are closed. There was a positive relationship between the transpiration rate and mineral content of sunflower (Helianthus annuus) and maize leaves (Tanner and Beevers, 2001; Shrestha et al., 2021). Since microbial spread and multiplication within leaves depend on macronutrients/micronutrients, the mineral deficiency could affect microbial growth and enhance pathogen tolerance (Fernández-Escobar, 2019). The N-status of leaves modulated defense-related hormones, NO content, and then genes (Sun et al., 2020). The deficiency of N increased the levels of phenolics and restricted the spread of powdery mildew (Bavaresco and Eibach, 1987). A similar situation under K+-deficiency was reported with leaf spot, caused by Helminthosporium cynodontis (Richardson and Croughan, 1989). Other examples of mineral deficiency that favor pathogen resistance were zinc (Cabot et al., 2019) and iron (Trapet et al., 2021). Readers can find a detailed description of the dual role of the macro-and micronutrients for the infection by bacterial and fungal pathogens elsewhere (Huber et al., 2012).
Continuing Effects of Secondary Messengers, Plant Hormones, and Secondary Metabolites
The secondary messengers produced during stomatal closure can continue to protect plants. For example, the combination of ROS/NO/Ca2+ was quite effective in limiting the spread and multiplication of microbes within the leaf. These secondary messengers trigger hypersensitive response (HR), synthesis of pathogenesis-related (PR) proteins, and programmed cell death (PCD) (Serrano et al., 2015; Marcec et al., 2019). Besides NO, H2S produced during stomatal closure could confer pathogen resistance (Vojtovič et al., 2020). It is possible that these components ROS/NO/Ca2+ can also induce priming effect individually or in combination.
When plants were infected by pathogens, the leaves responded by modulating the hormones, which interacted with each other to impart a long-lasting response. Plant hormones (e.g., SA, methyl salicylate, MJ) and even PAs could induce systemic resistance (Bürger and Chory, 2019; Chen et al., 2019; Seifi et al., 2019; Yuan et al., 2019). These hormones (ABA/MJ/SA) primed the plant tissue to stand against pathogens (Agostini et al., 2019; Feng et al., 2020). These observations open up several exciting lines of work for further research.
Several secondary metabolites produced by the plants are prominently associated with protection against bacterial, fungal, and viral attacks. The elevated levels of H2O2, NO, and Ca2+ induced accumulation of secondary metabolites like wax, callose, alkaloids, flavonoids, phenols, and PAs, reinforcing the protection against infection (Walters, 2003; Luna et al., 2011; Zaynab et al., 2018; Lewandowska et al., 2020). The PAs also prime the plants against Botrytis (Janse van Rensburg et al., 2021). Similarly, allyl isothiocyanate (AITC) keeps microbes like P. syringae out by inducing stomatal closure (Bednarek, 2012).
Conclusion and Future Perspective
Stomatal closure erects a physical barrier providing immediate relief against the entry of microbial pathogens into leaves while decreasing the rates of photosynthesis and transpiration. The closure has long-term consequences (Figure 1). The restricted CO2 supply to the mesophyll cells lowers the rate of photosynthesis, stimulates photorespiration and associated H2O2 production. The elevated levels of H2O2, along with NO, H2S, and Ca2+, can upregulate genes involved in HR, PR, and PCD to prevent the spread of pathogens within the leaf. These reactive molecules also promote the accumulation of antimicrobial secondary metabolites. Parallelly, reduced transpiration creates mineral deficiency and limits microbial growth. We suggest that stomatal closure is a trigger to set off long-term events involved in prolonged plant disease resistance.
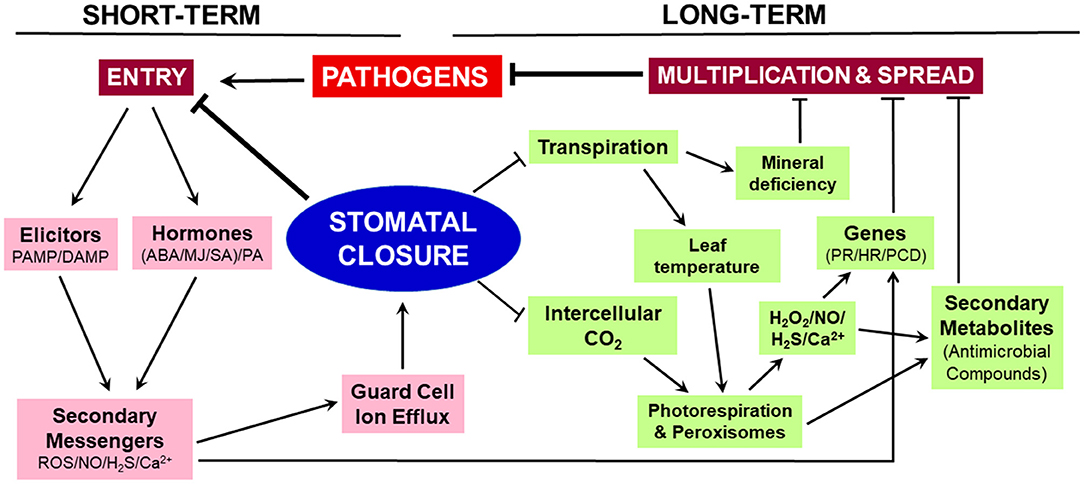
Figure 1. Schematic representation of events associated with stomatal closure. The closure restricts microbial entry during the short term, while the events initiated during closure contribute to long-term adaptation against microbes. On sensing the microbial elicitor molecules, the guard cells trigger a rise in ROS, NO, Ca2+, and H2S. These secondary messengers cause ion-efflux from guard cells and stomatal closure. As a consequence of closure, the entry of CO2 and transpirational H2O loss are restricted, leading to increased photorespiration, decreased leaf sugars, and mineral deficiency. All these events help in restricting microbial multiplication, spread, and growth. The disturbed hormonal status and reactive molecules ensure continued protection by upregulating PR/HR/PCD genes and promoting the levels of antimicrobial secondary metabolites. Arrow represents an increase and ⊣ indicates a decrease.
We know that stomatal closure may not be a universal mechanism to fight the microbial attack, e.g., root or stem pathogens. But several pathogens are air-borne and land on leaves (Melotto et al., 2008; Zeng et al., 2010). Plant-microbe interactions are not unilateral since the pathogens try to reopen stomata using compounds, such as, coronatine (Arnaud and Hwang, 2015). Further work is needed to understand the implications of stomatal closure on the antagonizing responses by the pathogens. Peroxisomal H2O2 limits microbial growth, but there are instances when microbes use peroxisomes to their advantage (Kubo, 2013). An improved understanding of peroxisomes and manipulation through biotechnological techniques could open up possibilities of designing plants for long-term adaptation to stress conditions. We believe that stomatal guard cells are ideal for studying plants' short-and long-term responses to challenging stress situations. Stomatal closure can be exploited to improve crop growth and grain yield under environmental stress conditions. In crops such as, wheat and rice, reduced water requirement due to stomatal closure was used as one of the physiological traits in crop breeding (Park et al., 2020; Paul et al., 2020). Further studies on the long-term effects of stomatal closure can be translated into additional field applications.
Author Contributions
AR conceptualized the idea, prepared the outline, and edited the final version. SG, PB, and AR pooled relevant literature, wrote the manuscript, and approved the final manuscript. All authors contributed to the article and approved the submitted version.
Funding
The stomatal work in our laboratory was supported by a grant (to AR) of the Council of Scientific and Industrial Research [No. 38 (1404)/15/EMR-II]. SG was supported by a Senior Research Fellowship from University Grant Commission, New Delhi. PB was supported partially by a University of Hyderabad BBL fellowship.
Conflict of Interest
The authors declare that the research was conducted in the absence of any commercial or financial relationships that could be construed as a potential conflict of interest.
Publisher's Note
All claims expressed in this article are solely those of the authors and do not necessarily represent those of their affiliated organizations, or those of the publisher, the editors and the reviewers. Any product that may be evaluated in this article, or claim that may be made by its manufacturer, is not guaranteed or endorsed by the publisher.
References
Agostini, R. B., Postigo, A., Rius, S. P., Rech, G. E., Campos-Bermudez, V. A., and Vargas, W. A. (2019). Long-lasting primed state in maize plants: salicylic acid and steroid signaling pathways as key players in the early activation of immune responses in silks. Mol. Plant Microbe Interact. 32, 95–106. doi: 10.1094/MPMI-07-18-0208-R
Agurla, S., Gahir, S., Munemasa, S., Murata, Y., and Raghavendra, A. S. (2018). Mechanism of stomatal closure in plants exposed to drought and cold stress. Adv. Exp. Med. Biol. 1081, 215–232. doi: 10.1007/978-981-13-1244-1_12
Ahammed, G. J., Li, X., Zhang, G., Zhang, H., Shi, J., Pan, C., et al. (2018). Tomato photorespiratory glycolate-oxidase-derived H2O2 production contributes to basal defence against Pseudomonas syringae. Plant Cell Environ. 41, 1126–1138. doi: 10.1111/pce.12932
Arnaud, D., and Hwang, I. (2015). A sophisticated network of signaling pathways regulates stomatal defenses to bacterial pathogens. Mol. Plant 8, 566–581. doi: 10.1016/j.molp.2014.10.012
Bavaresco, L., and Eibach, R. (1987). Investigations on the influence of N fertilizer on resistance to powdery mildew (Oidium tuckeri) downy mildew (Plasmopara viticola) and on phytoalexin synthesis in different grapevine varieties. Vitis 26, 192–200.
Bednarek, P. (2012). Chemical warfare or modulators of defence responses—the function of secondary metabolites in plant immunity. Curr. Opin. Plant Biol. 15, 407–414. doi: 10.1016/j.pbi.2012.03.002
Bezrutczyk, M., Yang, J., Eom, J. S., Prior, M., Sosso, D., Hartwig, T., et al. (2018). Sugar flux and signaling in plant-microbe interactions. Plant J. 93, 675–685. doi: 10.1111/tpj.13775
Bharath, P., Gahir, S., and Raghavendra, A. S. (2021). Abscisic acid-induced stomatal closure: an important component of plant defense against abiotic and biotic stress. Front. Plant Sci. 12:615114. doi: 10.3389/fpls.2021.615114
Bürger, M., and Chory, J. (2019). Stressed out about hormones: how plants orchestrate immunity. Cell Host Microbe. 26, 163–172. doi: 10.1016/j.chom.2019.07.006
Cabot, C., Martos, S., Llugany, M., Gallego, B., Tolrà, R., and Poschenrieder, C. (2019). A role for zinc in plant defense against pathogens and herbivores. Front. Plant Sci. 10:1171. doi: 10.3389/fpls.2019.01171
Chang, Q., Liu, J., Lin, X., Hu, S., Yang, Y., Li, D., et al. (2017). A unique invertase is important for sugar absorption of an obligate biotrophic pathogen during infection. New Phytol. 215, 1548–1561. doi: 10.1111/nph.14666
Chen, L., Wang, W. S., Wang, T., Meng, X. F., Chen, T. T., Huang, X. X., et al. (2019). Methyl salicylate glucosylation regulates plant defense signaling and systemic acquired resistance. Plant Physiol. 180, 2167–2181. doi: 10.1104/pp.19.00091
Chen, X. L., Wang, Z., and Liu, C. (2016). Roles of peroxisomes in the rice blast fungus. Biomed Res. Int. 2016:9343417. doi: 10.1155/2016/9343417
Cui, H., Tsuda, K., and Parker, J. E. (2015). Effector-triggered immunity: from pathogen perception to robust defense. Ann. Rev. Plant Biol. 66, 487–511. doi: 10.1146/annurev-arplant-050213-040012
Feng, J., Zhang, M., Yang, K. N., and Zheng, C. X. (2020). Salicylic acid-primed defence response in octoploid strawberry “Benihoppe” leaves induces resistance against Podosphaera aphanis through enhanced accumulation of proanthocyanidins and upregulation of pathogenesis-related genes. BMC Plant Biol. 20:149. doi: 10.1186/s12870-020-02353-z
Fernández-Escobar, R. (2019). Olive nutritional status and tolerance to biotic and abiotic stresses. Front. Plant Sci. 10:1151. doi: 10.3389/fpls.2019.01151
Furutani, R., Makino, A., Suzuki, Y., Wada, S., Shimakawa, G., and Miyake, C. (2020). Intrinsic fluctuations in transpiration induce photorespiration to oxidize P700 in photosystem I. Plants 9:1761. doi: 10.3390/plants9121761
Gahir, S., Bharath, P., and Raghavendra, A. S. (2020). The role of gasotransmitters in movement of stomata: mechanisms of action and importance for plant immunity. Biol. Plant. 64, 623–632. doi: 10.32615/bp.2020.071
Gimenez-Ibanez, S., Chini, A., and Solano, R. (2016). How microbes twist jasmonate signaling around their little fingers. Plants 5:9. doi: 10.3390/plants5010009
Gonugunta, V. K., Srivastava, N., and Raghavendra, A. S. (2009). Cytosolic alkalinization is a common and early messenger preceding the production of ROS and NO during stomatal closure by variable signals, including abscisic acid, methyl jasmonate and chitosan. Plant Signal. Behav. 4, 561–564. doi: 10.4161/psb.4.6.8847
Grimmer, M. K., John Foulkes, M., and Paveley, N. D. (2012). Foliar pathogenesis and plant water relations: a review. J. Exp. Bot. 63, 4321–4331. doi: 10.1093/jxb/ers143
Haider, M. S., Kurjogi, M. M., Khalil-Ur-Rehman, M., Fiaz, M., Pervaiz, T., Jiu, S., et al. (2017). Grapevine immune signaling network in response to drought stress as revealed by transcriptomic analysis. Plant Physiol. Biochem. 121, 187–195. doi: 10.1016/j.plaphy.2017.10.026
Hsu, P. K., Dubeaux, G., Takahashi, Y., and Schroeder, J. I. (2021). Signaling mechanisms in abscisic acid-mediated stomatal closure. Plant J. 105, 307–321. doi: 10.1111/tpj.15067
Huai, B., Yang, Q., Wei, X., Pan, Q., Kang, Z., and Liu, J. (2020). TaSTP13 contributes to wheat susceptibility to stripe rust possibly by increasing cytoplasmic hexose concentration. BMC Plant Biol. 20:49. doi: 10.1186/s12870-020-2248-2
Huber, D., Römheld, V., and Weinmann, M. (2012). “Relationship between nutrition, plant diseases and pests,” in Mineral Nutrition of Higher Plants, 3rd Edn, eds P. Marschner (Cambridge, MA: Academic press), 283–298. doi: 10.1016/B978-0-12-384905-2.00010-8
Hugouvieux, V., Barber, C. E., and Daniels, M. J. (1998). Entry of Xanthomonas campestris pv. campestris into hydathodes of Arabidopsis thaliana leaves: a system for studying early infection events in bacterial pathogenesis. Mol. Plant-Microbe Interact. 11, 537–543. doi: 10.1094/MPMI.1998.11.6.537
Janse van Rensburg, H. C., Limami, A. M., and Van den Ende, W. (2021). Spermine and spermidine priming against Botrytis cinerea modulates ROS dynamics and metabolism in Arabidopsis. Biomolecules 11:223. doi: 10.3390/biom11020223
Klüsener, B., Young, J. J., Murata, Y., Allen, G. J., Mori, I. C., Hugouvieux, V., et al. (2002). Convergence of calcium signaling pathways of pathogenic elicitors and abscisic acid in Arabidopsis guard cells. Plant Physiol. 130, 2152–2163. doi: 10.1104/pp.012187
Kohli, S. K., Khanna, K., Bhardwaj, R., Abd Allah, E. F., Ahmad, P., and Corpas, F. J. (2019). Assessment of subcellular ROS and NO metabolism in higher plants: multifunctional signaling molecules. Anti-oxidants 8:641. doi: 10.3390/antiox8120641
Kubo, Y. (2013). Function of peroxisomes in plant-pathogen interactions. Subcell. Biochem. 69, 329–345. doi: 10.1007/978-94-007-6889-5_18
Lal, A., Ku, M. S., and Edwards, G. E. (1996). Analysis of inhibition of photosynthesis due to water stress in the C3 species Hordeum vulgare and Vicia faba: electron transport, CO2 fixation and carboxylation capacity. Photosyn. Res. 49, 57–69. doi: 10.1007/BF00029428
Lewandowska, M., Keyl, A., and Feussner, I. (2020). Wax biosynthesis in response to danger: its regulation upon abiotic and biotic stress. New Phytol. 227, 698–713. doi: 10.1111/nph.16571
Liang, J. S., and Zhang, J. H. (1999). The relations of stomatal closure and reopening to xylem ABA concentration and leaf water potential during soil drying and rewatering. Plant Growth Regul. 29, 77–86. doi: 10.1023/A:1006207900619
Liu, H., and Xue, S. (2021). Interplay between hydrogen sulfide and other signaling molecules in the regulation of guard cell signaling and abiotic/biotic stress response. Plant Commun. 2:100179. doi: 10.1016/j.xplc.2021.100179
Luna, E., Pastor, V., Robert, J., Flors, V., Mauch-Mani, B., and Ton, J. (2011). Callose deposition: a multifaceted plant defense response. Mol. Plant-Microbe Interact. 24, 183–193. doi: 10.1094/MPMI-07-10-0149
Marcec, M. J., Gilroy, S., Poovaiah, B. W., and Tanaka, K. (2019). Mutual interplay of Ca2+ and ROS signaling in plant immune response. Plant Sci. 283, 343–354. doi: 10.1016/j.plantsci.2019.03.004
Melotto, M., Underwood, W., and He, S. Y. (2008). Role of stomata in plant innate immunity and foliar bacterial diseases. Annu. Rev. Phytopathol. 46, 101–122. doi: 10.1146/annurev.phyto.121107.104959
Melotto, M., Zhang, L., Oblessuc, P. R., and He, S. Y. (2017). Stomatal defense a decade later. Plant Physiol. 174, 561–571. doi: 10.1104/pp.16.01853
Nguyen, Q. M., Iswanto, A., Son, G. H., and Kim, S. H. (2021). Recent advances in effector-triggered immunity in plants: new pieces in the puzzle create a different paradigm. Int. J. Mol. Sci. 22:4709. doi: 10.3390/ijms22094709
Park, S. I., Kim, J. J., Shin, S. Y., Kim, Y. S., and Yoon, H. S. (2020). ASR enhances environmental stress tolerance and improves grain yield by modulating stomatal closure in rice. Front. Plant Sci. 10:1752. doi: 10.3389/fpls.2019.01752
Pascual, I., Azcona, I., Morales, F., Aguirreolea, J., and Sánchez-Díaz, M. (2010). Photosynthetic response of pepper plants to wilt induced by Verticillium dahliae and soil water deficit. J. Plant Physiol. 167, 701–708. doi: 10.1016/j.jplph.2009.12.012
Paul, J. M., Watson, A., and Griffiths, C. A. (2020). Linking fundamental science to crop improvement through understanding source and sink traits and their integration for yield enhancement. J. Exp. Bot. 71, 2270–2280. doi: 10.1093/jxb/erz480
Richardson, M. D., and Croughan, S. S. (1989). Potassium influence on susceptibility of bermudagrass to Helminthosporium cynodontis toxin. Crop Sci. 29, 1280–1282. doi: 10.2135/cropsci1989.0011183X002900050038x
Ruiz, J. M., and Romero, L. (2002). Renewed debate over transpiration and long-distance transport of minerals in plants. Trends Plant Sci. 7:56. doi: 10.1016/S1360-1385(02)02237-9
Saito, S., and Uozumi, N. (2019). Guard cell membrane anion transport systems and their regulatory components: an elaborate mechanism controlling stress-induced stomatal closure. Plants 8:9. doi: 10.3390/plants8010009
Sawinski, K., Mersmann, S., Robatzek, S., and Böhmer, M. (2013). Guarding the green: pathways to stomatal immunity. Mol. Plant-Microbe Interact. 26, 626–632. doi: 10.1094/MPMI-12-12-0288-CR
Scharte, J., Schön, H., and Weis, E. (2005). Photosynthesis and carbohydrate metabolism in tobacco leaves during an incompatible interaction with Phytophthora nicotianae. Plant Cell Environ. 28, 1421–1435. doi: 10.1111/j.1365-3040.2005.01380.x
Seifi, H. S., Zarei, A., Hsiang, T., and Shelp, B. J. (2019). Spermine is a potent plant defense activator against gray mold disease on Solanum lycopersicum, Phaseolus vulgaris, and Arabidopsis thaliana. Phytopathology 109, 1367–1377. doi: 10.1094/PHYTO-12-18-0470-R
Serrano, I., Romero-Puertas, M. C., Sandalio, L. M., and Olmedilla, A. (2015). The role of reactive oxygen species and nitric oxide in programmed cell death associated with self-incompatibility. J. Exp. Bot. 66, 2869–2876. doi: 10.1093/jxb/erv083
Shrestha, R. K., Lei, P., Shi, D., Hashimi, M. H., Wang, S., Xie, D., et al. (2021). Response of maize (Zea mays L.) towards vapor pressure deficit. Environ. Exp. Bot. 181:104293. doi: 10.1016/j.envexpbot.2020.104293
Solomon, P. S., Tan, K. C., and Oliver, R. P. (2003). The nutrient supply of pathogenic fungi; a fertile field for study. Mol. Plant Pathol. 4, 203–210. doi: 10.1046/j.1364-3703.2003.00161.x
Sørhagen, K., Laxa, M., Peterhänsel, C., and Reumann, S. (2013). The emerging role of photorespiration and non-photorespiratory peroxisomal metabolism in pathogen defence. Plant Biol. 15, 723–736. doi: 10.1111/j.1438-8677.2012.00723.x
Sun, L. R., Yue, C. M., and Hao, F. S. (2019). Update on roles of nitric oxide in regulating stomatal closure. Plant Signal. Behav. 14:e1649569. doi: 10.1080/15592324.2019.1649569
Sun, Y., Wang, M., Mur, L., Shen, Q., and Guo, S. (2020). Unravelling the roles of nitrogen nutrition in plant disease defences. Int. J. Mol. Sci. 21:572. doi: 10.3390/ijms21020572
Taler, D., Galperin, M., Benjamin, I., Cohen, Y., and Kenigsbuch, D. (2004). Plant eR genes that encode photorespiratory enzymes confer resistance against disease. Plant Cell 16, 172–184. doi: 10.1105/tpc.016352
Tanner, W., and Beevers, H. (2001). Transpiration, a prerequisite for long-distance transport of minerals in plants? Proc. Natl. Acad. Sci. U.S.A. 98, 9443–9447. doi: 10.1073/pnas.161279898
Trapet, P. L., Verbon, E. H., Bosma, R. R., Voordendag, K., Van Pelt, J. A., and Pieterse, C. (2021). Mechanisms underlying iron deficiency-induced resistance against pathogens with different lifestyles. J. Exp. Bot. 72, 2231–2241. doi: 10.1093/jxb/eraa535
van Butselaar, T., and Van den Ackerveken, G. (2020). Salicylic acid steers the growth-immunity tradeoff. Trends Plant Sci. 25, 566–576. doi: 10.1016/j.tplants.2020.02.002
Vo, K., Rahman, M. M., Rahman, M. M., Trinh, K., Kim, S. T., and Jeon, J. S. (2021). Proteomics and metabolomics studies on the biotic stress responses of rice: an update. Rice 14:30. doi: 10.1186/s12284-021-00461-4
Vojtovič, D., Luhová, L., and Petrivalský, M. (2020). Something smells bad to plant pathogens: production of hydrogen sulfide in plants and its role in plant defence responses. J. Adv. Res. 27, 199–209. doi: 10.1016/j.jare.2020.09.005
Voss, I., Sunil, B., Scheibe, R., and Raghavendra, A. S. (2013). Emerging concept for the role of photorespiration as an important part of abiotic stress response. Plant Biol. 15, 713–722. doi: 10.1111/j.1438-8677.2012.00710.x
Walters, D. (2003). Resistance to plant pathogens: possible roles for free polyamines and polyamine catabolism. New Phytol. 159, 109–115. doi: 10.1046/j.1469-8137.2003.00802.x
Wang, W., Paschalidis, K., Feng, J. C., Song, J., and Liu, J. H. (2019). Polyamine catabolism in plants: a universal process with diverse functions. Front. Plant Sci. 10:561. doi: 10.3389/fpls.2019.00561
Wang, X., Cai, X., Xu, C., Wang, Q., and Dai, S. (2016). Drought-responsive mechanisms in plant leaves revealed by proteomics. Int. J. Mol. Sci. 17:1706. doi: 10.3390/ijms17101706
Willmer, C. M., and Fricker, M. (1996). Stomata. London: Chapman and Hall. doi: 10.1007/978-94-011-0579-8
Ye, W., Munemasa, S., Shinya, T., Wu, W., Ma, T., Lu, J., et al. (2020). Stomatal immunity against fungal invasion comprises not only chitin-induced stomatal closure but also chitosan-induced guard cell death. Proc. Natl. Acad. Sci. U.S.A. 117, 20932–20942. doi: 10.1073/pnas.1922319117
Yuan, M., Huang, Y., Ge, W., Jia, Z., Song, S., Zhang, L., et al. (2019). Involvement of jasmonic acid, ethylene and salicylic acid signaling pathways behind the systemic resistance induced by Trichoderma longibrachiatum H9 in cucumber. BMC Genom. 20, 1–13. doi: 10.1186/s12864-019-5513-8
Zaynab, M., Fatima, M., Abbas, S., Sharif, Y., Umair, M., Zafar, M. H., et al. (2018). Role of secondary metabolites in plant defense against pathogens. Microb. Pathog. 124, 198–202. doi: 10.1016/j.micpath.2018.08.034
Keywords: guard cells, innate immunity, peroxisomes, photorespiration, secondary messengers, transpiration
Citation: Gahir S, Bharath P and Raghavendra AS (2021) Stomatal Closure Sets in Motion Long-Term Strategies of Plant Defense Against Microbial Pathogens. Front. Plant Sci. 12:761952. doi: 10.3389/fpls.2021.761952
Received: 20 August 2021; Accepted: 01 September 2021;
Published: 27 September 2021.
Edited by:
Juan Dong, The State University of New Jersey, United StatesReviewed by:
Mohammad Saidur Rhaman, Bangladesh Agricultural University, BangladeshCopyright © 2021 Gahir, Bharath and Raghavendra. This is an open-access article distributed under the terms of the Creative Commons Attribution License (CC BY). The use, distribution or reproduction in other forums is permitted, provided the original author(s) and the copyright owner(s) are credited and that the original publication in this journal is cited, in accordance with accepted academic practice. No use, distribution or reproduction is permitted which does not comply with these terms.
*Correspondence: Agepati S. Raghavendra, YXNfcmFnaGF2ZW5kcmEmI3gwMDA0MDt5YWhvby5jb20=; YXNyc2wmI3gwMDA0MDt1b2h5ZC5lcm5ldC5pbg==
†These authors have contributed equally to this work