- State Key Laboratory of Cotton Biology, State Key Laboratory of Crop Stress Adaptation and Improvement, College of Life Sciences, Henan University, Kaifeng, China
The adjustment of stomatal density and clustered ratio on the epidermis is the important strategy for plants to respond to drought, because the stoma-based water loss is directly related to plant growth and survival under drought conditions. But the relevant adjustment mechanism still needs to be explored. 1-Aminocyclopropane-1-carboxylate (ACC) is disclosed to promote stomatal development, while in vivo ACC levels depend on activation of ACC synthase (ACS) family members. Based on the findings of ACS expression involving in drought response and several ACS activity inhibitors reducing stomatal density and cluster in drought response, here we examined how ACS activation is involved in the establishment of stomatal density and cluster on the epidermis under drought conditions. Preliminary data indicated that activation of ACS2 and/or ACS6 (ACS2/6) increased stomatal density and clustered ratio on the Arabidopsis leaf epidermis by accumulating ACC under moderate drought, and raised the survival risk of seedlings under escalated drought. Further exploration indicated that, in Arabidopsis seedlings stressed by drought, the transcription factor SPEECHLESS (SPCH), the initiator of stomatal development, activates ACS2/6 expression and ACC production; and that ACC accumulation induces Ca2+ deficiency in stomatal lineage; this deficiency inactivates a subtilisin-like protease STOMATAL DENSITY AND DISTRIBUTION 1 (SDD1) by stabilizing the inhibition of the transcription factor GT-2 Like 1 (GTL1) on SDD1 expression, resulting in an increases of stomatal density and cluster ratio on the leaf epidermis. This work provides a novel evidence that ACS2/6 activation plays a key role in the establishment of stomatal density and cluster on the leaf epidermis of Arabidopsis in response to drought.
Introduction
Stomata are pore structures surrounded by guard cells (GCs) on the leaf epidermis that regulate the exchange of gases (i.e., H2O, CO2, or O2) between plants and the environment (Acharya and Assmann, 2009; Zoulias et al., 2018). In evolution from aquatic to terrestrial, plants had generated stomata on epidermis of aerial part to facilitate transpiration, and to guarantee plant survival and life on land (Croxdale, 2000; van Veen and Sasidharan, 2021). For terrestrial plant, stomata can sense environmental water status, especially water deficit or drought, and regulate water loss from plant (Acharya and Assmann, 2009; Zoulias et al., 2018). The ability of stomata to regulate water loss is generally estimated from stomatal density (number of stomata per unit leaf area), stomatal index (ratio of stomatal number to sum of epidermal cells and stomata), and pattern (whether stomata are distributed singly or in clusters). Evidences show that plants accurately set the number of epidermal cells between the starting position for development of new stoma and the preexisting stomata, that is, set the stomatal space under certain conditions (Croxdale, 2000; Hamanishi et al., 2012; Zoulias et al., 2018). That is to say, stomatal density and clustered ratio are the results of stomatal space setting; for example, stomatal cluster is formed by directly contacting stomata with no intervening epidermal cell, or, alternatively, zero-space establishment (Von Groll et al., 2002; Acharya and Assmann, 2009; Zoulias et al., 2018). The establishment of stomatal space is, therefore, an important aspect of plant growth and survival under drought conditions (Hepworth et al., 2015). However, the setting mechanism of stomatal space needs to be studied.
The regulation of stomatal development, which undergirds stomatal space setting, has been extensively investigated. Studies on the model plant Arabidopsis thaliana (L.) have shown that stomatal development includes a series of epidermal cell divisions, in which several basic helix-loop-helix transcription factors, such as SPCH, MUTE, and FAMA, are involved in this process. SPCH initiates stomatal development to transform meristemoid mother cells (MMCs) into a meristemoids and a sister cell; MUTE converts a sister cell into the guard mother cells (GMCs); then FAMA drives GMCs to form a stoma with differentiated GCs (Hamanishi et al., 2012; Zoulias et al., 2018). These findings show clearly that SPCH dominates stomatal development. This view is better interpreted, for example, the loss-of-function spch-1 or spch-3 homozygous mutant do not produce any stomata (MacAlister et al., 2007; Pillitteri et al., 2007; Han and Torii, 2016). Furthermore, SPCH expression is known to implicate the establishment of stomatal density (Tripathi et al., 2016; Zoulias et al., 2018). However, it is still unclear whether and how SPCH-dependent stomatal development affects stomatal space setting.
Evidences suggest that the subtilisin-like protease STOMATAL DENSITY AND DISTRIBUTION 1 (SDD1) participates in the establishment of stomatal density and clustered ratio on leaf epidermis (Berger and Altmann, 2000; Casson and Gray, 2008; Serna, 2009; Zoulias et al., 2018), as evidenced by the following: a loss-of-function sdd1-1 mutant showed a two- to four-fold increase in stomatal density and clusters in all aerial parts, whereas transgenic SDD1-overexpressing plants exhibited a two- to three-fold decrease in stomatal density and arrested stomata (Berger and Altmann, 2000; Von Groll et al., 2002). In line with these findings, SDD1-overexpressing plants displayed diminished transpiration because of a ∼25% reduction in abaxial stomatal density or clusters (Yoo et al., 2010). Evidently, SDD1 activity specifically increases stomatal density and stomatal clustering ratio, or reduces stomatal space. But the regulatory mechanism of SDD1 activity has been uncovered. Significantly, the trihelix transcription factor GT-2 LIKE 1 (GTL1) binds to the promoter of the SDD1 gene and inhibits its expression (Yoo et al., 2010; Weng et al., 2012; Virdi et al., 2015). This inhibition can be relieved by Ca2+ increase because Ca2+-loaded calmodulin (Ca2+-CaM) destabilizes the docking of GTL1 protein to the SDD1 promoter (Yoo et al., 2019). Clearly, elevated Ca2+ levels increase SDD1 activity but decrease stomatal density and clustered ratio. Nevertheless, it remains unclear how SPCH-dependent stomatal individual development is integrated with SDD1-controlled stomatal space setting.
The non-proteinogenic amino acid 1-aminocyclopropane-1-carboxylate (ACC) has recently been shown to independently promote stomatal generation by facilitating the differentiation of GMCs into GCs in Arabidopsis leaves (Yin et al., 2019). Unexpectedly, ethylene is not involved in this process (Yin et al., 2019), even though ACC is the precursor of ethylene (Bleecker and Kende, 2000). In fact, ACC is known to be involved in stomatal development and space setting (Acharya and Assmann, 2009). For example, ACC treatments increased the number of stomata by ∼33% on the hypocotyl (Saibo et al., 2003) or cotyledon epidermis (Serna and Fenoll, 1996) in Arabidopsis, and also induced stomatal clusters (Serna and Fenoll, 1996; Berger and Altmann, 2000). The production of ACC in vivo depends on the activity of ACC synthase (ACS), which converts S-adenosylmethionine to ACC (Bleecker and Kende, 2000). Various pieces of experimental evidence strongly suggest that ACS activity is an important mediator of stomata development. For example, the inhibitors of ACS activity, such as aminoethoxyvinylglycine (AVG), were shown to significantly reduce the frequency of stomatal appearance (Serna and Fenoll, 1996; Saibo et al., 2003; Yin et al., 2019), which is the prerequisite for stomatal space setting. It is known that ACS is encoded by a multi-gene family (Bleecker and Kende, 2000), and that the activity of ACS family members is unique, overlapping, and spatiotemporally specific (Tsuchisaka and Theologis, 2004; Tsuchisaka et al., 2009). The Arabidopsis genome contains nine ACS genes (ACS1, ACS2, ACS4–9, and ACS11) that encode authentic enzymes (Tsuchisaka et al., 2009). Few evidences imply that ACS activity may be involved in the stomata-based drought response, for example, the expression of ACS genes is induced by drought in Arabidopsis (Dubois et al., 2017, 2018), and chromatin immunoprecipitation assays indicate that SPCH may regulate the transcription activity of ACS2 and ACS6 genes (Lau et al., 2014). Nevertheless, further evidence needs to be provided that how SPCH directs ACS2/6 activity during stomatal developing and spacing.
In this study, we explored the specific involvement of ACS2/6 activity in the drought tolerance of Arabidopsis seedlings. Our results revealed that the T-DNA insertion mutants acs2-1, acs6-1, and acs2-1acs6-1 are more tolerant to drought than is the wild-type (WT) control. Subsequent research on the underlying mechanism indicated that SPCH activates the expression of ACS2 and/or ACS6 by directly binding to their promoters. ACS2/6-dependent ACC accumulation triggers a Ca2+ shortage in stomatal lineage cells, and thus stabilized the inhibition of the transcription factor GT-2 Like 1 (GTL1) on SDD1 expression. Stomatal density and cluster on the leaf epidermis are thereby increased, leading to increased seedling wilting and even death under intensified drought.
Materials and Methods
Plant Materials and Growth Conditions
Arabidopsis thaliana (Columbia-0 ecotype) was used as WT. The different ACS2 expression lines, including mutant acs2-1 (CS16564) with a T-DNA insertion, ACS2-complementation (ACS2/acs2-1), ACS2-overexpression (ACS2-OE), and pACS2::ACS2-GUS lines, have been described previously (Han et al., 2019). The T-DNA insertion mutant acs6-1 (CS16569) was obtained from the Arabidopsis Biological Resource Center (United States). The double mutant acs2-1acs6-1 was created by crossing acs2-1 with acs6-1. Seeds of spch-3 mutant with a T-DNA insertion were a friendly gift from Professor Sui-wen Hou (MOE Key Laboratory of Cell Activities and Stress Adaptations, Lanzhou, China) which were described in MacAlister et al. (2007). These homozygotes with T-DNA insertion were screened according to the method provided by the Salk Institute1. Seeds of the point mutant spch-1 was a friendly gift from Professor Xiao-lan Chen (School of Life Sciences, Yunnan University, China) which were created by MacAlister et al. (2007), and was identified by PCR amplification and sequencing of the fragment containing the mutation site. spch-1acs2-1acs6-1 were generated by genetic crossing with reference to Han et al. (2018). All primers used in this study are listed in the Supplementary Table 1.
Seeds of the transgenic pSPCH::SPCH-GFP line (created by Fred Sack, University of British Columbia) were a friendly gift from Professor Xiao-lan Chen (School of Life Sciences, Yunnan University, China), and GFP expression was detected by hygromycin screening and measurement of fluorescence in leaves. The seeds of Ca2+ sensor NES-YC3.6-expressing line were kindly gifted by Professor Jörg Kudla (Molecular Genetics and Cell Biology of Plants, University of Munich, Germany). NES-YC3.6-expressing acs2-1acs6-1 line was created by crossing acs2-1acs6-1 with NES-YC3.6-expressing WT plants. Progeny were selected on kanamycin-containing medium and by measuring fluorescence in leaves. All F3 progeny meeting the requirements were used in subsequent experiments.
All seeds were collected and stored under the same conditions. Prior to experiments, seeds were surface-sterilized and sown on Murashige-Skoog medium. After 3 days at 4°C in darkness, plates were transferred to a greenhouse (21 ± 2°C, 70% humidity, 100 μmol m–2 s–1 light intensity, and a 16-h light/8-h dark photoperiod). After germination and growth for 7 days, young seedlings were transplanted into water-saturated soil. Watering was halted according to the requirements of each specific drought treatment described in this paper.
Creation of Transgenic Plants
To generate ACS6- and SPCH-overexpression lines, the full-length coding sequence (CDS) of ACS6 or SPCH was amplified and cloned into the pSUPER 1300 vector. Each construct was then introduced into Agrobacterium strain GV3101 and transformed into the target plants by floral infiltration. The same method was used to generate the transformants described below. To generate ACS6-complementation (ACS6/acs6-1) lines, the promoter and CDS of ACS6 were cloned into a pCAMBIA1300 vector, which was transformed into acs6-1 plants. To generate pACS6::ACS6-GUS lines, the ACS6 promoter fragment and full-length CDS were cloned into the promoter-less β-glucuronidase (GUS) expression vector pCAMBIA1391, which was then transformed into WT. To generate pSDD1::SDD1-GFP lines, the promoter fragment and CDS of SDD1 were cloned into a pCAMBIA1300 vector, which was then introduced into acs2-1acs6-1 and WT. The T1 transgenic plants were selected on hygromycin-containing medium, and the T3 progeny were used for subsequent experiments.
Water Loss Assay
True leaves were collected from 28-day-old plants following previously described methods (Xie et al., 2019). The fresh weight of leaves was determined immediately. Leaves of five plants per line were weighed hourly on an electronic balance (Sartorius, Germany) at room temperature (23°C). Water loss was calculated using the following formula: [(W1–W2)/W1] × 100%, where W1 is the initial leaf fresh weight, and W2 is the leaf weight at a given time point.
Evaluation of Stomatal Density, Stomatal Index, and Rate of Stomatal Clustering
Stomatal density, stomatal index and clustering ratio were determined according to previously described methods (de Marcos et al., 2017; Qi et al., 2019). The sixth fully expanded rosette leaves (count up from cotyledons) were used for analyzing the stomatal phenotype of 28-day-old seedlings. Strips were peeled from leaf abaxial epidermis, fixed on a slide, and photographed under a differential contrast interference microscope (LSM710, Zeiss, Germany). Images were acquired under the 20× objective (0.18 mm2). Randomly selected images are shown in figures.
For analyses of stomatal density, index and clustering rate, 25 plants per line per plant were examined. In all counts, a stoma was considered to have a pair of complete guard cells. The calculation formula involved is as follows: Stomatal density = stomatal number/area (mm2); Stomatal index = (number of stomata)/(number of epidermal cells + number of stomata) × 100%; The rate of clustered stomata = number of clusters/(number of stomata + number of clusters) × 100%.
RNA Extraction and Quantitative Real-Time Polymerase Chain Reaction Analyses
Total RNA was extracted using a plant RNA MIDI kit (Life-Feng, Shanghai, China). First-strand complementary DNA (cDNA) was synthesized with a Reverse Transcription system (Toyobo, Osaka, Japan) and was used as the template for quantitative real-time polymerase chain reaction (RT-qPCR) analyses along with 2 × SYBR Green I master mix (Vazyme, Nanjing, China). The RT-qPCR analyses were performed on a Roche 480 real-time PCR system (Roche, Mannheim, Germany). The RNA levels were calculated as described by Livak and Schmittgen (2001). The reference gene was ACTIN8 (AT1G49240).
β-Glucuronidase Staining
Leaves excised from 21-day-old plants were incubated overnight in darkness at 37°C in GUS staining solution (0.1 M sodium phosphate buffer, pH 7.0; 0.05 mM K3[Fe(CN)6]; 0.05 mM K4[Fe(CN)6]; 1 mg ml–1 X-Gluc (Sigma, United States); and 0.1% Triton X-100). After staining, leaves were de-stained with 75% (v/v) ethanol until the chlorophyll was completely removed, and then were photographed using a digital camera (Canon 760D). Representative photographs are shown in figures.
Measurement of 1-Aminocyclopropane-1-Carboxylate Content
Leaves from the same line of 21-day-old plants were collected and ground into a powder. A 0.1-mg aliquot of powdered sample was transferred into an Eppendorf tube along with 1 ml ultrapure water. To completely extract ACC from leaf tissue, the sample was further fragmented using an ultrasonic crusher (Branson, Danbury, CT, United States). The supernatant was collected, the pH was adjusted to <4, and impurities were removed using 1 ml chloroform. The supernatant was then passed through a column containing C18 adsorbent (Oasis MCX, 30 μm, 3 cc/60 mg, Waters, Milford, MA, United States). The column was eluted with 1 M ammonia in water, with chromatographic methanol as the solvent. The eluent was evaporated to dryness in a Concentrator Plus evaporator (Eppendorf, Hamburg, Germany) under vacuum at 30°C and then re-suspended in solution (chromatographic methanol: 0.1% (v/v) acetic acid, 1:9). Samples were analyzed using an Applied Biosystems MDS SCIEX 4000 QTRAP liquid chromatography-tandem mass spectrometry system (AB Sciex, Foster City, CA, United States). Standard ACC (Sigma-Aldrich, Steinheim, Germany) was used for the quantitative analysis.
Protein Extraction and Western Blotting
Leaves of 21-day-old pSDD1::SDD1-GFP transgenic plants were collected according to the experimental requirements and ground into a powder. Powdered samples were transferred to RIPA lysis buffer (Boster Biotechnology, Wuhan, China) and micro-centrifuged at 16,000 × g for 15 min at 4°C. The concentration of crude protein in the supernatant was determined using a NanoDrop 2000 (Thermo Scientific Wilmington, DE, United States). The crude protein was separated by 12% SDS-PAGE and then transferred to a nitrocellulose filter membrane (Millipore, Billerica, MA, United States) using a Trans-Blot Semi-Dry transfer cell (Bio-Rad, Hercules, CA, United States). The membrane was then incubated at room temperature for 1–2 h in blocking solution before incubation with anti-GFP mouse monoclonal antibodies (1:10,000; Proteintech, Chicago, IL, United States) for 2 h at room temperature. The membrane was subjected to three 10-min washes with TBST and then incubated overnight at 4°C with horseradish peroxidase-conjugated secondary antibody (Proteintech). Protein bands were detected using a BeyoECL Plus kit (Beyotime, Shanghai, China) and then visualized using a Fusion FX7 Spectra system (Vilber Lourmat, Marne-la-Vallée, France). An anti-GAPDH antibody (1:5000; Proteintech) was used as the loading control.
Chromatin Immunoprecipitation Analyses
Immature leaves collected from pSPCH::SPCH-GFP of 21-day-old plants were cross-linked using 1% formaldehyde under vacuum for 10 min according to the EZ-ChIP chromatin IP kit protocol (Thermo Scientific). After washing with phosphate-buffered saline solution, leaves were ground in liquid nitrogen and then suspended in SDS lysis buffer containing protease inhibitor cocktail. The DNA of SPCH was sheared into small fragments (300–500 bp). The sheared chromatin was chromatin immunoprecipitation with GFP antibodies (Proteintech) overnight at 4°C. The ChIP DNA products were analyzed by RT-qPCR using three pairs of primers synthesized to amplify approximately 200-bp DNA fragments of the promoter region of ACS2 or ACS6, which were used in the ChIP analysis. Primers annealing to promoter regions of two Arabidopsis genes lacking an SPCH binding site were used as negative controls. An unrelated DNA sequence from the ACTIN8 gene was used as an internal control.
Transient Transcription Dual-Luciferase Assays
Detection was performed according to previously described methods (Bao et al., 2014). The 2400-bp promoter sequence of ACS2 was divided into three fragments (−1 to −1000, −900 to −1600, and −1500 to −2400 bp). The 2600-bp promoter sequence of ACS6 was also divided into three fragments (−1 to −1000, −900 to −2000, and −1900 to −2600 bp). Each fragment was cloned into pGreen II 0800-Luc to construct the corresponding reporter plasmid. The coding sequence of Arabidopsis SPCH was cloned into pGreenII 62-SK to construct the 35S-SPCH effector plasmid. The Agrobacterium strain GV3101 (pSoup-p19) was incubated in yeast mannitol medium and finally re-suspended in buffer to a final concentration of OD600 = 1.0. Equal amounts of different combined bacterial suspensions were infiltrated into young leaves of tobacco plants using a needleless syringe. After 3 days, the infected leaves were sprayed with D-luciferin (sodium salt) (Yeasen, Shanghai, China) and placed in darkness for 5 min. Firefly luciferase (LUC) signals were then detected using the NightSHADE system (LB 985, Berthold Technologies, Bad Wildbad, Germany). The ratio of LUC activity to Renilla luciferase (REN) activity was measured using a Dual-Luciferase Reporter Gene Assay kit (Solarbio, Beijing, China). Briefly, the tobacco leaves were ground in liquid nitrogen, and the extract was incubated in a low-temperature buffer. The LUC/REN ratio was measured using an enzyme standard instrument (Tecan, Männedorf, Switzerland).
Monitoring of Ca2+ Levels in Stomatal Lineage Cells
The Ca2+ levels in stomatal lineage cells were monitored according to Krebs et al. (2012). Immature leaves of Arabidopsis seedlings expressing the fluorescence resonance energy transfer (FRET)-based Ca2+ sensor NES-YC3.6 (Nagai et al., 2004; Krebs et al., 2012) were collected from the same position. During confocal laser scanning, strips were peeled from leaf abaxial epidermis and then fixed on a slide on the loading platform. The relative fluorescence intensity of YC3.6 protein was recorded under a Nikon A1 Plus laser scanning confocal microscope (Nikon, Tokyo, Japan) with the following scanning parameters: image dimension = 1024 × 1024, pinhole radius = 38.31 μm, scanning speed = 0.25, zoom = 3×, objective = 60× (water), numerical aperture = 1.27), plan apochromat objective, power = 6% (445 nm solid laser). Images were acquired every 5s. Emissions from cyan fluorescent protein (CFP; 465–499 nm) and FRET-dependent cpVenus (525–555 nm) in stomatal lineage were detected simultaneously. The cpVenus/CFP emission ratio was analyzed using NIS-Elements AR software.
Statistical Analysis
All experiments were independently repeated using three biological replicates and three technical replicates at least. Statistical analysis comparing two means were performed using the two-way ANOVA or Student’s t-test [P-values < 0.05 (*) and <0.01 (**) were considered to correspond to significant and extremely significant differences, respectively].
Results
ACS2/6 Activation and 1-Aminocyclopropane-1-Carboxylate Accumulation Facilitated Water Evaporation From Leaves in Response to Drought Treatment
Studies have shown that the activity of ACS2 in rice (Zhang et al., 2013) and ACS6 in maize (Young et al., 2004) regulates seedling sensitivity to drought, and that drought induces ACS2/6 activation and ACC accumulation in Arabidopsis (Catalá et al., 2014; Dubois et al., 2018). Therefore, we examined the possible roles of ACS2 and ACS6 in the drought response of Arabidopsis seedlings.
The expressions of ACS2 and ACS6 were modified in several genetic materials. For example, compared with WT, the loss-of-function mutant lines acs2-1, acs6-1, and acs2-1acs6-1 showed significantly reduced ACS2 or ACS6 mRNA levels; the transgenic ACS2-OE(#1), ACS2-OE(#3), ACS6-OE(#1), and ACS6-OE(#3) over-expression lines had significantly elevated ACS2 and ACS6 mRNA levels, respectively; the transgenic complemented lines ACS2/acs2-1(#1), ACS2/acs2-1(#3), ACS6/acs6-1(#1), and ACS6/acs6-1(#3) exhibited no changes in ACS2 or ACS6 expression, respectively (Figure 1A). Interestingly, the expression levels of ACS6 and ACS2 were relatively unchanged in the single mutants acs2-1 and acs6-1, respectively (Figure 1B). We first checked the growth phenotypes of the various ACS2/6 expression lines in response to drought caused by stopping watering for 12 days and the soil water content dropped to ∼39% (drought index refers to Yang et al., 2016). Compared with the control (normal watering), drought caused by stopping watering resulted in WT, ACS2/acs2-1(#1), ACS6/acs6-1(#1), ACS2-OE(#1), ACS2-OE(#3), ACS6-OE(#1), and ACS6-OE(#3) seedlings withered and even died as the drought intensifying, while the mutants acs2-1, acs6-1, and acs2-1acs6-1 seedlings alleviated these wilting or drying symptoms (Supplementary Figure 1). Apparently ACS2/6 activation promoted dehydration and wilting of seedlings in response to drought treatment.
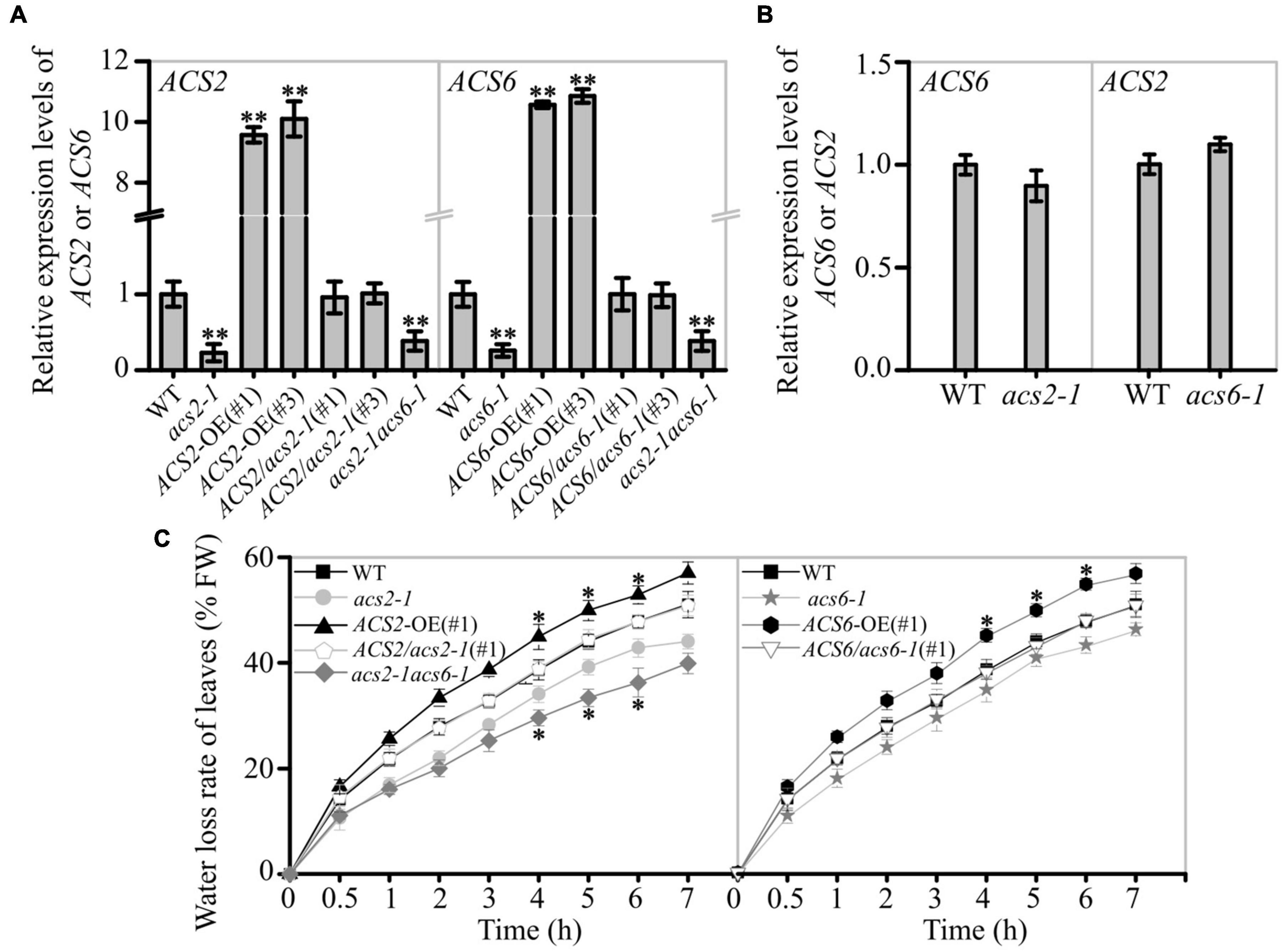
Figure 1. Effect of ACS2/6 expression on water loss from leaves of Arabidopsis seedlings. (A) RT-qPCR analysis of ACS2 and ACS6 mRNA levels in various lines, including the WT; loss-of-function mutants acs2-1, acs6-1, and acs2-1acs6-1; overexpression lines ACS2-OE(#1), ACS2-OE(#3), ACS6-OE(#1), and ACS6-OE(#3); and complementation lines ACS2/acs2-1(#1), ACS2/acs2-1(#3), ACS6/acs6-1(#1), and ACS6/acs6-1(#3). ACTIN8 was used as a reference gene. Experiments were repeated three times. Values are means ± SD (Student’s t-test; **P < 0.01). (B) RT-qPCR analysis of ACS6 mRNA level in acs2-1 and ACS2 mRNA level in acs6-1. Experiments were repeated three times with similar results. (C) Relative rate of water loss over time from detached rosette leaves of 28-day-old plants. All true leaves of five plants of the same line grown under identical conditions were collectively weighed every hour. The data represent the water loss percentage at a given time point, calculated as follows: [(initial weight – weight at each time point)/initial weight] × 100. Experiments were repeated at least three times with similar results. Values are means ± SD (Student’s t-test; *P < 0.05).
The rate of water evaporation from detached leaves of these lines was monitored. The water loss rate was decreased in acs2-1, acs6-1, and acs2-1acs6-1 leaves, compared with that in WT (Figure 1C). Conversely, the water evaporation rate was significantly increased in ACS2-OE(#1) or ACS6-OE(#1) compared with that of WT, whereas no significant change was observed in ACS2/acs2-1(#1) or ACS6/acs6-1(#1) (Figure 1C). The other transgenic lines, such as ACS2-OE(#3), and ACS6-OE(#3), ACS2/acs2-1(#3), and ACS6/acs6-1(#3), also have the same phenotype (Supplementary Figure 2). Data suggest that ACS2/6 activation was positively correlated with the rate of water evaporation from detached leaves.
The characteristics of ACS2/6 expression in leaves were examined in response to drought treatment. Histochemical staining revealed that the higher GUS-marked ACS2/6 expression was in immature leaves, followed by senescent leaves, and then mature leaves in WT plants; After withholding water for 6 days, ACS2 and ACS6 expressions in WT were significantly increased in immature leaves, slightly increased in mature leaves, and unchanged in senescent leaves (Figure 2A). Meantime, quantitative real-time PCR showed the same results (Figure 2B). This is, ACS2 and ACS6 expressions were always higher in senescent leaves regardless of drought, whereas both expressions increased in response to drought in non-senescent leaves.
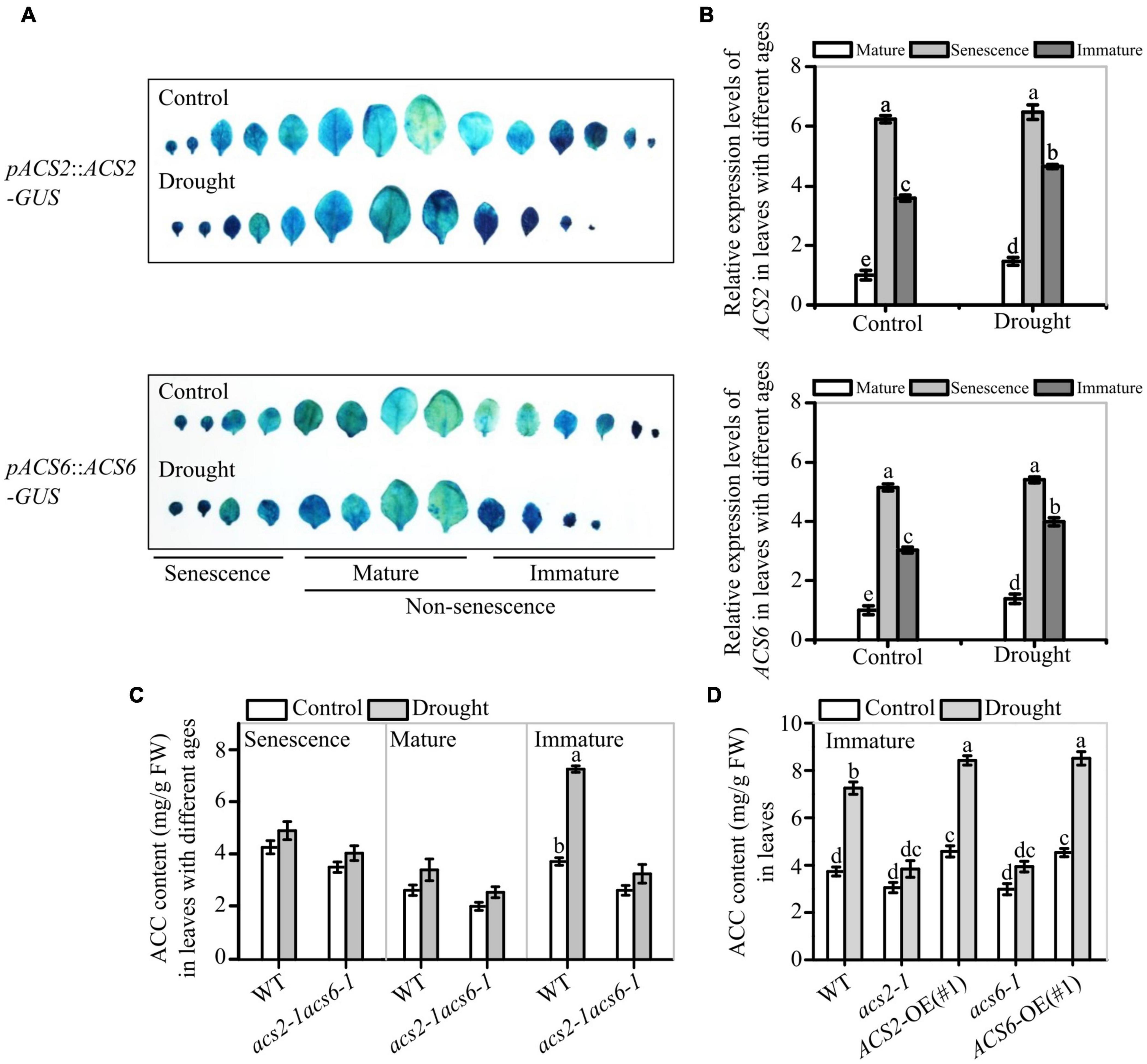
Figure 2. Effects of drought on ACS2 and ACS6 expressions and ACC accumulation in leaves of different ages. (A) ACS2 or ACS6 expression was monitored in immature, mature and senescence leaves of recombinant pACS2::ACS2-GUS or pACS6::ACS6-GUS lines, respectively, with or without stopping watering (drought) treatment. Representative images are shown. Experiments were repeated three times with similar results. (B) ACS2 or ACS6 expression was monitored by RT-qPCR in immature, mature and senescence leaves of WT, respectively, with or without drought treatment. Experiments were repeated three times with similar results. Values are means ± SD. Letters indicate significant differences (P < 0.05, two-way ANOVA). (C,D) HPLC analysis of ACC accumulation in leaves with different ages of 21-day-old seedlings with or without drought treatment. Experiments were repeated three times with similar results. Values are means ± SD. Letters indicate significant differences (P < 0.05, two-way ANOVA).
Next, the effects of ACS2/6 expression activity on ACC accumulation was analyzed in leaves. Under normal conditions, ACC mainly accumulated in immature and senescent leaves of WT seedlings, whereas ACC accumulated primarily in immature leaves in response to a 6-day halt in watering. More specifically, ACC levels were, respectively, 1.94- and 1.33-times higher in immature and mature leaves of WT after withholding water (Figure 2C). In contrast to WT, the double mutant acs2-1acs6-1 did not significantly accumulate ACC in the immature leaves in response to drought (Figure 2C). In the meantime, ACC accumulation in immature leaves was more in ACS2-OE(#1) and ACS6-OE(#1) than that in WT and mutants (acs2-1 or acs6-1) under drought stress (Figure 2D). These data suggest that drought-induced ACS2/6 expression and ACC accumulation was involved in the regulation of stomatal development and pattern in non-senescent leaves.
ACS2/6 Activation Affected Stomatal Space on the Epidermis of Leaves Subjected to Drought Treatment
Evidences suggest that the ACS activity was required for stomatal development (Serna and Fenoll, 1996; Saibo et al., 2003; Young et al., 2004; Zhang et al., 2013; Lau et al., 2014; Yin et al., 2019), we thus analyzed the effects of ACS2/6 expression activity on stomatal density and stomatal clustering ratio on the leaf abaxial epidermis of Arabidopsis seedlings.
Images of stomata and stomatal clusters on the abaxial epidermis of the sixth (count up from cotyledon) mature leaves of the various ACS2/6 expression lines under the control or drought conditions are shown in Figure 3A. Under normal watering conditions, there was no significant difference in the number of stomatal density, stomatal index and clustering rate between the mutants acs2-1, acs6-1, and acs2-1acs6-1, and over-expression lines ACS2-OE(#1), ACS6-OE(#1), and WT (Figure 3B), and also in other transgenic lines ACS2-OE(#3) and ACS6-OE(#3), ACS2/acs2-1(#3), and ACS6/acs6-1(#3) (Supplementary Figure 3A). After halting watering for 6 days, however, stomatal density was significantly reduced in acs2-1 (177.8 ± 8.2 mm–2), acs6-1 (183.8 ± 6.2 mm–2), and acs2-1acs6-1 (161.3 ± 8.2 mm–2), but significantly increased in ACS2-OE(#1) (255.6 ± 6.9 mm–2) and ACS6-OE(#1) (261.1 ± 4.9 mm–2), compared with that in WT (205.6 ± 5.4 mm–2) (Figure 3B). Meantime, stomatal index on the leaf epidermis of WT was 25.3%, by comparison, the mutants acs2-1 (19.9%), acs6-1 (20.8%), and acs2-1acs6-1 (20.2%) evidently reduced but ACS2-OE(#1) (28.5%) and ACS6-OE(#1) (28.5%) increased stomatal index (Supplementary Figure 3B). This is, the expression of ACS2/6 was a positive relationship with both stomatal density based on leaf area and stomatal index based on sum of epidermal cells and stomata. It is interesting to note that the percentage of the pairs of directly contacting stomata was significantly higher in ACS2-OE(#1) (2.1%) and ACS6-OE(#1) (1.87%), but was significantly lower in the mutants acs2-1 (0.14%), acs6-1 (0.14%) and acs2-1acs6-1 (0.11%) than in WT (0.28%) (Figure 3C). In addition, the reduction of stomatal density did not occur in other mutants (acs1-1, acs4-1, acs5-1, acs7-1, acs8-1, acs9-1, and acs11-1) (Supplementary Figure 4). These data indicate that ACS2/6 activation reduced stomatal space, presented as an increase of stomatal density and the rate of clustered stomata on the leaf abaxial epidermis under drought treatment.
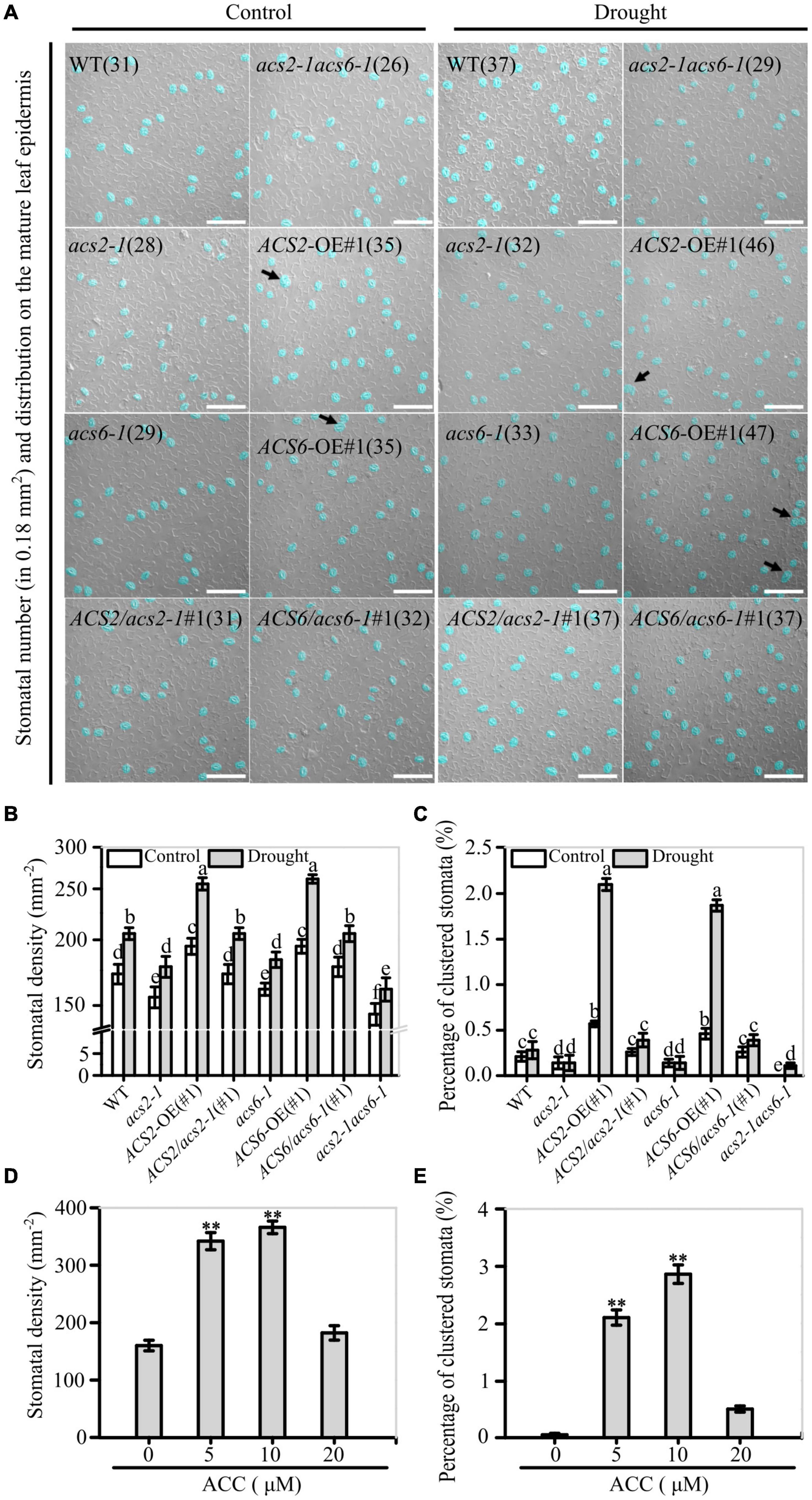
Figure 3. Correlation between ACS2/6 activation and stomatal density and rate of stomatal clustering. (A) Images of stomata distributed on strips of leaf abaxial epidermis under differential interference contrast (DIC) microscopy. Stomata and stomatal clusters are colored in blue for easier identification, and stomatal clusters are indicated by black arrows. The number of stomata is given in parentheses in each image. Sixth rosette were collected under drought or normal watering conditions from 28-day-old seedlings of the WT control; mutants acs2-1, acs6-1, and acs2-1acs6-1, overexpression lines ACS2-OE(#1) and ACS6-OE(#1), and complementation lines ACS2/acs2-1(#1) and ACS6/acs6-1(#1). Experiments were performed three times with similar results. The white scale bar represents 100 μm. (B,C) Statistical analysis of stomatal density (B) and percentage of clustered stomata (C) under normal and drought condition. Number of stomata and stomatal clusters on sixth leaves of 25 seedlings were counted. Values are means ± SD (n = 25 repeats). Letters indicate significant differences (P < 0.05, two-way ANOVA). (D,E) After treated immature leaves by ACC (0–20 μM) for 6 days, stomatal density (D) and percentage of clustered stomata (E) were analyzed, respectively. A total of 25 leaves from 25 seedlings were used to analyze the numbers of stomata and percentage of clustered stomata. Values are means ± SD. (Student’s t-test, **P < 0.01).
In addition, the validation experiments indicate that appropriate concentrations (5–10 μM) of ACC increased stomatal density and ratio of clustered stomata on the leaf epidermis of WT seedlings (Figures 3D,E). In contrast, ACS activity inhibitor AVG treatment significantly decreased stomatal density regardless of drought (Supplementary Figure 5). These observations suggest that ACS2/6 activation and ACC accumulation increased stomatal density and ratio of clustered stomata on the abaxial epidermis of leave, and these characteristic changes are consistent with the wilting or drying phenotype under drought conditions.
SPEECHLESS Promoted the Expression of ACS2 and ACS6 Genes by Docking to Each of Their Promoter Region
The above data suggest that the promotion of ACS2/6 activation and ACC accumulation on stomatal density and clustered ratio on the leaf epidermis is similar to that of SPCH (Tripathi et al., 2016; Zoulias et al., 2018), we speculated that SPCH may mediate ACS2/6 expression activity. Although a profile list generated by genome-wide ChIP-based sequencing of the targets of SPCH included both ACS2 and ACS6 (Lau et al., 2014), direct experimental evidence was still lacking.
To explore whether SPCH affect ACS2 and ACS6 expression, we checked mRNA levels of ACS2 and ACS6 in the spch-1 and spch-3 mutant seedlings, respectively. In order to explore the stomatal development on the epidermis of true leaves, the heterozygote of spch-1 and spch-3 were used, because the two homozygotes cannot grow true leaf (MacAlister et al., 2007; Pillitteri et al., 2007; Han and Torii, 2016). Observations indicated that both spch-1 and spch-3 had significantly reduced ACS2 and ACS6 mRNA levels, respectively, whereas SPCH-OE lines had significantly increased mRNA levels, compared with the WT control (Figure 4A). Interestingly, drought similarly induced the expressions of SPCH, ACS2, and ACS6 genes (Figure 4B). Data implies that SPCH activity was positively correlated with the expression of ACS2 and ACS6.
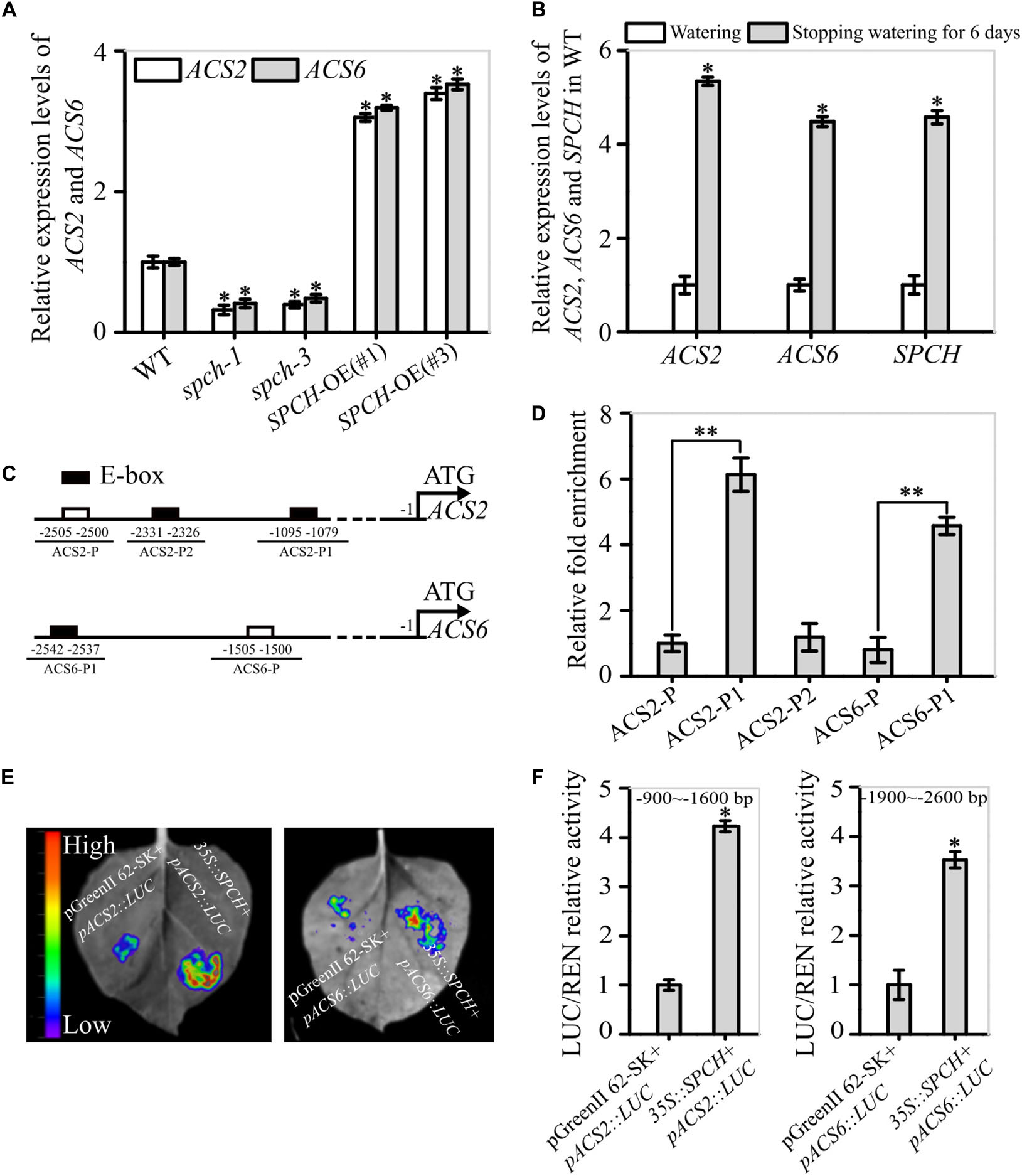
Figure 4. Evidence for the function of SPCH as a transcription factor of ACS2 and ACS6 genes. (A) Levels of ACS2 and ACS6 mRNA in immature leaves of WT control, loss-of-function spch-1 and spch-3 mutant, and SPCH-overexpressing plants of SPCH-OE(#1) and SPCH-OE(#3) based on RT-qPCR. Experiments were repeated three times with similar results. Values are means ± SD (Student’s t-test; *P < 0.05). (B) Relative levels of ACS2, ACS6, and SPCH mRNA transcripts in leaves of 21-day-old WT seedlings under normal watering conditions or after 6 days without watering. Experiments were repeated three times with consistent results. Values are means ± SD (Student’s t-test; *P < 0.05). (C) Diagram of the relative position of E-boxes (CACGTG or CGCGTG) and a reference DNA region in ACS2 and ACS6 gene promoters. Black rectangles indicate E-boxes in ACS2 (ACS2-P1 and ACS2-P2) and ACS6 (ACS6-P1) promoter regions, while white rectangles represent reference regions, namely randomly selected DNA fragments from ACS2 (ACS2-P) and ACS6 (ACS6-P) promoter regions. (D) Relative abundance of SPCH-immunoprecipitated DNA fragments under drought stress as determined by RT-qPCR. All experiments, which included three biological replicates, gave similar results. Values are means ± SD (Student’s t-test; **P < 0.01). (E,F) Binding of SPCH protein to ACS2 and ACS6 genes in tobacco leaves in a transient transcription dual-luciferase assay. The size and intensity of LUC fluorescence signals recorded by IndiGO software are proportional to binding ability (E). Relative binding ability was evaluated quantitatively by calculating the ratio of the fluorescence intensity of firefly luciferase (LUC) to that of an internal control, Renilla luciferase (REN) (F). Values are means ± SD (n = 3). Asterisks indicate significant differences (*P < 0.05) compared with leaf regions injected with Agrobacterium harboring an empty vector.
To confirm this experimentally, we used ChIP assays to detect the interaction between the transcription factor SPCH and the promoters of ACS2 and ACS6 under drought stress. The in silico analyses revealed three E-box motifs in the 3.0-kb promoter region of the ACS2 gene: CGCGTG and CACGTG (at −1079 and −1090), collectively named ACS2-P1 because of their close proximity, and CACGTG (at −2326), designated as ACS2-P2. Only one E-box motif was present in the 3.0-kb promoter region of the ACS6 gene: CACGTG (at −2537), named ACS6-P1 (Figure 4C). After randomly selecting DNA fragments from their promoter regions with the same length as the E-boxes (named ACS2-P and ACS6-P) as the reference, ChIP assays were performed to measure levels of immunoprecipitated DNA fragments by SPCH protein in vivo. In these assays, the abundance of DNA fragments from ACS2 promoters ACS2-P1 and ACS2-P2 was, respectively, 6.13- and 1.18-fold higher than that of the control ACS2-P (Figure 4D). Similarly, the abundance of ACS6-P1 immunoprecipitated by SPCH protein was 4.57-fold higher than that of the control ACS6-P (Figure 4D). Next, we conducted transient transcription activity assays to verify the binding of SPCH to the promoters of ACS2 and ACS6. According to the results, the fluorescence intensity of LUC linked to the specific promoter fragment of ACS2 (−900 to −1600 bp, containing ACS2-P1) was increased in the presence of SPCH, with LUC activity 4.2-times higher than that of the blank LUC control (Figures 4E,F). Similarly, the activity of LUC linked to the promoter fragment of ACS6 (−1900 to −2600 bp, containing ACS6-P1) in the presence of SPCH was 3.7-times higher than that of the blank LUC control (Figures 4E,F). This stimulatory effect was specific, as SPCH did not induce LUC activity alone or linked to the other promoter fragments of ACS2 or ACS6 gene (Supplementary Figure 6). This is, SPCH directed the transcription of ACS2 or ACS6 by docking to each of their promoter regions.
To verify that SPCH promoted ACS2/6 expression, we monitored the effects of SPCH activity on ACC levels. The results showed that the SPCH-overexpressing lines SPCH-OE(#1), SPCH-OE(#2), and SPCH-OE(#3) had significantly increased ACC levels, but the mutants spch-1 or spch-3 had reduced ACC levels in immature leaves, as compared with the ACC levels in leaves of WT (Figures 5A,B). Evidently, SPCH-directed ACS2/6 expression was directly related to ACC accumulation in immature leaves. Further observations helped to explain how SPCH mediated stomatal development via ACS2/6-dependent ACC production. The single mutant spch-1, the double mutant acs2-1acs6-1, and the triple mutant spch-1acs2-1acs6-1 had significantly reduced stomatal densities, compared with WT (Figures 5C,D). In addition, the stomatal density on leaves was lower in the triple mutant spch-1acs2-1acs6-1 than in its parents spch-1 and acs2-1acs6-1 (Figures 5C,D). Notably, ACS2- or ACS6-overexpression in spch-1 reversed the reduction in stomatal density (Figure 5D). These observations suggest that SPCH activity induced ACS2/6 activation and ACC accumulation.
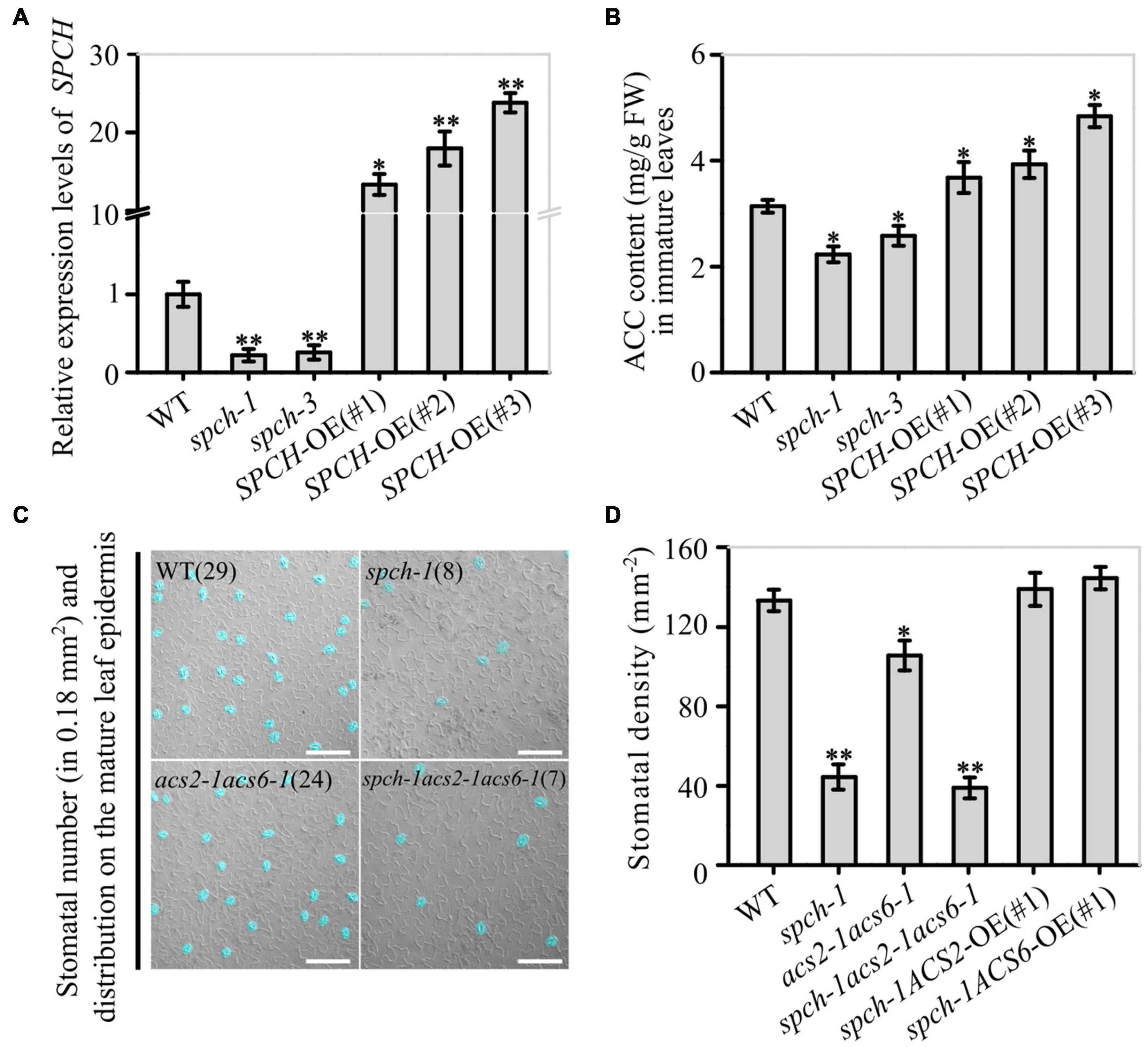
Figure 5. Correlation between SPCH activity and ACS2/6-dependent ACC accumulation and stomatal density and pattern. (A,B) RT-qPCR-based relative levels of SPCH mRNA transcripts and ACC content in immature leaves of WT control, loss-of-function mutant spch-1 and spch-3, and SPCH-overexpressing SPCH-OE(#1), SPCH-OE(#2), and SPCH-OE(#3) plants. The ACTIN8 gene was used as an internal control. The experiment was repeated three times with consistent results. Values are means ± SD (Student’s t-test; *P < 0.05; **P < 0.01). (C,D) DIC images and statistical summary of stomatal density and patterning on the abaxial epidermis of spch-1, acs2-1acs6-1, and spch-1acs2-1acs6-1 plants. Numbers of stomata are indicated in parentheses in each image. Stomata are false colored in blue for easier identification. The white scale bar represents 100 μm (C). Stomata on sixth leaves of 25 seedlings were counted (D). Values are means ± SD. Significant differences are indicated by asterisks (Student’s t-test; *P < 0.05; **P < 0.01).
ACS2/6-Generated 1-Aminocyclopropane-1-Carboxylate Accumulation Decreased the Expression Activity of STOMATAL DENSITY AND DISTRIBUTION 1 in Leaves
Evidences have shown that SDD1 expression reduces stomatal density and cluster (Yoo et al., 2010, 2019), but exogenously applied ACC increases stomatal density and cluster (Serna and Fenoll, 1996; Saibo et al., 2003; Acharya and Assmann, 2009). We therefore verified whether ACC cooperates with SDD1 to establish stomatal density and cluster.
The effect of ACS2/6-dependent ACC accumulation on SDD1 expression was surveyed in immature leaves. The SDD1 mRNA levels in acs2-1, acs6-1, acs2-1acs6-1, ACS2-OE(#1), ACS2-OE(#3), ACS6-OE(#1), and ACS6-OE(#3) were, respectively, 1.88-, 1.89-, 2.18-, 0.50-, 0.64-, 0.54-, and 0.67-fold that in WT (Figure 6A). This result suggests that SDD1 expression was negatively correlated with ACS2/6 activation in immature leaves. Further monitoring of SDD1 protein levels by western blotting indicated that GFP-marked SDD1 protein levels in immature leaves were higher in acs2-1acs6-1 than in WT under drought conditions (Figure 6B). This result is consistent with the expectation that ACC treatment would reduce SDD1 mRNA transcript levels in immature leaves of WT (Supplementary Figure 7A). As expected, ACC treatment reduced protein levels of SDD1 in WT leaves compared with the control (Supplementary Figure 7B). These data suggest that ACS2/6-generated ACC impeded SDD1 expression and SDD1 protein levels, thereby increasing stomatal density and cluster on the leaf epidermis.
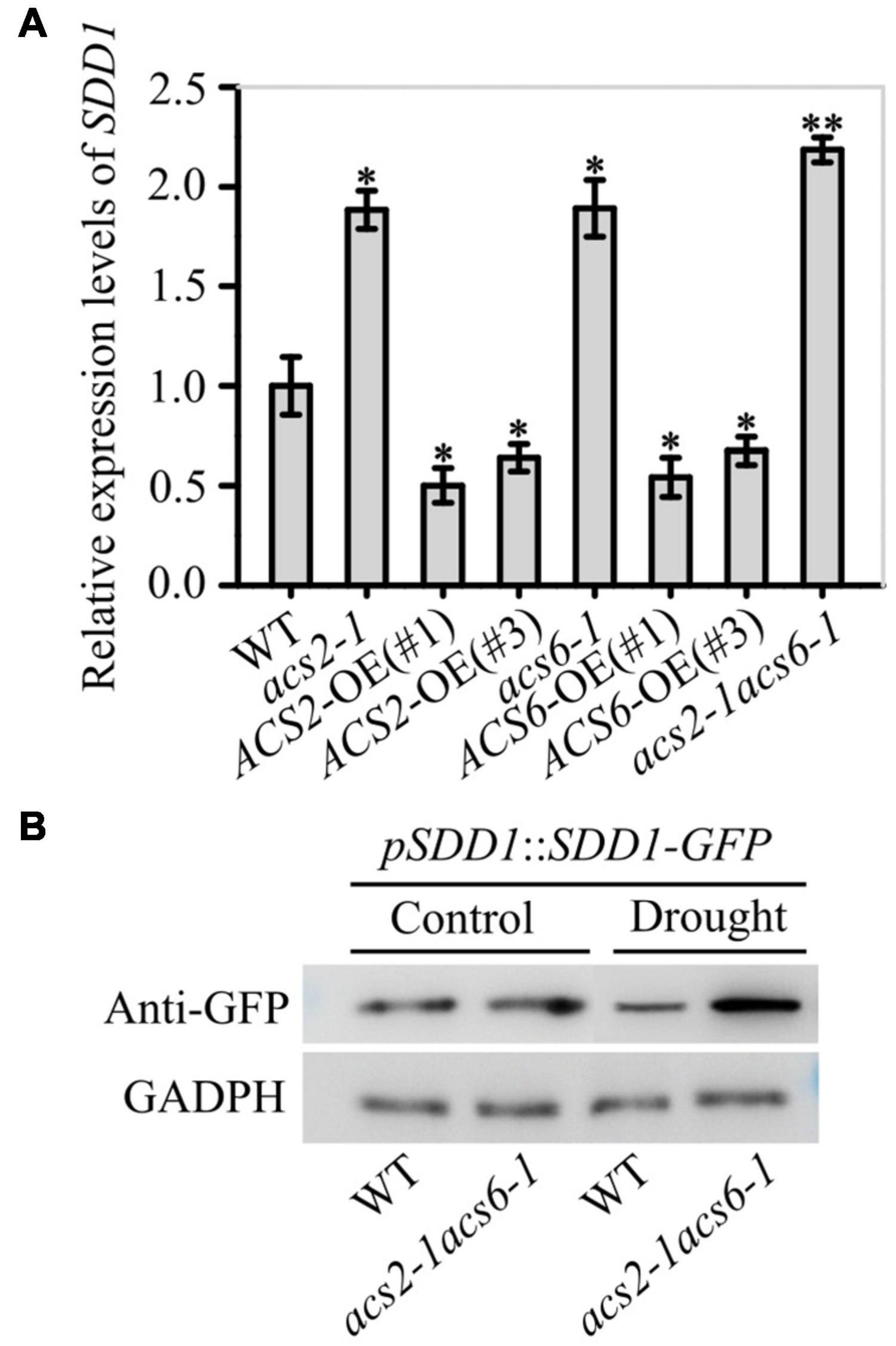
Figure 6. Inhibitory effects of ACS2/6 activation and ACC treatment on SDD1 expression and protein accumulation. (A) RT-qPCR-based relative levels of SDD1 mRNA transcripts in immature leaves of WT, acs2-1, acs6-1, acs2-1acs6-1, ACS2-OE(#1), ACS2-OE(#3), ACS6-OE(#1), and ACS6-OE(#3) seedlings. The experiment was repeated three times with consistent results. Values are means ± SD (Student’s t-test; *P < 0.05; **P < 0.01). (B) Evaluation of SDD1 protein levels by western blotting. The fusion protein GFP-SDD1 was collected from immature leaves of pSDD1::SDD1-GFP-expressing WT or acs2-1acs6-1 lines with or without drought treatment. Levels of GFP-SDD1 fusion protein were determined using GFP antibody. GAPDH protein was used as a loading control. The experiment was repeated three times with consistent results.
1-Aminocyclopropane-1-Carboxylate Buffered Ca2+ Activity in Stomatal Lineage Cells
Studies have shown that the trihelix transcription factor GTL1 is the inhibitor of SDD1 expression, and that Ca2+-loaded calmodulin can release the binding of GTL1 in the promoter region of SDD1 gene in stomatal lineage (Yoo et al., 2010, 2019; Weng et al., 2012; Virdi et al., 2015). That is to say, the increase of Ca2+ levels plays important roles in the establishment of stomatal space based on the SDD1 activity. Thus, it is required to monitor whether and how ACC mediates Ca2+ levels in stomatal lineage cells on the leaf epidermis under drought conditions.
We preliminarily evaluated the Ca2+ levels in stomatal lineage cells on the immature leaf epidermis by using the Ca2+ fluorescence probe Fluo-4/AM. Under normal conditions, the Ca2+ levels in stomatal lineage cells were slightly higher in acs2-1acs6-1 than in WT. However, after halting watering for 6 days, the Ca2+ levels in stomatal lineage cells were higher in acs2-1acs6-1 than in WT (Supplementary Figure 8). This hints that the decreased ACC accumulation increased Ca2+ levels in stomatal lineage cells.
The Ca2+-sensitive yellow cameleon protein YC3.6 has been developed as a Ca2+ biosensor (Krebs et al., 2012; Behera et al., 2017). After creating NES-YC3.6 expressing acs2-1acs6-1 lines, both fluorescence-symbolized and cpVenus/CFP ratio-labeled Ca2+ levels were analyzed. Under normal conditions, the fluorescence intensity of YC3.6 protein was slightly higher in acs2-1acs6-1 than in WT (Figure 7A). Moreover, the cpVenus/CFP ratio was slightly higher in acs2-1acs6-1 than in WT (Figure 7B). After halting watering for 6 days, the fluorescence intensity of YC3.6 protein was significantly higher in acs2-1acs6-1 than in WT (Figure 7A). Specifically, the Ca2+ levels in the stomatal lineage cells was 3.38-times higher in acs2-1acs6-1 than in WT (Figure 7B). That is, ACC accumulation in leaves significantly reduced Ca2+ levels in stomatal lineage cells, which is the basis of decreasing SDD1 activity and of increasing stomatal density and clustered stomata.
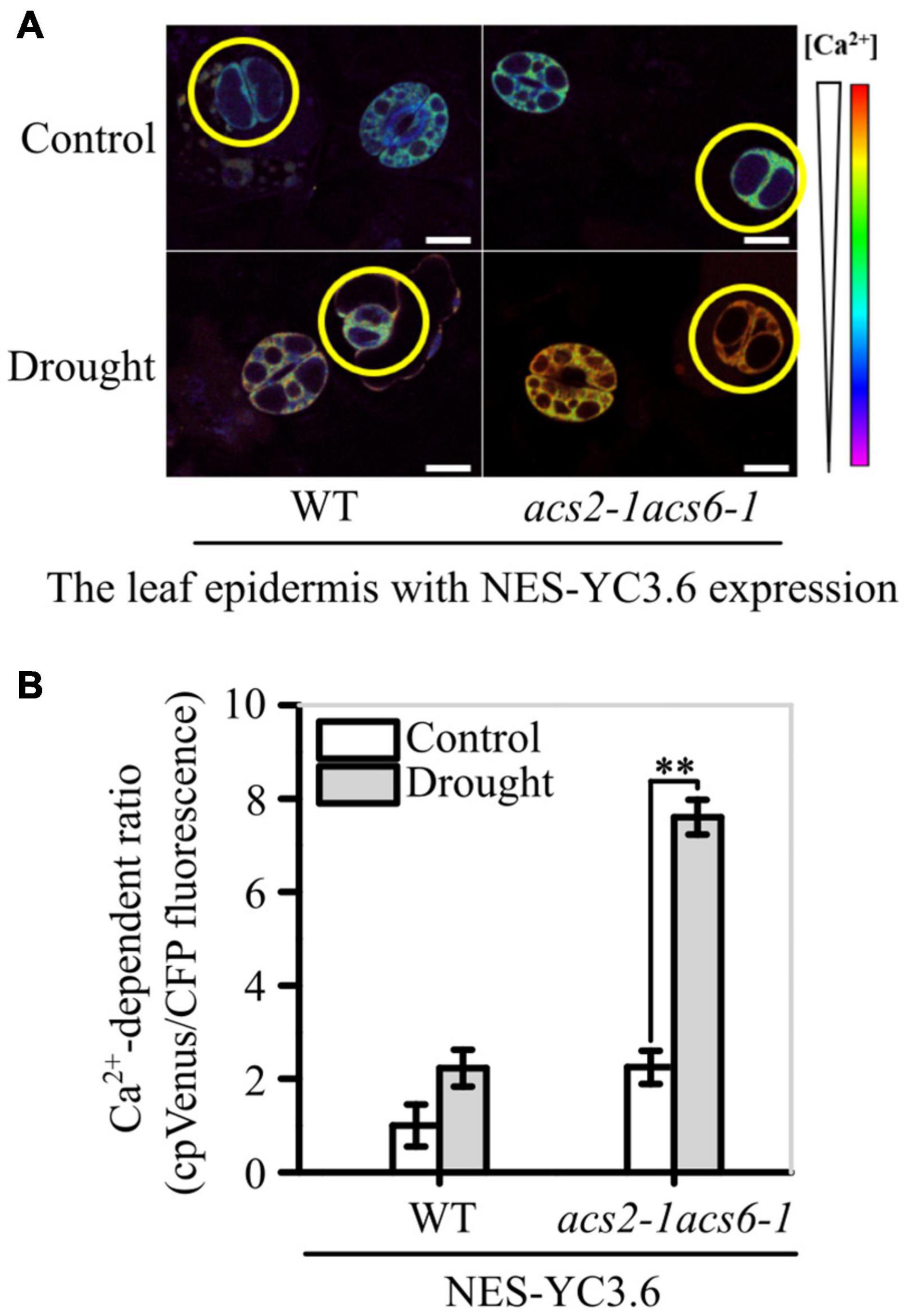
Figure 7. ACS2/6-generated ACC buffering of Ca2+ activity in stomatal lineage cells. (A) The relative fluorescence intensity of YC3.6 expression in stomatal lineage cells of immature leaves of NES-YC3.6-expressing WT or acs2-1acs6-1 plants or by drought treatment. Representative images from at least 25 leaves in each line are shown. Pseudocolors in images correspond to relative Ca2+ levels according to the color scale on the right. Scale bar, 10 μm. (B) Evaluation of Ca2+ levels in stomatal lineage cells (labeled with yellow circle) of immature by calculating the ratio of the FRET acceptor cpVenus (at 525–555 nm) to the FRET donor CFP (at 465–499 nm). The experiment was repeated three times with consistent results. Values are means ± SD (Student’s t-test; **P < 0.01).
Discussion
These findings first disclosed the mechanisms by which ACS2/6 activation directs the establishment of stomatal space by integrating SPCH signal with SDD1 function under drought conditions. A schematic overview of the inter-relationships among these processes is provided in Figure 8.
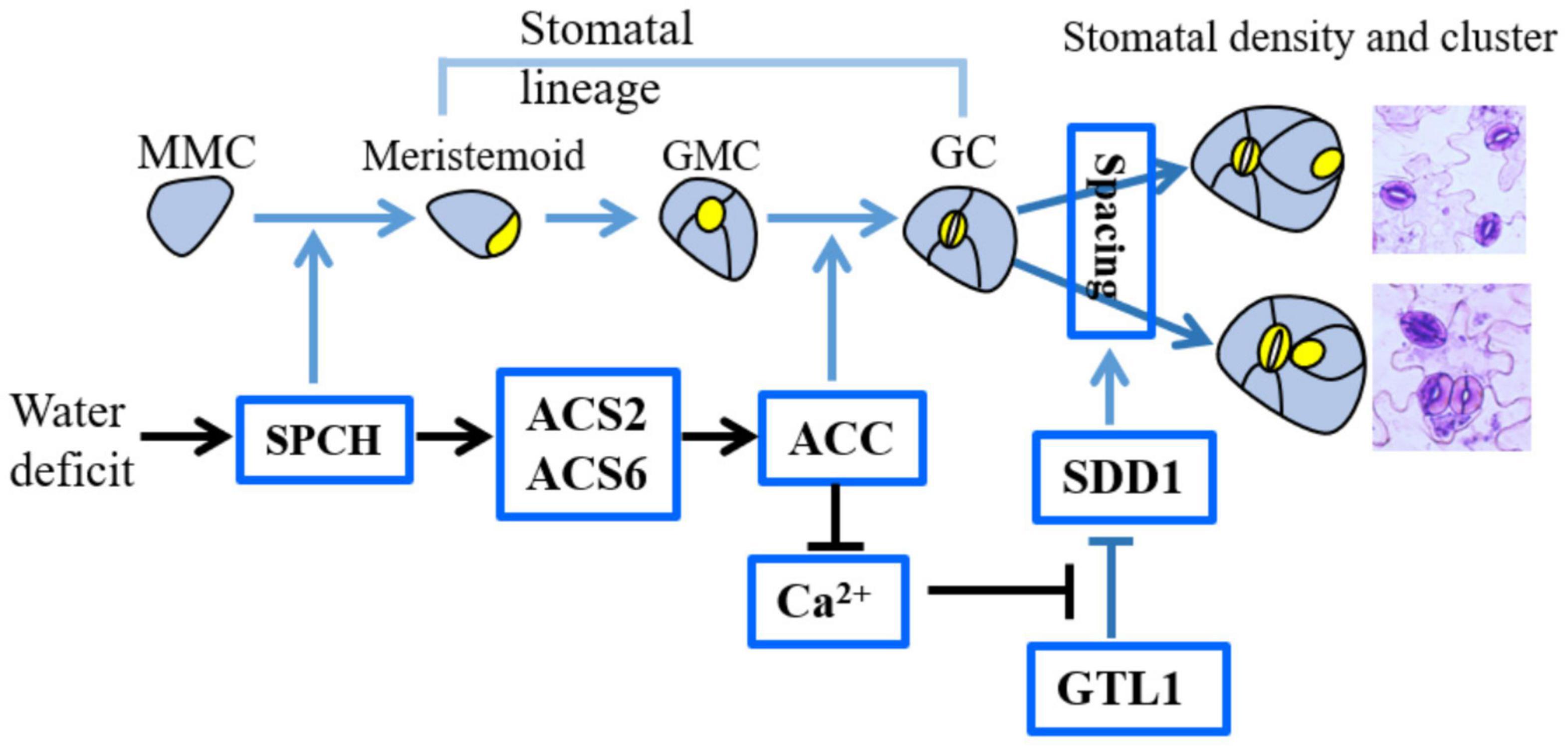
Figure 8. Diagram illustrating the role of ACS2/6 expression and ACC accumulation in the integration of SPCH-initiated stomatal development with SDD1-dependent stomatal spacing. In the top row depicting stomatal development, non-stomatal lineage cells, such as leaf epidermal cells, protodermal cells, and meristemoid mother cells (MMCs), are shown in blue–gray, while stomatal lineage cells, including meristemoid cells, guard mother cells (GMCs), and guard cell (GCs), are indicated in bright yellow. The factors involved in this study are shown in the bright blue box. Under water deficit conditions, SPCH increases ACS2 and ACS6 expressions. Next, ACS2/6-generated ACC accumulation may be involved in two processes: (1) promoting the transformation of GMCs into GCs, and (2) inducing a shortage of Ca2+ in stomatal lineage cells and decreasing SDD1 activity. As a consequence of these two processes, ACS2/6-generated ACC accumulation increases stomatal density, stomatal index and the rate of clustering. Relationships among these events are indicated by arrows: blue for conclusions drawn from the literature, and black for findings of the present study.
The activation of ACS2/6 lays the foundation for the ACC-dependent establishment of stomatal space in response to mild drought. According to our data, stomatal density (Figure 3B), stomatal index (Supplementary Figure 3B) and clustering ratio (Figure 3C) on the leaf epidermis were reduced in loss-of-function mutants acs2-1, acs6-1, and acs2-1acs6-1, but were increased in ACS2- and ACS6-overexpression lines; in other words, drought-activated ACS2/6 increased stomatal density, stomatal index and clustering, and these facilitated stomata-based water evaporation, in turn, seedlings withered and some even died with drought escalating. This finding provides a genetic explanation for the decrease in stomatal density and clustering caused by ACS activity inhibitor AVG in Arabidopsis (Serna and Fenoll, 1996; Saibo et al., 2003; Yin et al., 2019), and also provide theoretical explanations why ACS2- or ACS6-deficient rice (Zhang et al., 2013) or maize (Young et al., 2004) are less sensitive to water deficit than are WT controls. Considering the specificity of ACS activity (Tsuchisaka and Theologis, 2004; Tsuchisaka et al., 2009; Han et al., 2019; Lv et al., 2019), we presume that ACS2/6 activation is specific to stomatal development and spacing on the leaf epidermis when Arabidopsis seedlings are under drought. Because drought can induce ACS2/6 activation and ACC accumulation (Catalá et al., 2014; Dubois et al., 2018), the observed increase in stomatal density and cluster under drought conditions (Figures 2, 3) is easily understandable. Simply, ACS2/6-dependent ACC accumulation increases the susceptibility of seedlings to drought. Nevertheless, we could not rule out the possibility that ethylene followed ACC accumulation to be involved in stomatal density and cluster setting, because an ethylene perceiving blocker silver ions (Ag2+) mimicked AVG to establish stomatal density and cluster on the cotyledon epidermis of Arabidopsis (Serna and Fenoll, 1996). Furthermore, activated ACS2 and ACS6 may function in parallel, as growth phenotypes (Supplementary Figure 1), stomatal densities and clustering (Figure 3) were similar among acs2-1, acs6-1, and acs2-1acs6-1 mutants, and the expressions of ACS6 and ACS2 were relatively unaffected in the two single mutants acs2-1 and acs6-1, respectively (Figure 1). Importantly, our results show that SPCH separately regulates the expression of ACS2 and ACS6 by binding to their promoters (Figure 4). We speculate that ACS2 and ACS6 jointly ensure plants to fully respond to frequent drought stimuli.
ACS2/6-dependent ACC production is a turning point from SPCH-based stomatal development to the SDD1-directed establishment of stomatal space. Evidence for this conclusion is as follows: First, in line with a previous prediction (Lau et al., 2014), our results provide evidence that SPCH acts as a transcription factor to control the expression of ACS2 and ACS6 (Figure 4). The ability of SPCH to promote ACS2 and ACS6 expression was evidenced by the fact, for example, that spch-1 and spch-3 mutants showed reduced expressions of these genes (Figure 4A) and ACC content, whereas SPCH overexpression led to increased ACC levels (Figure 5B). This finding explains why plant tolerance to osmotic stress requires reduced SPCH activity (Han and Torii, 2016; Tripathi et al., 2016; Zoulias et al., 2018). Second, ACC mimics SPCH to reduce SDD1 activity, thereby increasing stomatal density and cluster (Figure 6 and Supplementary Figure 7). The mutants acs2-1, acs6-1, and acs2-1acs6-1 seedlings (Figure 2) mimicked transgenic SDD1-overexpressing plants by showing reduced stomatal density and cluster on leaves. Consistent with this observation, both the sdd1-1 mutant (Von Groll et al., 2002) and ACS2- and ACS6-overexpressing plants (Figure 3) exhibited increased stomatal density and cluster on the leaves. Third, ACC-associated Ca2+ insufficiency reduced SDD1 activity, or, alternatively, Ca2+ activity, in stomatal lineage cells, so that ACC levels were linked to SDD1 expression. Our findings indicated that Ca2+ levels in stomatal lineage cells on the leaf epidermis were higher in acs2-1acs6-1 plants than in WT (Figure 7 and Supplementary Figure 8). This suggests that ACC accumulation inhibits SDD1 activity by controlling Ca2+ activity in stomatal lineage cells. This result is reasonable because a Ca2+ shortage can stabilize the binding of GTL1 to the SDD1 promoter to prevent its expression in stomatal lineage cells (Yoo et al., 2019). These findings explicate the mechanisms in the recent discovery that Ca2+ activity intensifies stomata-based water evaporation from leaves of Arabidopsis seedlings under drought conditions (Teardo et al., 2019).
In summary, mild drought induces ACS2/6 activation and ACC accumulation, and thus reduces stomatal space, or increases stomatal density and cluster. This increase may be an adaptive response to mild drought, as the increase of stomata is beneficial to water absorption of plant roots, according to the view of evolution from aquatic to terrestrial (Croxdale, 2000; van Veen and Sasidharan, 2021). But this increase may also lead to wilting and drying of plants if drought intensifies and escalates. These findings provide a clear evidence that moderate drought increases stomata density and cluster, and a novel evidence that ACS2/6 activation is the key factor in the establishment of stomatal density and clustered ratio on the Arabidopsis leaf epidermis under moderate drought.
Data Availability Statement
The datasets presented in this study can be found in online repositories. The names of the repository/repositories and accession number(s) can be found in the article/Supplementary Material.
Author Contributions
JJ conceived the study and supervised the whole research. M-ZJ, L-YL, and CG performed experiments and analyzed the data. JJ and M-ZJ wrote the manuscript. All authors approved the submitted version.
Funding
This work was supported by the National Natural Science Foundation of China (31970735 and 31271510) to JJ.
Conflict of Interest
The authors declare that the research was conducted in the absence of any commercial or financial relationships that could be construed as a potential conflict of interest.
Publisher’s Note
All claims expressed in this article are solely those of the authors and do not necessarily represent those of their affiliated organizations, or those of the publisher, the editors and the reviewers. Any product that may be evaluated in this article, or claim that may be made by its manufacturer, is not guaranteed or endorsed by the publisher.
Acknowledgments
We thank Xiao-lan Chen (School of Life Sciences, Yunnan University, China) provided seeds of spch-1 and the transgenic pSPCH::SPCH-GFP line, Sui-wen Hou (MOE Key Laboratory of Cell Activities and Stress Adaptations, Lanzhou, China) provided seeds of spch-3, and Jörg Kudla (Institute of Biology and Biotechnology of Plants, University of Munster, Germany) provided seeds of the Ca2+ sensor NES-YC3.6-expressing line.
Supplementary Material
The Supplementary Material for this article can be found online at: https://www.frontiersin.org/articles/10.3389/fpls.2021.758785/full#supplementary-material
Footnotes
References
Acharya, B. R., and Assmann, S. M. (2009). Hormone interactions in stomatal function. Plant Mol. Biol. 69, 451–462.
Bao, Y., Wang, C., Jiang, C., Pan, J., Zhang, G., Liu, H., et al. (2014). The tumor necrosis factor receptor-associated factor (TRAF)-like family protein SEVEN IN ABSENTIA 2 (SINA2) promotes drought tolerance in an ABA-dependent manner in Arabidopsis. New Phytol. 202, 174–187. doi: 10.1111/nph.12644
Behera, S., Long, Y., Schmitz-Thom, I., Wang, X. P., Zhang, C., Li, H., et al. (2017). Two spatially and temporally distinct Ca2 + signals convey Arabidopsis thaliana responses to K+ deficiency. New Phytol. 213, 739–750. doi: 10.1111/nph.14145
Berger, D., and Altmann, T. (2000). A subtilisin-like serine protease involved in the regulation of stomatal density and distribution in Arabidopsis thaliana. Genes Dev. 14, 1119–1131.
Bleecker, A. B., and Kende, H. (2000). Ethylene: a gaseous signal molecule in plants. Annu. Rev. Cell Dev. Biol. 16, 1–18. doi: 10.1146/annurev.cellbio.16.1.1
Casson, S., and Gray, J. E. (2008). Influence of environmental factors on stomatal development. New Phytol. 178, 9–23. doi: 10.1111/j.1469-8137.2007.02351.x
Catalá, R., López-Cobollo, R., Mar Castellano, M., Angosto, T., Alonso, J. M., Ecker, J. R., et al. (2014). The Arabidopsis 14-3-3 protein RARE COLD INDUCIBLE 1A links low-temperature response and ethylene biosynthesis to regulate freezing tolerance and cold acclimation. Plant Cell 26, 3326–3342. doi: 10.1105/tpc.114.127605
de Marcos, A., Houbaert, A., Triviño, M., Delgado, D., Martín-Trillo, M., Russinova, E., et al. (2017). A mutation in the bHLH domain of the SPCH transcription factor uncovers a BR-dependent mechanism for stomatal development. Plant Physiol. 174, 823–842. doi: 10.1104/pp.17.00615
Dubois, M., Claeys, H., Van den Broeck, L., and Inzé, D. (2017). Time of day determines Arabidopsis transcriptome and growth dynamics under mild drought. Plant Cell Environ. 40, 180–189. doi: 10.1111/pce.12809
Dubois, M., Van den Broeck, L., and Inzé, D. (2018). The pivotal role of ethylene in plant growth. Trends Plant Sci. 23, 311–323. doi: 10.1016/j.tplants.2018.01.003
Hamanishi, E. T., Thomas, B. R., and Campbell, M. M. (2012). Drought induces alterations in the stomatal development program in Populus. J. Exp. Bot. 63, 4959–4971. doi: 10.1093/jxb/ers177
Han, S. K., and Torii, K. U. (2016). Lineage-specific stem cells, signals and asymmetries during stomatal development. Development 143, 1259–1270. doi: 10.1242/dev.127712
Han, X., Hu, Y., Zhang, G., Jiang, Y., Chen, X., and Yu, D. (2018). Jasmonate negatively regulates stomatal development in Arabidopsis cotyledons. Plant Physiol. 176, 2871–2885. doi: 10.1104/pp.17.00444
Han, S., Jia, M. Z., Yang, J. F., and Jiang, J. (2019). The integration of ACS2-generated ACC with GH3-mediated IAA homeostasis in NaCl-stressed primary root elongation of Arabidopsis seedling. Plant Growth Regul. 88, 151–158.
Hepworth, C., Doheny-Adams, T., Hunt, L., Cameron, D. D., and Gray, J. E. (2015). Manipulating stomatal density enhances drought tolerance without deleterious effect on nutrient uptake. New Phytol. 208, 336–341. doi: 10.1111/nph.13598
Krebs, M., Held, K., Binder, A., Hashimoto, K., Den Herder, G., Parniske, M., et al. (2012). FRET-based genetically encoded sensors allow high-resolution live cell imaging of Ca2 + dynamics. Plant J. 69, 181–192. doi: 10.1111/j.1365-313X.2011.04780.x
Lau, O. S., Davies, K. A., Chang, J., Adrian, J., Rowe, M. H., Ballenger, C. E., et al. (2014). Direct roles of SPEECHLESS in the specification of stomatal self-renewing cells. Science 345, 1605–1609. doi: 10.1126/science.1256888
Livak, K. J., and Schmittgen, T. D. (2001). Analysis of relative gene expression data using real-time quantitative PCR and the 2−ΔΔC(T) method. Methods 25, 402–408. doi: 10.1006/meth.2001.1262
Lv, S. F., Jia, M. Z., Zhang, S. S., Han, S., and Jiang, J. (2019). The dependence of leaf senescence on the balance between 1-aminocyclopropane-1-carboxylate acid synthase 1 (ACS1) -catalyzed ACC generation and nitric oxide associated 1 (NOS1)-dependent NO accumulation in Arabidopsis. Plant Biol. 21, 595–603. doi: 10.1111/plb.12970
MacAlister, C. A., Ohashi-Ito, K., and Bergmann, D. C. (2007). Transcription factor control of asymmetric cell divisions that establish the stomatal lineage. Nature 445, 537–540.
Nagai, T., Yamada, S., Tominaga, T., Ichikawa, M., and Miyawaki, A. (2004). Expanded dynamic range of fluorescent indicators for Ca2+ by circularly permuted yellow fluorescent proteins. Proc. Natl. Acad. Sci. U.S.A. 101, 10554–10559. doi: 10.1073/pnas.0400417101
Pillitteri, L. J., Sloan, D. B., Bogenschutz, N. L., and Torii, K. U. (2007). Termination of asymmetric cell division and differentiation of stomata. Nature 445, 501–505. doi: 10.1038/nature05467
Qi, S. L., Lin, Q. F., Feng, X. J., Han, H. L., Liu, J., Zhang, L., et al. (2019). IDD16 negatively regulates stomatal initiation via trans-repression of SPCH in Arabidopsis. Plant Biotechnol. J. 17, 1446–1457. doi: 10.1111/pbi.13070
Saibo, N. J., Vriezen, W. H., Beemster, G. T., and Van Der Straeten, D. (2003). Growth and stomata development of Arabidopsis hypocotyls are controlled by gibberellins and modulated by ethylene and auxins. Plant J. 33, 989–1000. doi: 10.1046/j.1365-313x.2003.01684.x
Serna, L. (2009). Cell fate transitions during stomatal development. Bioessays 31, 865–873. doi: 10.1002/bies.200800231
Serna, L., and Fenoll, C. (1996). Ethylene induces stomata differentiation in Arabidopsis. Int. J. Dev. Biol. 1, 123S–124S.
Teardo, E., Carraretto, L., Moscatiello, R., Cortese, E., Vicario, M., Festa, M., et al. (2019). A chloroplast-localized mitochondrial calcium uniporter transduces osmotic stress in Arabidopsis. Nat. Plants 5, 581–588. doi: 10.1038/s41477-019-0434-8
Tripathi, P., Rabara, R. C., Reese, R. N., Miller, M. A., Rohila, J. S., Subramanian, S., et al. (2016). A toolbox of genes, proteins, metabolites and promoters for improving drought tolerance in soybean includes the metabolite coumestrol and stomatal development genes. BMC Genomics 17:102. doi: 10.1186/s12864-016-2420-0
Tsuchisaka, A., and Theologis, A. (2004). Unique and overlapping expression patterns among the Arabidopsis 1-amino-cyclopropane-1-carboxylate synthase gene family members. Plant Physiol. 136, 2982–3000. doi: 10.1104/pp.104.049999
Tsuchisaka, A., Yu, G., Jin, H., Alonso, J. M., Ecker, J. R., Zhang, X., et al. (2009). A combinatorial interplay among the 1-aminocyclopropane-1-carboxylate isoforms regulates ethylene biosynthesis in Arabidopsis thaliana. Genetics 183, 979–1003. doi: 10.1534/genetics.109.107102
van Veen, H., and Sasidharan, R. (2021). Shape shifting by amphibious plants in dynamic hydrological niches. New Phytol. 229, 79–84. doi: 10.1111/nph.16347
Virdi, A. S., Singh, S., and Singh, P. (2015). Abiotic stress responses in plants: roles of calmodulin-regulated proteins. Front. Plant Sci. 6:809. doi: 10.3389/fpls.2015.00809
Von Groll, U., Berger, D., and Altmann, T. (2002). The subtilisin-like serine protease SDD1 mediates cell-to-cell signaling during Arabidopsis stomatal development. Plant Cell 14, 1527–1539. doi: 10.1105/tpc.001016
Weng, H., Yoo, C. Y., Gosney, M. J., Hasegawa, P. M., and Mickelbart, M. V. (2012). Poplar GTL1 is a Ca2+/calmodulin-binding transcription factor that functions in plant water use efficiency and drought tolerance. PLoS One 7:e32925. doi: 10.1371/journal.pone.0032925
Xie, Z., Nolan, T., Jiang, H., Tang, B., Zhang, M., Li, Z., et al. (2019). The AP2/ERF transcription factor tiny modulates brassinosteroid-regulated plant growth and drought responses in Arabidopsis. Plant Cell 31, 1788–1806. doi: 10.1105/tpc.18.00918
Yang, Z., Liu, J., Tischer, S. V., Christmann, A., Windisch, W., Schnyder, H., et al. (2016). Leveraging abscisic acid receptors for efficient water use in Arabidopsis. Proc. Natl. Acad. Sci. U.S.A. 113, 6791–6796. doi: 10.1073/pnas.1601954113
Yin, J., Zhang, X., Zhang, G., Wen, Y., Liang, G., and Chen, X. (2019). Aminocyclopropane-1-carboxylic acid is a key regulator of guard mother cell terminal division in Arabidopsis thaliana. J. Exp. Bot. 70, 897–908. doi: 10.1093/jxb/ery413
Yoo, C. Y., Mano, N., Finkler, A., Weng, H., Day, I. S., Reddy, A. S. N., et al. (2019). A Ca2 +/CaM-regulated transcriptional switch modulates stomatal development in response to water deficit. Sci. Rep. 9:12282. doi: 10.1038/s41598-019-47529-2
Yoo, C. Y., Pence, H. E., Jin, J. B., Miura, K., Gosney, M. J., Hasegawa, P. M., et al. (2010). The Arabidopsis GTL1 transcription factor regulates water use efficiency and drought tolerance by modulating stomatal density via transrepression of SDD1. Plant Cell 22, 4128–4141. doi: 10.1105/tpc.110.078691
Young, T. E., Meeley, R. B., and Gallie, D. R. (2004). ACC synthase expression regulates leaf performance and drought tolerance in maize. Plant J. 40, 813–825. doi: 10.1111/j.1365-313X.2004.02255.x
Zhang, H., Zhang, J., Quan, R., Pan, X., Wan, L., and Huang, R. (2013). EAR motif mutation of rice OsERF3 alters the regulation of ethylene biosynthesis and drought tolerance. Planta 237, 1443–1451. doi: 10.1007/s00425-013-1852-x
Keywords: ACC, ACS, Ca2+, stomatal density, stomatal cluster, stomatal space, drought
Citation: Jia M-z, Liu L-y, Geng C and Jiang J (2021) Activation of 1-Aminocyclopropane-1-Carboxylic Acid Synthases Sets Stomatal Density and Clustered Ratio on Leaf Epidermis of Arabidopsis in Response to Drought. Front. Plant Sci. 12:758785. doi: 10.3389/fpls.2021.758785
Received: 15 August 2021; Accepted: 16 November 2021;
Published: 06 December 2021.
Edited by:
Abidur Rahman, Iwate University, JapanReviewed by:
Gyeong Mee Yoon, Purdue University, United StatesSaptarshi Hazra, Fertis India Pvt. Ltd., India
Copyright © 2021 Jia, Liu, Geng and Jiang. This is an open-access article distributed under the terms of the Creative Commons Attribution License (CC BY). The use, distribution or reproduction in other forums is permitted, provided the original author(s) and the copyright owner(s) are credited and that the original publication in this journal is cited, in accordance with accepted academic practice. No use, distribution or reproduction is permitted which does not comply with these terms.
*Correspondence: Jing Jiang, jiangjing@henu.edu.cn