- 1Centro de Ciencias Genómicas, Universidad Nacional Autónoma de México, Cuernavaca, Mexico
- 2Programa de Doctorado en Ciencias Biomédicas, Centro de Ciencias Genómicas, Universidad Nacional Autónoma de México, Cuernavaca, Mexico
The chemical composition of a plant cuticle can change in response to various abiotic or biotic stresses and plays essential functions in disease resistance responses. Arabidopsis thaliana mutants altered in cutin content are resistant to Botrytis cinerea, presumably because of increased cuticular water and solute permeability, allowing for faster induction of defense responses. Within this context, our knowledge of wax mutants is limited against this pathogen. We tested the contribution of cuticular components to immunity to B. cinerea using mutants altered in either cutin or wax alone, or in both cutin and wax contents. We found that even all the tested mutants showed increased permeability and reactive oxygen species (ROS) accumulation in comparison with wild-type plants and that only cutin mutants showed resistance. To elucidate the early molecular mechanisms underlying cuticle-related immunity, we performed a transcriptomic analysis. A set of upregulated genes involved in cell wall integrity and accumulation of ROS were shared by the cutin mutants bdg, lacs2-3, and eca2, but not by the wax mutants cer1-4 and cer3-6. Interestingly, these genes have recently been shown to be required in B. cinerea resistance. In contrast, we found the induction of genes involved in abiotic stress shared by the two wax mutants. Our study reveals new insight that the faster recognition of a pathogen by changes in cuticular permeability is not enough to induce resistance to B. cinerea, as has previously been hypothesized. In addition, our data suggest that mutants with resistant phenotype can activate other defense pathways, different from those canonical immune ones.
Introduction
A cuticle is a hydrophobic structure that covers the surface of the epidermal cells of the aerial parts of plants, such as leaves, stems, flowers, seeds, and fruits; and represents one of the evolutionary adaptations that has allowed plants to counteract the adverse effects produced by biotic and abiotic factors (Jeffree, 2006; Jetter et al., 2006; Riederer, 2006; Nawrath et al., 2013). The structure and chemical composition of a cuticle vary widely among different plant species, and even between organs and stages of its development (Jeffree, 2006; Nawrath et al., 2013; Ingram and Nawrath, 2017). Despite this variability, all cuticles are mainly made up of two types of lipid compounds: cutin and waxes. Cutin is a polymer layer formed by a network of esterified ω-hydroxylated fatty acids via intermolecular ester bonds, leading to a three-dimensional structure that is produced and secreted by epidermal cells. Waxes comprise a mixture of very long-chain fatty acids (VLCFAs, 24–36 carbon atoms) and their derivatives, including alkanes, alcohols, and aldehydes, together with secondary metabolites, such as flavonoids and triterpenoids (Li-Beisson et al., 2013; Nawrath et al., 2013; Fernández et al., 2016).
Genetic approaches that use mutagenized populations of Arabidopsis thaliana (Bernard and Joubès, 2013; Yeats and Rose, 2013; Borisjuk et al., 2014; Domínguez et al., 2015; Fich et al., 2016), tomato, and maize (Isaacson et al., 2009; Javelle et al., 2010; Girard et al., 2012) have allowed for the identification of many key enzymes involved in cuticle biosynthesis and deposition. Some of these mutants, such as the cutin mutants bodyguard (bdg), lacs2, lacerata (lcr/cyp86a8), cyp86a2/att1, abcg32/pec1, and myb96 (Wellesen et al., 2001; Schnurr et al., 2004; Xiao et al., 2004; Kurdyukov et al., 2006; Seo and Park, 2010; Benikhlef et al., 2013; Fabre et al., 2016; Zhao et al., 2019), and the wax mutants fiddlehead (fdh/kcs10), cer1, cer3/wax2, and dewax (Yephremov et al., 1999; Chen et al., 2003; Kurata et al., 2003; Rowland et al., 2007; Voisin et al., 2009; Sakuradani et al., 2013; Go et al., 2014; Liu et al., 2020) show a strong reduction in cutin and wax contents. Despite the loss of its cuticular structure, which might be thought to be detrimental to the plant, these mutants can accumulate significantly either more cutin monomers or more wax components when the other is reduced relative to wild type as a compensatory mechanism to maintain the integrity of cuticle (Voisin et al., 2009; Nawrath et al., 2013; Serrano et al., 2014).
To date, studies have identified the role of cuticular components during interaction with pathogens, showing that a cuticle is a physical and chemical barrier and that its components may act as signaling and defense molecules for both fungi and plants (Serrano et al., 2014; Aragón et al., 2017; Ziv et al., 2018). For instance, cutin monomers induce the germination of Magnaporthe grisea during the infection process of rice (Oryza sativa) (Gilbert et al., 1996), appressorium formation of the powdery mildew Erysiphe graminisin barley (Hordeum vulgare) (Francis et al., 1996), and induction of a protein kinase-mediated pathway required for the pathogenic development of Colletotrichum trifolii (Dickman et al., 2003). Besides the cutin monomers, specific wax components, such as very-long-chain (VLC) aldehydes, induce the pre-penetration process of Blumeria graminis both in vitro (Hansjakob et al., 2010) and in planta (Hansjakob et al., 2011). Additionally, other wax components, such as VLC primary alcohols of avocado (Persea americana), induce germination and appressorium formation of Colletotrichum gloeosporioides (Podila et al., 1993). In contrast, plants might perceive cutin monomers released by the action of fungal cutinase as elicitors. This hypothesis was evaluated when rice and barley plants were treated with synthetic cutin monomers (C18 fatty acids) and showed resistance to E. graminis and M. grisea, respectively (Schweizer et al., 1996). In the same way, cucumber seedlings respond to hydrolyzates of cutin by producing H2O2, which has been associated with early defense responses against pathogens (Fauth et al., 1998).
Cuticular mutants and transgenic lines have contributed to the advancement of our knowledge of how defects in cuticle structure might lead to immunity of plants upon the attack by pathogenic fungi. Fungal cutinase-expressing (CUTE) plants and A. thaliana cutin mutants with an altered ultrastructure and increased permeability of the cuticle, such as lacs2 (deficient in the long-chain acyl-CoA synthetase 2 enzyme that catalyzes the synthesis of intermediates in the cutin pathway and in wax biosynthesis), bdg (mutated in BODYGUARD, an extracellular α/β hydrolase suggested to be involved in cutin polyester assembly), and lcr (mutated in CYP86A8, which is involved in the biosynthesis of cutin pathway), displayed increased resistance to the necrotrophic fungus Botrytis cinerea (Sieber et al., 2000; Bessire et al., 2007; Chassot et al., 2007; Tang et al., 2007; Voisin et al., 2009). Likewise, we have recently described that a mutant with a strong reduction in both cutin and wax contents, eca2 (expression constitutiva del genATL2), is resistant to B. cinerea and to the hemibiotrophic bacterial pathogen Pseudomonas syringae pv tomato strain DC3000 (Pst DC3000), but susceptible to Phytophthora brassicae compared with WT plants (Blanc et al., 2018). In contrast, the mutants lacs2,acp4, and myb96 with altered cutin content exhibited enhanced susceptibility against Pst DC3000 (Tang et al., 2007; Xia et al., 2009; Seo and Park, 2010). Besides these cutin mutants, only a few A. thaliana mutants with defects in wax biosynthesis or regulation have been screened for their responses to different pathogens. For instance, cer1-1 mutants affected in CER1 (wax biosynthetic gene fora VLC-aldehyde decarbonylase) have a significantly reduced wax load, showed susceptibility to the necrotrophic fungus Sclerotinia sclerotiorum (Aarts et al., 1995; Bourdenx et al., 2011), and enhanced resistance to the biotrophic fungus Golovinomyces orontii, whereas, in cer3-6 and cer3-8 mutants affected in CER3/WAX2/YRE (a wax biosynthetic gene for VLC-acyl-CoA reductase), the growth and reproduction of these fungi were slightly inhibited (Rowland et al., 2007; Inada and Savory, 2011). An evaluation of in planta bacterial growth in cer1-1 confirmed susceptibility to bacterium Pst DC3000 (Xia et al., 2009; Bourdenx et al., 2011), as well as in the mutant cer3-6 (Lee et al., 2016). The dewax mutant (knockout in DEWAX that codified to the transcription factor DEWAX, which represses cuticular wax biosynthesis) has been reported to be more susceptible to B. cinerea (Go et al., 2014; Ju et al., 2017). In order to explain the resistance against B. cinerea observed on mutants with altered cutin composition, several reports have characterized physiological changes and the induction of defense responses. These reports include analysis on cuticular water and solute permeability (hereafter referred to as cuticular permeability), the production of reactive oxygen species (ROS), expression of genes implicated in plant defense signaling pathways (Bessire et al., 2007; Chassot et al., 2007; Voisin et al., 2009; L’Haridon et al., 2011; Blanc et al., 2018), and analysis of the abscisic acid (ABA) signaling pathway (L’Haridon et al., 2011; Cui et al., 2016). Based on these reports, a model to explain the cuticle-derived resistance to B. cinerea was proposed. In the cutin mutants, changes in cuticular structure and permeability allow pathogen- and/or damage-associated molecular patterns (PAMPs or DAMPs), released from both the pathogen and the plant cuticle or cell wall, respectively, to be more rapidly recognized by plant pattern recognition receptors, triggering immune responses (Serrano et al., 2014). Nevertheless, we are far away from fully understanding the early mechanisms and role(s) that cuticular components might play during the plant-fungal pathogen interaction, especially against B. cinerea, which leads to resistance.
In this report, we characterized mutants altered in either cutin (bdg, lacs2-3) or wax (cer1-4 and cer3-6) alone, or altered in both cutin and wax (eca2) contents during the interaction with B. cinerea. We determined that while all the mutants have an increased permeability, only the cutin mutants were resistant to this pathogen. Additionally, in order to identify the molecular elements that lead to this resistance or susceptibility, we performed a genome-wide transcriptional characterization before and after the challenge with the fungus. This analysis allowed us to identify a set of genes, expressed only in mutants altered in cutin content, that have recently been described as part of resistance mechanisms against B. cinerea (Lionetti et al., 2017; Bacete et al., 2018; Del Corpo et al., 2020). Our study allows us to understand how modification in cuticular components activates defense responses against this agronomical important phytopathogen.
Materials and Methods
Plant Material and Growth Conditions
Arabidopsis thaliana plants were grown in a greenhouse at 22 to 23°C and 60% humidity under a long day photo period (16-h light) for 4 weeks. The following plants were used: C24 ecotype as wild-type (WT) for the eca2 mutant altered in both cutin and wax components (Salinas-Mondragón et al., 1999; Serrano and Guzmán, 2004; Blanc et al., 2018) and Columbia-0 (Col-0) as WT for mutants altered in cutin content: bdg (Kurdyukov et al., 2006; Voisin et al., 2009) and lacs2-3 (CS65776 (obtained from the Arabidopsis Biological Resource Center, ABRC) (Bessire et al., 2007). The mutants altered in wax content were cer1-4 (SALK_008544C) (Bourdenx et al., 2011) and cer3-6 (yre-1) (Rowland et al., 2007), and were kindly provided by Professor Ljerka Kunst, Department of Botany, University of British Columbia, Vancouver, BC, Canada. All the selected mutants have been genetically (confirmed homozygous lines) and chemically characterized in detail (Supplementary Table 1).
Pathogen Infection Assays
Botrytis cinerea strain B05.10 was cultured on potato dextrose agar (PDA, 39 g L–1) plates. Spores were harvested in distilled water and filtered to remove hyphae. For inoculations, spore concentration was adjusted to 5 × 104 spores ml–1 in 1/4 -strength potato dextrose broth (PDB, 6 g L–1; Sigma-Aldrich, United States). For the analysis of lesion development, six fully expanded leaves per 4-week-old soil-grown plant were inoculated with a single drop of 6 μl of a spore suspension over each leaf, and at least 30 lesions were evaluated in each experiment. The inoculated plants were covered with plastic lids to maintain moisture level and transferred to a growth chamber at 22°C and a 24-h dark cycle. After 72 hpi, symptoms were evaluated. The level of resistance (disease incidence) was expressed by the percentage of plants showing disease symptoms extending beyond the inoculation site in each mutant. The developed lesions were quantified using the Image J analysis software (Fiji Is Just Image J1) (Schindelin et al., 2012). The experiments were repeated with at least three individual biological replicates, each with 10 technical replicates.
Cuticular Permeability Assays
The toluidine blue staining performed was from a previously described method (Tanaka et al., 2004; Bessire et al., 2007). Rosette leaves of 4-week-old plants were detached and immersed for 2 h in 0.025% TB (Sigma-Aldrich, United States) solution in 1/4 PDB (Sigma-Aldrich, United States) and were rinsed with tap water. Photos were used to measure the stained area (mm2) using imageJ see text footnote 1. For staining with calcofluor white (Sigma-Aldrich, United States), the leaves were bleached in absolute ethanol overnight, incubated in 0.2 M sodium phosphate buffer (pH 9) for 1 h, and for 5 min in 0.5% calcofluor white in 0.2 M sodium phosphate buffer (pH 9). Then, the leaves were rinsed in sodium phosphate buffer to remove excess calcofluor solution, and photographed under UV light (L’Haridon et al., 2011). Chlorophyll leakage from the rosette leaves was determined by a previously described protocol (Schnurr et al., 2004). For chlorophyll measurement, the fresh weight of detached leaves of mutants and wild-type plants was measured, and they were immersed in 80% ethanol. After 1 h, 1-ml aliquots were removed, and absorbance was measured at 664 and 647 nm. The micromolar concentration of total chlorophyll per gram of fresh weight of leaves was calculated using the equation: total micromoles chlorophyll = 7.93 (A664) + 19.53 (A647) (Lolle et al., 1997; Voisin et al., 2009). The experiments were repeated with at least three biological replicates, each with six technical replicates.
Detection of Reactive Oxygen Species
3, 3′-Diaminobenzidine and NBT staining were performed to determine the presence of hydrogen peroxide (H2O2) and superoxide (O2–), respectively. The presence of H2O2 was visualized with 3, 3′-diaminobenzidine (Sigma-Aldrich, United States) (Thordal-Christensen et al., 1997; L’Haridon et al., 2011). Detached leaves were immersed in 1 mg ml–1 DAB-HCl, pH 3.8, by gentle vacuum infiltration. For superoxide (O2–) staining, detached leaves were immersed for 30 min in 0.1% nitroblue tetrazolium (NBT) chloride (Sigma-Aldrich, United States) in 50 mM potassium phosphate buffer pH 7.5 (L’Haridon et al., 2011; Lehmann et al., 2015). Following incubation, the DAB and NBT staining solutions were removed and replaced with a bleaching solution (ethanol: acetic acid: = 3:1). H2O2 was visualized as a reddish-brown stain formed by the reaction of DAB with endogenous H2O2. The O2– content was detected as a dark blue stain of a formazan compound formed as a result of NBT reacting with endogenous O2–. The reactive oxygen species (ROS) production in detached rosette leaves unchallenged and challenged with the pathogen was detected using 5-(6) carboxy-2′, 7′-dichlorofluorescein diacetate (DCF-DA; Sigma-Aldrich, United States). The leaves were immersed in 60 μM of DCF-DA in a standard medium (1 mM KCl, 1 mM MgCl2, 1 mM CaCl2, 5 mM 2-morpholinoethanesulfonic acid adjusted to pH 6.1 with NaOH) (L’Haridon et al., 2011; Benikhlef et al., 2013). The leaves were then observed using a Carl Zeiss Axioplan 2 epifluorescence microscope with a GFP filter set (excitation 480/40 nm, emission 527/30 nm). Microscope images were saved as TIFF files, and the accumulation of fluorescence was quantified as pixels using imageJ see text footnote 1.
RNA Extraction, RNA Sequencing, and Analysis
For RNA-seq, rosette leaves of the Col-0, C24, eca2, bdg, lacs2-3, cer1-4, and cer3-6 plants were inoculated by spraying the entire leaves with spore suspension (5 × 104 spores ml–1) of B. cinerea. At least eight whole rosettes of each plant were collected at 6 hpi and as well under non-infected conditions. Total RNA for RNA-seq was isolated from two different biological replicates for each mutant and WT using a SpectrumTM Total RNA kit (Sigma-Aldrich, United States). Total RNA concentration and purity were measured using NanoDropTM 2000 (Thermo Fisher Scientific, Inc., Waltham, MA, United States). Library construction and sequencing were performed by Beijing Genomics Institute (BGI) Americas2 using DNBSeqTM technology. The sequencing was performed using paired end generating 100-bp size reading. The sequences are publicly available in the following link: https://dataview.ncbi.nlm.nih.gov/object/PRJNA761130?reviewer=jgq29l4gjk5tenf7e4q3755opm. Approximately 20 million reads per sample were aligned to the A. thaliana transcriptome (3 TAIR version 10) using Bowtie2 (v2.3.5) (Langmead and Salzberg, 2012). The bioinformatics data processing summary for each mutant is shown in Supplementary Table 2. We calculated gene expression levels using the RNA-seq by expectation maximization (RSEM) method (v1.3.3) (Li and Dewey, 2011). Differentially expressed genes (DEGs) were identified using the software DESeq2 in the Integrated Differential Expression Analysis MultiEXperiment (IDEAMEX) (Jiménez-Jacinto et al., 2019), with a FoldChange ≥ 2, and adjusted p-value ≤ 0.05. Additionally, the DEGs were functionally annotated with Gene Ontology (GO) terms extracted with PANTHER (v16.0) (GO term enrichment analysis) and Database for Annotation, Visualization, and Integrated Discovery (DAVID) (v6.8), by using Fisher’s exact test and correction with an FDR. Plots were created with the ggplot2 library using RStudio (v1.4.1106). To further identify DEGs common among the cutin and wax mutants, we drew Venn diagrams using the VennDiagram package in R (v4.0.3), and the webtool Venn Diagram4. Figures showing heatmaps were generated using the webtool Heatmapper5 (Babicki et al., 2016).
Quantitative RT-PCR Analysis
Total RNA was isolated from the frozen rosette leaves of WT and the mutants infected and non-infected with B. cinerea (6 hpi) collected directly into liquid nitrogen and stored at −80°C. Wet weight of 100 mg was used for RNA isolation from a pool of leaves from six plants of each genotype using TRI Reagent® (Sigma-Aldrich, United States), following the instructions of the manufacturer. Sample quality was assessed by using denaturing gel electrophoresis and measured using NanoDrop 1000 Spectrophotometer (Thermo Fisher Scientific, Inc., Waltham, MA, United States). A 1-μg sample of total RNA was treated with DNase I, RNAse-free (Thermo Fisher Scientific, Inc., Waltham, MA, United States), and then used as the template for cDNA synthesis with a RevertAid H Minus RevertAid First Strand cDNA Synthesis kit (Thermo Fisher Scientific, Inc., Waltham, MA, United States). Quantitative RT-PCR (RT-qPCR) reactions contained cDNA (diluted 1/40) in Maxima SYBR Green/ROX qPCR Master Mix (2×) (Thermo Fisher Scientific, Inc., Waltham, MA, United States) and 0.5 μM of specific primers. Primers for the RT-qPCR gene expression analysis have been previously described: AtPME17 (Del Corpo et al., 2020), AtPME41 (Choi B. et al., 2017), RAP2.6/ERF108 (Imran et al., 2018), and CAT3 (Zou et al., 2015). All the reactions were performed in 96-well plates using the 7300 Real-Time PCR System and 7300 System Software (Applied Biosystems, Foster City, CA, United States). PCR conditions were 95°C initial denaturations for 15 min, 40 cycles of 15 s/95°C, 30 s/60°C, and 30 s/72°C, after each run, a dissociation curve was acquired to check for amplification specificity by heating the samples from 60 to 95°C. The relative gene expression level for each sample was calculated using the comparative Ct method (Schmittgen and Livak, 2008) and normalized with the geometrical mean of two housekeeping genes, CF150 (AT1G72150) and ACT2 (AT3G18780) (Serrano and Guzmán, 2004; Czechowski et al., 2005). One-way ANOVA followed by Tukey comparisons was performed to evaluate the significance of the differential gene expression using the mean values from three biological replicates for each sample.
Statistical Analysis
One-way analysis of variance, followed by Tukey’s (honestly significant difference (HSD) comparisons, was performed to determine statistical significance. GraphPad Prism8 v 8.0.1 (GraphPad Software, San Diego CA, United States) was used. Data represent the mean ± SE. Differences at p < 0.001 were considered significant.
Results
Mutants With Alteration in Cuticular Wax or Cutin Composition Confer Differential Resistance to Botrytis cinerea
In recent years, a number of Arabidopsis mutants with defects in different steps of cutin biosynthesis, transgenic plants expressing a fungal cutinase and/or direct application of cutinase on wild type rosette leaves, have shown resistance to B. cinerea. These results has been interpreted as evidence of the participation of the cutin monomers in the resistance against this pathogen (Sieber et al., 2000; Chassot et al., 2007; Voisin et al., 2009). Despite the previous data from several mutants involved in the synthesis and regulation of cuticular waxes, in interaction with pathogens (Bourdenx et al., 2011; Lee et al., 2016; Ju et al., 2017), the link between wax composition/structure and the resistance or susceptibility of mutants against B. cinerea have not been described in detail. In addition, molecular defense mechanisms underlying the phenotypes of both the wax and cutin mutant lines mainly affected in cuticular biosynthesis are still unknown. To determine if changes in wax composition lead to resistance to this phytopathogen, we confronted the eca2 mutant (with modified cutin and wax components), cutin mutants lacs2-3 and bdg, and two mutants with reduced wax content, cer1-4 and cer3-6, with B. cinerea and compared them with their corresponding wild-type plants. After inoculation (3 dpi), we observed that only eca2, bdg, and lacs2-3 showed resistance to this pathogen (Figure 1A). These cutin-altered mutants exhibited less than 20 and 35% of disease incidence, respectively, while their corresponding WT plants showed an incidence of 100% (Figure 1B). However, in the cer1-4 and cer3-6 mutants affected only in wax composition, susceptibility similar to that of Col-0 was observed (Figures 1A,B). Additionally, the lesion average area was significantly smaller in the cutin mutants eca2,bdg, and lacs2-3 compared with the wax mutants cer1-4 and cer3-6 (Figure 1C). One interesting observation is that under our experimental conditions, the majority of leaves from eca2 remained free of disease symptoms 7 dpi compared with their corresponding WT plants (C24), whereas only some of the leaves from bdg and lacs2-3 remained free of disease symptoms at this time. In contrast, all the inoculated leaves of cer1-4 and cer3-6 already showed signs of fungal infection at 3 dpi, similar to the WT plants (data not shown). These results indicated that an altered wax composition does not correlate with resistance against the necrotrophic fungi B. cinerea, as previously observed in cutin mutants.
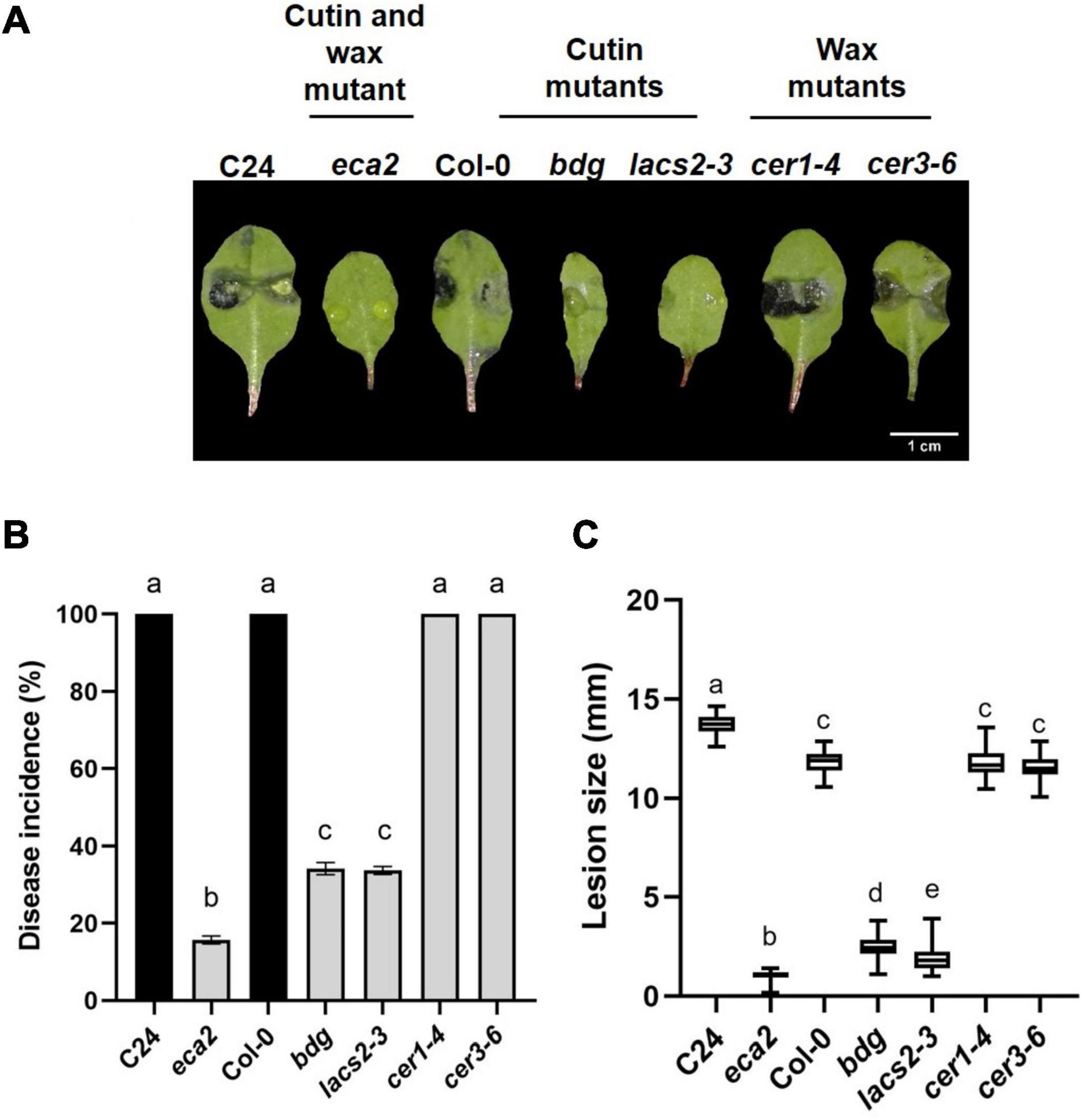
Figure 1. Differential phenotypic response of cuticular mutants to B. cinerea. Leaves of WT plants C24 and Col-0 and the cuticular mutants eca2, bdg, lacs2-3, cer1-4, and cer3-6 were infected with B. cinerea. Disease symptoms on rosette leaves after 72 hpi (A), disease incidence (B), and lesion size (C) were evaluated. Three independent experiments were done (n = 60 ±SD). Different lowercase letter columns indicate significant difference, according to one-way analysis of variance (ANOVA) (p-value < 0.001) followed by Tukey test. Representative pictures are shown.
Changes in Cutin or Wax Content Lead to Increased Leaf Permeability
Previous reports on the bdg, lacs2-3, and eca2 mutants have shown that they have a permeable cuticle (Bessire et al., 2007; Chassot et al., 2007; Voisin et al., 2009; L’Haridon et al., 2011; Blanc et al., 2018). To determine if the wax mutants present similar permeability, we assessed it by toluidine blue (TB) and calcofluor staining and measuring the increased efflux of chlorophyll, as previously described (Tanaka et al., 2004; L’Haridon et al., 2011; Cui et al., 2016). The eca2, bdg, and lacs2-3 leaves showed the characteristic dark blue and bright patterns correlated with the TB and calcofluor stain, respectively, while cer1-4 and cer3-6 had weaker calcofluor staining and smaller TB-stained area compared with the cutin mutants (Figures 2A,B). Nevertheless, the quantification of the TB-stained area shows that all the mutants (such as cer1-4 and cer3-6) present statistically significant increased permeability compared with their corresponding WT plants (Figure 2B). Next, we analyzed cuticular permeability by chlorophyll leaching. When non-infected rosette leaves are immersed in 80% ethanol, mutants defective either in cutin and/or wax composition lose chlorophyll more rapidly than their corresponding WT plants (Figure 2C). We observed that the cutin mutants lacs2-3 and eca2 had similar chlorophyll-leaching rates of approximately 4-fold more than their corresponding WT plants. The bdg, cer1-4 and cer3-6 mutants show 3- and 2-fold greater chlorophyll leaching, respectively, compared with Col-0 (Figure 2C), thus corroborating the results of the toluidine blue and calcofluor tests. Taken together, these results indicate that modification of the content of cutin and/or wax leads to a more permeable cuticle.
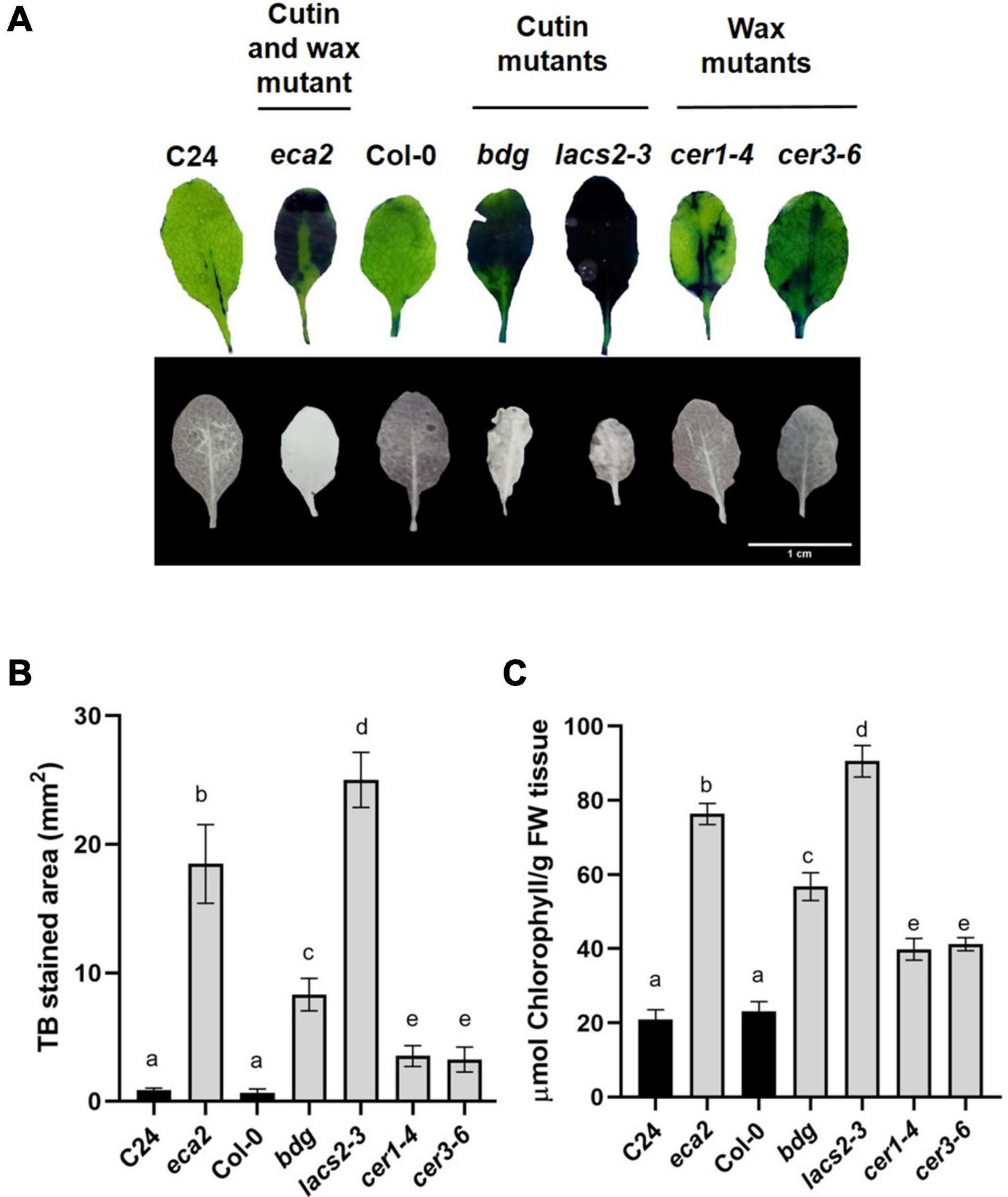
Figure 2. Cuticular mutants present increased leaf permeability. (A) Upper panel: leaves of 4-week-old WT plants and the cuticular mutants were stained with toluidine blue. Lower panel: leaves were stained with calcofluor white and viewed under UV light. (B) Toluidine blue-stained areas were quantified using the Fiji software. (C) Chlorophyll leaching 60 min after immersion of the leaves on 80% EtOH was spectrophotometrically measured as previously described (L’Haridon et al., 2011). Different lowercase letter columns indicate significant differences according to one-way analysis of variance (ANOVA) (p-value < 0.001) followed by Tukey’s test. Representative pictures are shown.
Cuticle-Related Mutants Show Basal Reactive Oxygen Species Accumulation
Previous reports on Arabidopsis mutants with defects in cuticle structure associated with alterations in the composition of cutin monomers showed increased reactive oxygen species (ROS) levels, even when leaves were not challenged with a pathogen (Chassot et al., 2007; L’Haridon et al., 2011; Blanc et al., 2018). To test if the production of ROS, one of the most early and rapid defense reactions to pathogen attack, is present in mutants with alterations in the composition of cuticular wax, we evaluated the accumulation of ROS in uninfected leaves of eca2, bdg, lacs2-3, cer1-4, and cer3-6 using three different dyes: 5-(and-6)-carboxy-2,7-dichlorodihydrofluorescein diacetate (DCF-DA) that detects a broad range of oxidizing reagents (L’Haridon et al., 2011); 3, 3′-diaminobenzidine (DAB), which detects H2O2; and nitroblue tetrazolium (NBT), which detects O2–(Thordal-Christensen et al., 1997; Mengiste et al., 2003). Stronger DCF-DA fluorescence was observed in eca2, bdg, and lacs2-3 compared with the mutants with altered wax content, cer1-4 and cer3-6 (Figure 3A). Nevertheless, ROS accumulation in the latter mutants was stronger than in Col-0 (Figure 3A). The DAB and NBT staining showed that coloration in the mutants with altered cutin (eca2, bdg, and lacs2) was much darker than in cer1-4 and cer3-6, indicating higher ROS accumulation (Figure 3B). To further study this immune response, we analyzed ROS accumulation in leaves at 6 hpi with B. cinerea. We observed that all the mutants, such as cer1-4 and cer3-6, showed stronger accumulation than their corresponding WT plants in all the staining methodologies (DCF-DA, DAB, and NBT) (Supplementary Figure 1). At this point, our data illustrate a possible correlation between cuticle permeability, ROS production, and B. cinerea resistance associated with alterations in the composition of cutin monomers, as previously described. However, although cuticle permeability and ROS accumulation are observed in the wax mutants, their susceptibility suggests that these changes might not only be associated with the resistance against B. cinerea, as we have previously hypothesized.
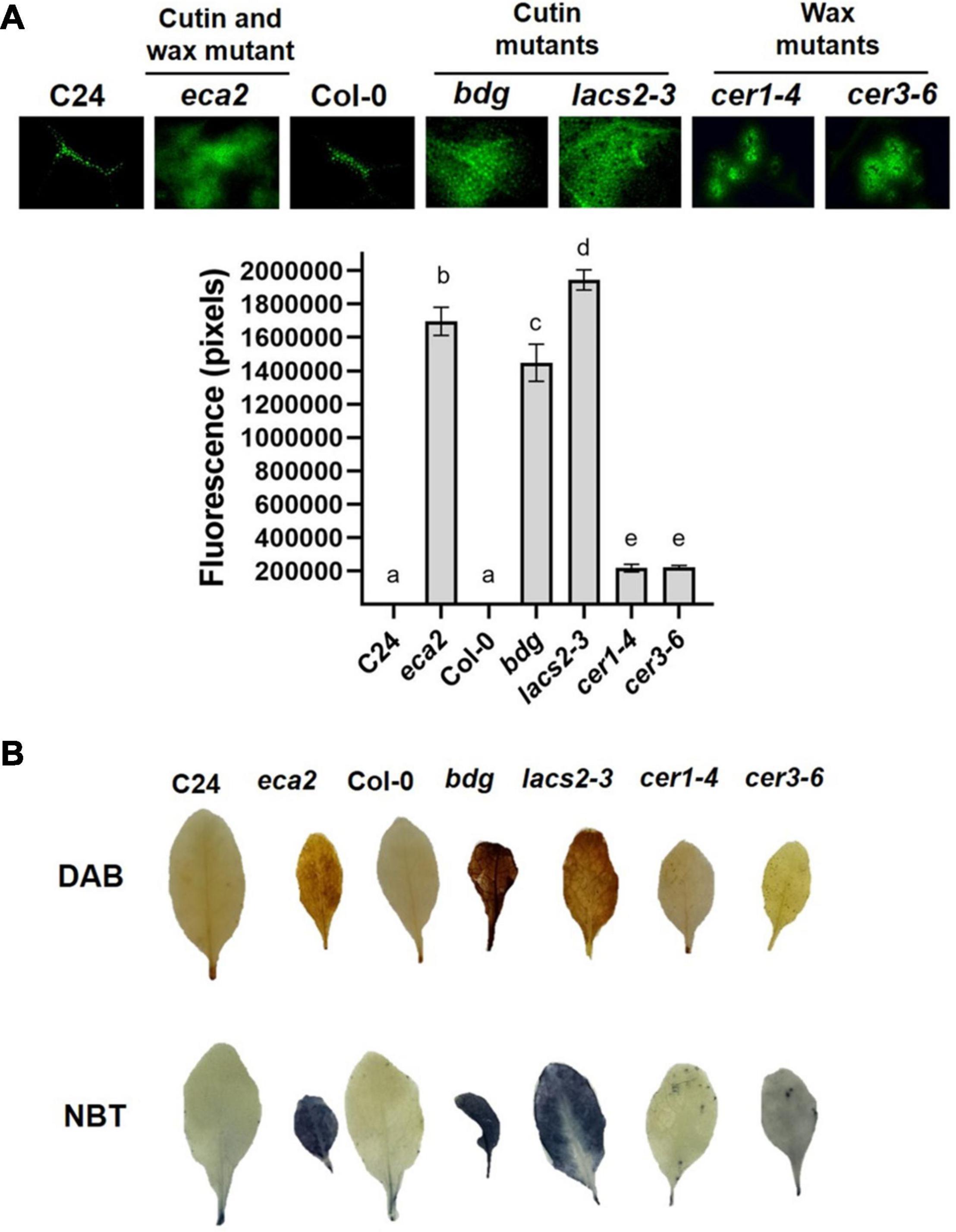
Figure 3. Reactive oxygen species (ROS) accumulation in the cuticular mutants and WT plants. (A) DCF-DA staining was observed on leaves by using epifluorescence microscopy. (B) 3, 3′-diaminobenzidine (DAB) and nitroblue tetrazolium (NBT) were used to detect the accumulation of hydrogen peroxide (H2O2) and superoxide (O2–), respectively. Six independent experiments were carried out with similar results (n = 6 ± SD). Scale bar = 100 μm. Different lowercase letter columns indicate significant differences, according to one-way analysis of variance (ANOVA) (p-value < 0.001) followed by Tukey’s test. Representative pictures are shown.
Reduction in Cutin and Wax Contents Induced Differential Transcriptional Changes
To investigate the molecular basis that might contribute to the differential response against B. cinerea among the cuticular mutants, RNA transcriptome sequencing (RNA-seq) and analysis were performed on non-infected plants. We identified that the number of differentially expressed genes (DEGs) was different in each mutant, compared with their corresponding WT plant, as follows: 2,386 in eca2, 3,506 in bdg, 1,688 in lacs2-3, 2,819 in cer1-4, and 611 in cer3-6 (Figure 4A and Supplementary Data 1). Additionally, we studied if these DEGs were shared among the mutants (Figure 4B). Interestingly, a large proportion of the DEGs (up- and down-regulated genes) was unique in most of the mutants, except for lacs2-3 and cer3-6. For instance, 68, 51, and 69% of the DEGs were only detected in eca2, bdg, and cer1-4, respectively, while 35 and 23% of the DEGs were only identified in lacs2-3 and cer3-6 (Figure 4B). We also looked at DEGs shared only in B. cinerea-resistant or susceptible mutants (Supplementary Data 2). The Gene Ontology (GO) analysis of these DEGs from the cutin and wax mutants showed substantial differences. Among the 36 induced genes identified in the three cutin mutants, there is enrichment in the transmembrane receptor protein tyrosine kinase signaling pathway, wax biosynthetic process, and cuticle development. Among the 29 induced genes in the wax mutants, there is enrichment in the regulation of response to stress, regulation of response to external stimulus, and developmental process involved in reproduction (Supplementary Data 3). On the other hand, for 67 genes that are downregulated in the wax mutants, the DEG biological process categories were associated with photosynthesis and response to abiotic stress, while the 33 downregulated genes identified in the cutin mutants were classified as regulation of metabolic process (Supplementary Data 3). Finally, we identified that only two genes were upregulated in all five mutants. They were GRP3 (AT2G05520), which encodes an Arabidopsis Glycine Rich Protein, and the AT3G14470, which encodes an NB-ARC domain-containing disease resistance protein. Similarly, only two genes were downregulated in all the five mutants: CBF3 (AT4G25480), which encodes a member of the ERF/AP2 transcription factor family, and a gene that encodes a xyloglucan endotransglycosylase/hydrolase (AT3G44990) (Figure 4B). These results suggest a differential transcriptional regulation in all the cuticular mutants.
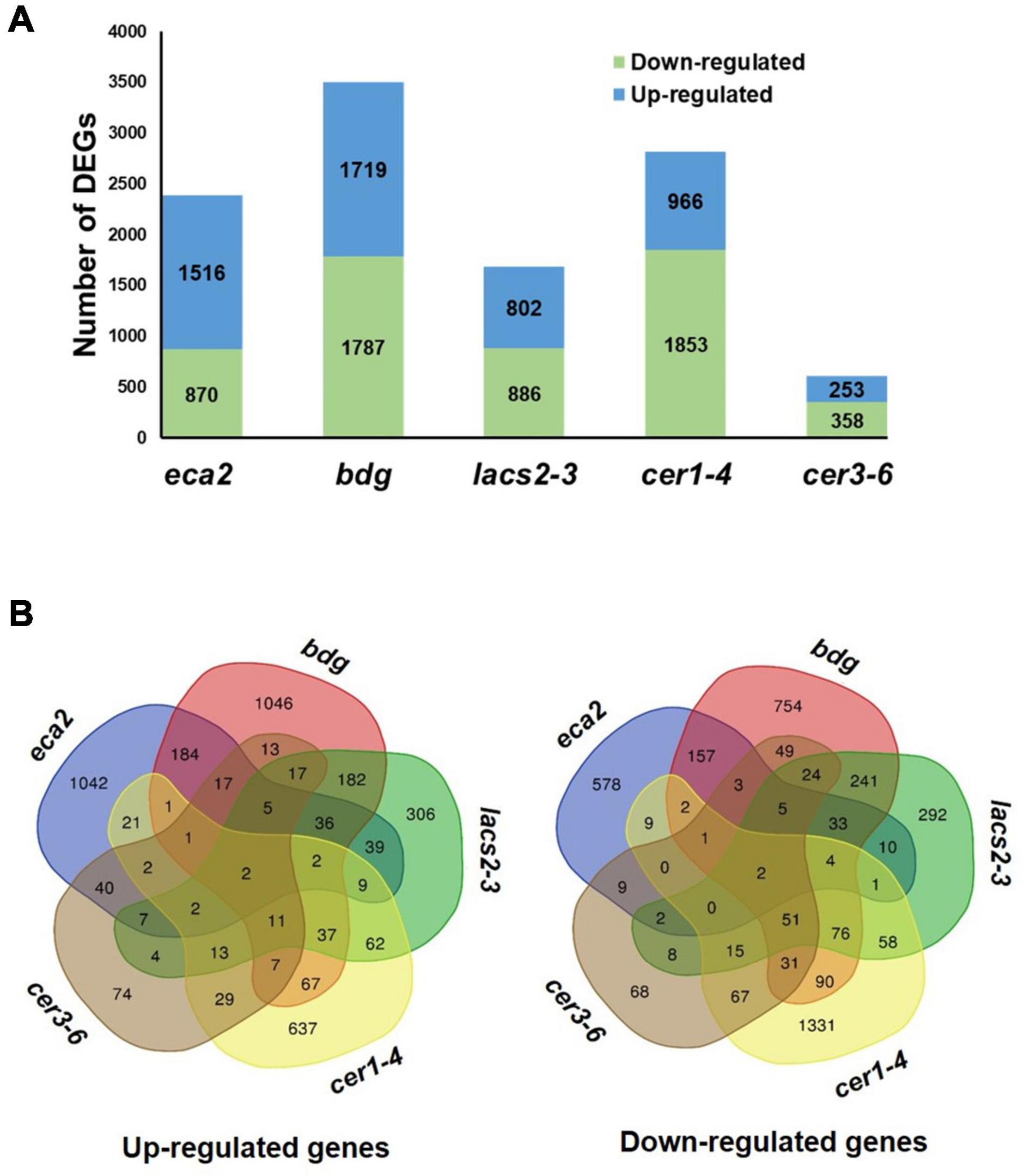
Figure 4. Transcriptome analysis of non-infected cuticular mutants. (A) Number of differentially expressed genes (DEGs) (Log2FC ≥ 1 or ≤ –1 with an adjusted p-value ≤ 0.05) identified in the cutin and wax mutants. (B) Venn diagrams show the overlap of upregulated and downregulated genes among the cutin or wax mutants.
Under Non-challenged Conditions, Cutin Mutants Transcriptionally Induced Differential Defense Responses
A transcriptional modification of plant defense response genes in non-infected cutin mutants has been previously described (Voisin et al., 2009; Nawrath et al., 2013). However, to our knowledge, it has not been shown in wax mutants. The GO analysis on the identified DEGs for each mutant reveals that only eca2, bdg, and lacs2-3 show the modification of expression of genes classified as part of the response to biotic stresses (Figure 5 and Supplementary Data 4). These GO processes included: response to biotic stimulus and response to other organisms, involved in interspecies interaction between organisms and defense responses, while in the mutants cer1-4 and cer3-6, statistically significant GO processes were classified into the response to abiotic stresses (Figure 5 and Supplementary Data 4). In order to further characterize these results in cutin mutants, we analyzed the transcriptome profile of selected marker genes related to the jasmonic acid/ethylene- (JA/ET), salicylic acid (SA), and abscisic acid (ABA) pathways that have been described to be induced during the interaction with this necrotrophic pathogen (AbuQamar et al., 2006; Windram et al., 2012) (Supplementary Figure 2, Supplementary Data 1). Interestingly, under non-infected conditions, most of these genes were actually downregulated and only a few were induced. These results suggest that while the basal transcriptomic response to biotic stresses is activated in the cutin mutants, canonical defense responses against this pathogen are not induced before the interaction occurs.
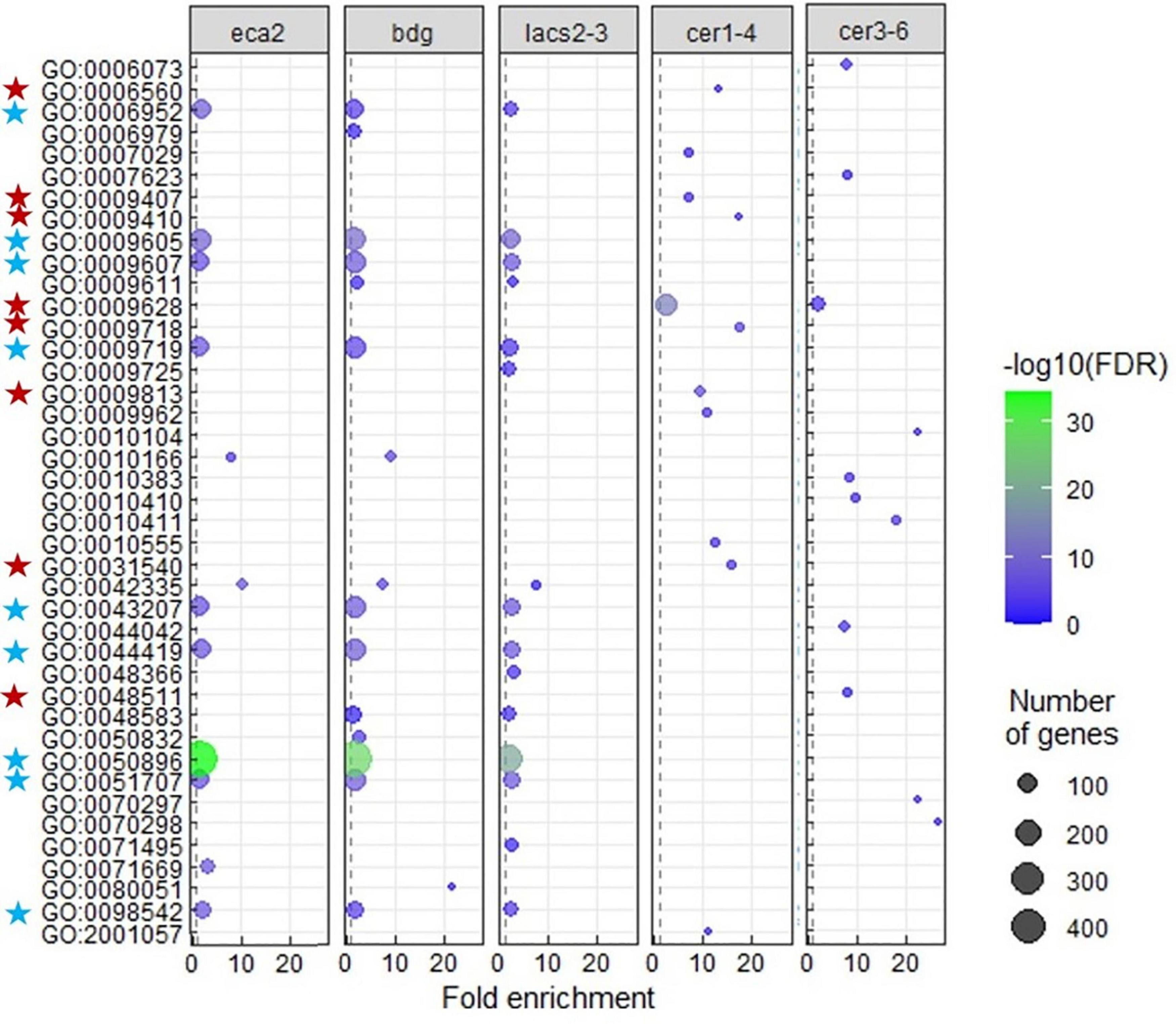
Figure 5. Gene ontology (GO) enrichment among upregulated genes in each cutin or wax mutant. Terms that were largely redundant were removed. Enrichment of “Biological process” GO-terms (FDR < 0.01). The color of the circles indicates the significance of the term (–log FDR), and dot size indicates the number of genes that are associated with that term. The blue stars indicate the GO terms common and mainly related to response to biotic stress, and the red stars indicate the GO terms related to response to abiotic stress, in the cutin and wax mutants, respectively.
Plant Defense Response Genes Are Induced Only in Cutin Mutants During the Interaction With the Pathogen
To further study the modification of the plant transcriptome in cutin and wax mutants, we performed RNA-seq analysis of B. cinerea-infected Arabidopsis leaves at 6 hpi. Interestingly, we observed a clear difference in the number of genes that are induced or repressed in the cutin mutants compared with the wax mutants. For instance, the number of DEGs in eca2, bdg, and lacs2-3 was 2,595, 4,823, and 4,865; while in cer1-4 and cer3-6, the number was only 241 and 411, respectively (Figure 6A, Supplementary Data 5). This represents approximately 10- to 8-fold more DEGs in the cutin mutants than in the mutants with reduced content of wax. To determine the processes that are transcriptionally induced, we performed a GO analysis of upregulated genes (Figure 6C). This analysis reveals a clear enrichment of GO terms related to response to a biotic stimulus only in the mutants with a reduced level of cutin. These terms include the biological process involved in interspecies interaction between organisms, defense response, response to biotic stimulus, response to fungus, and defense response to other organisms, among others (Figure 6C, Supplementary Data 6), while the GO analysis of upregulated genes in cer1-4 and cer3-6 reveals enrichment in response to abiotic stimuli, such as response to an organic substance, cytokinin-activated signaling pathway, response to chemical, and response to stimulus (Figure 6C, Supplementary Data 7). Additionally, we found that 214 and 11 DEGs were commonly induced in the cutin and wax mutants, respectively (Figure 6B, Supplementary Data 7). Additionally, 240 common downregulated genes were identified in eca2, bdg, and lacs2-3 (Figure 6B, Supplementary Data 7), which were classified into the following GO terms: response to salt stress, response to oxidative stress, response to water deprivation, and response to cold. The seven repressed genes common between cer3-6 and cer1-4 belong to response to stimulus, single organism process, and response to external stimulus (Supplementary Data 8). Taken together, these data indicate that the enhanced resistance against B. cinerea observed in eca2, bdg, and lac2-3 could be explained by the expression of defense-related genes, which are not induced in the wax mutants.
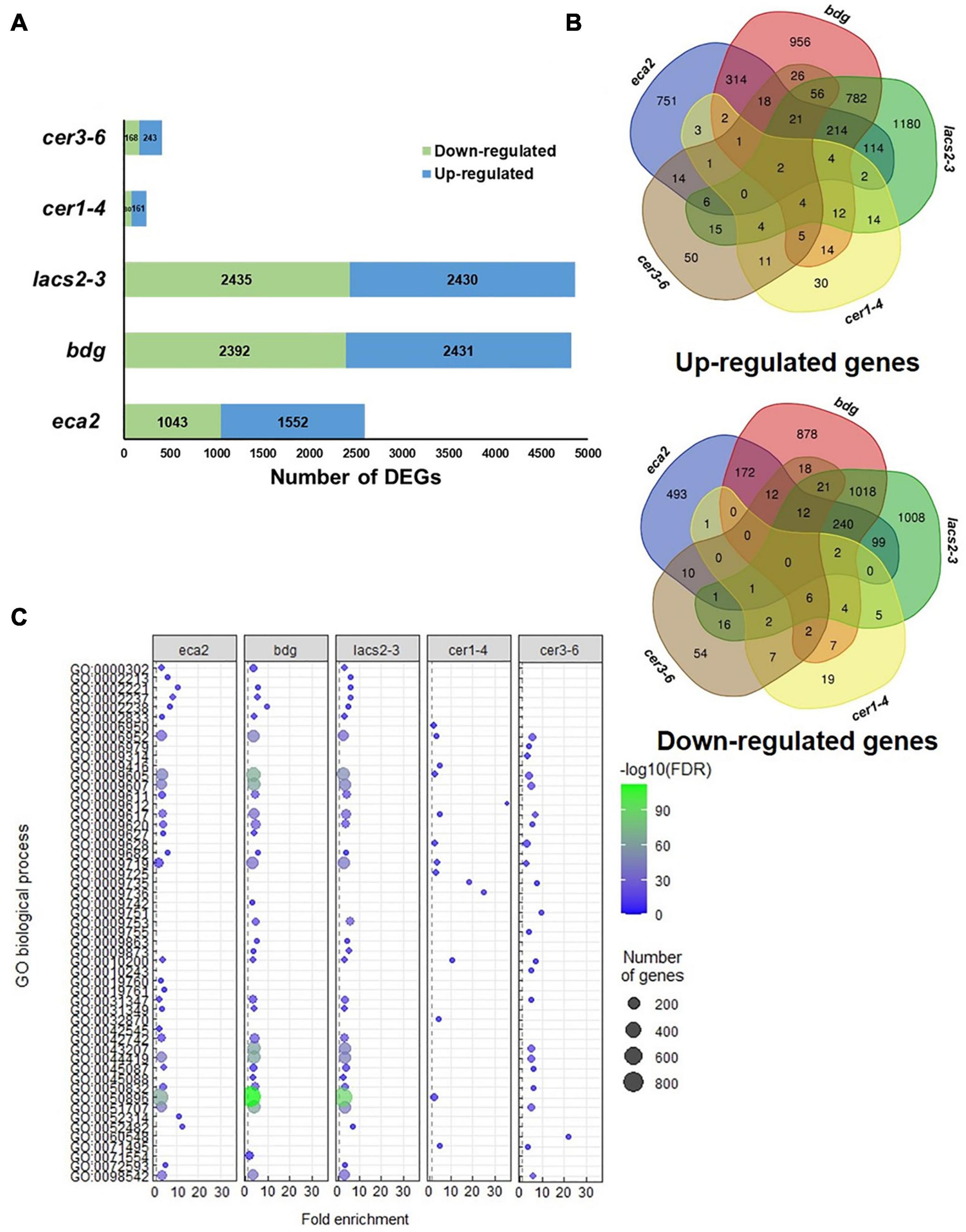
Figure 6. Transcriptome analysis of cuticular mutants infected with Botrytis cinerea (6 hpi). (A) Number of DEGs (Log2FC ≥ 1 or ≤ –1 with an adjusted p-value ≤ 0.05) identified in the cutin and wax mutants. (B) Venn diagrams show the overlap of upregulated and downregulated genes among the cutin or wax mutants. (C) GO analysis for upregulated genes in each cuticular mutant. Dot plot shows fold enrichment (FDR < 0.01) of the top 25 most significant enrichment terms of biological process.
Canonical Defense Response Genes Are Differentially Induced in Cutin Mutants
We further characterized the defense mechanisms in the mutants with reduced levels of cutin that might participate in the resistance against B. cinerea. We were interested in identifying if JA/ET-, SA-, ABA- and/or other defense-related genes were differentially expressed in the cutin and wax mutants (Figure 7). Remarkably, even though many of the hormone- and defense-related genes were induced in all the cutin mutants, we did not detect genes that were expressed simultaneously in all of them, except for the LRR receptor-like protein kinase (BAK1) and its interactor Botrytis-induced kinase 1 (BIK1), which are involved in the early stages of recognition of pathogens (Veronese et al., 2005; Liu et al., 2017; van der Burgh et al., 2019). This result suggests that resistance against B. cinerea might not be exclusively mediated by these well-described genes.
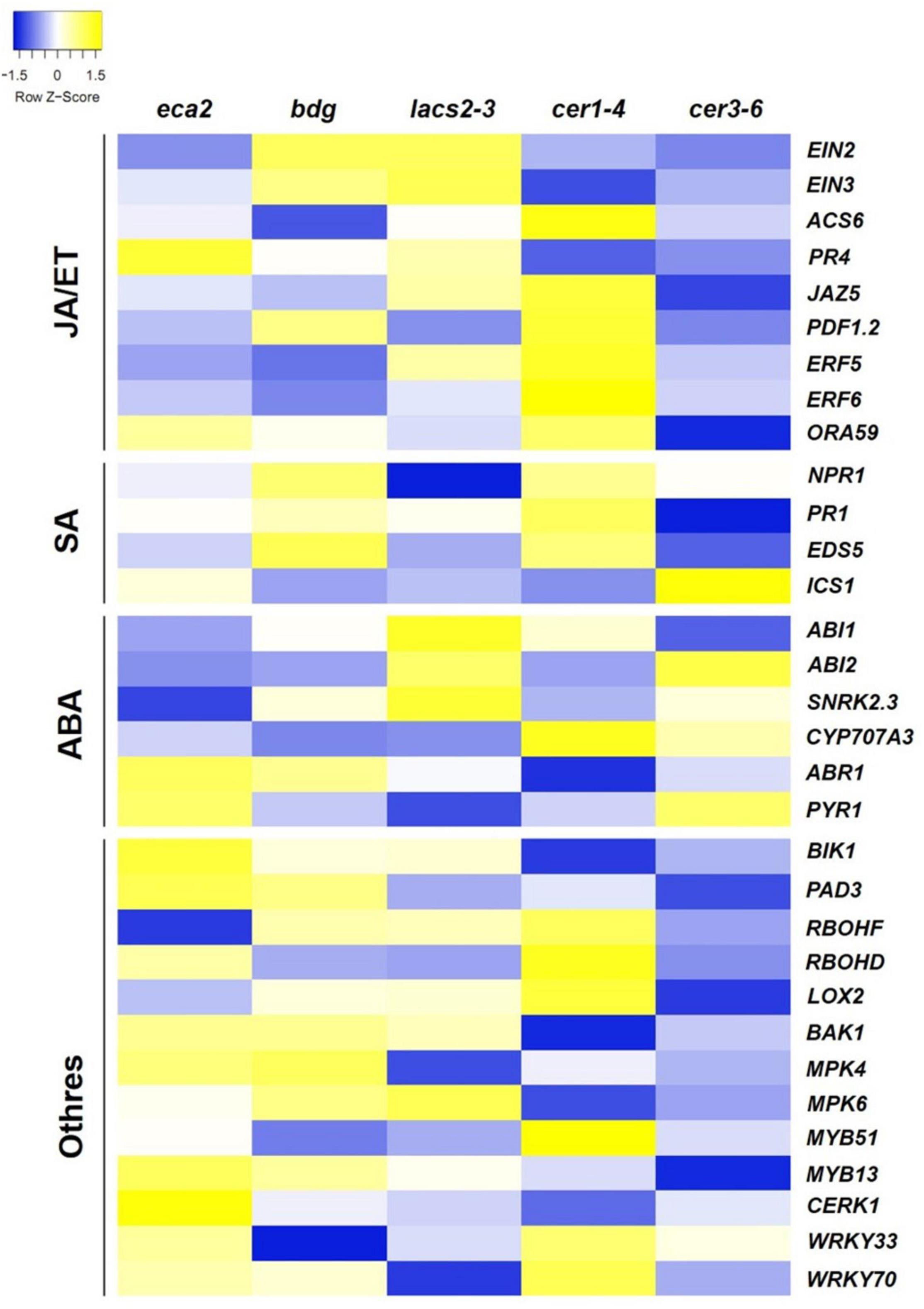
Figure 7. Expression pattern of the jasmonic acid (JA)/ethylene (ET), salicylic acid (SA), and abscisic acid (ABA) signaling pathways and other defense-related genes in response to B. cinerea (6 hpi). The colors of the heat map represent the z-score of each gene expression calculated between the expression of the mutant compared with its corresponding WT, ranging from blue (–1.5) to yellow (1.5).
A Set of Genes Related to Cell Wall Remodeling Is Induced in Mutants With Altered Cutin Monomer Content
Based on the expression profile of BAK1 and BIK1, we identified all the genes that are commonly upregulated among the three cutin resistant mutants, eca2, bdg, and lacs2-3, but downregulated in cer1-4 and cer3-6 (Figures 6B, 8A). We identified 214 genes that share this expression pattern (Supplementary Data 7). From this set of genes, we performed a GO analysis and the genes were classified into the response to biotic stimulus, response to fungus, innate immune response, and defense response to other organisms (Table 1). Interestingly, among these DEGs, we found genes that are involved in cell wall remodelings, such as AtPME17 (AT2G45220) and AtPME41 (AT4G02330), and encoding pectin methylesterases (PMEs), which have been recently identified in response to B. cinerea (Lionetti et al., 2012; Bellincampi et al., 2014; Bethke et al., 2014, 2016). Likewise, two members of the APETALA2/ETHYLENE RESPONSIVE FACTOR (AP2/ERF) family, transcription factors RAP2.6/ERF108 and RAP2.6L/ERF113, catalase CAT3, peroxidases AtPRX71 [recently identified in resistance to B. cinerea (Lorrai et al., 2021)], and Sugar Transporter Protein STP13, and genes related to pattern-recognition receptors (PRRs), such as RLK7, RLK5, and RLP30, were identified as DEGs (Figure 8A). In order to confirm these expression patterns and to validate our RNA-seq analysis, we analyzed the expression of AtPME17, AtPME41, RAP2.6/ERF108, and CAT3 by RT-qPCR (Figure 8B). As expected, we found that all these selected genes were induced 6 hpi in eca2, bdg, and lacs2-3, and that they were downregulated in cer1-4 and cer3-6 (Figure 8B).
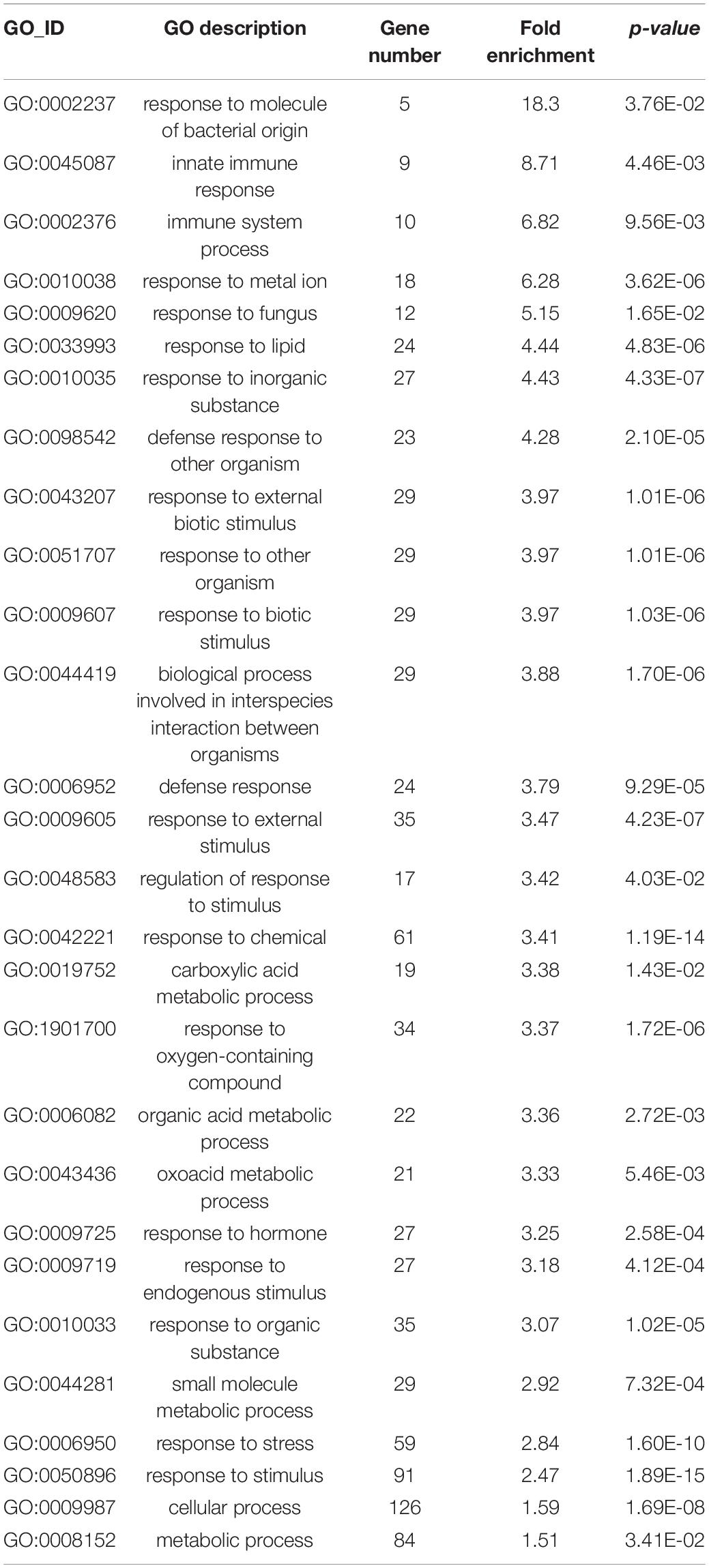
Table 1. GO enrichment analysis of Biological process of the 214 common upregulated genes in eca2, bdg and lacs2-3 infected with Botrytis cinerea (genes are listed in Supplementary Data 6).
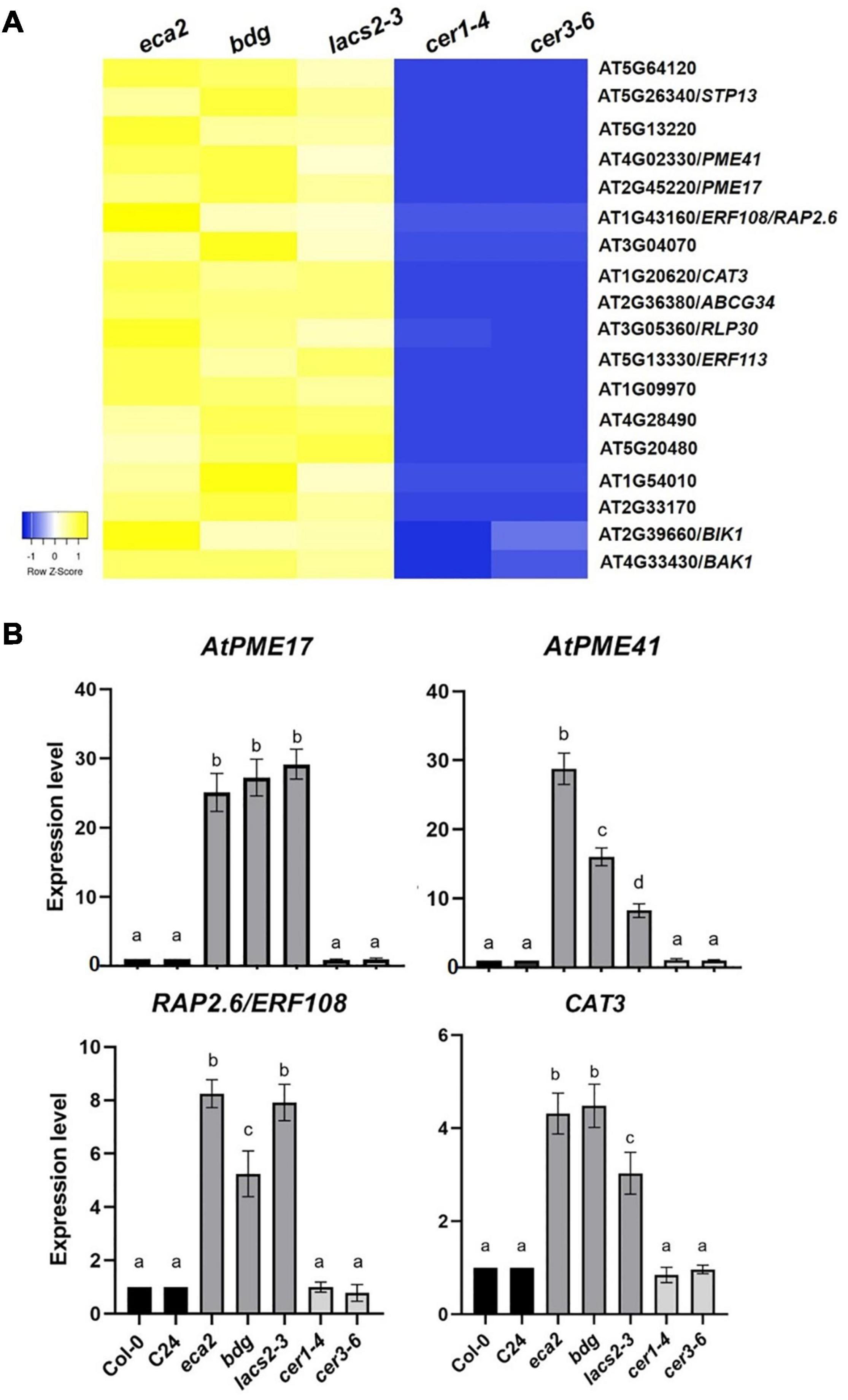
Figure 8. Commonly expressed genes induced in mutants with a reduced level of cutin monomers. (A) Heatmap shows the expression levels of upregulated genes shared by the cutin mutants in response to B. cinerea (6 hpi). The colors of the heat map represent the z-score of the log2FC of each gene ranging from blue (–1) to yellow (1). (B) Expression analysis of some shared up-regulated genes between cutin mutants infected with B. cinerea. Expression levels were determined by RT-qPCR in rosette leaves harvested 6 hpi, based on Ct values, normalized with the expression of the two housekeeping genes. The data represent the means of three independent biological replicates with their respective technical replicates, for each sample. Error bars indicate ±SD. Different letters indicate significance differences among samples according to one-way ANOVA followed by Tukey test (p ≤ 0.001).
Discussion
Plants have developed sophisticated responses, such as effects of abiotic and biotic stimuli, to survive in a challenging environment. One of these mechanisms includes preformed physical barriers on the plant surface, such as the cuticle (Chassot et al., 2007; Serrano et al., 2014; Aragón et al., 2017; Bacete et al., 2018; Engelsdorf et al., 2018; Molina et al., 2021). The cuticle, mainly formed by cutin and waxes, also serves as a source of signaling molecules that coordinate the dialog between plants and microorganisms (Aragón et al., 2017; Ziv et al., 2018). In A. thaliana and Solanum lycopersicum, changes in permeability, associated with modifications in cutin composition, have been linked to resistance against the necrotrophic fungus B. cinerea (Bessire et al., 2007; Chassot et al., 2007; Isaacson et al., 2009; Voisin et al., 2009; Martin et al., 2017; Blanc et al., 2018). This resistance has been attributed to faster recognition of pathogens due to a permeable cuticle, accumulation of ROS, and induction of plant defense responses. However, plants with reduced content of waxes, which also have a modified cuticle structure, have not been characterized in detail with respect to this phenotype. In this study, we determined that the wax mutants have a more permeable cuticle but are as susceptible as the wild-type plants. This is in line with the fact that not all cuticle mutants show resistance to B. cinerea. For instance, the cuticle mutants acp4 and gl1 showed susceptibility to both bacterial pathogens Pseudomonas syringae and B. cinerea (Xia et al., 2009, 2010; Benikhlef et al., 2013; Lim et al., 2020). Similarly, shn1, with cutin monomer content altered, has a more permeable cuticle but is more susceptible to three necrotrophic fungal pathogens, B. cinerea, S. sclerotiorum, and Alternaria brassicicola (Sela et al., 2013; Buxdorf et al., 2014). These results suggest that, as expected, cuticle structural integrity is an important physical barrier against multiple pathogens (such as B. cinerea), and that mutants sma4, lcr, bdg, lacs2, and eca2, which possess a low content of cutin, a permeable cuticle, and are resistant to B. cinerea, are exceptions to the rule. Additionally, our results indicate that faster recognition of the pathogen by changing cuticle permeability is not enough to induce plant innate immunity responses and resistance to B. cinerea, as previously hypothesized (Bessire et al., 2007; Serrano et al., 2014). Nevertheless, these cutin mutants can be used as models to investigate the molecular mechanisms behind the successful induction of defense responses against this agronomically important pathogen.
Plant development and responses to the environment are induced and regulated by signaling networks of “trio signaling” messengers: ROS, electrical signals, and calcium (Choi W.G. et al., 2017). In particular, ROS are induced by activating various oxidases and peroxidases in response to abiotic and biotic conditions (Torres and Dangl, 2005; O’Brien et al., 2012; Baxter et al., 2014; Saini et al., 2018). During host-pathogen interactions, the production of ROS is important for plants and for necrotrophic fungi, such as B. cinerea and S. sclerotiorum. For instance, one of the earliest defense responses to a pathogen attack is the so-called “oxidative burst,” the production of ROS at the site of invasion. A plant releases high amounts of reactive oxygen species (ROS) to counteract the pathogen. On the other hand, Botrytis cinerea also takes advantage of this plant defense to kill host cells before they are invaded by hyphae and is able to cope with external oxidative stress in order to survive in the necrotic tissue (Siegmund and Viefhues, 2016). We have described a direct relationship between the accumulation of ROS and resistance to B. cinerea after mechanical stimulus or by triggering defense responses through elicitors (Benikhlef et al., 2013; Narváez-Barragán et al., 2020; Batista-Oliveira et al., 2021). Additionally, the resistance against this pathogen observed in the cutin mutants has been also linked to increased levels of ROS under non-infected conditions (reviewed in Serrano et al., 2014). Here, we show that under non-challenged conditions, although all the mutants accumulated more ROS than the WT plants, the cutin mutants eca2, bdg, and lacs2-3 have higher levels of superoxide and hydrogen peroxide than the wax mutants cer1-4 and cer3-6 (Figure 3). Interestingly, at 6 hpi, all the cuticular mutants have higher levels of superoxide and hydrogen peroxide than the WT plants (Supplementary Figure 1). Despite the B. cinerea-induced ROS burst observed in the wax mutants, they showed susceptibility. Two possibilities can be explored to explain this phenotype based on ROS accumulation. First, since a moderate level of ROS is detected in the susceptible mutants cer1-4 and cer3-6, the level of ROS during the initial interaction with the pathogen could be important in triggering resistance against this pathogen. In agreement with this, it has been described that the inhibition of the size of lesions caused by B. cinerea is directly proportional to the level of ROS induced by soft mechanical stress (Benikhlef et al., 2013). On the other hand, during biotic interactions, ROS have been characterized to participate in multiple processes, such as reinforcement of the cell wall, regulation of hormone-induced signaling pathways, and triggering of hypersensitive response (Lehmann et al., 2015). Since we observed an increase of ROS in the wax mutants only after the interaction with the pathogen, it is possible that timing, not only the activation of the oxidative burst, is important to efficiently build up a defense response. Either way, our results suggest that besides ROS-dependent resistance, it is probable that other(s) mechanism(s) may be involved to trigger the plant response and, therefore, induce resistance against B. cinerea.
To further characterize the molecular mechanisms underlying the resistance or susceptibility in the cuticular mutants, we performed a transcriptomic analysis on the non-infected plants. Up-regulated genes identified in the cutin mutants were classified into the response to biotic stimulus, while GO terms in cer1-4 and cer3-6 were mainly associated with response to abiotic stimulus (Figure 5), suggesting that the identified DEGs in cutin mutants could be part of a primed defense response mechanism. These results are in line with previous reports, where a defense priming mechanism has been observed in plants treated with cutin monomers, as well as in other cuticular mutants, leading to expression of a suite of faster and stronger defense responses upon challenge with B. cinerea (Schweizer et al., 1996; Fauth et al., 1998; Bessire et al., 2007; Chassot et al., 2007; Conrath, 2011; Conrath et al., 2015; Mauch-Mani et al., 2017). Notably, our data also identified genes related to PRRs, highlighting the leucine-rich repeat receptor kinase (LRR-RK) (Figure 4). Previous studies have reported the importance of PRRs in the priming state. The plant receptor FLS2 and its co-receptor BAK1 were associated with the enhanced responsiveness of Arabidopsis plants to the bacterial flagellin peptide flg22 (Tateda et al., 2014), as well as the malectin-like LRR receptor-like kinase IOS1, which is associated with FLS2 and the bacterial receptor EF-Tu (Yeh et al., 2016). Taken together, our results suggest that before the interaction with the pathogen occurs, the cutin mutants are in a priming state compared with the wax mutants or WT plants and that once the infection takes place, they might respond in a faster and more efficient manner, stopping the infection.
Previous reports have characterized secondary responses transcriptionally induced during the interaction with B. cinerea. Among these defense responses, SA-, ET-, ABA- and JA-related pathways are induced (AbuQamar et al., 2006; Mengiste, 2012; Windram et al., 2012). To determine if a similar set of genes was present in the cutin and wax mutants, we performed a transcriptome analysis at 6 hpi. In cer1-4 and cer3-6 the defense responses are not induced, and most of the DEGs were classified into the response to abiotic stimulus, while in the cutin mutants, enrichment of defense-related genes was identified (Figure 6). However, in eca2, bdg, and lacs2-3, a similar profile of these defense marker genes, which could explain the resistance phenotype for all these mutants based on these hormone-induced responses, was not observed (Figure 7). From this set of genes, only two, involved in the early stages of recognition of pathogen, were identified to be induced in all the cutin mutants: the LRR receptor-like protein kinase (BAK1) and its interactor Botrytis-induced kinase 1 (BIK1). This result is in accordance with previous reports showing that the Arabidopsis mutant sma4 with a defective cuticle displayed increased resistance to B. cinerea, and that this process was independent of the JA and ET signaling pathways (Tang et al., 2007; Wang et al., 2020). Similarly, in CUTE plants, resistance to B. cinerea was not found to correlate with the induction of genes associated with the SA, ET, or JA signaling pathways (Chassot et al., 2007). In sum, these results suggest that the resistance to B. cinerea observed in eca2, bdg, and lacs2-3 might not only be driven by the induction of canonical defense responses, previously identified to be important in stopping the infection.
Since the stronger difference between cutin and wax mutants was resistance and susceptibility to B. cinerea, respectively, we hypothesized that a set of genes should be induced only in eca2, bdg, and lacs2-3 but repressed in cer1-4 and cer3-6 (Supplementary Data 7). Among these DEGs, we identified genes previously characterized to be involved in ROS regulation and cell wall biosynthesis, and are discussed next. The peroxidase (AtPRX71) and catalases (CAT3 andAT4G37530) genes were identified as DEGs in the cutin mutants. These genes have been described as part of ROS-scavenging systems to maintain ROS homeostasis in different compartments of the cell, and could, thus, restrict the ROS-dependent damage or finely coordinate the ROS-dependent signal transduction in the presence of a pathogen (Torres et al., 2006; O’Brien et al., 2012). However, necrotrophic pathogens also produce ROS to kill host cells triggering a hypersensitive reaction (HR), thereby facilitating the infection (Govrin and Levine, 2000; Torres et al., 2006; van Kan, 2006). Based on this evidence, it is possible that the expression of these scavengers might help either the plant to inhibit the proper ROS-induced infection process of B. cinerea, or regulate ROS-dependent plant defense responses. Clearly, this hypothesis should be tested in future studies.
Additionally, we identified the induction of pectin methylesterases (PMEs) AtPME41 and AtPME17 (Figure 8) in the cutin mutants in response to B. cinerea. Importantly, these genes have not been reported before as a part of the defense genes against B. cinerea in any cuticular mutants. Our data are in accordance with previous results showing that plants activate a local and strong PME activity in response to pathogens with different lifestyles (Lionetti et al., 2012; Bethke et al., 2014; Lionetti, 2015). Once the pathogen overcomes the cuticle, the pectin matrix, which is the major fraction of the cell wall of dicots and non-aminaceous monocots, is the next target for fungal necrotrophs (van Kan, 2006; Laluk and Mengiste, 2010; Lionetti et al., 2012; Bellincampi et al., 2014). PME activity was proposed to be involved in the release and perception of defense of endogenous signals with elicitor activities from cellular components during infection, such as oligogalacturonides (OGs) considered as DAMPs (Lionetti et al., 2012; Ferrari et al., 2013; De Lorenzo et al., 2019). AtPME41 synthesizes a member of PMEs that has an important role in activating the immune response when Arabidopsis is challenged with the necrotroph A. brassicicola (Lionetti et al., 2012; Bethke et al., 2014). Additionally, Del Corpo et al. demonstrated the functional role of AtPME17 in triggering PME activity by the JA/ET-dependent pathway and in resistance against pectinolytic necrotrophic fungi, such as B. cinerea (Del Corpo et al., 2020).
Finally, this study illustrates that B. cinerea resistance in the cutin mutants eca2, bdg, and lacs2-3, compared with cer1-4 and cer3-6, clearly consists of a multitude of signaling events, from pre-activated defense or priming as initial resistance, production of ROS, and increased expression of non-canonical defense-related genes (Figure 9).
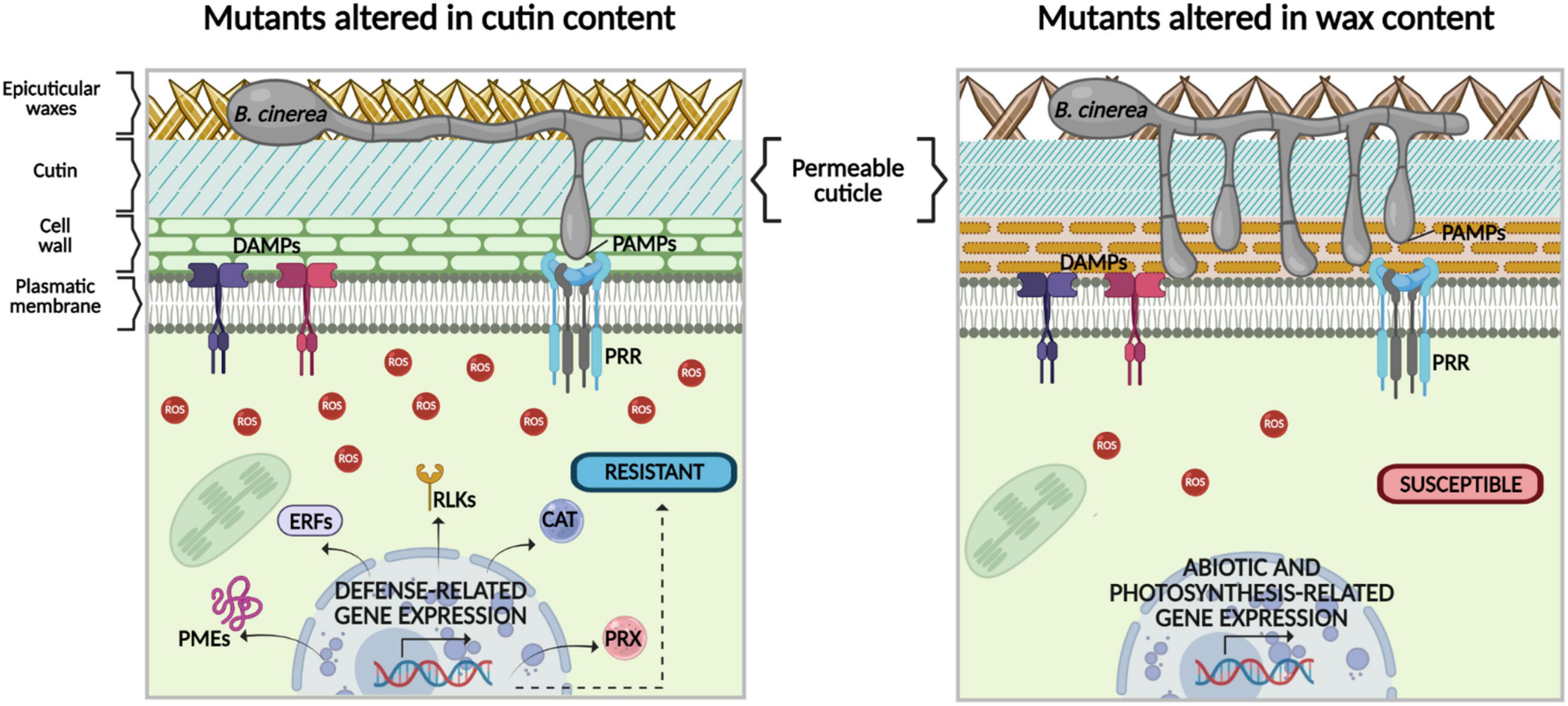
Figure 9. Hypothetical model of the role of cuticular components cutin and wax in differential response to the necrotrophic fungus B. cinerea. In previous studies, a direct relationship between increased permeability in cutin mutants and resistance to B. cinerea has been proposed. However, our data show that despite both cuticular mutants having enhanced cuticular permeability, compared with the WT plants, only mutants with altered cutin content show resistance to B. cinerea. This phenotype could be explained as follows: (1) in the cutin mutants, once plant plasma membrane-localized receptors recognize pathogen-/damage-associated molecular patterns (PAMPs/DAMPs) a more intense ROS production is observed, compared with mutants with altered wax content. (2) During cutin mutant-B. cinerea interaction, defense-related signaling pathways are triggered, which are different from canonical immune ones, such as expression genes encoding catalases (CAT3) and peroxidases (PRX), as well as LRR-receptor-like kinases (RLK5, RLK7, RLP30), transcription factors (ERF108 and ERF113), and genes involved in the remodeling of the cell wall, such as PMEs. (3) In contrast, according to our data, in the mutants with altered wax content, ROS production is not enough to effectively prevent B. cinerea colonization. In these mutants, other secondary signals might be activated to control the expression of genes related to abiotic stimuli, instead of the expression of defense-related genes (the image was designed using bioRENDER application, https://biorender.com/).
Conclusion
We have shown that while cer1-4 and cer3-6 have altered cuticle permeability, they present a susceptible phenotype, suggesting that the faster recognition of the pathogenic fungus B. cinerea is not enough to induce plant innate immune responses and resistance as previously hypothesized. In the cutin mutants eca2, bdg, and lacs2-3 responding to B. cinerea, a profile of previously characterized defense-response gene markers was not observed, suggesting that mutants with resistant phenotypes can activate other defense pathways, different from these canonical immune ones. Nevertheless, we identified the induction of genes involved in cell wall remodeling in the cutin mutants, which have not been previously reported as part of the defense genes against B. cinerea in any cuticular mutants. Based on these results, our study can be used as a starting point to understand the molecular basis involved in early defense mechanisms related to cuticular components against this agronomically important necrotrophic pathogen.
Data Availability Statement
The datasets presented in this study can be found in online repositories. The names of the repository/repositories and accession number(s) can be found below: NCBI BioProject; PRJNA761130.
Author Contributions
WA, DF, and MS conceived and designed the experiments. WA, DF, NA-B, and MT performed the experiments. WA, DF, and MS wrote and revised the manuscript. All authors contributed to the article and approved the submitted version.
Funding
This study was supported by funds from Dirección General de Asuntos del Personal Académico-UNAM (PAPIIT), grants IA202620 and IN203720 to DF and MS, respectively, as well as the Ciencias Básicas grant from CONACYT No. A1-S-16129 to DF and UC MEXUS-CONACYT Grant No. CN-10-109 to MS. WA is a doctoral student from Programa de Doctoradoen Ciencias Biomédicas, UNAM, and she received the fellowship No. 240087 from Consejo Nacional de Ciencia y Tecnología (CONACYT).
Conflict of Interest
The authors declare that the research was conducted in the absence of any commercial or financial relationships that could be construed as a potential conflict of interest.
Publisher’s Note
All claims expressed in this article are solely those of the authors and do not necessarily represent those of their affiliated organizations, or those of the publisher, the editors and the reviewers. Any product that may be evaluated in this article, or claim that may be made by its manufacturer, is not guaranteed or endorsed by the publisher.
Acknowledgments
We thank Michael F. Dunn and Antony Buchala for the critical reading and comments on the manuscript. Thank you is also extended to Daniel Tapia Maruri from CEPROBI-IPN and MC Yordan Jhovani Romero-Contreras from CCG-UNAM for the technical help. NA-B acknowledges the fellowship, No. 929445, from CONACYT, Mexico.
Supplementary Material
The Supplementary Material for this article can be found online at: https://www.frontiersin.org/articles/10.3389/fpls.2021.738949/full#supplementary-material
Supplementary Figure 1 | Reactive oxygen species (ROS) production at 6 hpi with Botrytis cinerea in leaves from 4-week-old plants of Arabidopsis thaliana cuticular mutants and wild-type (WT) Columbia-0 (Col-0) and C24 plants. Fluorescence after DCF-DA staining was observed on leaves by using epifluorescence microscopy. 3, 3′-Diaminobenzidine (DAB) and nitroblue tetrazolium (NBT) were used to detect the accumulation of hydrogen peroxide (H2O2) and superoxide (O2–), respectively. The experiment was carried out six times, with similar results (n = 6 ± SD). Scale bar = 100 μm. Different lowercase letter columns indicate significant differences, according to one-way analysis of variance (ANOVA) (p-value < 0.001) followed by Tukey’s test. Representative pictures are shown.
Supplementary Figure 2 | The heat map shows the expression pattern in non-infected cutin and wax mutants, as well as the WT plants of the jasmonic acid (JA)/ethylene (ET), salicylic acid (SA), and abscisic acid (ABA) signaling pathways, and other defense-related genes selected from the literature. The colors of the heat map represent the z-score of each gene ranging from blue (−2) to yellow (2).
Supplementary Table 1 | Cuticular wax and cutin composition of rosette leaves of Arabidopsis Col-0 (wt), cer1-4, cer3-6 (yre), bdg, lacs2-3, C24 (wt), and eca2.
Supplementary Table 2 | Summary of bioinformatics data and mapped reads of Arabidopsis thaliana cutin and wax mutants in the RNA-seq libraries in non-infected and infected with Botrytis cinerea (6 hpi).
Supplementary Data 1 | List of significantly differentially expressed genes (DEGs) in non-infected cutin and wax mutants, related to Figure 4A. List of defense-related genes abundant in the non-infected mutants and wild-type (WT) plants, related to Supplementary Figure 2.
Supplementary Data 2 | List of up- and down-regulated genes shared by non-infected cutin or wax mutants, related to Figure 4B.
Supplementary Data 3 | Gene Ontology (GO) term enrichment of up- and down-regulated genes shared by non-infected cutin or wax mutants, related to Figure 4B.
Supplementary Data 4 | GO term enrichment of up- and downregulated genes in each non-infected cutin or wax mutant, related to Figure 5.
Supplementary Data 5 | List of significantly DEGs in cutin and wax mutants infected with B. cinerea (6 hpi), related to Figure 6A. List of defense-related genes abundant in the infected mutants and WT plants, related to Figure 7.
Supplementary Data 6 | List of up- and downregulated genes shared by cutin or wax mutants infected with B. cinerea (6 hpi), related to Figure 6B.
Supplementary Data 7 | GO term enrichment of up- and downregulated genes shared by infected cutin or wax mutants, related to Figure 6C.
Supplementary Data 8 | GO term enrichment of up- and downregulated genes shared by infected cutin or wax mutants, related to Figure 6B.
Footnotes
- ^ https://imagej.net/Fiji
- ^ https://www.bgi.com/us
- ^ https://www.arabidopsis.org/
- ^ http://bioinformatics.psb.ugent.be/webtools/Venn/
- ^ http://www.heatmapper.ca/expression/
References
Aarts, M. G., Keijzer, C. J., Stiekema, W. J., and Pereira, A. (1995). Molecular characterization of the CER1 gene of Arabidopsis involved in epicuticular wax biosynthesis and pollen fertility. Plant Cell 7, 2115–2127. doi: 10.1105/tpc.7.12.2115
AbuQamar, S., Chen, X., Dhawan, R., Bluhm, B., Salmeron, J., Lam, S., et al. (2006). Expression profiling and mutant analysis reveals complex regulatory networks involved in Arabidopsis response to Botrytis infection. Plant J. 48, 28–44. doi: 10.1111/j.1365-313X.2006.02849.x
Aragón, W., Reina-Pinto, J. J., and Serrano, M. (2017). The intimate talk between plants and microorganisms at the leaf surface. J. Exp. Bot. 68, 5339–5350. doi: 10.1093/jxb/erx327
Babicki, S., Arndt, D., Marcu, A., Liang, Y., Grant, J. R., Maciejewski, A., et al. (2016). Heatmapper: web-enabled heat mapping for all. Nucleic Acids Res. 44, W147–W153. doi: 10.1093/nar/gkw419
Bacete, L., Mélida, H., Miedes, E., and Molina, A. (2018). Plant cell wall-mediated immunity: cell wall changes trigger disease resistance responses. Plant J. 93, 614–636. doi: 10.1111/tpj.13807
Batista-Oliveira, J. S., Formey, D., Torres, M., Aragón, W., Romero-Contreras, Y. J., Maruri-López, I., et al. (2021). Gadolinium protects Arabidopsis thaliana against Botrytis cinerea through the activation of JA/ET-induced defense responses. Int. J. Mol. Sci. 22:4938. doi: 10.3390/ijms22094938
Baxter, A., Mittler, R., and Suzuki, N. (2014). ROS as key players in plant stress signalling. J. Exp. Bot. 65, 1229–1240. doi: 10.1093/jxb/ert375
Lionetti, V. (2014). Plant cell wall dynamics and wall-related susceptibility in plant-pathogen interactions. Front. Plant Sci. 5: 228. doi: 10.3389/fpls.2014.00228
Benikhlef, L., L’Haridon, F., Abou-Mansour, E., Serrano, M., Binda, M., Costa, A., et al. (2013). Perception of soft mechanical stress in Arabidopsis leaves activates disease resistance. BMC Plant Biol. 13: 133. doi: 10.1186/1471-2229-13-133
Bernard, A., and Joubès, J. (2013). Arabidopsis cuticular waxes: advances in synthesis, export and regulation. Prog. Lipid Res. 52, 110–129. doi: 10.1016/j.plipres.2012.10.002
Bessire, M., Chassot, C., Jacquat, A.-C., Humphry, M., Borel, S., Petétot, J. M.-C., et al. (2007). A permeable cuticle in Arabidopsis leads to a strong resistance to Botrytis cinerea. EMBO J. 26, 2158–2168. doi: 10.1038/sj.emboj.7601658
Bethke, G., Grundman, R. E., Sreekanta, S., Truman, W., Katagiri, F., and Glazebrook, J. (2014). Arabidopsis PECTIN METHYLESTERASEs contribute to immunity against Pseudomonas syringae. Plant Physiol. 164, 1093–1107. doi: 10.1104/pp.113.227637
Bethke, G., Thao, A., Xiong, G., Li, B., Soltis, N. E., Hatsugai, N., et al. (2016). Pectin biosynthesis is critical for cell wall integrity and immunity in Arabidopsis thaliana. Plant Cell 28, 537–556. doi: 10.1105/tpc.15.00404
Blanc, C., Coluccia, F., L’Haridon, F., Torres, M., Ortiz-Berrocal, M., Stahl, E., et al. (2018). The cuticle mutant eca2 modifies plant defense responses to biotrophic and necrotrophic pathogens and herbivory insects. Mol. Plant Microbe Interact. 31, 344–355. doi: 10.1094/MPMI-07-17-0181-R
Borisjuk, N., Hrmova, M., and Lopato, S. (2014). Transcriptional regulation of cuticle biosynthesis. Biotechnol. Adv. 32, 526–540. doi: 10.1016/j.biotechadv.2014.01.005
Bourdenx, B., Bernard, A., Domergue, F., Pascal, S., Léger, A., Roby, D., et al. (2011). Overexpression of Arabidopsis ECERIFERUM1 promotes wax very-long-chain alkane biosynthesis and influences plant response to biotic and abiotic stresses. Plant Physiol. 156, 29–45. doi: 10.1104/pp.111.172320
Buxdorf, K., Rubinsky, G., Barda, O., Burdman, S., Aharoni, A., and Levy, M. (2014). The transcription factor SlSHINE3 modulates defense responses in tomato plants. Plant Mol. Biol. 84, 37–47. doi: 10.1007/s11103-013-0117-1
Chassot, C., Nawrath, C., and Métraux, J.-P. (2007). Cuticular defects lead to full immunity to a major plant pathogen. Plant J. 49, 972–980. doi: 10.1111/j.1365-313X.2006.03017.x
Chen, X., Goodwin, S. M., Boroff, V. L., Liu, X., and Jenks, M. A. (2003). Cloning and characterization of the WAX2 gene of Arabidopsis involved in cuticle membrane and wax production. Plant Cell 15, 1170–1185. doi: 10.1105/tpc.010926
Choi, B., Ghosh, R., Gururani, M. A., Shanmugam, G., Jeon, J., Kim, J., et al. (2017). Positive regulatory role of sound vibration treatment in Arabidopsis thaliana against Botrytis cinerea infection. Sci. Rep. 7:2527. doi: 10.1038/s41598-017-02556-9
Choi, W. G., Miller, G., Wallace, I., Harper, J., Mittler, R., and Gilroy, S. (2017). Orchestrating rapid long-distance signaling in plants with Ca(2+), ROS and electrical signals. Plant J. 90, 698–707. doi: 10.1111/tpj.13492
Conrath, U. (2011). Molecular aspects of defence priming. Trends Plant Sci. 16, 524–531. doi: 10.1016/j.tplants.2011.06.004
Conrath, U., Beckers, G. J. M., Langenbach, C. J. G., and Jaskiewicz, M. R. (2015). Priming for enhanced defense. Annu. Rev. Phytopathol. 53, 97–119. doi: 10.1146/annurev-phyto-080614-120132
Cui, F., Brosché, M., Lehtonen, M. T., Amiryousefi, A., Xu, E., Punkkinen, M., et al. (2016). Dissecting abscisic acid signaling pathways involved in cuticle formation. Mol. Plant 9, 926–938. doi: 10.1016/j.molp.2016.04.001
Czechowski, T., Stitt, M., Altmann, T., Udvardi, M. K., and Scheible, W. R. (2005). Genome-wide identification and testing of superior reference genes for transcript normalization in Arabidopsis. Plant Physiol. 139, 5–17. doi: 10.1104/pp.105.063743
De Lorenzo, G., Ferrari, S., Giovannoni, M., Mattei, B., and Cervone, F. (2019). Cell wall traits that influence plant development, immunity, and bioconversion. Plant J. 97, 134–147. doi: 10.1111/tpj.14196
Del Corpo, D., Fullone, M. R., Miele, R., Lafond, M., Pontiggia, D., Grisel, S., et al. (2020). AtPME17 is a functional Arabidopsis thaliana pectin methylesterase regulated by its PRO region that triggers PME activity in the resistance to Botrytis cinerea. Mol. Plant Pathol. 21, 1620–1633. doi: 10.1111/mpp.13002
Dickman, M. B., Ha, Y. S., Yang, Z., Adams, B., and Huang, C. (2003). A protein kinase from Colletotrichum trifolii is induced by plant cutin and is required for appressorium formation. Mol. Plant Microbe Interact. 16, 411–421. doi: 10.1094/MPMI.2003.16.5.411
Domínguez, E., Heredia-Guerrero, J. A., and Heredia, A. (2015). Plant cutin genesis: unanswered questions. Trends Plant Sci. 20, 551–558. doi: 10.1016/j.tplants.2015.05.009
Engelsdorf, T., Gigli-Bisceglia, N., Veerabagu, M., Mckenna, J. F., Vaahtera, L., Augstein, F., et al. (2018). The plant cell wall integrity maintenance and immune signaling systems cooperate to control stress responses in Arabidopsis thaliana. Sci. Signal. 11:eaao3070. doi: 10.1126/scisignal.aao3070
Fabre, G., Garroum, I., Mazurek, S., Daraspe, J., Mucciolo, A., Sankar, M., et al. (2016). The ABCG transporter PEC1/ABCG32 is required for the formation of the developing leaf cuticle in Arabidopsis. New Phytol. 209, 192–201. doi: 10.1111/nph.13608
Fauth, M., Schweizer, P., Buchala, A., Markstadter, C., Riederer, M., Kato, T., et al. (1998). Cutin monomers and surface wax constituents elicit H2O2 in conditioned cucumber hypocotyl segments and enhance the activity of other H2O2 elicitors. Plant Physiol. 117, 1373–1380. doi: 10.1104/pp.117.4.1373
Fernández, V., Guzmán-Delgado, P., Graça, J., Santos, S., and Gil, L. (1998). Cuticle structure in relation to chemical composition: re-assessing the prevailing model. Front. Plant Sci. 7: 427. doi: 10.3389/fpls.2016.00427
Ferrari, S., Savatin, D., Sicilia, F., Gramegna, G., Cervone, F., and De Lorenzo, G. (2013). Oligogalacturonides: plant damage-associated molecular patterns and regulators of growth and development. FrFront. Plant Sci. 4: 49. doi: 10.3389/fpls.2013.00049
Fich, E. A., Segerson, N. A., and Rose, J. K. (2016). The plant polyester cutin: biosynthesis, structure, and biological roles. Annu. Rev. Plant Biol. 67, 207–233. doi: 10.1146/annurev-arplant-043015-111929
Francis, S. A., Dewey, F. M., and Gurr, S. J. (1996). The role of cutinase in germling development and infection byErysiphe graminisf.sp.hordei. Physiol. Mol. Plant Pathol. 49, 201–211. doi: 10.1006/pmpp.1996.0049
Gilbert, R. D., Johnson, A. M., and Dean, R. A. (1996). Chemical signals responsible for appressorium formation in the rice blast fungusMagnaporthe grisea. Physiol. Mol. Plant Pathol. 48, 335–346. doi: 10.1006/pmpp.1996.0027
Girard, A.-L., Mounet, F., Lemaire-Chamley, M., Gaillard, C., Elmorjani, K., Vivancos, J., et al. (2012). Tomato GDSL1 is required for cutin deposition in the fruit cuticle. Plant Cell 24, 3119–3134. doi: 10.1105/tpc.112.101055
Go, Y. S., Kim, H., Kim, H. J., and Suh, M. C. (2014). Arabidopsis cuticular wax biosynthesis is negatively regulated by the DEWAX gene encoding an AP2/ERF-type transcription factor. Plant Cell 26, 1666–1680. doi: 10.1105/tpc.114.123307
Govrin, E. M., and Levine, A. (2000). The hypersensitive response facilitates plant infection by the necrotrophic pathogen Botrytis cinerea. Curr. Biol. 10, 751–757. doi: 10.1016/s0960-9822(00)00560-1
Hansjakob, A., Bischof, S., Bringmann, G., Riederer, M., and Hildebrandt, U. (2010). Very-long-chain aldehydes promote in vitro prepenetration processes of Blumeria graminis in a dose- and chain length-dependent manner. New Phytol. 188, 1039–1054. doi: 10.1111/j.1469-8137.2010.03419.x
Hansjakob, A., Riederer, M., and Hildebrandt, U. (2011). Wax matters: absence of very-long-chain aldehydes from the leaf cuticular wax of the glossy11 mutant of maize compromises the prepenetration processes of Blumeria graminis. Plant Pathol. 60, 1151–1161. doi: 10.1111/j.1365-3059.2011.02467.x
Imran, Q. M., Hussain, A., Lee, S.-U., Mun, B.-G., Falak, N., Loake, G. J., et al. (2018). Transcriptome profile of NO-induced Arabidopsis transcription factor genes suggests their putative regulatory role in multiple biological processes. Sci. Rep. 8:771. doi: 10.1038/s41598-017-18850-5
Inada, N., and Savory, E. A. (2011). Inhibition of prepenetration processes of the powdery mildew Golovinomyces orontii on host inflorescence stems is reduced in the Arabidopsis cuticular mutant cer3 but not in cer1. J. Gen. Plant Pathol. 77:273. doi: 10.1007/s10327-011-0331-0
Ingram, G., and Nawrath, C. (2017). The roles of the cuticle in plant development: organ adhesions and beyond. J. Exp. Bot. 68, 5307–5321. doi: 10.1093/jxb/erx313
Isaacson, T., Kosma, D. K., Matas, A. J., Buda, G. J., He, Y., Yu, B., et al. (2009). Cutin deficiency in the tomato fruit cuticle consistently affects resistance to microbial infection and biomechanical properties, but not transpirational water loss. Plant J. 60, 363–377. doi: 10.1111/j.1365-313X.2009.03969.x
Javelle, M., Vernoud, V., Depège-Fargeix, N., Arnould, C., Oursel, D., Domergue, F., et al. (2010). Overexpression of the epidermis-specific homeodomain-leucine zipper IV transcription factor Outer Cell Layer1 in maize identifies target genes involved in lipid metabolism and cuticle biosynthesis. Plant Physiol. 154, 273–286. doi: 10.1104/pp.109.150540
Jeffree, C. E. (2006). “The fine structure of the plant cuticle,” in Annual Plant Reviews Volume 23: Biology of the Plant Cuticle, eds M. Riederer and C. Muller (Hoboken, NJ: Blackwell Publishing Ltd), 11–125. doi: 10.1002/9780470988718.ch2
Jetter, R., Kunst, L., and Samuels, A. L. (2006). “Composition of plant cuticular waxes,” in Annual Plant Reviews Volume 23: Biology of the Plant Cuticle, eds M. Riederer and C. Muller (Hoboken, NJ: Blackwell Publishing Ltd), 145–181. doi: 10.1002/9781119312994.apr0232
Jim nez-Jacinto, V., Sanchez-Flores, A., and Vega-Alvarado, L. (2019). Integrative differential expression analysis for multiple experiments (IDEAMEX): a web server tool for integrated RNA-Seq data analysis. Front. Genet. 10: 279. doi: 10.3389/fgene.2019.00279
Ju, S., Go, Y. S., Choi, H. J., Park, J. M., and Suh, M. C. (2017). DEWAX transcription factor is involved in resistance to Botrytis cinerea in Arabidopsis thaliana and Camelina sativa. Front. Plant Sci. 8: 1210. doi: 10.3389/fpls.2017.01210
Kurata, T., Kawabata-Awai, C., Sakuradani, E., Shimizu, S., Okada, K., and Wada, T. (2003). The YORE-YORE gene regulates multiple aspects of epidermal cell differentiation in Arabidopsis. Plant J. 36, 55–66. doi: 10.1046/j.1365-313x.2003.01854.x
Kurdyukov, S., Faust, A., Nawrath, C., Bär, S., Voisin, D., Efremova, N., et al. (2006). The epidermis-specific extracellular BODYGUARD controls cuticle development and morphogenesis in Arabidopsis. Plant Cell 18, 321–339. doi: 10.1105/tpc.105.036079
Laluk, K., and Mengiste, T. (2010). Necrotroph attacks on plants: wanton destruction or covert extortion? Arabidopsis Book 8:e0136. doi: 10.1199/tab.0136
Langmead, B., and Salzberg, S. L. (2012). Fast gapped-read alignment with Bowtie 2. Nat. Methods 9, 357–359. doi: 10.1038/nmeth.1923
Lee, S., Fu, F., Xu, S., Lee, S. Y., Yun, D.-J., and Mengiste, T. (2016). Global regulation of plant immunity by histone lysine methyl transferases. Plant Cell 28, 1640–1661.
Lehmann, S., Serrano, M., L’Haridon, F., Tjamos, S. E., and Metraux, J.-P. (2015). Reactive oxygen species and plant resistance to fungal pathogens. Phytochemistry 112, 54–62. doi: 10.1016/j.phytochem.2014.08.027
L’Haridon, F., Besson-Bard, A., Binda, M., Serrano, M., Abou-Mansour, E., and Balet, F., et al. (2011). A permeable cuticle is associated with the release of reactive oxygen species and induction of innate immunity. PLoS Pathog. 7, e1002148. doi: 10.1371/journal.ppat.1002148
Li, B., and Dewey, C. N. (2011). RSEM: accurate transcript quantification from RNA-Seq data with or without a reference genome. BMC Bioinform. 12: 323. doi: 10.1186/1471-2105-12-323
Li-Beisson, Y., Shorrosh, B., Beisson, F., Andersson, M. X., Arondel, V., Bates, P. D., et al. (2013). Acyl-lipid metabolism. Arabidopsis Book 11: e0161.
Lim, G.-H., Liu, H., Yu, K., Liu, R., Shine, M. B., Fernandez, J., et al. (2020). The plant cuticle regulates apoplastic transport of salicylic acid during systemic acquired resistance. Sci. Adv. 6:eaaz0478.
Lionetti, V. (2015). PECTOPLATE: the simultaneous phenotyping of pectin methylesterases, pectinases, and oligogalacturonides in plants during biotic stresses. Front. Plant Sci. 6:331. doi: 10.3389/fpls.2015.00331
Lionetti, V., Cervone, F., and Bellincampi, D. (2012). Methyl esterification of pectin plays a role during plant-pathogen interactions and affects plant resistance to diseases. J. Plant Physiol. 169, 1623–1630. doi: 10.1016/j.jplph.2012.05.006
Lionetti, V., Fabri, E., De Caroli, M., Hansen, A. R., Willats, W. G. T., Piro, G., et al. (2017). Three pectin methylesterase inhibitors protect cell wall integrity for Arabidopsis immunity to Botrytis. Plant Physiol. 173, 1844–1863. doi: 10.1104/pp.16.01185
Liu, J., Chen, S., Chen, L., Zhou, Q., Wang, M., Feng, D., et al. (2017). BIK1 cooperates with BAK1 to regulate constitutive immunity and cell death in Arabidopsis. J. Integr. Plant Biol. 59, 234–239. doi: 10.1111/jipb.12529
Liu, N., Zhao, L., Tang, L., Stobbs, J., Parkin, I., Kunst, L., et al. (2020). Mid-infrared spectroscopy is a fast screening method for selecting Arabidopsis genotypes with altered leaf cuticular wax. Plant Cell Environ. 43, 662–674. doi: 10.1111/pce.13691
Lolle, S. J., Berlyn, G. P., Engstrom, E. M., Krolikowski, K. A., Reiter, W. D., and Pruitt, R. E. (1997). Developmental regulation of cell interactions in the Arabidopsis fiddlehead-1 mutant: a role for the epidermal cell wall and cuticle. Dev. Biol. 189, 311–321. doi: 10.1006/dbio.1997.8671
Lorrai, R., Francocci, F., Gully, K., Martens, H. J., De Lorenzo, G., and Nawrath, C., et al. (2021). Impaired cuticle functionality and robust resistance to Botrytis cinerea in Arabidopsis thaliana plants with altered homogalacturonan integrity are dependent on the class III peroxidase AtPRX71. Front. Plant Sci. 12:696955. doi: 10.3389/fpls.2021.696955
Martin, L. B. B., Romero, P., Fich, E. A., Domozych, D. S., and Rose, J. K. C. (2017). Cuticle biosynthesis in tomato leaves is developmentally regulated by abscisic acid. Plant Physiol. 174, 1384–1398. doi: 10.1104/pp.17.00387
Mauch-Mani, B., Baccelli, I., Luna, E., and Flors, V. (2017). Defense priming: an adaptive part of induced resistance. Annu. Rev. Plant Biol. 68, 485–512. doi: 10.1146/annurev-arplant-042916-041132
Mengiste, T. (2012). Plant immunity to necrotrophs. Annu. Rev. Phytopathol. 50, 267–294. doi: 10.1146/annurev-phyto-081211-172955
Mengiste, T., Chen, X., Salmeron, J., and Dietrich, R. (2003). The Botrytis susceptible1 gene encodes an R2R3MYB transcription factor protein that is required for biotic and abiotic stress responses in Arabidopsis. Plant Cell 15, 2551–2565. doi: 10.1105/tpc.014167
Molina, A., Miedes, E., Bacete, L., Rodríguez, T., Mélida, H., Denancé, N., et al. (2021). Arabidopsis cell wall composition determines disease resistance specificity and fitness. Proc. Natl. Acad. Sci. U.S.A. 118:e2010243118. doi: 10.1073/pnas.2010243118
Narváez-Barragán, D. A., Tovar-Herrera, O. E., Torres, M., Rodríguez, M., Humphris, S., Toth, I. K., et al. (2020). Expansin-like Exl1 from Pectobacterium is a virulence factor required for host infection, and induces a defence plant response involving ROS, and jasmonate, ethylene and salicylic acid signalling pathways in Arabidopsis thaliana. Sci. Rep. 10:7747. doi: 10.1038/s41598-020-64529-9
Nawrath, C., Schreiber, L., Franke, R. B., Geldner, N., Reina-Pinto, J. J., and Kunst, L. (2013). Apoplastic diffusion barriers in Arabidopsis. Am. Soc. Plant Biol. 11:e0167. doi: 10.1199/tab.0167
O’Brien, J. A., Daudi, A., Butt, V. S., and Paul Bolwell, G. (2012). Reactive oxygen species and their role in plant defence and cell wall metabolism. Planta 236, 765–779. doi: 10.1007/s00425-012-1696-9
Podila, G. K., Rogers, L. M., and Kolattukudy, P. E. (1993). Chemical signals from avocado surface wax trigger germination and appressorium formation in Colletotrichum gloeosporioides. Plant Physiol. 103, 267–272. doi: 10.1104/pp.103.1.267
Riederer, M. (2006). “Introduction: biology of the plant cuticle,” in Annual Plant Reviews Volume 23: Biology of the Plant Cuticle, eds M. Riederer and C. Muller (Hoboken, NJ: Blackwell Publishing Ltd), 1–10. doi: 10.1002/9780470988718.ch1
Rowland, O., Lee, R., Franke, R., Schreiber, L., and Kunst, L. (2007). The CER3 wax biosynthetic gene from Arabidopsis thaliana is allelic to WAX2/YRE/FLP1. FEBS Lett. 581, 3538–3544. doi: 10.1016/j.febslet.2007.06.065
Saini, P., Gani, M., Kaur, J. J., Godara, L. C., Singh, C., Chauhan, S. S., et al. (2018). “Reactive oxygen species (ROS): a way to stress survival in plants,” in Abiotic Stress-Mediated Sensing and Signaling in Plants: An Omics Perspective, eds S. M. Zargar and M. Y. Zargar (Singapore: Springer), 127–153. doi: 10.1007/978-981-10-7479-0_4
Sakuradani, E., Zhao, L., Haslam, T. M., and Kunst, L. (2013). The CER22 gene required for the synthesis of cuticular wax alkanes in Arabidopsis thaliana is allelic to CER1. Planta 237, 731–738. doi: 10.1007/s00425-012-1791-y
Salinas-Mondragón, R. E., Garcidueñas-Piña, C., and Guzmán, P. (1999). Early elicitor induction in members of a novel multigene family coding for highly related RING-H2 proteins in Arabidopsis thaliana. Plant Mol. Biol. 40, 579–590. doi: 10.1023/a:1006267201855
Schindelin, J., Arganda-Carreras, I., Frise, E., Kaynig, V., Longair, M., Pietzsch, T., et al. (2012). Fiji: an open-source platform for biological-image analysis. Nat. Methods 9, 676–682. doi: 10.1038/nmeth.2019
Schmittgen, T. D., and Livak, K. J. (2008). Analyzing real-time PCR data by the comparative C(T) method. Nat. Protoc. 3, 1101–1108. doi: 10.1038/nprot.2008.73
Schnurr, J., Shockey, J., and Browse, J. (2004). The Acyl-CoA synthetase encoded by LACS2 is essential for normal cuticle development in Arabidopsis. Plant Cell 16, 629–642. doi: 10.1105/tpc.017608
Schweizer, P., Felix, G., Buchala, A., Müller, C., and Métraux, J.-P. (1996). Perception of free cutin monomers by plant cells. Plant J. 10, 331–341. doi: 10.1046/j.1365-313x.1996.10020331.x
Sela, D., Buxdorf, K., Shi, J. X., Feldmesser, E., Schreiber, L., and Aharoni, A., et al. (2013). Overexpression of AtSHN1/WIN1 provokes unique defense responses. PLoS One 8:e70146. doi: 10.1371/journal.pone.0070146.g006
Seo, P. J., and Park, C.-M. (2010). MYB96-mediated abscisic acid signals induce pathogen resistance response by promoting salicylic acid biosynthesis in Arabidopsis. New Phytol. 186, 471–483. doi: 10.1111/j.1469-8137.2010.03183.x
Serrano, M., Coluccia, F., Torres, M., L’Haridon, F., and M traux, J. P. (2014). The cuticle and plant defense to pathogens. Front. Plant Sci. 5:274. doi: 10.3389/fpls.2014.00274
Serrano, M., and Guzmán, P. (2004). Isolation and gene expression analysis of Arabidopsis thaliana mutants with constitutive expression of ATL2, an early elicitor-response RING-H2 zinc-finger gene. Genetics 167, 919–929. doi: 10.1534/genetics.104.028043
Sieber, P., Schorderet, M., Ryser, U., Buchala, A., Kolattukudy, P., Métraux, J.-P., et al. (2000). Transgenic Arabidopsis plants expressing a fungal cutinase show alterations in the structure and properties of the cuticle and postgenital organ fusions. Plant Cell 12, 721–738. doi: 10.1105/tpc.12.5.721
Siegmund, U., and Viefhues, A. (2016). “Reactive oxygen species in the Botrytis - host interaction,” in Botrytis - the Fungus, the Pathogen and its Management in Agricultural Systems, eds S. Fillinger and Y. Elad (Cham: Springer International Publishing), 269–289. doi: 10.1007/978-3-319-23371-0_14
Tanaka, T., Tanaka, H., Machida, C., Watanabe, M., and Machida, Y. (2004). A new method for rapid visualization of defects in leaf cuticle reveals five intrinsic patterns of surface defects in Arabidopsis. Plant J. 37, 139–146. doi: 10.1046/j.1365-313x.2003.01946.x
Tang, D., Simonich, M. T., and Innes, R. W. (2007). Mutations in LACS2, a long-chain Acyl-Coenzyme a synthetase, enhance susceptibility to avirulent Pseudomonas syringae but confer resistance to Botrytis cinerea in Arabidopsis. Plant Physiol. 144, 1093–1103. doi: 10.1104/pp.106.094318
Tateda, C., Zhang, Z., Shrestha, J., Jelenska, J., Chinchilla, D., and Greenberg, J. T. (2014). Salicylic acid regulates Arabidopsis microbial pattern receptor kinase levels and signaling. Plant Cell 26, 4171–4187. doi: 10.1105/tpc.114.131938
Thordal-Christensen, H., Zhang, Z., Wei, Y., and Collinge, D. B. (1997). Subcellular localization of H2O2 in plants. H2O2 accumulation in papillae and hypersensitive response during the barley—powdery mildew interaction. Plant J. 11, 1187–1194. doi: 10.1046/j.1365-313x.1997.11061187.x
Torres, M. A., and Dangl, J. L. (2005). Functions of the respiratory burst oxidase in biotic interactions, abiotic stress and development. Curr. Opin. Plant Biol. 8, 397–403. doi: 10.1016/j.pbi.2005.05.014
Torres, M. A., Jones, J. D. G., and Dangl, J. L. (2006). Reactive oxygen species signaling in response to pathogens. Plant Physiol. 141, 373–378. doi: 10.1104/pp.106.079467
van der Burgh, A. M., Postma, J., Robatzek, S., and Joosten, M. H. A. J. (2019). Kinase activity of SOBIR1 and BAK1 is required for immune signalling. Mol. Plant Pathol. 20, 410–422. doi: 10.1111/mpp.12767
van Kan, J. A. (2006). Licensed to kill: the lifestyle of a necrotrophic plant pathogen. Trends Plant Sci. 11, 247–253. doi: 10.1016/j.tplants.2006.03.005
Veronese, P., Nakagami, H., Bluhm, B., Abuqamar, S., Chen, X., Salmeron, J., et al. (2005). The membrane-anchored Botrytis-induced kinase1 plays distinct roles in Arabidopsis resistance to necrotrophic and biotrophic pathogens. Plant Cell 18, 257–273. doi: 10.1105/tpc.105.035576
Voisin, D., Nawrath, C., Kurdyukov, S., Franke, R. B., Reina-Pinto, J. J., and Efremova, N., et al. (2009). Dissection of the complex phenotype in cuticular mutants of Arabidopsis reveals a role of SERRATE as a mediator. PLoS Genet. 5:e1000703. doi: 10.1371/journal.pgen.1000703
Wang, X., Kong, L., Zhi, P., and Chang, C. (2020). Update on cuticular wax biosynthesis and its roles in plant disease resistance. Int. J. Mol. Sci. 21:5514. doi: 10.3390/ijms21155514
Wellesen, K., Durst, F., Pinot, F., Benveniste, I., Nettesheim, K., Wisman, E., et al. (2001). Functional analysis of the LACERATA gene of Arabidopsis provides evidence for different roles of fatty acid ω-hydroxylation in development. Proc. Natl. Acad. Sci. U.S.A. 98, 9694–9699. doi: 10.1073/pnas.171285998
Windram, O., Madhou, P., Mchattie, S., Hill, C., Hickman, R., Cooke, E., et al. (2012). Arabidopsis defense against Botrytis cinerea: chronology and regulation deciphered by high-resolution temporal transcriptomic analysis. Plant Cell 24, 3530–3557. doi: 10.1105/tpc.112.102046
Xia, Y., Gao, Q.-M., Yu, K., Lapchyk, L., Navarre, D., Hildebrand, D., et al. (2009). An intact cuticle in distal tissues is essential for the induction of systemic acquired resistance in plants. Cell Host Microbe 5, 151–165. doi: 10.1016/j.chom.2009.01.001
Xia, Y., Yu, K., Navarre, D., Seebold, K., Kachroo, A., and Kachroo, P. (2010). The glabra1 mutation affects cuticle formation and plant responses to microbes. Plant Physiol. 154, 833–846. doi: 10.1104/pp.110.161646
Xiao, F., Mark Goodwin, S., Xiao, Y., Sun, Z., Baker, D., Tang, X., et al. (2004). Arabidopsis CYP86A2 represses Pseudomonas syringae type III genes and is required for cuticle development. EMBO J. 23, 2903–2913. doi: 10.1038/sj.emboj.7600290
Yeats, T. H., and Rose, J. K. (2013). The formation and function of plant cuticles. Plant Physiol. 163, 5–20. doi: 10.1104/pp.113.222737
Yeh, Y. H., Panzeri, D., Kadota, Y., Huang, Y. C., Huang, P. Y., Tao, C. N., et al. (2016). The Arabidopsis Malectin-Like/LRR-RLK IOS1 is critical for BAK1-dependent and BAK1-independent pattern-triggered immunity. Plant Cell 28, 1701–1721. doi: 10.1105/tpc.16.00313
Yephremov, A., Wisman, E., Huijser, P., Huijser, C., Wellesen, K., and Saedler, H. (1999). Characterization of the FIDDLEHEAD gene of Arabidopsis reveals a link between adhesion response and cell differentiation in the epidermis. Plant Cell 11, 2187–2201. doi: 10.1105/tpc.11.11.2187
Zhao, L., Haslam, T. M., Sonntag, A., Molina, I., and Kunst, L. (2019). Functional overlap of long-chain Acyl-CoA synthetases in Arabidopsis. Plant Cell Physiol. 60, 1041–1054. doi: 10.1093/pcp/pcz019
Ziv, C., Zhao, Z., Gao, Y. G., and Xia, Y. (2018). Multifunctional roles of plant cuticle during plant-pathogen interactions. Front. Plant Sci. 9:1088. doi: 10.3389/fpls.2018.01088
Zou, J.-J., Li, X.-D., Ratnasekera, D., Wang, C., Liu, W.-X., Song, L.-F., et al. (2015). Arabidopsis CALCIUM-DEPENDENT PROTEIN KINASE8 and CATALASE3 function in abscisic acid-mediated signaling and H2O2 homeostasis in stomatal guard cells under drought stress. Plant Cell 27, 1445–1460. doi: 10.1105/tpc.15.00144
Keywords: cuticle, cuticular mutants, B. cinerea, permeability, ROS, cell wall
Citation: Aragón W, Formey D, Aviles-Baltazar NY, Torres M and Serrano M (2021) Arabidopsis thaliana Cuticle Composition Contributes to Differential Defense Response to Botrytis cinerea. Front. Plant Sci. 12:738949. doi: 10.3389/fpls.2021.738949
Received: 09 July 2021; Accepted: 06 October 2021;
Published: 05 November 2021.
Edited by:
Isabel Molina, Algoma University, CanadaReviewed by:
Ye Xia, The Ohio State University, United StatesLucas Busta, University of Minnesota Duluth, United States
Copyright © 2021 Aragón, Formey, Aviles-Baltazar, Torres and Serrano. This is an open-access article distributed under the terms of the Creative Commons Attribution License (CC BY). The use, distribution or reproduction in other forums is permitted, provided the original author(s) and the copyright owner(s) are credited and that the original publication in this journal is cited, in accordance with accepted academic practice. No use, distribution or reproduction is permitted which does not comply with these terms.
*Correspondence: Wendy Aragón, d2FyYWdvbkBjY2cudW5hbS5teA==; Mario Serrano, c2VycmFub0BjY2cudW5hbS5teA==