- 1Plant and Environmental Sciences, Clemson University, Clemson, SC, United States
- 2Agilent Technologies, Glasgow, United Kingdom
- 3Grain Legume Genetics and Physiology Research Unit, Washington State University, Pullman, WA, United States
Chickpea is a highly nutritious pulse crop with low digestible carbohydrates (40–60%), protein (15–22%), essential fats (4–8%), and a range of minerals and vitamins. The fatty acid composition of the seed adds value because fats govern the texture, shelf-life, flavor, aroma, and nutritional composition of chickpea-based food products. Therefore, the biofortification of essential fatty acids has become a nutritional breeding target for chickpea crop improvement programs worldwide. This paper examines global chickpea production, focusing on plant lipids, their functions, and their benefits to human health. In addition, this paper also reviews the chemical analysis of essential fatty acids and possible breeding targets to enrich essential fatty acids in chickpea (Cicer arietinum) biofortification. Biofortification of chickpea for essential fatty acids within safe levels will improve human health and support food processing to retain the quality and flavor of chickpea-based food products. Essential fatty acid biofortification is possible by phenotyping diverse chickpea germplasm over suitable locations and years and identifying the candidate genes responsible for quantitative trait loci mapping using genome-wide association mapping.
Introduction
Chickpea (Cicer arietinum) is a self-pollinating diploid (2n=2x=16) pulse crop with a 738Mbp genome (Varshney et al., 2013). Chickpea primarily extended from Cicer reticulatum Ladizinsky approximately 11,000years ago (Zohari and Hopf, 2000; Kerem et al., 2007), a variable wild species that originated in several regions of southeastern Turkey (37.3–39.3°N, 38.2–43.6°E; Kerem et al., 2007). Chickpea presently has 44 species, of which 35 are perennial, and nine are annual. Chickpea has two market classes—kabuli and desi—based on seed morphology (Knights and Hobson, 2016). Kabuli has become popular in Western markets as hummus and canned and raw seeds for salads and soups, whereas desi seeds are split and consumed in Southeast Asia as “channa dal.”
Chickpea consumption is popular in many regions around the globe, mainly due to its high nutritional quality. The chickpea seed matrix is comprised of carbohydrates (50–58%), protein (15–22%), moisture (7–8%), fat (3.8–10.20%), and micronutrients (<1%; Jukanti et al., 2012; USDA, 2021). Chickpea carbohydrates include a range of prebiotic carbohydrates, including sugar alcohols, fructooligosaccharides, raffinose family oligosaccharides, inulin, and resistant starch (Peterbauer and Richter, 2001; Johnson et al., 2020), which modulate the gut microbiome and improve human gut health (Roberfroid et al., 2009). The mean protein content in chickpea is nearly 18% [(kabuli: 18.4% (16.2–22.4%); desi: 18.2% (15.6–21.4%)], which is higher than lentil and field pea (Upadhyaya et al., 2016). Chickpea is rich in lysine and arginine and low in sulfur (S)-containing amino acids such as cysteine and methionine (Jukanti et al., 2012). Moreover, chickpea is a rich source of minerals, including iron (Fe), zinc (Zn), and selenium (Se).
The United Nations established Sustainable Development Goals to end global hunger and malnutrition by 2030 (United Nations, 2021a,b). Biofortification or conventional breeding with modern biotechnology to enhanced micronutrient concentrations in staple food crops has been vital to combat global hunger and malnutrition. To date, many staple food crops have been biofortified with micronutrients, and cultivars were released to these vulnerable populations globally (Harvest Plus, 2021). Chickpea is a target candidate pulse crop for mineral and vitamin biofortification (Thavarajah and Thavarajah, 2012; Vandemark et al., 2018, 2020; Kumar and Pandey, 2020). During the last decade, several global research foundations have attempted to develop Fe-, Zn-, and Se-enriched chickpea cultivars to combat micronutrient malnutrition or “hidden hunger” (Thavarajah and Thavarajah, 2012; Vandemark et al., 2018). Biofortified chickpea provides 5.2–6.0mg of Fe, 2.5–5.3mg of Zn, and 15.3–56.3mg of Se in a 100-g serving, representing a significant portion of the recommended daily allowance (RDA) of these essential elements (Thavarajah and Thavarajah, 2012; Ray et al., 2014; Vandemark et al., 2018). A 100-g serving also provides 125–159mg of magnesium (Mg), 93–197mg of calcium (Ca), 0.7–1.1mg of copper (Cu), 732–1,126mg of potassium (K), and 263–370mg of phosphorus (P; Thavarajah and Thavarajah, 2012). Chickpea is also a significant source of carotenoids; beta-carotenoid is the most abundant, followed by canthaxanthin and xanthophyll (Thavarajah and Thavarajah, 2012). Vitamins such as folic acid, tocopherols, and vitamin B complex (B2, B5, and B6) are also found in chickpea (Jukanti et al., 2012). Overall, chickpea is a rich source of prebiotic carbohydrates, protein, and several micronutrients, and these components have already been incorporated into global chickpea biofortification programs (Thavarajah and Thavarajah, 2012; Vandemark et al., 2020). However, fat composition is the least-studied nutritional trait of chickpea, and genetic advancement studies are required to advance fatty acid biofortification.
Fats, which provide the storage energy required for seed germination (Nelson and Cox, 2008), occupy a minor proportion of the chickpea seed matrix compared to other nutrients. Chickpea is not an oilseed crop but has a higher fat content than other pulse crops (Jukanti et al., 2012). Sterols, tocopherols (phytosterols), and lipids are components of fat found in chickpea (Jukanti et al., 2012). The fatty acids in chickpeas—polyunsaturated fatty acids (PUFAs), monounsaturated fatty acids (MUFAs), and saturated fatty acids (SFAs)—mainly originate from the lipids. These are essential fatty acids (EFAs; ω-6 and ω-3 PUFAs), vital for humans in the biosynthesis of hormones and maintaining cellular integrity (Di Pasquale, 2009). Consequently, chickpea consumption can benefit human health by providing important fatty acids. This review focuses on global chickpea production, biofortification, the function of fats and benefits to human health, chemical analysis of EFAs, and possible breeding targets to optimize ω-6 and ω-3 fatty acids chickpea.
Chickpea Production
Chickpea ranks third in the global production of pulses at ~11.6 million tons per annum, 80% of which is desi and the remaining 20% is kabuli (Merga and Haji, 2019). Chickpea is grown in nearly 57 countries worldwide in varying climatic and growing conditions (Merga and Haji, 2019). India was the leading global chickpea producer in 2019, followed by Turkey, Russia, Myanmar, Pakistan, and Ethiopia (FAOSTAT, 2020; Table 1). In great part due to India’s large-scale production, Asia dominated global chickpea production in 2019 compared to the Americas (83.4 vs. 6.1%, respectively; FAOSTAT, 2020). In the last two decades, the harvested area has correlated with chickpea production, and both generally show an increase over time (except for lower production in 2015 and 2019; FAOSTAT, 2020). Notably, India has lower yields than smaller producers such as Ethiopia and Mexico (FAOSTAT, 2020), resulting in its position as the world’s largest chickpea importer despite its large-scale production (Merga and Haji, 2019). During the last 2years, India’s imports increased from 0.19 MT in 2018 to 0.37 MT in 2019, possibly due to the lower yields in 2019 (9.93 MT) than in 2018 (11.3 MT).
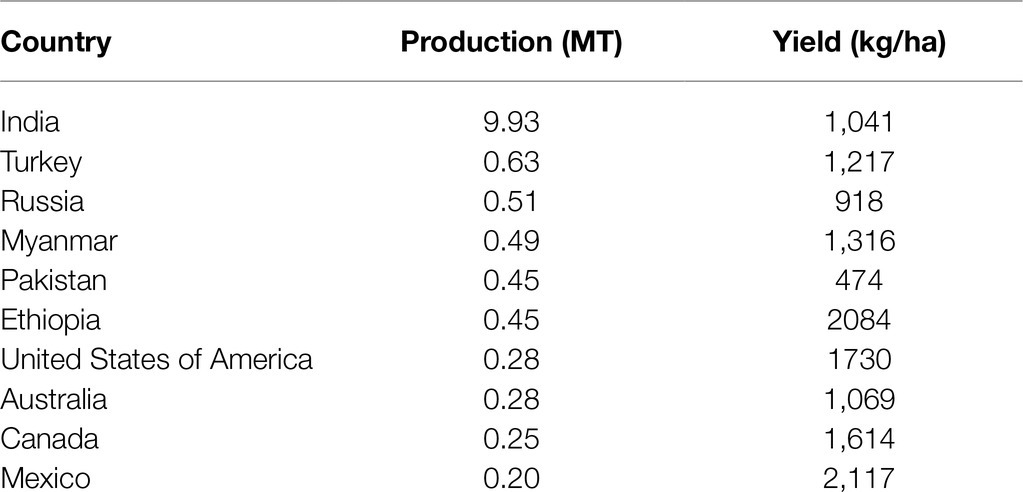
Table 1. Global chickpea production and mean grain yields in 2019 (FAOSTAT, 2020).
Biofortification
Malnutrition is a persisting global calamity that is prevalent mainly in Africa and South Asia. It exists in three aspects: undernutrition (stunting, wasting, and underweight), obesity, and malnutrition associated with micronutrient deficiency (hidden hunger). The World Health Organization (WHO) estimates over 2 billion people suffer from hidden hunger (Ritchie and Roser, 2017). At the same time, 150.8 million, 50.5 million, and 38.3 million children aged below 5years are stunted, wasted, and overweight, respectively (Ritchie and Roser, 2017; Global Nutrition Report, 2018). South Asian women and school children are highly vulnerable to malnutrition. One-third of women of reproductive age are anemic and show higher susceptibility to obesity than men (Global Nutrition Report, 2018). Plant breeding and agronomical practices introduced in the 1960s during the green revolution primarily combatted global hunger, especially through large-scale cereal production, providing the necessary calories or proteins to these vulnerable populations (Thavarajah et al., 2014; Roorkiwal et al., 2021). However, the consumption of cereals contributed to hidden hunger or micronutrient malnutrition in most developing nations (Roorkiwal et al., 2021). Micronutrients mediate human physical and mental development and further serve as cofactors of enzymes that catalyze biochemical reactions in the body, modulating human physiology and growth (White and Broadley, 2005; Malik and Maqbool, 2020).
Several global approaches have been implemented to increase the bioavailability of nutrients in staple food crops (Welch and Graham, 2004; White and Broadley, 2005; Thavarajah and Thavarajah, 2012). However, technological, socio-economical, financial, and demographical constraints are challenges with nutrient fortification programs. Biofortification is breeding crops to optimize micronutrient concentration and bioavailability, enriching their nutritional value to combat hidden hunger (Garcia-Casal et al., 2017; Roorkiwal et al., 2021). Biofortification has three strategies: agronomic (fertilizing the soil or foliar application), conventional breeding approaches, and molecular technologies (Garcia-Casal et al., 2017). Biofortification is a convenient approach to combat ‘hidden hunger,’ primarily due to low financial investment, tendency to penetrate demographic barriers benefitting rural populations, and provision of germplasm to farmers at zero marginal expenditure during early investment (Bouis and Saltzman, 2017). In 2015, crops were biofortified for vitamin A (fleshy-orange sweet potato, cassava, and orange corn), Fe (beans and millet), and Zn (rice and wheat) by officially releasing the germplasm of biofortified varieties in 30 countries, further expanding trials and official breeding in more than 50 countries (Birol et al., 2015). However, biofortification attempts have been less frequent in pulses than in cereals (rice, wheat, and corn) during the present decade (Kumar and Pandey, 2020).
Current biofortification efforts in chickpea are focused on enriching micronutrients and reducing antinutrient factors (Sreeramaiah et al., 2007; Jukanti et al., 2012; Thavarajah and Thavarajah, 2012; Vandemark et al., 2020). Agronomic approaches such as fertilizer application (including foliar spraying) and genetic engineering (GE) have been attempted on chickpea to enrich minerals, such as Fe, Zn, and Se (Table 2, Poblaciones et al., 2014; Khalid et al., 2015; Pal et al., 2019, 2021). Soil and foliar application of Zn and urea can increase chickpea’s Zn and Fe content (Pal et al., 2019). A combined application of Fe, Zn, and urea (in a tank mix) can increase Fe and Zn concentrations in chickpea seeds and positively influence grain yields and protein levels (Pal et al., 2021). Two separate studies indicate inoculating Zn-solubilizing bacteria (B. altitudinis) and rhizobacteria at chickpea planting increase seed Zn and Fe concentration in low Zn soils (Khalid et al., 2015; Kushwaha et al., 2021). Transgenic approaches have also been used for Fe biofortification in chickpea. For example, overexpression of the nicotamine synthase 2 (CaNAS2) and ferritin (GmFER) genes in chickpea increased seed Fe concentration (Tan et al., 2018). However, the above transgenic approach has not demonstrated any significant outcome for conventional chickpea breeding programs. Although biofortification significantly focuses on micronutrients, the techniques followed can be applied to other macro nutritional traits (Garcia-Casal et al., 2017; Roorkiwal et al., 2021). Linoleic acid (LA; ω-6) is the most abundant (essential) fatty acid in chickpea, while α-linolenic acid (ALA; ω-3), the other essential fatty acid, is far less available in the seed (Jukanti et al., 2012). ALA is known for its human health benefits (Simopoulos, 2002, 2006, 2016). Thus, breeding chickpea to enrich the seed in ALA is important; however, the quantitative nature of these genetic traits makes chickpea breeding much complicated than for traits controlled by a single gene.
Chickpea Lipids
In chickpea, lipids persist as storage and membrane molecules. Storage lipids are triacylglycerols (TAGs), which are suspended as oil droplets (oily phase) on the cell cytosol serve as primary sinks of fatty acids (including EFAs; Nelson and Cox, 2008; Cagliari et al., 2011). TAGs are the most abundant neutral lipid in desi-type chickpea and typically serve as biosynthetic precursors and energy supplements during seed germination (Zia-Ul-Haq et al., 2007; Jukanti et al., 2012; Weselake et al., 2021). The general structure of TAGs includes a glycerol group esterified with three fatty acids, either similar or different (Figure 1A). Chickpea also has phospholipids (glycerophospholipids; Figure 1B), sphingolipids (Figure 1C), glycolipids (galactolipids and sulpholipids; Figure 2), and phytosterols as membrane lipids (Clemente et al., 1998; Zia-Ul-Haq et al., 2007; Michaelson et al., 2016). Both storage and membrane lipids contribute to the total chickpea fat composition. The majority of the fatty acids in chickpea are originated from the storage lipids (TAGs), which are the most abundant neutral lipids in seeds (Jukanti et al., 2012). Chickpea has a general fat content of 3.8–10.2%, which is higher than other pulse crops (e.g., lentils, red kidney beans, etc.; Jukanti et al., 2012); the fat content also varies with market class, with ranges from 3.4–8.8% and 2.9–7.4% for kabuli and desi, respectively (Yadav, 2007).
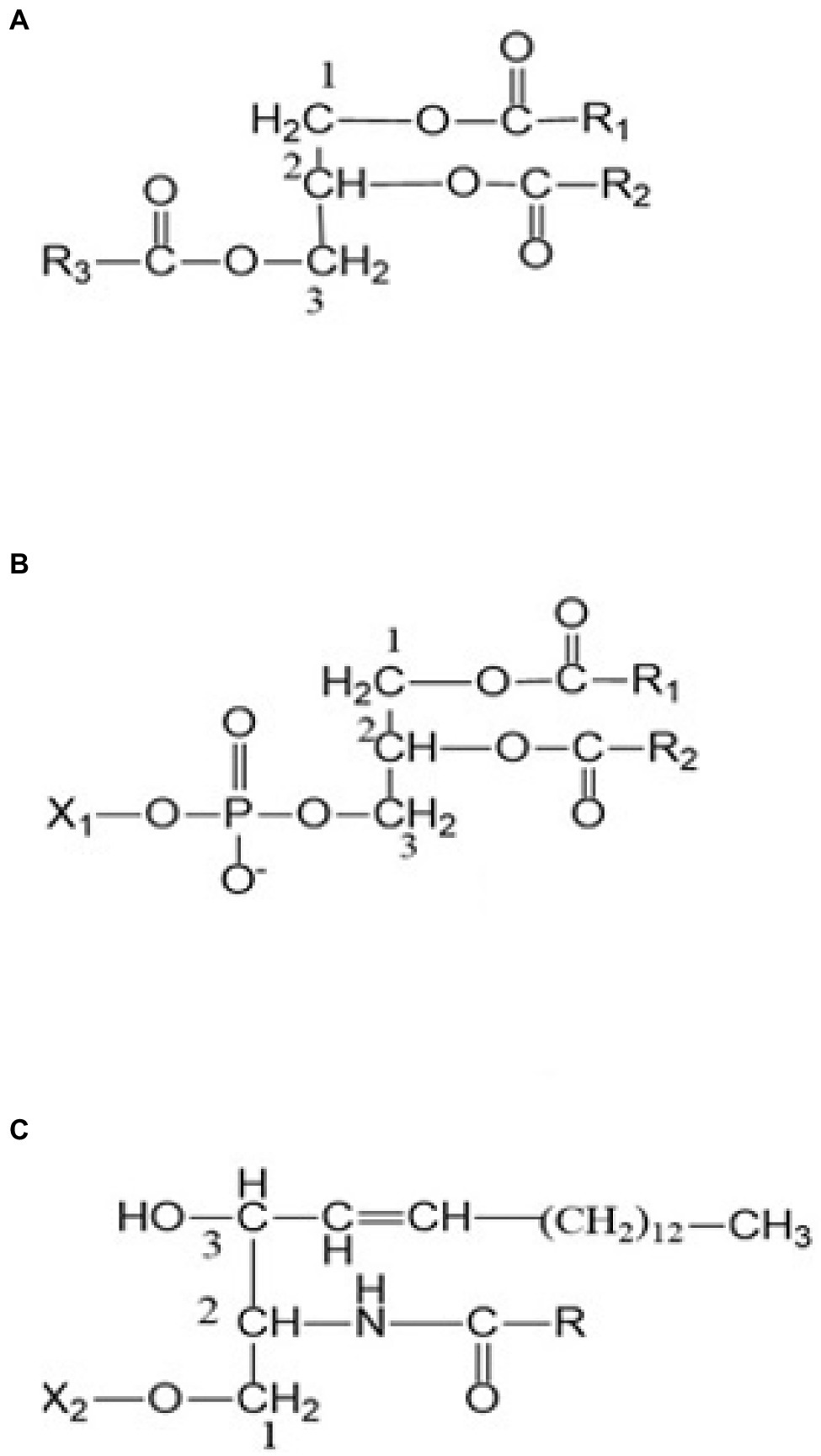
Figure 1. (A) A triacylglycerol (TAG) (B) a phospholipid, and (C) a sphingolipid. R, R1, and R2 are alkyl or alkenyl groups attached to ester carbonyls. X1: H, ethanolamine, choline, serine, glycerol, or phosphatidylcholine functional groups. X2: H, phosphocholine, glucose, or oligosaccharide functional groups.
Fatty Acids and EFAs
Typically, fatty acids are long-chain hydrocarbon molecules with an attached carboxylic acid group. In chickpea, fatty acids mainly originate from TAGs (Zia-Ul-Haq et al., 2007; Jukanti et al., 2012) as previously indicated and are classified as saturated (with double bonds) or unsaturated (no double bonds) based on the bonding nature (Fahy et al., 2005; Rustan, 2005; Figure 3). Unsaturated fatty acids are divided into PUFAs and MUFAs. LA (ω-6) and ALA (ω-3) are PUFAs (Innis, 1991), while oleic acid (OA; ω-9) is a MUFA. LA and ALA are EFAs because they are not synthesized in humans (animals) and must be supplemented from the diet, while OA is not (because animals produce it; Warude et al., 2006) but serves as a precursor for LA. The enzymes to convert OA to LA and then LA to ALA (12-desaturase and 15-desaturase, respectively) exist in plants (Warude et al., 2006; Lee et al., 2016, i.e., chickpea). Within total chickpea fats, 66% are PUFAs, 19% are MUFAs, and 15% are SFAs. Both market classes have considerable amounts of LA (kabuli: ~51.2%, desi: ~61.62%) and OA (kabuli: ~32.6%, desi: ~ 22.31%), which are generally higher than for other edible pulses such as lentils (LA: ~44.4%, OA: ~20.9%), beans (LA: ~46.7%, OA: ~28.1%), and peas (LA: ~45.6%, OA: ~23.2%; Wang and Daun, 2004). Chickpea also contains palmitic acid (kabuli: ~9.41%, desi: ~9.41%) and ALA (kabuli: ~2.69%, desi: ~3.15%; Wang and Daun, 2004; Jukanti et al., 2012).
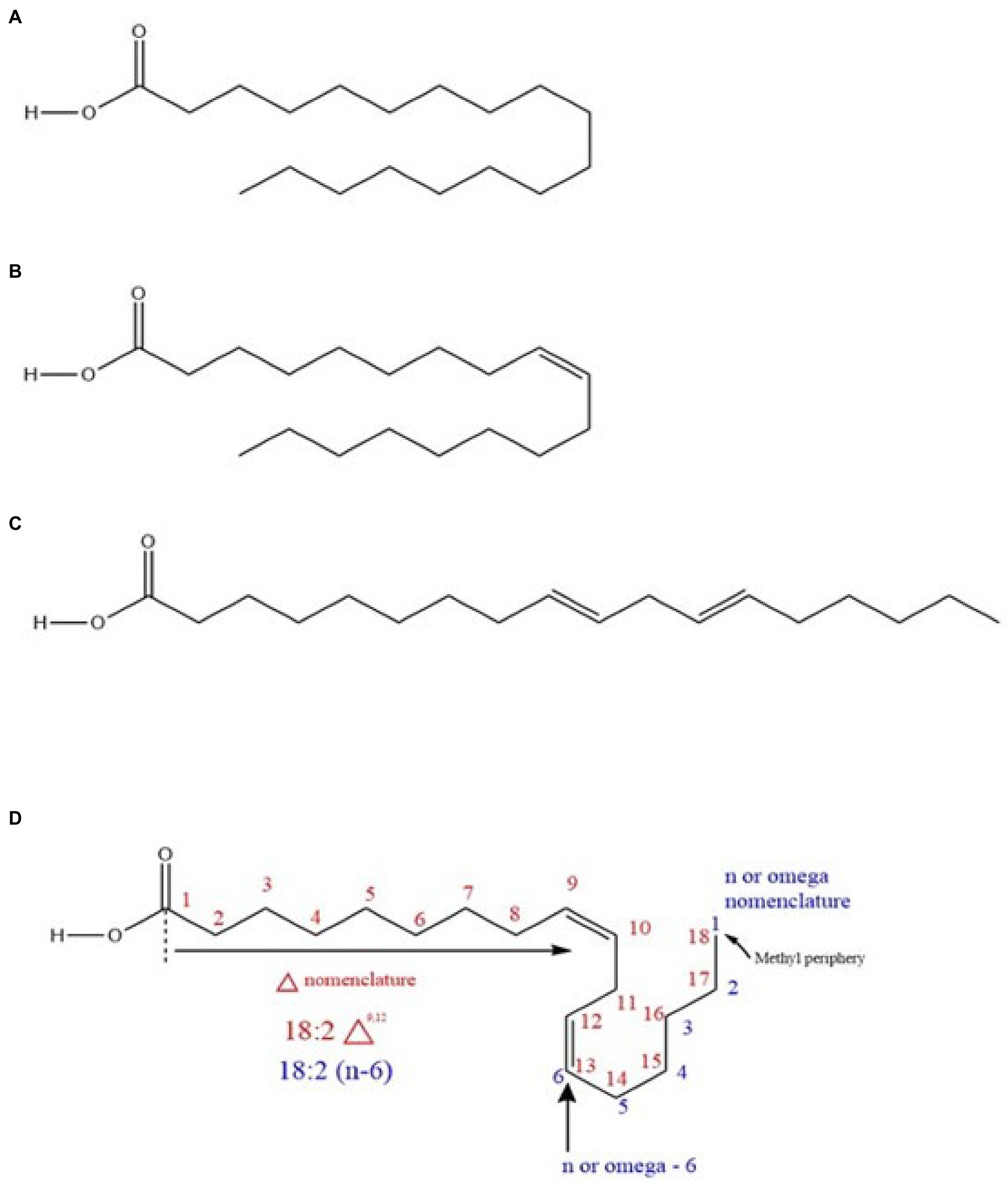
Figure 3. (A) A saturated fatty acid (stearic acid) (B) a monounsaturated fatty acid (MUFA; oleic acid) (C) a polyunsaturated fatty acid (PUFA; trans linoleic acid), and (D) nomenclature system of a PUFA (cis linoleic acid).
Fatty Acids and Human Health Benefits
A chickpea-based diet provides significant EFAs; the consumption of unsaturated vs. saturated fats can help maintain healthy cholesterol levels and reduce obesity and diabetic conditions (Kaur and Prasad, 2021). Furthermore, the presence of ALA in a chickpea-based diet reduces angiotensin-converting enzyme inhibition, which contributes to antihypertensive effects (Ogawa et al., 2009; Kaur and Prasad, 2021). Once EFAs are ingested, LA is metabolized to arachidonic acid (AA, an ω-6 EFA). In contrast, ALA is metabolized into eicosapentaenoic acid (EPA, an ω-3 EFA) and docosahexadecaenoic acid (DHA, an ω-3 EFA). AA and EPA undergo further biosynthesis to prostanoids and leukotrienes (de Caterina et al., 2007). These metabolites have several beneficial physiological effects on humans (Singh, 2005). Metabolites with an ω-6 origin enhance platelet aggregation, while those of ω-3 origin are anti-inflammatory (Singh, 2005). The ω-6/ω-3 fatty acid ratio is an important indicator of the impact of EFAs on human health (Simopoulos, 2002). This ratio is a disease-controlling parameter, where the optimum range is 1–4:1 or 1–5:1 (Simopoulos, 2002, 2006; Singh, 2005). This value ranges from 1–2:1 for optimum health benefits for combating obesity (Simopoulos, 2016). However, in Western countries, this value ranges from 15–16.7:1 due to the low levels of ω-3 fatty acids in diets and comparatively high proportions of LA consumption (Simopoulos, 2002). Yet, no studies regarding the true impact of chickpea on this disease controlling parameter (ω-6/ω-3 ratio) and human metabolism have been published.
Chickpea based diet has a positive effect on diabetes and obesity. Adiponectin is a hormone that prevents type two diabetes and atherosclerosis (Achari and Jain, 2017). A randomized cross-over clinical trial with diabetic patients (n=32) served with a chickpea diet (substituting two servings of red meat) increased levels of adiponectin in all patients (Mirmiran et al., 2019; Acevedo Martinez et al., 2021). Additionally, a study with diabetic rats has demonstrated reduced blood glucose and triglyceride levels upon feeding 400mg/kg of aqueous and methanol-based doses of chickpea diets (Yagi and Yagi, 2018). Another clinical study (n=30; men =17 and women=13) reported that body weights, systolic blood pressure, low-density lipoprotein (LDL), high-density lipoprotein (HDL), and total cholesterol reduced with a diet rich in chickpea and other legumes (Gupta et al., 2017). The above changes were significantly comparative to a diet restricted with legumes (Gupta et al., 2017). The effects of chickpea on obesity have been further studied using rats for 8months. Their study has included a fatty diet as control and control with 10% (w/w) chickpea. The results indicated a 35% increment in HDL whereas a 23% decrement in LDL with an overall 30% reduction in LDL/HDL ratio (Gupta et al., 2017). The efficacy of a chickpea-based diet on diabetes and obesity needs further investigation with extensive clinical studies for the long term. Few studies indicated that nutritional responses in pulses may have been due to its high levels of low digestible carbohydrates, proteins, micronutrients, and low in anti-nutrients such as phytic acid, amylase inhibitors and lectins (Thavarajah and Thavarajah, 2012; Gupta et al., 2017).
The Impact of Food Processing on Fatty Acids
The fatty acid composition of chickpeas is sensitive to food processing. Cooking can increase the fat content in both kabuli and desi varieties (Wang et al., 2010), but pressure cooking can reduce the levels of the four main fatty acids in chickpea flour (Rajni et al., 2012; Table 3). Furthermore, food processing affects the quality and quantity of chickpea EFAs, as unsaturated fatty acids are directly exposed to oxygen and other reactants leading to auto-oxidation (Damodaran and Parkin, 2017). In particular, PUFAs are highly susceptible to auto-oxidation because they have more double bonds, any one of which could react with oxygen radicals (Damodaran and Parkin, 2017). Alkyl radicals with a PUFA origin are the major reactants that initiate PUFA depletion. High-temperature conditions in food processing could further increase these food quality-degrading reactions. Heat can significantly decompose the radicals formed (hydroperoxyl radicals) and multiply PUFA depletion (Damodaran and Parkin, 2017). The alterations depicted in Table 3 result from such chemical changes while cooking (Rajni et al., 2012; Damodaran and Parkin, 2017). The presence of certain minerals (especially Fe) and isoenzymes such as lipoxygenase in raw chickpea (Halliwell and Gutteridge, 1990; Sanz et al., 1992; Girotti, 1998; Damodaran and Parkin, 2017) may catalyze EFA depletion during storage. Lipoxygenase mainly contributes to depleting ALA and LA, initiating hydroperoxide formation (Damodaran and Parkin, 2017). As a result, storage conditions must inhibit lipoxygenase in the chickpea to preserve the food quality and enhance the shelf life. Another impact of auto-oxidation is forming volatile aldehydes and unsaturated by-products with rearranged double bonds (trans fats; Damodaran and Parkin, 2017). Trans fat formation from PUFAs could occur due to unsaturated double bond cleavage and rearranging during higher temperature food processing. Volatile compounds (aldehydes) formed due to storage and food processing deplete the quality and aroma, leading to rancidity (Damodaran and Parkin, 2017), while trans fats are detrimental to human health. However, no studies related to rancidity and trans fats originating from chickpea foods are available in the literature. Future studies are required to understand these fatty acid concentrations after processing, cooking, and storage.
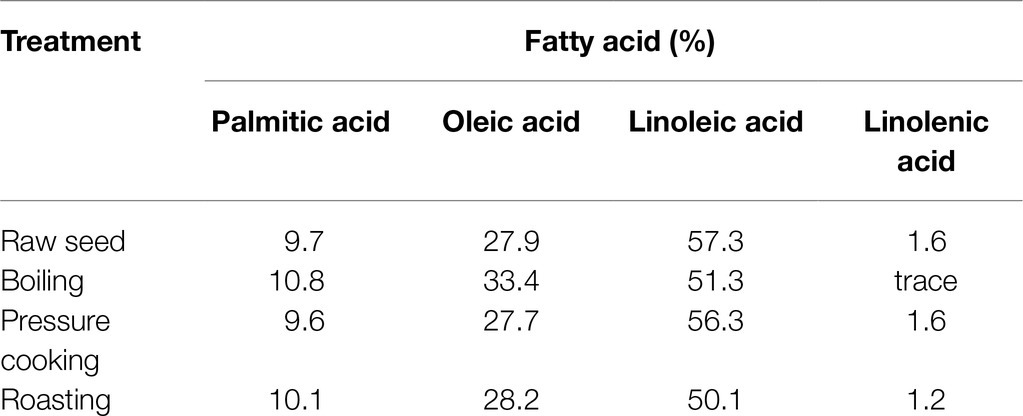
Table 3. Fatty acid composition of raw and processed chickpea (Rajni et al., 2012).
Fatty Acid Analysis
Fatty acid extraction procedures and analytical instrumentation are essential for the accurate quantification of EFAs. Fatty acid profiles are measured using gas chromatography (GC) paired with a flame ionization detector (FID) or a mass spectrometer (MS; Laakso and Hiltunen, 2009; Chiu and Kuo, 2020). The FID is a universal detector, which creates signals for organic molecules (due to C-H bond cleavage), but fails in molecular identification (qualitative analysis; Skoog et al., 2018). Accordingly, MS is the most superior detection method for qualitative and quantitative analysis of fatty acids by GC. The advantage of a mass-based detector is the ability to run a selective ion monitoring (SIM) analysis for all analytes (Sleeman and Carter, 1997). The SIM mode enables quantification irrespective of two analytes having close retention times. FID detection requires tedious efforts in terms of temperature programming to obtain entirely resolved chromatograms with minimal errors. Therefore, MS with SIM is the most appropriate and convenient method for fatty acid quantification and identification (Sleeman and Carter, 1997; Dodds et al., 2005). However, a major drawback of GC–MS techniques is the analysis time, cost, and labor. A short analysis time with high throughput is ideal for collecting data to screen fatty acids in breeding populations before advancing to varietal development stages.
Fourier-transform infrared (FTIR) spectroscopy measures the infrared spectrum of absorption or emission of a solid, liquid, or gas (Sindhu et al., 2015) and is a suitable technique to reduce the analytical time, cost, and labor but preserve high throughput. FTIR data models validated with GC–MS methods are robust tools to quantify fatty acids for high-throughput plant breeding research (Gómez-Caravaca et al., 2013). Non-destructive sample preparation and the application of hand-held FTIR devices in the field will enhance future chickpea breeding to select for fatty acid-rich accessions without the need for an analytical laboratory.
The electromagnetic spectrum’s IR region is less energetic compared to the ultraviolet (UV)-visible region. Consequently, IR energy induces molecular vibrations rather than electronic excitations. The midsection of the IR (mid-IR, MIR) region has the most fundamental resonant frequencies that cause distinct molecular vibrations (Skoog et al., 2018). Consequently, FTIR utilizes MIR energy to generate signals based on molecular vibrations for qualitative and quantitative analysis. The working window of FTIR is 500–4,000cm−1, wherein signals due to functional group vibrations occur mainly between 1,500 and 4,000cm−1 (functional group region; Lohumi et al., 2015). For fatty acids (Figure 3), the C=O (carbonyl), C=C (unsaturated double bonds), and C-H bonds undergo distinct oscillations in the functional group region. Therefore, based on the signal intensities (C=C and C-H signal ratios), fatty acids can be characterized by the degree of saturation and chain length, followed by quantification (Meiklejohn et al., 1957; Rabelo et al., 2015). Carbonyl stretching (~1742–1750cm−1) is one of the most distinct signals and is strongly applicable to fatty acid quantification (Yang et al., 2005; Rabelo et al., 2015). FTIR is a powerful tool for fatty acid analysis. NIR (near-IR spectroscopy) is another common tool in plant breeding programs used in parallel with FTIR technology (Downey, 1999). NIR technology is also available with added Fourier-transformation technology (i.e., FTNIR; Skoog et al., 2018). The primary difference between FTNIR and FTIR techniques is the working window. The FTNIR range (4000–12,820cm−1) is beyond the MIR range employed in FTIR, and the molecular vibrations occur as overtones and combined bands in the NIR range (Yang et al., 2005; Lohumi et al., 2015; Rabelo et al., 2015). FTIR and FTNIR have both been used for total fat analysis in food and seed composition analysis, and each method has advantages and disadvantages. Generally, calibration models for total fat based on FTNIR are better than those based on FTIR (Yang et al., 2005; Oliveira et al., 2006); however, FTIR is more informative than FTNIR due to its well-resolved spectral signals and because it provides better qualitative insight (Lohumi et al., 2015). In addition to fat analysis, NIR spectroscopy has been used in routine seed composition analysis for moisture, protein, starch, kernel hardness, color, and seed viability (William and Norris, 2001; Kusumaningrum et al., 2018; Skoog et al., 2018). FTNIR spectroscopy fits well with quantitative measurements of compounds with functional groups containing C-H, N-H, and O-H bonds based on NIR vibrational overtones (Skoog et al., 2018). Furthermore, the qualitative identification of functional groups using NIR is not optimal due to low resolution (William and Norris, 2001). Overall, the FTIR technique is unique and accurate with good resolution as a high-throughput tool to measure individual nutritional trails with low concentrations.
Breeding Approaches
Current chickpea breeding is mainly focused on grain yield, disease resistance, and nutritional quality traits, including protein, minerals, prebiotic carbohydrates, and environmental stresses (Wang et al., 2017; Vandemark et al., 2018, 2020). Seed yield can be positively or negatively correlated with chickpea agronomic traits. For example, Toker (2009) shows chickpea seed yield is positively correlated with biomass (r=0.975), harvest index (r=0.935), plant height (r=0.853), number of branches (r=0.797), and pods per plant (r=0.675) but negatively correlated with seed weight (r=−0.660) and ascochyta blight infection (r=−0.872). Wang et al. (2017) show positive correlations between seed protein concentration, plant height, and days of maturity and negative correlations between seed protein concentration, grain yield, and seed size. The concentrations of minerals, including K, P, and Zn, in chickpea seeds are influenced by genotype, location, and genotype×location interaction (Vandemark et al., 2018). Chickpea prebiotic carbohydrate concentrations vary across location, year, and genotype (Vandemark et al., 2020). Chickpea grain yield is negatively correlated with several prebiotic carbohydrates, including verbose (r=−0.80), stachyose (r=−0.77), sorbitol (r=−0.66), and mannitol (r=−0.65; G. Vandemark et al., 2020). Overall, grain yield is negatively correlated with most nutritional traits, including protein content, certain prebiotic carbohydrates, and minerals (Vandemark et al., 2018, 2020).
Heat, drought, and cold stresses are the common abiotic stresses affecting chickpea production worldwide (Jha et al., 2014). Plant lipids are linked to increased cold and heat tolerance in food crops. Fats alleviate environmental stresses by changing their PUFA composition in chloroplast lipids (Nelson and Cox, 2008). Drought stress generally increases LA and decreases ALA concentrations in response to desaturase enzymes (Yordanov et al., 2000). Lipids, including phospholipids and glycolipids, help chickpea plants withstand cold stress during the winter (Vigh et al., 1998). Desaturation of fatty acids is positively correlated with preventing cell lysis at colder temperatures (Bakht et al., 2006; Shah et al., 2013). The increase in double bonds in PUFA chains contributes to plant cell membrane fluidity, increasing cold tolerance due to freezing point depression (Vigh et al., 1998). Increased ALA and reduced LA levels in chickpea leaves during cold stress indicate fatty acid desaturation at low temperatures (Bakht et al., 2006). Higher double bond indices (DBI) in extracted leaf fats at negative LT50 (lethal temperatures) values indicate higher levels of unsaturated fats at lower temperatures (a significant negative correlation, r<0; Bakht et al., 2006; Figure 4). Genomic and gene-editing technology may enhance PUFA desaturation and accelerate breeding efforts to develop chickpea cultivars resistant to cold stress (Jaglo-Ottosen et al., 1998; Gilmour et al., 2000; Bakht et al., 2006). PUFA-induced mutations in chickpea have revealed higher PUFA (LA) content leads to improved cold stress tolerance (Shah et al., 2013). Mutant desi genotypes (CM72/02 and CM137-01) and mutant genotypes of desi×kabuli introgression can also tolerate sustained cold stress (Shah et al., 2013).
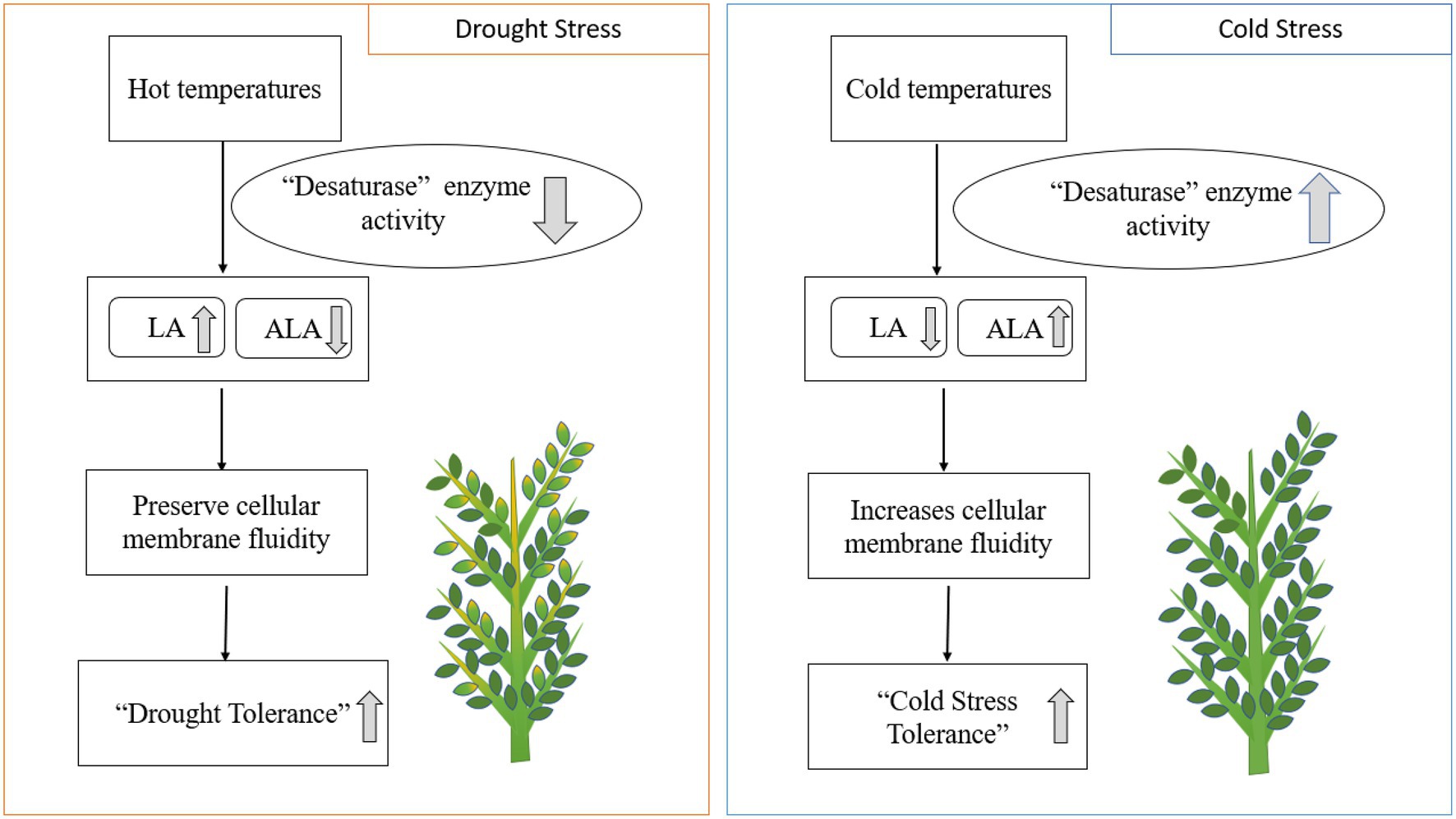
Figure 4. Drought and cold stresses impact enzymatic activity and EFA composition in plants. LA; linoleic acid. ALA; alpha-linolenic acid.
Integrating traditional breeding and biotechnology approaches would benefit the development of chickpea cultivars resilient to climate change. Planting time and growing conditions also affect fatty acid composition in chickpea seeds, with OA and LA concentrations higher in chickpea planted in the fall than in the spring (Gül et al., 2008). Interactions between genotype and planting date can significantly affect the concentration of α and β tocopherols and palmitic acid, OA, and LA concentrations; ALA concentration is positively correlated with LA concentration and negatively correlated with OA and tocopherol concentrations (Gül et al., 2008). Nine candidate genes related to fats have been identified in soybean using quantitative trait loci (QTL) mapping (Nian and Cheng, 2020). A single gene associated with a lipid synthesizing and storage enzyme named diacylglycerol O-acyltransferase has also been identified in chickpea (Verma et al., 2015). Detailed QTL mapping studies on candidate genes associated with essential fatty acids in chickpea have not been reported.
Conclusion and Future Prospects
Chickpea is a highly nutritious pulse crop rich in protein, prebiotic carbohydrates, fat, and a range of micronutrients. Chickpea is a rich source of EFAs, phytosterols, TAGs, and phospholipids. TAGs are the most dominant neutral lipid in chickpea. PUFAs, MUFAs, and SFAs are esterified within the lipids and bind to TAG’s glycerol end or a phospholipid. The most dominant PUFA in chickpea is LA, followed by OA (MUFA) and ALA. LA is an ω-6 EFA, whereas ALA is an ω-3 EFA. The consumption of diets with an ω-6/ω-3 ratio of 4 to 5 is recommended for better human health. The ratio of EFAs from a chickpea diet and related human health benefits have yet to be studied using large clinical trials. EFA traits have not been extensively studied in chickpea breeding. Optimizing EFA levels in chickpea should be feasible by applying the genetic and transgenic approaches followed in chickpea biofortification for micronutrients. FTIR and FTNIR techniques should be incorporated into breeding programs to screen breeding populations; FTIR within the functional group region will assist qualitative and quantitative fatty acid analysis. Future genome-wide association studies are needed to develop marker-assisted breeding approaches for improving chickpea nutritional traits. Genome mapping studies could support the identification of corresponding QTLs and candidate genes associated with fatty acid biosynthesis (Figure 5). In general, chickpea produces LA (2.87g/100g) and ALA (0.112g/100g), but the cultivar information is not available (USDA, 2021). So far, human clinical studies have been published to confirm the impact of the prevailing chickpea EFA composition on human health. Percent recommended daily allowance (%RDA) for LA (ω-6 fatty acid) is not published; however, the %RDA of ALA (ω-3 fatty acid) for adult men and women is 1.6 and 1.1g, respectively (Hjalmarsdottir, 2019). Future chickpea breeding strategies should address the safe, adequate increase of these essential fatty acids for human health. Future genomics and plant breeding advancements will also enhance chickpea’s EFA concentrations and other nutritional traits and improve human health.
Author Contributions
AU is a doctoral graduate student working on this project with DT; they created the hypothesis, objectives, outline the draft, and wrote the manuscript. LT, PT, WB, ES, and GV edited and added discipline-specific feedback. All authors contributed to the article and approved the submitted version.
Funding
This project was supported by the American people via the Feed the Future Innovation Lab for Crop Improvement through the United States Agency for International Development (USAID, award no 7200AA19LE00005/subaward no 89915-11295 awarded to DT); the Pulse Health Initiative (USDA-ARS awarded to DT and GV); and the USDA National Institute of Food and Agriculture, [Hatch] project [1022664] awarded to DT. Its contents are solely the responsibility of the authors and do not necessarily represent the official views of the USDA, USAID, or the United States Government.
Conflict of Interest
The authors declare that the research was conducted in the absence of any commercial or financial relationships that could be construed as a potential conflict of interest.
Publisher’s Note
All claims expressed in this article are solely those of the authors and do not necessarily represent those of their affiliated organizations, or those of the publisher, the editors and the reviewers. Any product that may be evaluated in this article, or claim that may be made by its manufacturer, is not guaranteed or endorsed by the publisher.
References
Acevedo Martinez, K. A., Yang, M. M., and Gonzalez de Mejia, E. (2021). Technological properties of chickpea (Cicer arietinum): production of snacks and health benefits related to type-2 diabetes. Compr. Rev. Food Sci. Food Saf. 20, 3762–3787. doi: 10.1111/1541-4337.12762
Achari, A. E., and Jain, S. K. (2017). Adiponectin, a therapeutic target for obesity, diabetes, and endothelial dysfunction. Int. J. Mol. Sci. 18. doi: 10.3390/ijms18061321
Bakht, J., Bano, A., and Dominy, P. (2006). The role of abscisic acid and low temperature in chickpea (Cicer arietinum) cold tolerance. II. Effects on plasma membrane structure and function. J. Exp. Bot. 57, 3707–3715. doi: 10.1093/jxb/erl120
Birol, E., Meenakshi, J. V., Oparinde, A., Perez, S., and Tomlins, K. (2015). Developing country consumers’ acceptance of biofortified foods: a synthesis. Food Secur. 7, 555–568. doi: 10.1007/s12571-015-0464-7
Bouis, H. E., and Saltzman, A. (2017). Improving nutrition through biofortification: A review of evidence from HarvestPlus, 2003 through 2016. Glob. Food Sec. 12, 49–58. doi: 10.1016/j.gfs.2017.01.009
Cagliari, A., Margis, R., Dos Santos Maraschin, F., Turchetto-Zolet, A. C., Loss, G., and Margis-Pinheiro, M. (2011). Biosynthesis of triacylglycerols (TAGs) in plants and algae. Int. J. Plant Biol. 2, 40–52. doi: 10.4081/pb.2011.e10
Chiu, H. H., and Kuo, C. H. (2020). Gas chromatography-mass spectrometry-based analytical strategies for fatty acid analysis in biological samples. J. Food Drug Anal. 28, 60–73. doi: 10.1016/j.jfda.2019.10.003
Clemente, A., Vioque, J., Bautistab, J., and Millin, F. (1998). Polar lipids of defatted chickpea (Cicer arietinum L.) flour and protein isolates. Food Chem. 63, 357–361. doi: 10.1016/S0308-8146(98)00015-6
Damodaran, S., and Parkin, K. L. (2017). “Fennema’s food chemistry,” in Fennema’s Food Chemistry. 5th Edn. eds. S. Damodaran and K. L. Parkin (Boca Raton: CRC Press), 205–216. doi: 10.1201/9781315372914
Caterina, R.de, Bertolotto, A., Madonna, R., and Schmidt, E. B. (2007). N-3 fatty acids in the treatment of. Biabetes Care, 30, 1012–1026. doi: 10.2337/dc06-1332.R.D.C.
Di Pasquale, M. G. (2009). The essentials of essential fatty acids. J. Dietary Suppl. 6, 143–161. doi: 10.1080/19390210902861841
Dodds, E. D., McCoy, M. R., Rea, L. D., and Kennish, J. M. (2005). Gas chromatographic quantification of fatty acid methyl esters: flame ionization detection vs. electron impact mass spectrometry. Lipids 40, 419–428. doi: 10.1007/s11745-006-1399-8
Downey, G. (1999). Food and food ingredient authentication by mid-infrared spectroscopy and chemometrics. Eur. J. Pharmacol. 367, 418–424. doi: 10.1016/S0165-9936(98)00042-9
Fahy, E., Subramaniam, S., Brown, H. A., Glass, C. K., Merrill, A. H., Murphy, R. C., et al. (2005). A comprehensive classification system for lipids. J. Lipid Res. 46, 839–861. doi: 10.1194/jlr.E400004-JLR200
FAOSTAT. (2020). http://www.fao.org/faostat/en/#data/QC
Garcia-Casal, M. N., Peña-Rosas, J. P., Giyose, B., Bechoff, A., Blancquaert, D., Birol, E., et al. (2017). Staple crops biofortified with increased vitamins and minerals: considerations for a public health strategy. Ann. N. Y. Acad. Sci. 1390, 3–13. doi: 10.1111/nyas.13293
Gilmour, S. J., Sebolt, A. M., Salazar, M. P., Everard, J. D., and Thomashow, M. F. (2000). Overexpression of the arabidopsis CBF3 transcriptional activator mimics multiple biochemical changes associated with cold acclimation. Plant Physiol. 124, 1854–1865. doi: 10.1104/pp.124.4.1854
Girotti, A. W. (1998). Lipid hydroperoxide generation, turnover, and effector action in biological systems. J. Lipid Res. 39, 1529–1542. doi: 10.1016/S0022-2275(20)32182-9
Global nutrition report. (2018). https://globalnutritionreport.org/reports/global-nutrition-report-2018/executive-summary/
Gómez-Caravaca, A. M., Maggio, R. M., Verardo, V., Cichelli, A., and Cerretani, L. (2013). Fourier transform infrared spectroscopy-partial least squares (FTIR-PLS) coupled procedure application for the evaluation of fly attack on olive oil quality. LWT Food Sci. Technol. 50, 153–159. doi: 10.1016/j.lwt.2012.06.007
Gül, M. K., Egesel, C. Ö., and Turhan, H. (2008). The effects of planting time on fatty acids and tocopherols in chickpea. Eur. Food Res. Technol. 226, 517–522. doi: 10.1007/s00217-007-0564-5
Gupta, R. K., Gupta, K., Sharma, A., Das, M., Ansari, I. A., and Dwivedi, P. D. (2017). Health risks & benefits of chickpea (Cicer arietinum) consumption. J. Agric. Food Chem. 65, 6–27. doi: 10.1021/acs.jafc.6b02629
Halliwell, B., and Gutteridge, J. M. C. (1990). Role of free radicals and catalytic metal ions in human disease: An overview. Methods Enzymol. 186, 1–88. doi: 10.1016/0076-6879(90)86093-B
Harvest Plus. (2021). It all Starts with a Seed. Available at: https://www.harvestplus.org/what-we-do/crops (Accessed July 01, 2021).
Hjalmarsdottir, F. (2019). How Much Omega-3 Should You Take per Day? https://www.healthline.com/nutrition/how-much-omega-3 (Accessed August 30, 2021).
Hussain, M., Mehboob, N., Naveed, M., Shehzadi, K., and Yasir, T. A. (2020). Optimizing boron seed coating level and boron-tolerant bacteria for improving yield and biofortification of chickpea. J. Soil Sci. Plant Nutr. 20, 2471–2478. doi: 10.1007/s42729-020-00313-y
Innis, S. M. (1991). Essential fatty acids in growth and development. Prog. Lipid Res. 30, 39–103. doi: 10.1016/0163-7827(91)90006-Q
Jaglo-Ottosen, K. R., Gilmour, S. J., Zarka, D. G., Schabenberger, O., and Thomashow, M. F. (1998). Arabidopsis CBF1 overexpression induces COR genes and enhances freezing tolerance. Science 280, 104–106. doi: 10.1126/science.280.5360.104
Jha, U. C., Chaturvedi, S. K., Bohra, A., Basu, P. S., Khan, M. S., and Barh, D. (2014). Abiotic stresses, constraints and improvement strategies in chickpea. Plant Breed. 133, 163–178. doi: 10.1111/pbr.12150
Johnson, N., Johnson, C. R., Thavarajah, P., Kumar, S., and Thavarajah, D. (2020). The roles and potential of lentil prebiotic carbohydrates in human and plant health. Plants People Planet 2019, 310–319. doi: 10.1002/ppp3.10103
Jukanti, A. K., Gaur, P. M., Gowda, C. L. L., and Chibbar, R. N. (2012). Nutritional quality and health benefits of chickpea (Cicer arietinum L.): A review. Br. J. Nutr. 108(Suppl. 1), S11–S26. doi: 10.1017/S0007114512000797
Kaur, R., and Prasad, K. (2021). Technological, processing and nutritional aspects of chickpea (Cicer arietinum) – A review. Trends Food Sci. Technol. 109, 448–463. doi: 10.1016/j.tifs.2021.01.044
Kerem, Z., Lev-Yadun, S., Gopher, A., Weinberg, P., and Abbo, S. (2007). Chickpea domestication in the Neolithic Levant through the nutritional perspective. J. Archaeol. Sci. 34, 1289–1293. doi: 10.1016/j.jas.2006.10.025
Khalid, S., Asghar, H. N., Akhtar, M. J., Aslam, A., and Zahir, Z. A. (2015). Biofortification of iron in chickpea by plant growth promoting rhizobacteria. Pak. J. Bot. 47, 1191–1194.
Knights, E. J., and Hobson, K. B. (2016). “Chickpea overview,” in Reference Module in Food Science (2nd Ed., Vol. 1) (Elsevier Ltd.) doi: 10.1016/b978-0-08-100596-5.00035-4
Kumar, S., and Pandey, G. (2020). Biofortification of pulses and legumes to enhance nutrition. Heliyon 6, 4–9. doi: 10.1016/j.heliyon.2020.e03682
Kushwaha, P., Srivastava, R., Pandiyan, K., Singh, A., Chakdar, H., Kashyap, P. L., et al. (2021). Enhancement in plant growth and zinc biofortification of chickpea (Cicer arietinum L.) by Bacillus altitudinis. J. Soil Sci. Plant Nutr. 21, 922–935. doi: 10.1007/s42729-021-00411-5
Kusumaningrum, D., Lee, H., Lohumi, S., Mo, C., Kim, M. S., and Cho, B. K. (2018). Non-destructive technique for determining the viability of soybean (Glycine max) seeds using FT-NIR spectroscopy. J. Sci. Food Agric. 98, 1734–1742. doi: 10.1002/jsfa.8646
Laakso, T. S., and Hiltunen, I. L. (2009). Analysis of fatty acids by gas chromatography, and its relevance to research on health and nutrition. Anal. Chem. Acta 28, 40–57. doi: 10.1016/S0003-2670(02)00397-5
Lee, J. M., Lee, H., Kang, S., and Park, W. J. (2016). Fatty acid desaturases, polyunsaturated fatty acid regulation, and biotechnological advances. Nutrients 2015, 1–13. doi: 10.3390/nu8010023
Lohumi, S., Lee, S., Lee, H., and Cho, B. K. (2015). A review of vibrational spectroscopic techniques for the detection of food authenticity and adulteration. Trends Food Sci. Technol. 46, 85–98. doi: 10.1016/j.tifs.2015.08.003
Malik, K. A., and Maqbool, A. (2020). Transgenic crops for biofortification. Front. Sustainable Food Syst. 4, 1–15. doi: 10.3389/fsufs.2020.571402
Meiklejohn, R. A., Meyer, R. J., Aronovic, S. M., Schuette, H. A., and Meloch, V. W. (1957). Characterization of long-chain fatty acids by infrared spectroscopy. Anal. Chem. 29, 329–334. doi: 10.1021/ac60123a002
Merga, B., and Haji, J. (2019). Economic importance of chickpea: production, value, and world trade. Cogent Food Agric. 5:1615718. doi: 10.1080/23311932.2019.1615718
Michaelson, L. V., Napier, J. A., Molino, D., and Faure, J. (2016). Biochimica et biophysica acta plant sphingolipids: their importance in cellular organization and adaption ☆. BBA – Mol. Cell Biol. Lipids 1861, 1329–1335. doi: 10.1016/j.bbalip.2016.04.003
Mirmiran, P., Hosseini, S., Hosseinpour-Niazi, S., and Azizi, F. (2019). Legume consumption increase adiponectin concentrations among type 2 diabetic patients: A randomized crossover clinical trial. Endocrinología, Diabetes y Nutrición 66, 49–55. doi: 10.1016/j.endien.2018.07.011
Nelson, D. L., and Cox, M. M. (2008). “Lehninger principles of biochemistry 5th (fifth) edition,” in Biochemistry. 5th Edn. (ed.) A. Katherine. (New York, NY: W. H Freeman and Company).
Nian, H., and Cheng, Y. (2020). Identification and mapping of stable QTLs for seed oil and protein content in soybean [Glycine max (L.) Merr.]. J. Agric. Food Chem. 68, 6448–6460. doi: 10.1021/acs.jafc.0c01271
Ogawa, A., Suzuki, Y., Aoyama, T., and Takeuchi, H. (2009). Dietary alpha-linolenic acid inhibits angiotensin-converting enzyme activity and mrna expression levels in the aorta of spontaneously hypertensive rats. J. Oleo Sci. 58, 355–360. doi: 10.5650/jos.58.355
Oliveira, J. S., Montalvão, R., Daher, L., Suarez, P. A. Z., and Rubim, J. C. (2006). Determination of methyl ester contents in biodiesel blends by FTIR-ATR and FTNIR spectroscopies. Talanta 69, 1278–1284. doi: 10.1016/j.talanta.2006.01.002
Pal, V., Singh, G., and Dhaliwal, S. S. (2019). Agronomic biofortification of chickpea with zinc and iron through application of zinc and urea. Commun. Soil Sci. Plant Anal. 50, 1864–1877. doi: 10.1080/00103624.2019.1648490
Pal, V., Singh, G., and Dhaliwal, S. S. (2021). A new approach in agronomic biofortification for improving zinc and iron content in chickpea (Cicer arietinum L.) grain with simultaneous foliar application of zinc sulphate, ferrous sulphate and urea. J. Soil Sci. Plant Nutr. 21, 883–896. doi: 10.1007/s42729-021-00408-0
Peterbauer, T., and Richter, A. (2001). Biochemistry and physiology of Raffinose family oligosaccharides and galactosyl cyclitols in seeds biochemistry and physiology of raffinose family oligosaccharides and galactosyl cyclitols in seeds. Seed Sci. Res. 11, 185–197. doi: 10.1079/SSR200175
Poblaciones, M. J., Rodrigo, S., Santamaria, O., Chen, Y., and Mcgrath, S. P. (2014). Selenium accumulation and speciation in biofortified chickpea (Cicer arietinum L.) under Mediterranean conditions. J. Sci. Food Agric. 94, 1101–1106. doi: 10.1002/jsfa.6372
Rabelo, S. N., Ferraz, V. P., Oliveira, L. S., and Franca, A. S. (2015). FTIR analysis for quantification of fatty acid methyl esters in biodiesel produced by microwave-assisted transesterification. Int. J. Environ. Sci. Dev. 6, 964–969. doi: 10.7763/IJESD.2015.V6.730
Rajni, M., Nagi, H. P. S. P, S., and Sharma, S. (2012). Effect of processing on chemical composition and antinutritional factors in chickpea flour. J. Food Sci. Eng. 2, 180–186. doi: 10.17265/2159-5828/2012.03.008
Ray, H., Bett, K., Tar’an, B., Vandenberg, A., Thavarajah, D., and Warkentin, T. (2014). Mineral micronutrient content of cultivars of field pea, chickpea, common bean, and lentil grown in Saskatchewan, Canada. Crop Sci. 54, 1698–1708. doi: 10.2135/cropsci2013.08.0568
Ritchie, H., and Roser, M. (2017). Micronutrient Deficiency. Our World in Data. https://ourworldindata.org/micronutrient-deficiency (Accessed April 21, 2021).
Roberfroid, M., Gibson, G. R., Hoyles, L., McCartney, A. L., Rastall, R., Rowland, I., et al. (2009). Prebiotic effects: metabolic and health benefits. Br. J. Nutr. 101, 1–63. doi: 10.1017/S0007114509990511
Roorkiwal, M., Pandey, S., Thavarajah, D., and Hemalatha, R. (2021). Molecular mechanisms and biochemical pathways for micronutrient acquisition and storage in legumes to support biofortification for nutritional security. Front. Plant Sci. 12:682842. doi: 10.3389/fpls.2021.682842
Rustan, A. C. (2005). Fatty acids: structures and properties. Encyclopedia of Life Sciences (John Wiley & Sons), 1–7. doi: 10.1038/npg.els.000389.
Sanz, L. C., Perez, A. G., Rios, J. J., and Olias, J. M. (1992). Physico-chemical properties of chickpea lipoxygenases. Phytochemistry 31, 3381–3384. doi: 10.1016/0031-9422(92)83690-Z
Shah, T. M., Iqbal, Z., Asi, M. R., and Atta, B. M. (2013). Induced genetic variability for fatty acids and oil contents in chickpea (Cicer arietinum). Int. J. Agric. Biol. 15, 419–426.
Shivay, Y. S., Prasad, R., and Pal, M. (2015). Effects of source and method of zinc application on yield, zinc biofortification of grain, and Zn uptake and use efficiency in chickpea (Cicer arietinum L.). Commun. Soil Sci. Plant Anal. 46, 2191–2200. doi: 10.1080/00103624.2015.1069320
Simopoulos, A. P. (2002). The importance of the ratio of omega-6/omega-3 essential fatty acids. Biomed. Pharmacother. 56, 365–379. doi: 10.1016/S0753-3322(02)00253-6
Simopoulos, A. P. (2006). Evolutionary aspects of diet, the omega-6/omega-3 ratio and genetic variation: nutritional implications for chronic diseases. Biomed. Pharmacother. 60, 502–507. doi: 10.1016/j.biopha.2006.07.080
Simopoulos, A. P. (2016). An increase in the Omega-6/Omega-3 fatty acid ratio increases the risk for obesity. Nutrients 8, 1–17. doi: 10.3390/nu8030128
Sindhu, R., Binod, P., and Pandey, A. (2015). “Microbial poly-3-hydroxybutyrate and related copolymers,” in Industrial Biorefineries and White Biotechnology (Elsevier B.V)
Singh, M. (2005). Essential fatty acids, DHA human brain. Indian J. Pediatr. 72, 239–242. doi: 10.1007/BF02859265
Singh, S., Sivakumar, K. B., and Arora, A. (2021). Genetic Studies for Biofortification Traits in Chickpea Genetic Studies for Biofortification Traits in Chickpea. J. Food Legumes 34, 17–20.
Skoog, D. A., Holler, F. J., and Crouch, S. R. (2018). Principles of Instrumental Analysis. Boston: Cengage Learning.
Sleeman, R., and Carter, J. F. (1997). Selected ion monitoring. Spectroscopy 12, 423–430. doi: 10.1007/978-3-662-48986-4_301499
Sreeramaiah, H., Platel, K., and Srinivasan, K. (2007). Zinc and iron contents and their bioaccessibility in cereals and pulses consumed in India. Food Chem. 102, 1328–1336. doi: 10.1016/j.foodchem.2006.07.015
Tan, G. Z. H., Das Bhowmik, S. S., Hoang, T. M. L., Karbaschi, M. R., Long, H., Cheng, A., et al. (2018). Investigation of baseline iron levels in australian chickpea and evaluation of a transgenic biofortification approach. Front. Plant Sci. 9:788. doi: 10.3389/fpls.2018.00788
Thavarajah, D., and Thavarajah, P. (2012). Evaluation of chickpea (Cicer arietinum L.) micronutrient composition: biofortification opportunities to combat global micronutrient malnutrition. Food Res. Int. 49, 99–104. doi: 10.1016/j.foodres.2012.08.007
Thavarajah, D., Thavarajah, P., Gupta, D., and Sen, (2014). “Pulses biofortification,” in Genomic Era: Multidisciplinary Opportunities and Challenges BT - Legumes in the Omic Era. eds. S. Gupta, N. Nadarajan, and D. S. Gupta (New York: Springer), 207–220.
Toker, C. (2009). A note on the evolution of kabuli chickpeas as shown by induced mutations in Cicer reticulatum Ladizinsky. Genet. Resour. Crop. Evol. 56, 7–12. doi: 10.1007/s10722-008-9336-8
United Nations. (2021a). End Hunger, Achieve Food Security and Improved Nutrition and Promote Sustainable Agriculture. https://sdgs.un.org/topics/food-security-and-nutrition-and-sustainable-agriculture (Accessed August 30, 2021).
United Nations. (2021b). Food security and nutrition and sustainable agriculture. https://sdgs.un.org/goals/goal2 (Accessed August 30, 2021).
Upadhyaya, H. D., Bajaj, D., Narnoliya, L., Das, S., Kumar, V., Gowda, C. L. L., et al. (2016). Genome-wide scans for delineation of candidate genes regulating seed-protein content in chickpea. Front. Plant Sci. 7, 1–13. doi: 10.3389/fpls.2016.00302
USDA. (2021). https://fdc.nal.usda.gov/fdc-app.html#/food-details/174288/nutrients (Accessed August 30, 2021).
Vandemark, G. J., Grusak, M. A., and McGee, R. J. (2018). Mineral concentrations of chickpea and lentil cultivars and breeding lines grown in the U.S. Pacific northwest. Crop J. 6, 253–262. doi: 10.1016/j.cj.2017.12.003
Vandemark, G., Thavarajah, S., Siva, N., and Thavarajah, D. (2020). Genotype and environment effects on prebiotic carbohydrate concentrations in Kabuli chickpea cultivars and breeding lines grown in the U.S. Pacific northwest. Front. Plant Sci. 11, 1–8. doi: 10.3389/fpls.2020.00112
Varshney, R. K., Song, C., Saxena, R. K., Azam, S., Yu, S., Sharpe, A. G., et al. (2013). Draft genome sequence of chickpea (Cicer arietinum) provides a resource for trait improvement. Nat. Biotechnol. 31, 240–246. doi: 10.1038/nbt.2491
Verma, S., Gupta, S., Bandhiwal, N., Kumar, T., Bharadwaj, C., and Bhatia, S. (2015). High-density linkage map construction and mapping of seed trait QTLs in chickpea (Cicer arietinum L.) using genotyping-by-sequencing (GBS). Sci. Rep. 5:17512. doi: 10.1038/srep17512
Vigh, L., Maresca, B., and Harwood, J. L. (1998). Does the membrane’s physical state control the expression of heat shock and other genes? Trends Biochem. Sci. 23, 369–374. doi: 10.1016/S0968-0004(98)01279-1
Wang, K., and Daun, N. (2004). The Chemical Composition and Nutritive Value of Canadian Pulses. Canadian Grain Commision. https://www.yumpu.com/en/document/read/15342392/the-chemical-composition-and-nutritive-value-of-pulse-canada (Accessed January 25, 2021).
Wang, R., Gangola, M. P., Jaiswal, S., Gaur, P. M., Båga, M., and Chibbar, R. N. (2017). Genotype, environment and their interaction influence seed quality traits in chickpea (Cicer arietinum L.). J. Food Compos. Anal. 63, 21–27. doi: 10.1016/j.jfca.2017.07.025
Wang, N., Hatcher, D. W., Tyler, R. T., Toews, R., and Gawalko, E. J. (2010). Effect of cooking on the composition of beans (Phaseolus vulgaris L.) and chickpeas (Cicer arietinum L.). Food Res. Int. 43, 589–594. doi: 10.1016/j.foodres.2009.07.012
Warude, D., Joshi, K., and Harsulkar, A. (2006). Polyunsaturated fatty acids: biotechnology. Crit. Rev. Biotechnol. 26, 83–93. doi: 10.1080/07388550600697479
Welch, R. M., and Graham, R. D. (2004). Breeding for micronutrients in staple food crops from a human nutrition perspective. J. Exp. Bot. 55, 353–364. doi: 10.1093/jxb/erh064
Weselake, R., Zou, J., and Taylor, D. (2021). AOAC. https://lipidlibrary.aocs.org/chemistry/physics/plant-lipid/plant-triacylglycerol-synthesis#:~:text=In higher plants%2C triacylglycerol is,the early stages of germination. (Accessed March 10, 2021).
White, P. J., and Broadley, M. R. (2005). Biofortifying crops with essential mineral elements. Trends Plant Sci. 10, 586–593. doi: 10.1016/j.tplants.2005.10.001
William, P., and Norris, K. (2001). Near – Infrared Technology in the Agriculture and Food Industries. 2nd Edn. (University of Wisconsin–Madison: American Assocaition of cereal chemists).
Yadav, S. S. (2007). Chickpea breeding and management. (Ed.) (B. Yadav, S. S. Redden, R. J. Chen, and W. Sharma) CABI ebooks.
Yagi, S. M., and Yagi, A. I. (2018). Traditional medicinal plants used for the treatment of diabetes in the Sudan: review. Afr. J. Pharm. Pharmacol 12, 27–40. doi: 10.5897/AJPP2017.4878
Yang, H., Irudayaraj, J., and Paradkar, M. M. (2005). Discriminant analysis of edible oils and fats by FTIR, FT-NIR and FT-Raman spectroscopy. Food Chem. 93, 25–32. doi: 10.1016/j.foodchem.2004.08.039
Yordanov, I., Velikova, V., and Tsonev, T. (2000). Plant responses to drought,accimilation,and stress tolerance. Photosynthetica 38, 171–186. doi: 10.1023/A:1007201411474
Zia-Ul-Haq, M., Ahmad, M., Iqbal, S., Ahmad, S., and Ali, H. (2007). Characterization and Compositional Studies of Oil from Seeds of Desi Chickpea (Cicer arietinum L.) Cultivars Grown in Pakistan. J. Am. Oil Chem. Soc. 84, 1143–1148. doi: 10.1007/s11746-007-1136-3
Keywords: chickpea (Cicer arietinum L.), essential fatty acids, biofortification, nutritional breeding, fourier transform infrared spectroscopy
Citation: Madurapperumage A, Tang L, Thavarajah P, Bridges W, Shipe E, Vandemark G and Thavarajah D (2021) Chickpea (Cicer arietinum L.) as a Source of Essential Fatty Acids – A Biofortification Approach. Front. Plant Sci. 12:734980. doi: 10.3389/fpls.2021.734980
Edited by:
Aditya Pratap, Indian Institute of Pulses Research (ICAR), IndiaReviewed by:
Muhammad Imtiaz, Huazhong Agricultural University, ChinaGyan P. Mishra, Indian Agricultural Research Institute (ICAR), India
Copyright © 2021 Madurapperumage, Tang, Thavarajah, Bridges, Shipe, Vandemark and Thavarajah. This is an open-access article distributed under the terms of the Creative Commons Attribution License (CC BY). The use, distribution or reproduction in other forums is permitted, provided the original author(s) and the copyright owner(s) are credited and that the original publication in this journal is cited, in accordance with accepted academic practice. No use, distribution or reproduction is permitted which does not comply with these terms.
*Correspondence: Dil Thavarajah, ZHRoYXZhckBjbGVtc29uLmVkdQ==