- 1Department of Genetics, Ecology and Evolution, Federal University of Minas Gerais, Belo Horizonte, Brazil
- 2Department of Ecology and Evolutionary Biology, University of Michigan, Ann Arbor, MI, United States
Intraspecific genetic variation plays a fundamental role in maintaining the evolutionary potential of wild populations. Hence, the assessment of genetic diversity patterns becomes essential to guide biodiversity conservation policies, particularly for threatened species. To inform management strategies for conservation of Mimosa catharinensis – a narrow endemic, critically endangered plant species – we identified 1,497 unlinked SNP markers derived from a reduced representation sequencing method (i.e., double digest restriction site associated DNA sequencing, or ddRADseq). This set of molecular markers was employed to assess intrapopulation genetic parameters and the demographic history of one extremely small population of M. catharinensis (N=33) located in the Brazilian Atlantic Forest. Contrary to what is expected for narrow endemic and threatened species with small population sizes, we observed a moderate level of genetic diversity for M. catharinensis [uH E(0%missing data)=0.205, 95% CI (0.160, 0.250); uH E(30%missing data)=0.233, 95% CI (0.174, 0.292)]. Interestingly, M. catharinensis, which is a lianescent shrub with no indication of seed production for at least two decades, presented high levels of outcrossing [t (0%missing data)=0.883, SE±0.0483; t (30%missing data)=0.909, SE±0.011] and an apparent absence of inbreeding [F (0%missing data)=−0.145, 95% CI (−0.189, −0.101); F (30%missing data)=−0.105, 95% CI (−0.199, −0.011)]. However, the reconstruction of demographic history of M. catharinensis indicated that the population should be suffered a recent bottleneck. Our population genomic study tackles a central issue in evolution and conservation biology and we expect that it will be useful to help safeguard the remaining genetic diversity reported for this unique genetic resource.
Introduction
Differences in DNA sequences among individuals within a population represent its genetic diversity, a key component for the long-term survival of natural populations that is related to the maintenance of its evolutionary potential in the face of environmental change (e.g., Huenneke, 1991; Reed and Frankham, 2003; Spielman et al., 2004; Elegren and Galtier, 2016; Frankham et al., 2017; but see Zimmermann et al., 2018). As a matter of fact, the impact of the loss of genetic variation on increasing risk of extinction for wild populations has been extensively debated in the past (e.g., Lande, 1988; Caro and Laurenson, 1994; Frankham, 1995; Hedrick et al., 1995; Caughley and Gunn, 1996; Frankham and Ralls, 1998; Dobson, 1999), suggesting that demographic parameters (e.g., population size, density, sex ratio, age structure, fecundity; see Tarsi and Tuff, 2012) associated with stochastic and deterministic events should affect species on the verge of extinction before microevolutionary forces take effect (e.g., Frankham et al., 2010). Nonetheless, Spielman et al. (2004) reported that most threatened taxa, including plant species, present lower levels of genetic diversity than closely related non-threatened taxa, implying a higher risk of (local) extinction due to small population sizes, and as a consequence, a reduction in reproductive fitness.
Although the amount of genetic diversity in a population is commonly linked to its size and range (e.g., Frankham, 1996; Hamrick and Godt, 1996; Elegren and Galtier, 2016), a better understanding is needed of the susceptibility of species that are rare, endemic, and with small population sizes to extinction due to reductions in genetic diversity. Species that present small population sizes and a restricted geographic range (i.e., narrow endemic) tend to present lower levels of genetic diversity than those with larger population sizes and wide distribution (Kimura and Crow, 1964; Hamrick and Godt, 1989; Honnay and Jacquemyn, 2007; but see Ellis et al., 2006; Turchetto et al., 2016; Forrest et al., 2017; Martel et al., 2021). For instance, Honnay and Jacquemyn (2007) highlight significantly lower levels of genetic variation in small plant populations compared to large populations. The same pattern (i.e., low levels of genetic diversity) has been reported for threatened plant species with a history of fragmentation and/or population decline (e.g., Jiménez et al., 2014; Bupp et al., 2017; Simmons et al., 2018; Downey and Baskauf, 2020). In addition, high levels of inbreeding are expected for plant species with small and isolated populations (e.g., Falconer, 1989; Barret and Kohn, 1991; Allendorf and Luikart, 2007; Frankham et al., 2014; Oleas et al., 2014; Perrier et al., 2017; Rhoads et al., 2017; Toczydlowski and Waller, 2019; but see Nazareno and Carvalho, 2009; Guidugli et al., 2016), making the effects of genetic drift more pronounced. As a result, such populations are particularly prone to (local) extinction, as they lose variability more readily when compared to populations in which drift is an unexpressive microevolutionary force (e.g., Barret and Kohn, 1991; Bani et al., 2018; Toczydlowski and Waller, 2019). In addition to genetic factors, small populations are subject to rapid decline and extinction due to demographic fluctuations and environmental changes (e.g., Primack and Rodrigues, 2001; Frankham et al., 2010).
In this study, we aimed to assess the levels of genetic diversity and demographic history of a unique population of Mimosa catharinensis Burkart (Fabaceae), a rare, critically endangered (CONSEMA, 2014), and narrow endemic species that occurs in a small area (~700m2) in the Brazilian Atlantic Forest (Burkart, 1979). Considering the vulnerability of M. catharinensis to extinction and its unique reproductive biology (i.e., a plant species that is ecologically sterile), and based on the assumptions of population theory and the findings of empirical studies about genetic diversity in rare and endemic plant species occurring in small populations, we expected to find low levels of genetic diversity for M. catharinensis. Further, we expected to find indications of population retraction given the historic threats that have occurred in its biome (presented in the below section). To this end, and to contribute to in situ and ex situ conservation strategies, we used a high-throughput sequencing approach (i.e., double digest restriction site associated DNA sequencing, ddRADseq; Peterson et al., 2012) to identify unlinked and neutral SNP markers. This reduced representation sequencing method had been used extensively for species conservation studies (e.g., Nazareno et al., 2017; Chattopadhyay et al., 2019; Amor et al., 2020; Wright et al., 2020; Bard et al., 2021; Nazareno and Knowles, 2021) mainly due to its robustness to generate thousands of neutral and non-neutral molecular markers at a relative low cost. Our population genomic study tackles a central issue in evolution and conservation biology and we expect that it will be useful to safeguard the genetic diversity reported for the only remaining population of M. catharinensis. The approach applied in this study can also be used to guide conservation efforts for other plant species on the brink of extinction.
Materials and Methods
Species Description
Mimosa catharinensis is a plant species endemic to the Brazilian Atlantic Rainforest with an extremely restricted distribution, limited to one population located in the Rio Vermelho State Park (Parque Estadual do Rio Vermelho – PAERVE; Figure 1), Santa Catarina State, Southern Brazil (Burkart, 1979; Ferreira, 2010). The only documented population of M. catharinensis (~700m2) occurs in one of the most heavily impacted areas of the PAERVE (Figure 1) due to deforestation and environmental degradation for over 200years (Berenhauser, n.d.). This narrow endemic and critically endangered plant species (CONSEMA, 2014) is a lianescent shrub that produces masculine and hermaphrodite white, glabrous, and tetramerous flowers (Burkart, 1979). Pollen dispersal occurs via zoophily (e.g., Apis mellifera; Silva et al., 2005), although nectar-dependent visitors are infrequent due to a lack of floral nectar (Silva et al., 2005). The pods are of the indehiscent craspedium type and are linear-oblong with curved prickles on the margins (Medeiros and Stefani, 2018). Although the reproductive structures of M. catharinensis have been characterized morphologically, pods without seeds were observed during field collection in 2019. Based on herbarium records, the lack of seed production in M. catharinensis was first recorded in 1994 (Voucher 30,482; FLOR herbarium, UFSC). As no seedlings were found in its area of occurrence, M. catharinensis may be considered an ecologically sterile plant species (i.e., a plant species in which the recruitment rate over time is nil as a consequence of lack of sexual reproduction). As successful in vitro pollen germination had been reported for M. catharinensis (Silva et al., 2005), further pollination studies are needed to improve our understanding of its reproductive biology.
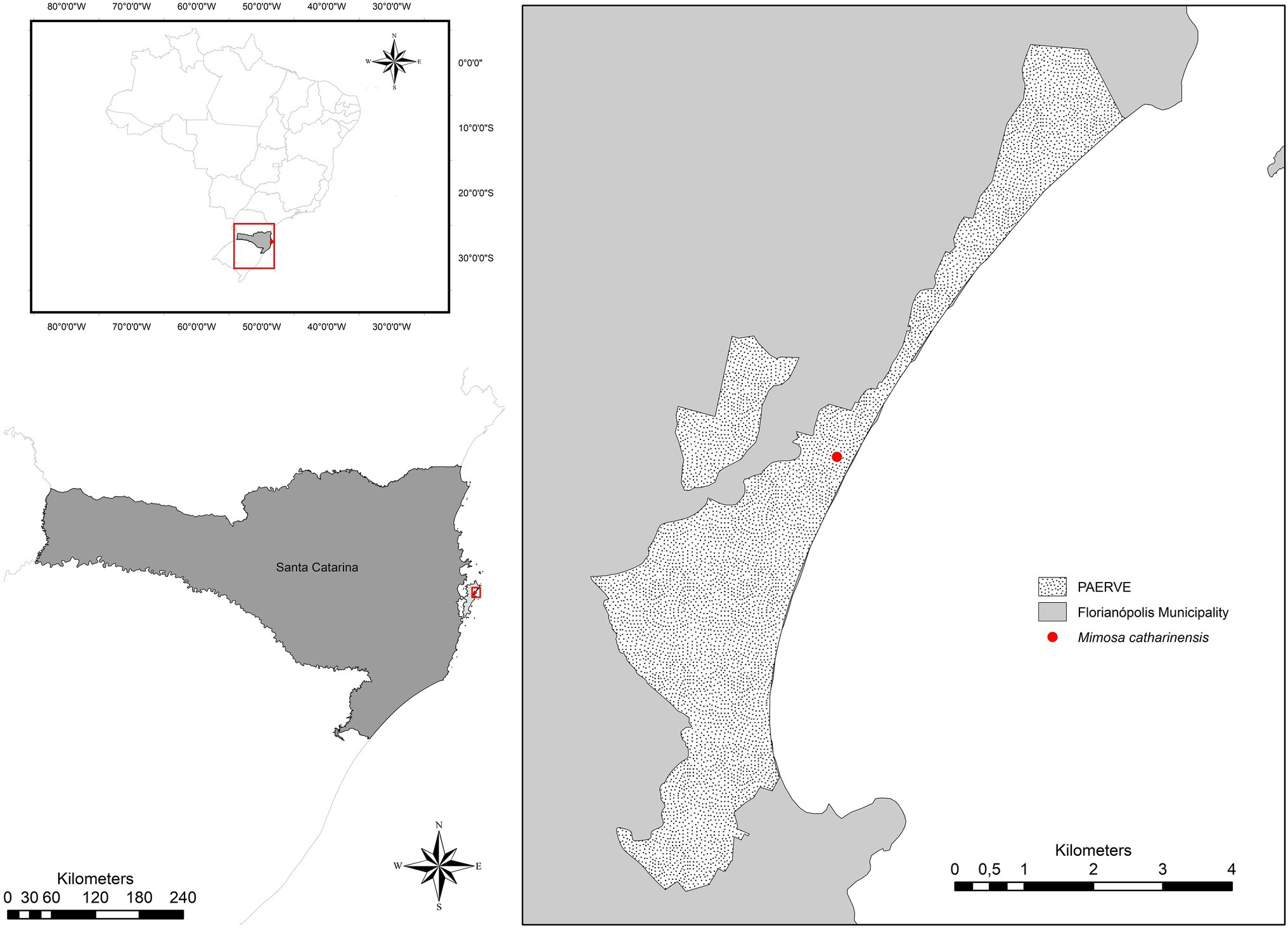
Figure 1. Location of the extremely small and unique population of Mimosa catharinensis in Rio Vermelho State Park (Parque Estadual do Rio Vermelho; PAERVE), Santa Catarina State, Southern Brazil.
Although there are efforts by the Santa Catarina State government (Gasper et al., 2012) to generate genetic and ecological data to inform conservation efforts and to safeguard plant species threatened with extinction, no previous evolutionary studies – including phylogenetic analysis and genomic characterization – have been conducted on this critically endangered species.
History of the Research Area
Since 2002, the PAERVE has been recognized by UNESCO as one of the core areas of the Atlantic Forest Biosphere Reserve and consists of 1,530 hectares of Atlantic Rainforest biome (Ferreira, 2010; Bechara et al., 2013). The introduction of pine and eucalyptus into the park has brought about significant changes to its restinga (sandy plains) vegetation and is the main cause of degradation of the coastal ecosystem of Santa Catarina Island (Ferreira, 2010). Currently, it is estimated that 750ha of the park are covered by Pinus spp., of which about 33% are located in dunes and sandbanks that have been progressively invaded since pine was first introduced (Bechara et al., 2013). Nevertheless, approximately 400ha of dense rainforest and 250ha of restinga vegetation fragments remain protected in the park (Bechara et al., 2013), the latter located mainly at the southern and northern tips of the conservation area (Ferreira, 2010).
Population Sampling
We collected leaf samples from all 33 identified adult individuals of unknown age that constitute the only remaining M. catharinensis population. Leaves were stored in silica gel for later extraction. One voucher (FLOR30482) is deposited at the FLOR herbarium at the Federal University of Santa Catarina – UFSC.
Genomic Library Preparation and Sequencing
DNA was extracted from leaf samples of all collected individuals employing the NucleoSpin® kit (Machereney-Nagel GmbH & Co. KG) following manufacture’s guidelines. After extraction, the quality of each sample was verified using Thermo Scientific NanoDrop 2000 Spectrophotometer (Thermo Fisher Scientific Inc.) and the concentration of double-strand DNA (dsDNA) was obtained by using Qubit dsDNA Assay Kit (Invitrogen). The genomic library was prepared using a double-digest restriction site-associated DNA sequencing (i.e., ddRADseq) protocol (Peterson et al., 2012) with modifications proposed by Nazareno et al. (2017). Digestions reactions were performed using 0.5μg of genomic DNA, 5,000 units of MseI, 5,000 units of HF-EcoRI, and 1x CutSmart buffer (New England Biolabs) in a total 20μl reaction volume for 3h at 37°C, ending with a 20min deactivation step at 65°C (Nazareno et al., 2017). Reactions products were cleaned using Agencourt AMPure XP system (Beckman Coulter) according to manufacturer’s recommendations. The dsDNA was quantified using Qubit and the amount of DNA was standardized before to procedure with the ligation. We used 80ng DNA, 1M of MseI adaptor, 0.33M of EcoRI double-strand adaptor unique for each sample, 1U of T4 DNA ligase (New England Biolabs) and 1.40μl of T4 ligase buffer in 30μl ligation reaction that was stored at a room temperature (23°C) for 30min After, heat-killed the reaction at 65°C for 10min and then, slowly cooled until reach room temperature. Details of barcode sequences can be found in Nazareno et al. (2017). Products of the ligation reaction were purified using the Agencourt AMPure XP system and used in PCR reactions. PCR reactions were carried out in a total volume of 20μl, containing 13.5μl of ligation product, 0.2M dNTPs, 1.0μM MgCl2 0.5U iProof™ High-Fidelity DNA Polymerase (Bio-Rad) and 2X of iProof buffer. The PCR protocol (98°C for 30s, 20cycles of 98°C for 20s, 60°C for 30s and 72°C for 40s, followed by a final extension at 72°C for 10min) was performed in an Eppendorf System. The amplicons were cleaned with the Agencourt AMPure XP system and quantified using Qubit dsDNA assay Kit. We used an automated size-selection technology (i.e., Pippin Prep; Sage Science, Beverly, MA, United States) at 2% agarose cartridge to select DNA fragments at a target range size of 375–475bp. The library was sequenced (100-bp single-end) on a lane of Illumina HiSeq 2,500 flow cell (Illumina Inc., San Diego, CA, United States) at The Centre for Applied Genomics in Toronto, Canada.
Raw Data Processing and SNPs Identification
Data quality was checked using the program FastQC version: 0.11.8.1 The file containing raw sequence reads was analyzed in Stacks 2.41 (Catchen et al., 2011, 2013; Rochette et al., 2019) using de novo assembly. Initially, we used the process_radtags program in Stacks to examine individual barcodes, enzyme cutsite integrity and to demultiplex the data. For barcodes rescue we admitted at most two mismatches (−barcode_dist 1 2). We filtered raw reads using the phred score 33 and used the option −t to trim all of it at 85 base pairs (−phred 33, −t85). With the process_radtags output, we run the Ustacks program, that uses a maximum likelihood framework to aligns short-read sequences and create putative alleles (stacks). The maximum distance allowed between “stacks” was two nucleotides. The minimum depth of coverage required to create a “stack” and the maximum number of stacks at a single de novo locus was set as three. We enabled the deleveraging algorithm (−d) to solve merged tags, and a bounded-error model to identify SNPs. An alpha value of 0.05 and an upper bound of 0.1 were used. In the next step, we performed Cstacks to merge alleles of all samples and create a catalog of consensus loci. Three mismatches were allowed between loci in catalog build. Each individual sample loci were then compared against the catalog through the Sstacks program. We ran tsv2bam program to organize the single-end reads by locus, instead by sample, creating a BAM file that was used as input to the Gstacks program. Gstacks uses the single-reads to build contigs and merges them into loci. It also aligns reads to the loci using Ukkonen’s algorithm, identifies SNPs for each locus and each individual genotype and converts SNPs into phased haplotypes. Finally, we used POPULATIONS in Stacks (Catchen et al., 2011, 2013; Rochette et al., 2019) to generate and export the SNP data set, as well as FASTA files containing the per-locus consensus sequences, and individual loci sequences, that were applied in the further analyses. To assess the effects of missing data on genetic estimates, we run POPULATIONS several times with a Minor Allele Frequency (MAF) of 5% (−min_maf 0.05), a maximum heterozygosity (−max_obs_het) of 0.65, and the percentage of missing data varying from 0 to 30%. All data sets include one random SNP per locus. To avoid potential bias sources in the forward genetic analyses, Hardy–Weinberg (H–W) equilibrium tests was done using the adegenet and pegas packages2 (Jombart, 2008; Paradis, 2010; Jombart and Ahmed, 2011) implemented in R. In addition, linkage disequilibrium (LD) between loci was tested using Arlequin 3.5.2 (Excoffier and Lischer, 2010). Type I error rates for these tests were corrected for multiple comparisons using the sequential Bonferroni procedure (Rice, 1989), and SNPs that failed the H–W equilibrium test and/or SNP pairs in LD were excluded from further analyses. To exclude non-nuclear loci, the per-locus consensus sequences were aligned against reference chloroplast and mitochondrial genomes using Acacia dealbata (NCBI accession number KX852435) and Acacia ligulata (NCBI accession number MH933866), respectively. We used the BLASTn program3 to identify loci that presented identity greater than or equal to 80%.
Genetic Diversity
To assess the influence of missing data on genetic diversity estimates, we used the “BasicStats” function in the DiveRsity package in the R software environment4 (Keenan et al., 2013). We estimated unbiased expected genetic diversity (uH E; Nei and Roychoudhury, 1974), observed heterozygosity (H O), and the inbreeding coefficient (Wright’s Fixation Index F IS; Wright, 1943). Population genetics statistics were averaged across loci using the DiveRsity package in R (Keenan et al., 2013). Confidence intervals at 95% were obtained to evaluate differences among means of genetic parameters estimated for all data sets. We used the final data set to calculate minor allele frequencies using the adegenet package in R (Jombart, 2008; Jombart and Ahmed, 2011).
Mating System of Mimosa catharinensis
We used the kinship coefficient (Loiselle et al., 1995) implemented in the SPAGeDi program (version 1.5; Hardy and Vekemans, 2002) to estimate random outcrossing rates (1-s, where s indicates the estimated selfing rate) based on standardized identity disequilibrium for all data sets. Significance for the identity disequilibrium coefficient was obtained with 1,000 permutations, and a jackknife over loci approach was applied to calculate the standard error of outcrossing estimates.
Clonality Assessment
We evaluated the power of discrimination (PD) for the complete single nucleotide polymorphism (SNP) data set (i.e., the data set without missing data; MD) using the equation 1-PI, where PI represents the probability that two individuals drawn at random from a population will have the same genotype at multiple loci (Waits et al., 2001). This analysis was performed in GeneAlEx 6.5 program (Peakall and Smouse, 2012). Taking into account that clonal reproduction has been reported for plant species of the Fabaceae family (e.g., Dev et al., 2010; Gui et al., 2013; Gaddis et al., 2014; Mori et al., 2018; Amor et al., 2020), including M. catharinensis (Rogalski et al., 2005), a clonality test was applied for M. catharinensis using the “poppr” package (Kamvar et al., 2014, 2015) in R version 3.5.3 (R Core Team, 2019). The “bitwise.dist” function was used to calculate a pairwise genetic distance matrix between individuals. Then, we ran the “mlg.filter” function to apply a threshold that defines the minimum distance to determine distinct multi-locus genotypes (MLGs; see Kamvar et al., 2015). Applying a threshold when using SNP markers is important in order to deal with the limited observable genetic differences between genets due to sporadic somatic mutations or issues associated with high-throughput sequencing technologies (e.g., genotyping errors and missing data; Kamvar et al., 2015; Mastretta-Yanes et al., 2015). We chose the average neighbor as the clustering algorithm with a threshold of 0.04, which has been shown to be sufficient to explain intra-clonal variation when SNPs derived from ddRADseq are used (Amor et al., 2020). In addition, a less conservative threshold of 0.06 was used to infer the numbers of MLGs in M. catharinensis. The threshold of 0.06 was chosen in order to minimize the limitation of our experimental design, which did not include replicates to estimate genotyping errors. To visualize the number of putative lineages, we ran the “upgma” function (Phangorn package; Schliep, 2011) to construct ultrametric trees.
Demographic History
The demographic history of M. catharinensis was inferred using a composite likelihood-based approach implemented in FastSimCoal v. 2.6 (Excoffier et al., 2013). VCF files were converted into the *.arp format using the program PGDSpider 2.1.1.5 (Lischer and Excoffier, 2012). Assuming genetic distances of 0.04 and 0.06, we obtained two observed folded Site Frequency Spectrums (SFS based on minor allele frequency) for our filtered SNP data set using the Arlequin 3.5 program (Excoffier and Lischer, 2010). We tested four evolutionary scenarios: constant population size, bottleneck, population decline, and population expansion (Figure 2). We performed 100 replicates for each tested model and folded SFS. The parameters for each run were estimated based on 100,000 simulations and 40 ECM optimization cycles. We used an overall substitution rate of 7×10−9 per site/generation as reported for Arabdopsis thaliana (Ossowski et al., 2010). The maximum estimated likelihood for each demographic scenario and SFS were used to identify the model with the best fit, which was chosen based on Akaike’s Information Criterion (AIC; Akaike, 1973, 1974) estimated as ΔAIC. All models were ranked, with the model with the lowest ΔAIC being considered the most plausible. It is important to note that models with ΔAIC ≤2 have substantial support (Burnham and Anderson, 2001). We also assessed the probability that each model is the best fit by estimating Akaike weights (w i). Once the best model was selected, its estimated parameters were used to simulate 100 SFS data sets to built confidence intervals based on bootstrap distribution.
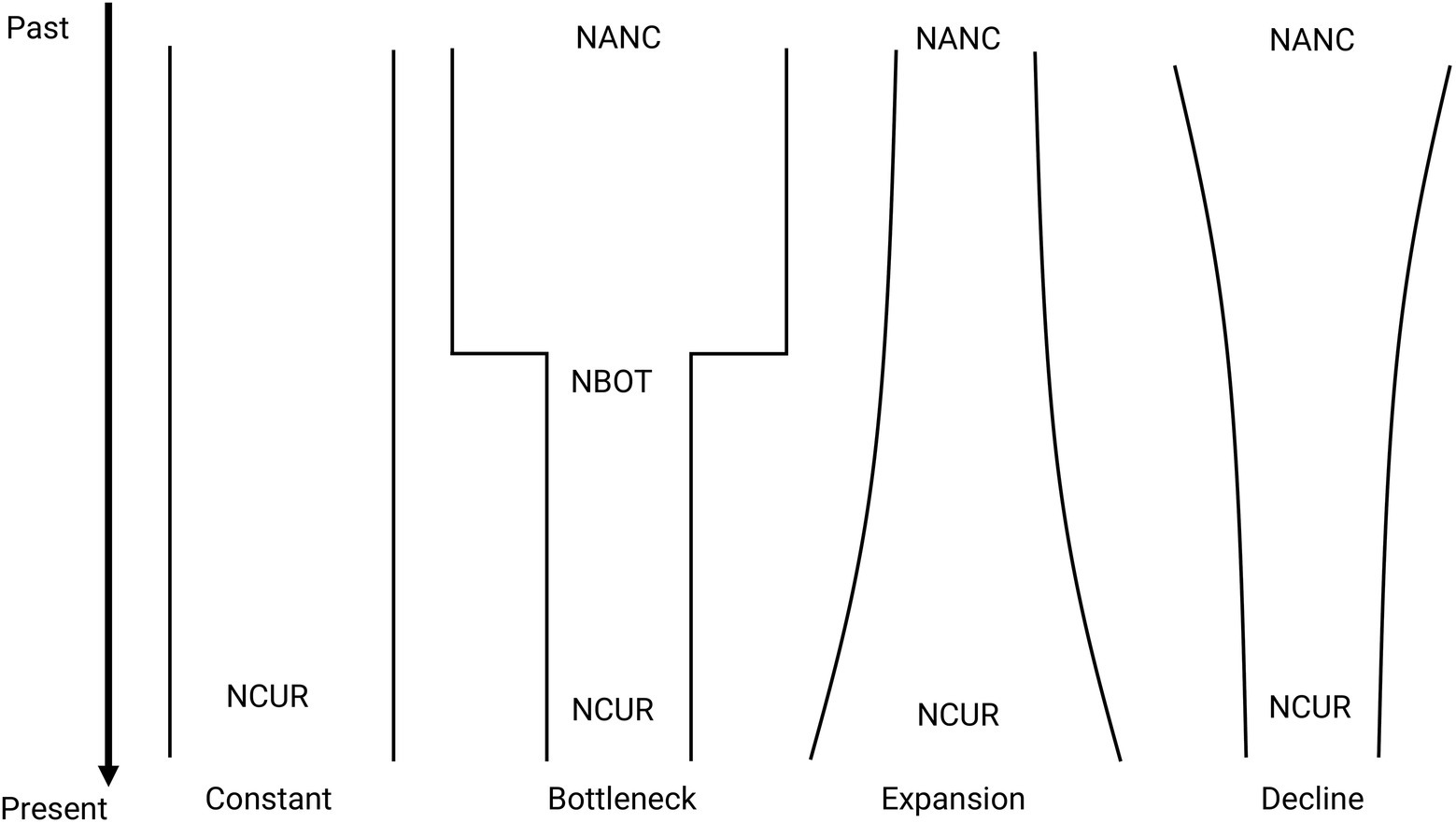
Figure 2. Representation of the demographic scenarios tested for M. catharinensis in FastSimCoal 2.6. Estimates include coalescent-based current (NCUR) and ancestral (NANC) population size, number of generations since the bottleneck occurred (TBOT), and population size at the end of the bottleneck (NBOT).
Results
Raw Data Processing and SNP Calling
The Illumina sequencing generated a total of 79,759,227 raw reads, of which less than 1% was discarded due to low quality. The average (±SE), minimum, and maximum retained reads were 2,384,161 (±115,118 SE), 1,470,849, and 4,011,918. The total number of genotyped RADtag loci was 274,113 with a mean coverage depth of 12.7 (±2.7 SD). After Bonferroni adjustment, significant deviations from Hardy–Weinberg equilibrium were observed for all data sets (Table 1). No linkage disequilibrium was observed between loci after Bonferroni correction for k tests (Table 1). After alignment, one locus matched the plastome of A. dealbata (96.47% identity) and it was excluded from the data set. The final numbers of unlinked SNPs used in each data set are shown in Table 1.
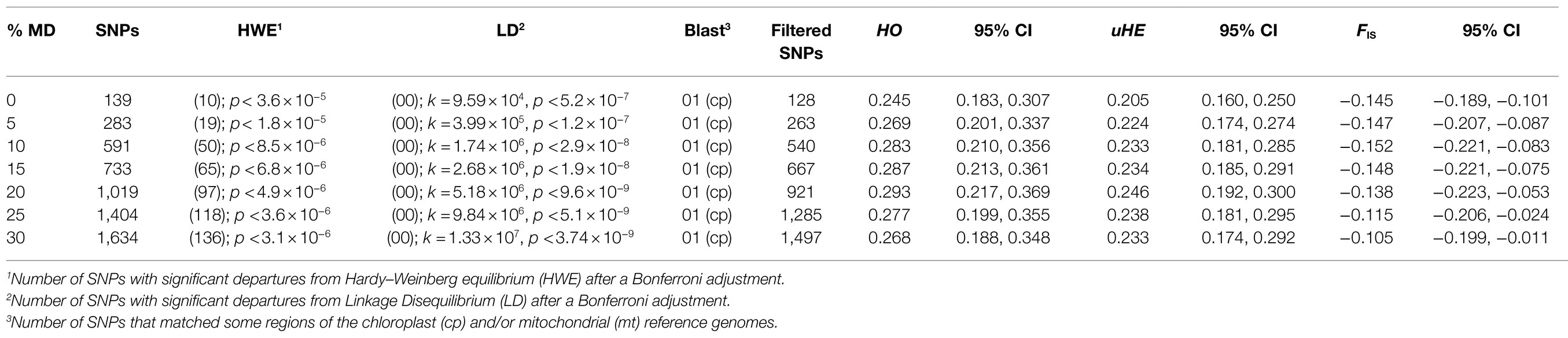
Table 1. Variation of genetic parameters (HO, observed heterozygosity; uHE, unbiased expected heterozygosity; FIS, Wright’s Fixation Index) according to changes in percent of missing data for the only known population of M. catharinensis.
Genetic Diversity Parameters, Inbreeding Coefficient, and Outcrossing Rate
Genetic diversity parameters (uHE and HO ) and Wright’s Fixation Index (F IS) for data sets with different percentages of missing data are shown in Table 1. For all genetic indices (uHE , HO , and F IS) obtained for the M. catharinensis population, no statistical differences were observed among the data sets (Table 1). Further analyses were performed using the data set without missing data, which presented a minor allele frequency (MAF) averaged at 0.125 (±0.093 SD).
Based on mating system analysis for the data sets with different percentages of missing data (Table 1), the outcrossing rates varied from 0.883 (SE±0.0483) to 0.909 (SE±0.011). Although the outcrossing rate was inflated with an increase in MD, no statistical differences were observed among the data sets (Supplementary Table S1).
Clonality Assessment
The results of the PD analysis showed that at least 13 loci are enough to discriminate closely related individuals with high accuracy, indicating that the data set with 0% missing data (i.e., 128 SNPs) has high discriminatory power to identify M. catharinensis individuals. The defined threshold of 0.04 did not cluster MLGs into multilocus lineages (MLLs; Figure 3A). While the genetic distance of 0.04 did not indicate the presence of clonal genotypes for M. catharinensis, the threshold of 0.06 indicates 23 putative MLLs (Figure 3B).
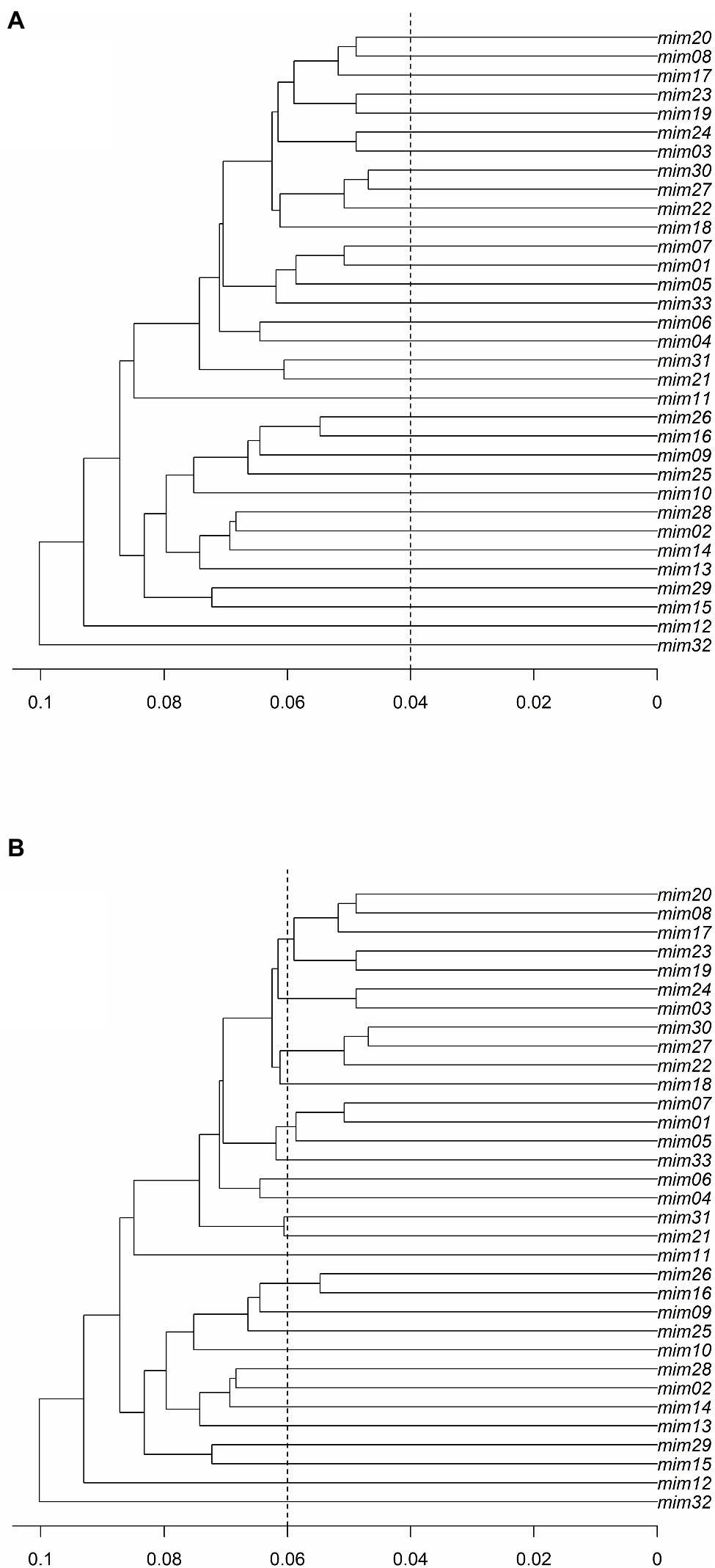
Figure 3. The ultrametric topology trees for the M. catharinensis population based on genetic distances (horizontal axis) and using a threshold of (A) 0.04 and (B) 0.06.
Demographic History
The highest likelihoods are summarized in Table 2, as well as the results for the Akaike estimates (ΔAIC and w i). The model with the best fit for the complete (N=33) and the reduced (n=23) data sets was the bottleneck (Table 2). The inferred parameters for the bottleneck model and the 95% CIs obtained for the data sets with different numbers of individuals are shown in Supplementary Table S2. The parameter estimates under the empirical SFS suggests that population contraction occurred only a few generations ago (TBOTcompl=82; TBOT33=10), with a continuous decline in population size after the bottleneck. However, the 95% CIs were wide and overlapping.
Discussion
Genomic approaches are valuable as they can provide new insights and a better understanding of the amount, distribution, and functional significance of genetic variation in natural populations (Allendorf et al., 2010). As predicted by Funk et al. (2012), the use of genomic data is on the rise for species in relation to discussions around conservation (Hawkins et al., 2018; Huy et al., 2018; Lanes et al., 2018; Ball et al., 2020; Bao et al., 2020; Chen et al., 2020; Liu et al., 2020; Zang et al., 2020; Zimmerman et al., 2020; Bradbury et al., 2021). Thus, such data can play a critical role in informing management strategies and policy for species on the verge of extinction, many of which are narrow endemics and prime targets for conservation genomic assessments (Silva et al., 2020). Considering that estimates of genetic parameters have a direct influence on conservation decision-making, we applied a reduced representation library approach (i.e., ddRADseq) to generate genomic data for M. catharinensis. This data was used to assess intrapopulation genetic variation, as well as the demographic history for this critically endangered, narrow endemic species of the Atlantic Rainforest.
Our findings show that, contrary to what is expected for narrow endemics (Kimura and Crow, 1964; Hamrick and Godt, 1989; Spielman et al., 2004; Honnay and Jacquemyn, 2007), and despite its extremely small population size, M. catharinensis has moderate intrapopulation genetic diversity, expressed here by H E, with an apparent absence of inbreeding. Although the use of only one metric to assess genetic diversity is not an optimal condition, our results bring to light several factors that can influence the observed patterns of genetic diversity and inbreeding in relictual populations of threatened species. Our results underscore the importance of assessing the genetic patterns of each species in order to critically evaluate their genetic variability and the microevolutionary forces by which they are shaped (Amos and Harwood, 1998; Turchetto et al., 2016). Below, we discuss how our findings can inform conservation and management strategies for M. catharinensis.
Contradicting Patterns of Genetic Diversity in Small Populations
Despite the general expectation of low intrapopulation genetic variability and high susceptibility to inbreeding depression associated with pronounced effects of genetic drift in small populations (e.g., Kimura and Crow, 1964; Hamrick and Godt, 1989; Barret and Kohn, 1991; Ellstrand and Elam, 1993; Honnay and Jacquemyn, 2007), the results for M. catharinensis do not support the hypothesized loss of genetic diversity, which should reflect high levels of inbreeding, as a consequence of reduced population size and narrow endemism. Although contradictory to theoretical predictions, unexpectedly high levels of genetic diversity are not unusual for narrow endemics (e.g., Fernández-Mazuecos et al., 2014; Jiménez-Mejías et al., 2015; Turchetto et al., 2016; Forrest et al., 2017; Goetze et al., 2018; Sękiewicz et al., 2020; Bard et al., 2021; Garcia-Jacas et al., 2021), creating an indistinct pattern for this kind of plant species. For instance, in the studies by Fernández-Mazuecos et al. (2014) and Jiménez-Mejías et al. (2015), the authors highlight the paradox of genetic diversity levels in narrow and extremely narrow endemic plant species from the Mediterranean. While Fernández-Mazuecos et al. (2014) found moderate levels of genetic diversity for Naufraga balearica, Jiménez-Mejías et al. (2015) reported high levels of genetic diversity for Pseudomisopates rivas-martinezii. In comparison to previous genetic assessments for narrow endemics in the same regions, the authors (Fernández-Mazuecos et al., 2014; Jiménez-Mejías et al., 2015) found that, although some species have low genetic variability, most species showed moderate to high levels of genetic diversity (e.g., Sales et al., 2001; Coppi et al., 2008; Mameli et al., 2008; Mayol et al., 2012; De Castro et al., 2013; Forrest et al., 2017; Sękiewicz et al., 2020; Garcia-Jacas et al., 2021). Thus narrow endemism does not always imply limited genetic diversity. However, such contrasting results may be due to differences in population size, distribution range, ecological traits, and evolutionary history (Fernández-Mazuecos et al., 2014).
In addition, several studies have indicated that life-history traits (e.g., lifecycle, growth form, mating and breeding systems) strongly influence the amount and distribution of genetic variation in natural populations (e.g., Hamrick and Godt, 1996; Ellis et al., 2006; Elegren and Galtier, 2016; Goetze et al., 2018; Bard et al., 2021; De Kort et al., 2021). For Aechmea kertesziae, a narrow endemic species of Brazilian Coastal restingas (sandy vegetation), traits such as self-incompatibility, long-term persistence, clonal reproduction, and consistent population size may explain the high levels of genetic diversity observed for the species (Goetze et al., 2018). Specifically for M. catharinensis, the predominance of historical outcrossing, as shown in our analysis, seems to be an important factor that contributes to the moderate levels of intrapopulation genetic diversity. It is important to note that outcrossing plants are likely to present higher levels of intrapopulation genetic diversity than self-compatible species (Hamrick and Godt, 1996; Glémin et al., 2006; Ball et al., 2020). Moreover, a lack of successful sexual reproduction in M. catharinensis (i.e., production of pods without seeds) suggests that the level of intrapopulation genetic diversity has remained static for at least two decades, even though our demographic inferences indicated a recent decline in population size.
As life-history traits play a significant role in shaping the patterns of genetic diversity, it is important to compare the levels of genetic diversity among congeneric species in order to mitigate phylogenetic effects (Bevill and Louda, 1999; Simon and Hay, 2003). This is essential to better understand how features such as population sizes and geographic distribution ranges affect reproductive biology and, as a consequence, the genetic variability of closely related plant species. For example, population genetics studies indicate that Petunia secreta and Petunia exserta, both of which are narrow endemic species, have high genetic diversity indices; however, these indices are lower than those reported for its congener Petunia axillaris, which has a wider geographic distribution range (Turchetto et al., 2016). Beyond dissimilarities in the area of occurrence of these Petunia species, different floral syndromes and reproductive systems have been described as the probable causes of the observed genetic variation (Kokubun et al., 2006; Turchetto et al., 2016).
Although Mimosa is one of the largest genera from Mimosoideae (Barneby, 1991), there are few population genetics studies for species of this genus (Moreira et al., 2011; Pramual et al., 2011; Arruda et al., 2019; Araújo et al., 2020; Morales et al., 2020), and none of these previous studies used SNP markers. Nevertheless, genetic diversity has been estimated using markers such as RAPD (Mimosa pigra; Pramual et al., 2011), AFLP (Mimosa subser. Dolentes–Brevipedes; Morales et al., 2020), ISSR (M. caesalpiniifolia Benth.; Araújo et al., 2020), and allozyme (Mimosa scabrella; Moreira et al., 2011; Arruda et al., 2019). In this context, M. catharinensis presented lower levels of intrapopulation genetic diversity than that reported for its widespread congener M. scabrella (HE =0.362 to 0.469; Moreira et al., 2011; Arruda et al., 2019).
When comparing studies on narrow endemics assessed using SNPs, and considering the theoretical maximum heterozygosity for biallelic markers (HE =0.5), we are unable to make generalizations about the genetic diversity of narrow endemics. Indeed, M. catharinensis displays higher levels of genetic diversity than self-compatible (e.g., Ball et al., 2020) and clonal and functional sterile species (e.g., Amor et al., 2020). However, some tropical plant species (e.g., Lanes et al., 2018; Ball et al., 2020) showed the most similar genetic diversity to M. catharinensis.
Inbreeding and Mating System of Mimosa catharinensis
Our findings indicate an excess of heterozygosity for this plant species, counteracting the theoretical expectation that reductions in population size will result in high levels of inbreeding. In terms of genetic variability, the levels of inbreeding in narrow endemic plant species with small population sizes vary according to individual features of each species/population (Angeloni et al., 2011). Reports for plant species with very small populations vary from high levels of inbreeding (Jiménez et al., 2014; Finlay et al., 2017; Rodrigues et al., 2019; Wang, 2020), to no evidence of inbreeding (Edwards et al., 2014, 2021; Spoladore et al., 2017), to an excess of heterozygotes (Cabrera-Toledo et al., 2008; Radosavljević et al., 2015; Amor et al., 2020) as found herein. Barret and Kohn (1991) emphasize that the mating pattern is a prime determinant of inbreeding levels in natural populations regardless of their size. In fact, self-compatible species can be more susceptible to inbreeding considering that self-incompatibility is likely to have evolved to prevent inbreeding depression (Charlesworth and Charlesworth, 1987). On the other hand, predominantly outcrossing species can suffer from inbreeding depression due to mating between relatives (Husband and Schemske, 1996).
The (historical) outcrossing rate observed in M. catharinensis suggests that it has a mixed mating system, which is characterized as ranging from 5 to 95% depending on environmental conditions and pollination frequency (Karasawa, 2015). Such a wide range in outcrossing rates for mixed-mating species are common and can change over time and space due to environmental conditions and intrinsic population features, such density and demographic structure (e.g., Wright et al., 2013). As with M. catharinensis, high outcrossing rates have been reported for many Neotropical plant species (e.g., Ward et al., 2005; Feres et al., 2012, 2021; Nazareno and Reis, 2012; Spoladore et al., 2017; Godoy et al., 2018; Montagna et al., 2018; Sujii et al., 2021), including two congener Mimosa species (Moreira et al., 2011; Arruda et al., 2020). Studies on populations of M. scabrella (Arruda et al., 2020), for instance, showed a similar reproductive pattern with high outcrossing rates (t=0.925/0.845) and low estimates of selfing (s=0.075/0.155). For M. catharinensis, the interpretation of negative F IS values coupled with high t values should be viewed with caution due to the distinct biology of the species (i.e., production of seedless pods and absence of seedlings in the study area). Such biological characteristics observed for M. catharinensis suggest that a significant selective pressure against homozygotes must have occurred in previous generations, especially when taking into account the excesses of heterozygosity observed in its small population. As M. catharinensis population is constituted only by adult trees, a further study investigating inbreeding depression seems not to be a feasible task as a consequence of the lack of different ontogenic stages in its population.
Although pollen grains have been reported as viable for M. catharinensis (Silva et al., 2005), the apparent reproductive failure seems to be due to an extremely low frequency of floral visitors (Silva et al., 2005), implying low pollination efficiency. This phenomenon has been also described for other Mimosa species, such as Mimosa bimucronata (Seijo and Neffa, 2004). As with M. catharinensis, M. bimucronata does not produce nectar, however, its newly opened flowers exude a slight fruit odor to attract pollinators (Silva et al., 2011). Despite poor fruit set due to inefficient pollination, M. bimucronata produces viable fruits and seeds (Seijo and Neffa, 2004). In contrast, Mimosa pudica showed high pollination efficiency, mediated by A. mellifera, resulting in high fruit and seed yields and quality (Taimanga and Fohouo, 2018). Further pollination biology studies are needed to clarify the causes of reproductive failure in M. catharinensis.
Reconstruction of the Demographic History of Mimosa catharinensis
The selection of the best demographic model was the main focus of the coalescent-based inference for M. catharinensis, considering a comparison of two data sets with different numbers of individuals and excluding putative clonal individuals based on their genetic distance. The fact that confidence intervals were wide and overlapping did not affect our results and can be related to the uncertainty of the estimated parameters and/or the small SFS (Rödin-Mörch et al., 2019). Nonetheless, the values presented in Supplementary Table S2 should be interpreted with caution. The generated SFS for both data sets suggest that a recent bottleneck is the best explanation for the current levels of genetic diversity in M. catharinensis. This corroborates our findings of excess heterozygosity for the species, which is a characteristic of populations that have experienced a recent bottleneck (Luikart et al., 1998). Of the factors that may have resulted in a drastic decrease in species population sizes in the restinga, the fragmentation of Santa Catarina Island’s native vegetation is particularly relevant, especially the degradation that occurred within the PAERVE before it was deemed a protected area. The implementation of reforestation projects in the park using exotic, invasive species also resulted in fires, changes to the soil, and deforestation of native vegetation, all of which accentuated the damage occurring to such a heterogeneous vegetal formation (Heberle, 2011).
In contrast to the evidence of population contraction for M. catharinensis, the majority of studies on past demographic changes in tropical plant species from South America have reported indications of expansion during the Quaternary period (e.g., Turchetto-Zolet et al., 2013). On the other hand, demographic stability has been suggested for the period for species from the Southern Brazil coastal restinga (Goetze et al., 2018). Therefore, further studies are needed to infer historical changes in population sizes of plant species exclusive to Santa Catarina Island (Hassemer et al., 2015), whose recent demographic changes may be similar to that reported herein for M. catharinensis.
Implications for Conservation
The extremely small number of individuals (N=33) in the only known M. catharinensis population, coupled with strong evidence for a lack of sexual reproduction, suggests that the conservation perspectives for this critically endangered species are concerning. Although no inbreeding was detected, the species is facing imminent risk of extinction because of its reduced population size, making it more susceptible to stochastic events. As a matter of fact, ex situ conservation activities must be implemented in order to safeguard the remaining genetic diversity of M. catharinensis. One potential strategy suggested in studies on biodiversity conservation of threatened species consists of the preservation of tissue culture, an in vitro cultivation of isolated live tissue, enabling the propagation of species facing difficulties with natural reproduction (Paiva and Paiva, 2001; Santos et al., 2019). Protocols to apply tissue culture techniques for conservation of endangered species have been successfully developed (e.g., Sherif et al., 2018; Choudary et al., 2020; Lerin et al., 2021; Mishra et al., 2020), and such an approach has been used to conserve the narrow endemic species Styphelia longissima (Thomas et al., 2021).
In addition, as in vitro germination tests show pollen grain viability (Silva et al., 2005), additional pollination biology studies (e.g., pollination treatments such as manual cross-pollination and self-pollination) should be performed to test for viable seed production. The information available through pollination biology studies can help us to solve the puzzle of M. catharinensis reproduction, allowing us to better plan effective conservation measures for this rare plant species. In terms of in situ conservation, the fact that the species is found in a protected area is a significant starting point, although to date this fact has been insufficient for its conservation. Today, the management of invasive, exotic species (e.g., Pinus spp. and Eucalyptus spp.), one of the main issues threatening the flora of PAERVE, should be prioritized. Furthermore, it is widely reported that adequate restoration of the contaminated areas of the park are urgently needed (Ferreira, 2010; Bechara et al., 2013), and efforts to achieve this goal have been implemented according to the park’s Management Plan (IMA, 2020). Moreover, although a robust floristic survey of Santa Catarina State has been conducted (Vibrans et al., 2012), not all the biodiversity present in the state was sampled. As such, biodiversity inventories within Brazil’s protected areas are crucial (Oliveira et al., 2017), and efforts on field surveys must be undertaken to verify the narrow occurrence of this critically endangered plant species. Likewise, we are committed to monitoring the M. catharinensis population size over time to avoid further losses in genetic diversity.
Data Availability Statement
SNP data sets for M. catharinensis are available for download from the Dryad Digital Repository (https://doi.org/10.5061/dryad.j9kd51ccz).
Author Contributions
AN designed the study, collected the samples, and conducted molecular work. TT performed analyses, and led the writing of the manuscript with input from AN, who also provided analytical support. All authors contributed to the article and approved the submitted version.
Funding
We thank the Conselho Nacional de Desenvolvimento Científico e Tecnológico (CNPq) to AN (429266/2018-9) for funding the field and molecular work associated with this project. Additional funds were provided by the Conselho Nacional de Desenvolvimento Científico e Tecnológico (CNPq) through a PQ-2 grant to AN (306182/2020-3) and the Fundação de Amparo à Pesquisa de Minas Gerais (FAPEMIG) for the scholarship to TT.
Conflict of Interest
The authors declare that the research was conducted in the absence of any commercial or financial relationships that could be construed as a potential conflict of interest.
Publisher’s Note
All claims expressed in this article are solely those of the authors and do not necessarily represent those of their affiliated organizations, or those of the publisher, the editors and the reviewers. Any product that may be evaluated in this article, or claim that may be made by its manufacturer, is not guaranteed or endorsed by the publisher.
Acknowledgments
We thank the Core Facility for Scientific Research (CEFAP) of the Universidade de São Paulo for computational support. We also thank Evelyn Nimmo for editing the English of the manuscript. We also thank the reviewers for helpful comments that improved the manuscript. For field assistance in the PAERVE, we also thank Elaine Zuchiwschi (Instituto do Meio Ambiente do Estado de Santa Catarina).
Supplementary Material
The Supplementary Material for this article can be found online at: https://www.frontiersin.org/articles/10.3389/fpls.2021.730258/full#supplementary-material
Footnotes
1. ^http://www.bioinformatics.babraham.ac.uk/projects/fastqc/
2. ^https://CRAN.R-project.org/package=adegenet
References
Akaike, H. (1973). “Information theory as an extension of the maximum likelihood principle.” in 2nd International Symposium on Information Theory. eds.
Akaike, H. (1974). A new look at the statistical model identification. IEEE Trans. Automat. Contr. 19, 716–723.
Allendorf, F. W., Hohenlohe, P. A., and Luikart, G. (2010). Genomics and the future of conservation genetics. Nat. Rev. Genet. 11, 697–709. doi: 10.1038/nrg2844
Allendorf, F. W., and Luikart, G. (2007). Conservation and the Genetics of Populations. Malden, Massachusetts, USA: Blackwell.
Amor, M. D., Johnson, J. C., and James, E. A. (2020). Identification of clonemates and genetic lineages using next-generation sequencing (ddRADseq) guides conservation of a rare species, Bossiaea vombata (Fabaceae). Perspect. Plant Ecol. Evol. Syst. 45:125544. doi: 10.1016/j.ppees.2020.125544
Amos, W., and Harwood, J. (1998). Factors affecting levels of genetic diversity in natural populations. Philos. Trans. R. Soc. Lond. B 353, 177–186. doi: 10.1098/rstb.1998.0200
Angeloni, F., Ouborg, N. J., and Leimu, R. (2011). Meta-analysis on the association of population size and life history with inbreeding depression in plants. Biol. Conserv. 144, 35–43. doi: 10.1016/j.biocon.2010.08.016
Araújo, F. S., Felix, F. C., Ferrari, C. S., Vieira, F. A., and Pacheco, M. V. (2020). Seed quality and genetic diversity of a cultivated population of Mimosa caesalpiniifolia Benth. Caatinga 33, 1000–1006. doi: 10.1590/1983-21252020v33n415rc
Arruda, G. O. S. F., Mantovani, A., Montagna, T., Bernardi, A. P., da Costa, N. C. F., and Reis, M. S. (2019). Genetic diversity of Mimosa scabrella Benth. In two populations located at landscapes with different historical. Sci. For. 47, 744–753. doi: 10.18671/scifor.v47n124.16
Arruda, G. O. S. F., Mantovani, A., Montagna, T., Bernardi, A. P., da Costa, N. C. F., and Reis, M. S. (2020). Reproductive system of Mimosa scabrella in consecutive reproductive events in the mountainous region of Santa Catarina state. Floresta 50, 1259–1266. doi: 10.5380/rf.v50i2.62515
Ball, J. W., Robinson, T. P., Wardell-Johnson, G. W., Bovill, J., Byrne, M., and Nevill, P. G. (2020). Fine-scale species distribution modelling and genotyping by sequencing to examine hybridisation between two narrow endemic plant species. Sci. Rep. 10:1562. doi: 10.1038/s41598-020-58525-2
Bani, L., Orioli, V., Pisa, G., Dondina, O., Fagiani, S., Fabbri, E., et al. (2018). Landscape determinants of genetic differentiation, inbreeding and genetic drift in the hazel dormouse (Muscardinus avellanarius). Conserv. Genet. 19, 283–296. doi: 10.1007/s10592-017-0999-6
Bao, W., Ao, D., Wuyun, T., Wang, L., and Bai, Y. (2020). Development of 85 SNP markers for the endangered plant species Prumus mira (Rosaceae) based on restriction site associated DNA sequencing (RAD-seq). Conserv. Genet. Resour. 12, 525–527. doi: 10.1007/s12686-020-01140-0
Bard, N. W., Miller, C. S., and Bruederle, L. (2021). High genomic diversity maintained by populations of Carex scirpoidea supsp. Convolute, a paraphyletic Great Lakes ecotype. Conserv. Genet. 22, 169–185. doi: 10.1007/s10592-020-01326-x
Barneby, C. R. (1991). Sensitivae censitae: a description of the genus Mimosa Linnaeus (Mimosaceae) in the New World. Mem. N. Y. Bot. Gard. 65, 1–835.
Barret, S. C. H., and Kohn, J. R. (1991). “Genetic and evolutionary consequences of small population size in plants: Implications for conservation,” in Genetics and Conservation of Rare Plants. eds. D. A. Falk and K. E. Holsinger (Oxford: Oxford University Press), 3–30.
Bechara, F. C., Reis, A., and Trentin, B. E. (2013). Invasão biológica de Pinus elliottii var. elliottii no Parque Estadual do Rio Vermelho, Florianópolis, SC. Floresta 44, 63–72. doi: 10.5380/rf.v44i1.32013
Berenhauser, H. (n.d.). Reflorestamento de mil hectares de dunas e alagados na Ilha de Santa Catarina. Acervo “Henrique Berenhauser.” Setor de Obras Raras da Biblioteca Universitária da Universidade Federal de Santa Catarina. 3.
Bevill, R. L., and Louda, S. M. (1999). Comparison of related rare and common species in the study of plant rarity. Conserv. Biol. 13, 493–498. doi: 10.1046/j.1523-1739.1999.97369.x
Bradbury, D., Binks, R. M., and Byrne, M. (2021). Genomic data inform conservation of rare tree species: clonality, diversity and hybridity in eucalyptus series in a global biodiversity hotspot. Biodivers. Conserv. 30, 619–641. doi: 10.1007/s10531-020-02106-2
Bupp, G., Ricono, A., Peterson, C. L., and Pruett, C. L. (2017). Conservation implications of small population size and habitat fragmentation in an endangered lupine. Conserv. Genet. 18, 77–88. doi: 10.1007/s10592-016-0883-9
Burkart, A. (1979). Flora ilustrada catarinense: Leguminosas − Mimosoideas. Itajaí: Herbário Barbosa Rodrigues, 299.
Burnham, K. P., and Anderson, D. R. (2001). Kullback-Leibler information as a basis for strong inference in ecological studies. Wildl. Res. 28, 111–119. doi: 10.1071/WR99107
Cabrera-Toledo, D., González-Astorga, J., and Vovides, A. P. (2008). Heterozygote excess in ancient populations of the critically endangered Dioon caputoi (Zamiaceae, Cycadales) from Central Mexico. Bot. J. Linn. Soc. 158, 436–447. doi: 10.1111/j.1095-8339.2008.00868.x
Caro, T. M., and Laurenson, M. K. (1994). Ecological and genetic factors in conservation: a cautionary tale. Science 263, 485–486. doi: 10.1126/science.8290956
Catchen, J. M., Amores, A., Hohenlohe, P., Cresko, W., and Postlethwait, J. H. (2011). STACKS: building and genotyping loci de novo from short-read sequences. G3 1, 171–182. doi: 10.1534/g3.111.000240
Catchen, J., Hohenlohe, P. A., Bassham, S., Amores, A., and Cresko, W. A. (2013). Stacks: an analysis tool set for population genomics. Mol. Ecol. 22, 3124–3140. doi: 10.1111/mec.12354
Caughley, G., and Gunn, A. (1996). Conservation Biology in Theory and Practice. Cambridge, MA: Blackwell Scientific.
Charlesworth, D., and Charlesworth, B. (1987). Inbreeding depression and its evolutionary consequences. Annu. Rev. Ecol. Syst. 18, 237–268. doi: 10.1146/annurev.es.18.110187.001321
Chattopadhyay, B., Garg, K. M., Soo, Y. J., Low, G. W., Frechette, J. L., and Rheindt, E. (2019). Conservation genomics in the fight to help the recovery of the critically endangered Siamese crocodile Crocodylus siamensis. Mol. Ecol. 28, 936–950. doi: 10.1111/mec.15023
Chen, Y., Liu, Y., Song, M., Lai, J., and Gong, Q. (2020). Isolation and characterization of 47 SNP markers in the critically endangered Acipenser dabryanus. Conserv. Genet. Resour. 12, 9–12. doi: 10.1007/s12686-018-1052-x
Choudary, D., Rai, M. K., and Shekhawat, N. S. (2020). In vitro propagation of Farsetia macrantha blatt. & Hallb.: an endemic and threatened plant of Indian Thar Desert. Plant Cell Tissue Organ Cult. 142, 519–526. doi: 10.1007/s11240-020-01876-5
CONSEMA (2014). “Reconhece a lista oficial das espécies da flora ameaçada de extinção no Estado de Santa Catarina e dá outras providências”. Resolução 51, de 05 de dezembro de 2014. Available at: https://www.ima.sc.gov.br/index.php/downloads/biodiversidade/flora/2435-lista-das-especies-vegetais-catarinenses
Coppi, A., Mengoni, A., and Selvi, F. (2008). AFLP fingerprinting of Anchusa (Boraginaceae) in the Corso-Sardinian system: genetic diversity, population differentiation and conservation priorities in an insular endemic group threatened with extinction. Biol. Conserv. 141, 2000–2011. doi: 10.1016/j.biocon.2008.05.015
De Castro, O., Sepe, F., Di Maio, A., Cennamo, P., De Luca, P., Gianguzzo, L., et al. (2013). Genetic structure in the paleoendemic and endangered Petagnaea gussonei (Spreng.) Rauschert (Saniculoideae, Apiaceae) and implications for its conservation. Plant Syst. Evol. 299, 209–223. doi: 10.1007/s00606-012-0716-3
De Kort, H., Prunier, J. G., Ducatez, S., Honnay, O., Baguette, M., Stevens, V. M., et al. (2021). Life history, climate and biogeography interactively affect worldwide genetic diversity of plant and animal populations. Nat. Commun. 12:516. doi: 10.1038/s41467-021-20958-2
Dev, S. A., Shenoy, M., and Borges, R. (2010). Genetic and clonal diversity of the endemic ant-plant Humboldtia brunonis (Fabaceae) in the Western Ghats of India. J. Biosci. 35, 267–279. doi: 10.1007/s12038-010-0031-5
Dobson, A. P. (1999). “Introduction: genetics and conservation biology,” in Genetics and the Extinction of Species. eds. L. F. Landweber and A. Dobson (Princeton, NJ: Princeton University Press), 575.
Downey, K. M., and Baskauf, C. J. (2020). Population genetics of a rare wetland species, the Tennessee yellow-eyed grass (Xyris tenesseensis, Xyridaceae). Conserv. Genet. 21, 735–746. doi: 10.1007/s10592-020-01285-3
Edwards, C. E., Lindsay, D. L., Bailey, P., and Lancey, R. F. (2014). Patterns of genetic diversity in the rare Erigeron lemmoni and comparison with its more widespread congener, Erigeron arisolius (Asteraceae). Conserv. Genet. 15, 419–428. doi: 10.1007/s10592-013-0549-9
Edwards, C. E., Tessier, B. C., Swift, J. F., Bassssüner, B., Linan, A. G., Albrecht, M. A., et al. (2021). Conservation genetics of the threatened plant species Physaria filiformis (Missouri bladderpod) reveals strong genetic structure and a possible cryptic species. PLoS One 16:e0247586. doi: 10.1371/journal.pone.0247586
Elegren, H., and Galtier, N. (2016). Determinants of genetic diversity. Nat. Rev. Genet. 17, 422–433. doi: 10.1038/nrg.2016.58
Ellis, J. R., Pashley, C. H., Burke, J. M., and McCauley, D. E. (2006). High genetic diversity in a rare and endangered sunflower as compared to a common congener. Mol. Ecol. 15, 2345–2355. doi: 10.1111/j.1365-294X.2006.02937.x
Ellstrand, N. C., and Elam, D. R. (1993). Population genetic consequences of small population size: implications for plant conservation. Annu. Rev. Ecol. Syst. 24, 217–242. doi: 10.1146/annurev.es.24.110193.001245
Excoffier, L., Dupanloup, A., Huerta-Sánchez, E., Sousa, V. C., and Foll, M. (2013). Robust demographic inference from genomic and SNP data. PLoS Genet. 10:e1003905. doi: 10.1371/journal.pgen.1003905
Excoffier, L., and Lischer, H. E. L. (2010). Arlequin suite ver. 3.5: a new series of programs to perform population genetics analyses under Linux and windows. Mol. Ecol. Resour. 10, 564–567. doi: 10.1111/j.1755-0998.2010.02847.x
Falconer, D. S. (1989). Introduction to Quantitative Genetics. 3rd Edn. New York: Longman Scientific and Technical.
Feres, J. M., Nazareno, A. G., Borges, L. M., Guidugli, M. C., Bonifacio-Anacleto, F., and Alzate-Marin, A. L. (2021). Depicting the mating system and patterns of contemporary pollen flow in trees of the genus Anadenanthera (Fabaceae). PeerJ 9:e10579. doi: 10.7717/peerj.10579
Feres, J. M., Sebbenn, A. M., and Guidugli, M. C. (2012). Mating system parameters at hierarchical levels of fruits, individuals and populations in the Brazilian insect-pollinated tropical tree, Tabebuia roseo-alba (Bignoniaceae). Conserv. Genet. 13, 293–405. doi: 10.1007/s10592-011-0292-z
Fernández-Mazuecos, M., Jiménez-Mejías, P., Rotllan-Puig, X., and Vargas, P. (2014). Narrow endemics to Mediterranean islands: moderate genetic diversity but narrow climatic niche of the ancient, critically endangered Naufraga (Apiaceae). Perspect. Plant Ecol. Evol. Syst. 16, 190–202. doi: 10.1016/j.ppees.2014.05.003
Ferreira, F. A. C. (2010). Projeto Parque Estadual do Rio Vermelho: Subsídios ao plano de manejo. Florianópolis: Insular.
Finlay, C. M. V., Bradley, C. R., Preston, J., and Provan, J. (2017). Low genetic diversity and potential inbreeding in an isolated population of alder buckthorn (Frangula alnus) following a founder effect. Sci. Report. 7:3010. doi: 10.1038/s41598-017-03166-1
Forrest, A., Escudero, M., Heuertz, M., Wilson, Y., Cano, E., and Vargas, P. (2017). Testing the hypothesis of low genetic diversity and population structure in narrow endemic species: the endangered antirrhinum charidemi (Plantaginaceae). Bot. J. Linn. Soc. 183, 260–270. doi: 10.1093/botlinnean/bow002
Frankham, R. (1995). Conservation genetics. Annu. Rev. Genet. 29, 305–327. doi: 10.1146/annurev.ge.29.120195.001513
Frankham, R. (1996). Relationship of genetic variation to population size in wildlife. Conserv. Biol. 10, 1500–1508. doi: 10.1046/j.1523-1739.1996.10061500.x
Frankham, R., Ballou, J. D., and Briscoe, D. A. (2010). Introduction to Conservation Genetics. Vol. 2. Cambridge: Cambridge University Press.
Frankham, R., Ballou, J. D., Ralls, K., Eldrigde, B., Dudash, M. R., Fenster, C. B., et al. (2017). Genetic Management of Fragmented Animal and Plant Populations. Oxford: Oxford University Press.
Frankham, R., Bradshaw, C. J. A., and Brook, B. W. (2014). Genetics in conservation management: revised recommendations for the 50/500 rules, red list criteria and population viability analyses. Biol. Conserv. 170, 56–63. doi: 10.1016/j.biocon.2013.12.036
Frankham, R., and Ralls, K. (1998). Conservation biology: inbreeding leads to extinction. Nature 392, 441–442. doi: 10.1038/33022
Funk, W. C., McKay, J. K., Hohenlohe, P. A., and Allendorf, F. W. (2012). Harnessing genomics for delineating conservation units. Trends Ecol. Evol. 27, 489–496. doi: 10.1016/j.tree.2012.05.012
Gaddis, K. D., Zukin, H. L., Dieterich, I. A., Braker, E., and Sork, V. L. (2014). Effect of clonal reproduction on genetic structure in Pentaclethra macroloba (Fabaceae: Mimosoideae). Rev. Biol. Trop. 62, 443–454. doi: 10.15517/rbt.v62i2.11639
Garcia-Jacas, N., Requena, J., Massó, S., Vilatersana, R., Blanche, C., and López-Pujol, J. (2021). Genetic diversity and structure of the narrow endemic Seseli farrenyi (Apiaceae): implications for translocation. PeerJ 9:e10521. doi: 10.7717/peerj.10521
Gasper, A. L., Meyer, L., Sevegnani, L., Sobral, M., and Bonnet, A. (2012). “Flora Vascular de Santa Catarina,” in Inventário Florístico Florestal de Santa Catarina, Vol. I, Diversidade e conservação dos remanescentes florestais. eds. A. C. Vibrans, L. Sevegnani, A. L. Gasper, and D. V. Lingner (Blumenau: Edifurb), 99–111.
Glémin, S., Bazin, E., and Charlesworth, D. (2006). Impact of mating systems on patterns of sequence polymorphism in flowering plants. Proc. R. Soc. B 273, 3011–3019. doi: 10.1098/rspb.2006.3657
Godoy, F. M. R., Lenzi, M., Ferreira, B. H. S. F., da Silva, L. V., Zanella, C. M., and Paggi, G. M. (2018). High genetic diversity and moderate genetic structure in the self-incompatible, clonal Bromelia hieronymi (Bromeliaceae). Bot. J. Linn. Soc. 187, 672–688. doi: 10.1093/botlinnean/boy037
Goetze, M., Capra, F., Büttow, M. V., Zanella, C. M., and Bered, F. (2018). High genetic diversity and demographic stability in Aechmea kertesziae (Bromeliaceae), a species of sandy coastal plains (Restinga habitat) in southern Brazil. Bot. J. Linn. Soc. 186, 374–388. doi: 10.1093/botlinnean/box103
Gui, D., Zeng, F., Liu, Z., and Zhang, B. (2013). Characteristics of the clonal propagation of Alhagi sparsifolia Shap. (Fabaceae) under different groundwater depths in Xinjiang, China. Rangel. J. 35, 355–362. doi: 10.1071/RJ13004
Guidugli, M. C., Nazareno, A. G., Feres, J. M., Contel, E. P. B., Mestriner, M. A., and Alzate-Marin, A. L. (2016). Small but not isolated: a population genetic survey of the tropical tree Cariniana estrellensis (Leythidaceae) in a highly fragmented habitat. Heredity 116, 339–347. doi: 10.1038/hdy.2015.108
Hamrick, J. L., and Godt, M. J. W. (1989). “Allozyme diversity in plant species,” in Plant Population Genetics, Breeding and Genetic Resources. eds. A. H. D. Brown, M. T. Clegg, A. L. Kahler, and B. S. Weir (Sunderland, Massachusetts, USA: Sinauer Associates), 43–63.
Hamrick, J. T., and Godt, M. J. W. (1996). Effects of life history traits on genetic diversity in plant species. Philos. Trans. R. Soc. B 351, 1291–1298. doi: 10.1098/rstb.1996.0112
Hardy, O. J., and Vekemans, X. (2002). SPAGeDi: a versatile computer program to analyze spatial genetic structure ate the individual or population levels. Mol. Ecol. Notes 2, 618–620. doi: 10.1046/j.1471-8286.2002.00305.x
Hassemer, G., Ferreira, P. M. A., and Trevisan, R. (2015). A review of vascular plant endemisms in Santa Catarina, southern Brazil, highlights critical knowledge gaps and urgent need of conservation efforts. J. Torrey Bot. Soc. 142, 78–95. doi: 10.3159/TORREY-D-14-00033.1
Hawkins, M. T. R., Culligan, R. R., Frasier, C. L., Dikow, R. B., Hagenson, R., Lei, R., et al. (2018). Genome sequence and population declines in the critically endangered greater bamboo lemur (Prolemur simus) and implications for conservation. BMC Genomics 19:445. doi: 10.1186/s12864-018-4841-4
Heberle, D. A. (2011). Heterogeneidade Ambiental do Parque Estadual do Rio Vermelho, Florianópolis-SC. Master's thesis. University of the State of Santa Catarina.
Hedrick, P. W., Lacy, R. C., Allendorf, F. W., and Soulé, M. E. (1995). Directions in conservation biology: comments on caughley. Conserv. Biol. 10, 1312–1320.
Honnay, O., and Jacquemyn, H. (2007). Susceptibility of common and rare plant species to the genetic consequences of habitat fragmentation. Conserv. Biol. 21, 823–831. doi: 10.1111/j.1523-1739.2006.00646.x
Huenneke, L. F. (1991). “Ecological implications of genetic variation,” in Genetics and Conservation of Rare Plants. eds. D. A. Falk and K. E. Holsinger (Oxford: Oxford University Press), 3–30.
Husband, B. C., and Schemske, D. W. (1996). Evolution of the magnitude and timing of inbreeding depression in plants. Evolution 50, 54–70. doi: 10.1111/j.1558-5646.1996.tb04472.x
Huy, N. V., Hung, H. V., Buckney, R. T., and De Filippis, L. F. (2018). “Molecular biodiversity convergence with biogeography and ethnobotany of rare and endangered medicinal plants from northern Vietnam,” in Plant and Human Health. Vol. 1. eds. M. Ozturk and K. Hakeem (Cham: Springer).
IMA (2020). Plano de manejo do Parque Estadual do Rio Vermelho. Instituto do Meio Ambiente de Santa Catarina.
Jiménez, A., Mansour, H., Keller, B., and Conti, E. (2014). Low genetic diversity and high levels of inbreeding in the Sinai primrose (Primula boveana), a species on the brink of extinction. Plant Syst. Evol. 300, 1199–1208. doi: 10.1007/s00606-013-0955-y
Jiménez-Mejías, P., Fernández-Mazuecos, M., Amat, M. E., and Vargas, P. (2015). Narrow endemics in European mountains: high genetic diversity within the monospecific genus Pseudomisopates (Plantaginaceae) despite isolation since the late Pleistocene. J. Biogeogr. 42, 1455–1468. doi: 10.1111/jbi.12507
Jombart, T. (2008). Adegenet: a R package for the multivariate analysis of genetic markers. Bioinformatics 24, 1403–1405. doi: 10.1093/bioinformatics/btn129
Jombart, T., and Ahmed, I. (2011). Adegenet 1.3-1: new tools for the analysis of genome-wide SNP data. Bioinformatics 27, 3070–3071. doi: 10.1093/bioinformatics/btr521
Kamvar, Z. N., Brooks, J. C., and Grünwald, N. J. (2015). Novel R tools for analysis of genome-wide population genetic data with emphasis on clonality. Front. Genet. 6:208. doi: 10.3389/fgene.2015.00208
Kamvar, Z. N., Tabima, J. F., and Grünwald, N. J. (2014). Poppr: an R package for genetic analysis of populations with clonal, partially clonal, and/or sexual reproduction. PeerJ 2:e281. doi: 10.7717/peerj.281
Karasawa, M. M. G. (2015). Reproductive Diversity of Plants: An Evolutionary Perspective and Genetic Basis. New York: Springer, 87.
Keenan, K., McGinnity, P., Cross, T. F., Crozier, W. W., and Prodöhl, P. A. (2013). DiveRsity: an R package for the estimation and exploration of population genetics parameters and their associated errors. Methods Ecol. Evol. 4, 782–788. doi: 10.1111/2041-210X.12067
Kimura, M., and Crow, J. F. (1964). The number of alleles that can be maintained in a finite population. Genetics 49, 725–738. doi: 10.1093/genetics/49.4.725
Kokubun, H., Nakano, M., Tsukamoto, T., Watanabe, H., Hashimoto, G., Marchesi, E., et al. (2006). Distribution of self-compatible and self-incompatible populations of Petunia axillaris (Solanaceae) outside Uruguay. J. Plant Res. 119, 419–430. doi: 10.1007/s10265-006-0002-y
Lande, R. (1988). Genetics and demography on biological conservation. Science 241, 1455–1460. doi: 10.1126/science.3420403
Lanes, E. C., Pope, N. S., Alves, R., Filho, N. M. C., Giannini, T. C., Giullete, A. M., et al. (2018). Landscape genomic conservation assessment of a narrow endemic and widespread morning glory from Amazonian Savannas. Front. Plant Sci. 9:532. doi: 10.3389/fpls.2018.00532
Lerin, J., Ribeiro, Y. R. S., and Oliveira, T. R. (2021). Histomorphology and proteomics during rooting of in vitro shoots in Cariniana legalis (Lecythidaceae), a dificult-to-root endangered species from the Brazilian Atlantic Forest. Plant Cell Tissue Organ Cult. 144, 325–344. doi: 10.1007/s11240-020-01955-7
Lischer, H. E. L., and Excoffier, L. (2012). PGDSpider: an automated data conversion tools for connecting population genetics and genomics programs. Bioinformatics 28, 298–299. doi: 10.1093/bioinformatics/btr642
Liu, D., Zhang, L., Wang, J., and Ma, Y. (2020). Conservation genomics of a threatened rhododendron: contrasting patterns of population structure revealed from neutral and selected SNPs. Front. Genet. 11:757. doi: 10.3389/fgene.2020.600227
Loiselle, B. A., Sork, V. L., Nason, J., and Graham, C. (1995). Spatial genetic structure of a tropical understory shrub, Psychotria officinalis (Rubiaceae). Am. J. Bot. 82, 1420–1425. doi: 10.1002/j.1537-2197.1995.tb12679.x
Luikart, G., Allendorf, F. W., Cornuet, J.-M., and Sherwin, W. B. (1998). Distortion of allele frequency distributions provides a test for recent population bottlenecks. J. Hered. 89, 238–247. doi: 10.1093/jhered/89.3.238
Mameli, G., Filigheddu, R., Binelli, G., and Meloni, M. (2008). The genetic structure of the remnant populations of Centaurea horrida in Sardinia and associated islands. Ann. Bot. 101, 633–640. doi: 10.1093/aob/mcn012
Martel, C., Scopece, G., Cozzolino, S., Ayasse, M., Pinheiro, F., and Cafasso, D. (2021). Genetic diversity in natural populations of the endangered neotropical orchid Tellipogon peruvianus. Plant Species Biol. 26, 6–16. doi: 10.1111/1442-1984.12291
Mastretta-Yanes, A., Arrigo, N., Alvarez, N., Jorgensen, T. H., Piñero, D., and Emerson, B. C. (2015). Restriction site-associated DNA sequencing, genotyping error estimation and de novo assembly optimization for population genetic inference. Mol. Ecol. Resour. 15, 28–41. doi: 10.1111/1755-0998.12291
Mayol, M., Palau, C., Rosselló, J. A., González-Martínez, S. C., Molins, A., and Riba, M. (2012). Patterns of genetic variability and habitat occupancy in Crepis triasii (Asteraceae) at different spatial scales: insights on evolutionary processes leading to diversification in continental islands. Ann. Bot. 109, 429–441. doi: 10.1093/aob/mcr298
Medeiros, J. D., and Stefani, M. R. (2018). Anatomia da madeira de Mimosa catharinensis Burkart (Leguminosae—Mimosoideae). Biotemas 31, 11–19. doi: 10.5007/2175-7925.2018v31n1p11
Mishra, M. K., Pandey, S., Misra, P., Niranjan, A., and Srivastava, A. (2020). An efficient protocol for clonal regeneration and excised root culture with enhanced alkaloid content in Thalictrum foliolosum DC.-an endemic and important medicinal plant of temperate Himalayan region. Ind. Crop. Prod. 152:112504. doi: 10.1016/j.indcrop.2020.112504
Montagna, T., Silva, J. Z., Pikart, T. G., and Reis, M. S. (2018). Reproductive ecology of Ocotea catharinensis, an endangered tree species. Plant Biol. 20, 926–935. doi: 10.1111/plb.12847
Morales, M., Giannoni, F., Inza, M. V., Soldati, M. C., Bessega, C. F., Poggio, L., et al. (2020). Genetic and morphological diversity and population structure of a polyploid complex of Mimosa (Leguminosae). Syst. Biodivers. 18, 237–254. doi: 10.1080/14772000.2019.1696421
Moreira, P. A., Steenbock, W., Peroni, N., and Reis, M. S. (2011). Genetic diversity and mating system of bracatinga (Mimosa scabrella) in a re-emergent agroforestry system in southern Brazil. Agrofor. Syst. 83, 245–256. doi: 10.1007/s10457-011-9428-x
Mori, H., Ueno, S., Matsumoto, A., Kamijo, T., Tsumura, Y., and Masaki, T. (2018). Large contribution of clonal reproduction to the distribution of deciduous liana species (Wisteria floribunda) in an old-growth cool temperate forest: evidence from genetic analysis. Ann. Bot. 121, 359–365. doi: 10.1093/aob/mcx153
Nazareno, A. G., Bemmels, J. B., Dick, C. W., and Lohmann, L. G. (2017). Minimum sample sizes for population genomics: an empirical study from an Amazonian plant species. Mol. Ecol. Resour. 17, 1136–1147. doi: 10.1111/1755-0998.12654
Nazareno, A. G., and Carvalho, D. (2009). What the reasons for no inbreeding and high genetic diversity of the neotropical fig tree Ficus arpazusa? Conserv. Genet. 10, 1789–1793. doi: 10.1007/s10592-008-9776-x
Nazareno, A. G., and Knowles, L. L. (2021). There is no ‘rule of thumb’: genomic filter settings for a small plant population to obtain unbiased gene flow estimates. Front. Plant Sci. 12:626405. doi: 10.3389/fpls.2021.626405
Nazareno, A. G., and Reis, M. S. (2012). Linking phenology to mating system: exploring the reproductive biology of the threatened palm species Butia eriospatha. J. Hered. 103, 842–852. doi: 10.1093/jhered/ess070
Nei, M., and Roychoudhury, A. K. (1974). Sampling variances of heterozygosity and genetic distance. Genetics 76, 379–390. doi: 10.1093/genetics/76.2.379
Oleas, N. H., Von Wettberg, E. J. B., and Negrón-Ortiz, V. (2014). Population genetics of the federally threatened miccosukee gooseberry (Ribes echinellum), an endemic north American species. Conserv. Genet. 15, 749–755. doi: 10.1007/s10592-014-0575-2
Oliveira, U., Soares-Filho, B. S., Paglia, A. P., Brescovit, A. D., de Carvalho, J. B., Silva, D. P., et al. (2017). Biodiversity conservation gaps in the Brazilian protected areas. Sci. Rep. 7:9141. doi: 10.1038/s41598-017-08707-2
Ossowski, S., Schneeberger, K., Lucas-Lledo, J. I., Warthman, N., Clark, R. M., Shaw, R. G., et al. (2010). The rate and molecular spectrum of spontaneous mutations in Arabidopsis thaliana. Science 327, 92–94. doi: 10.1126/science.1180677
Paradis, E. (2010). Pegas: an R package for population genetics with an integrated-modular approach. Bioinformatics 26, 419–420. doi: 10.1093/bioinformatics/btp696
Peakall, R., and Smouse, P. E. (2012). GenAlEx 6.5: genetic analysis in excel. Populations genetic software for teaching and research: an update. Bioinformatics 28, 2537–2539. doi: 10.1093/bioinformatics/bts460
Perrier, C., Ferchaud, A., Sirois, P., Thibault, I., and Bernatchez, L. (2017). Do genetic drift and accumulation of deleterious mutations preclude adaptation? Empirical investigation using RADseq in a northern lacustrine fish. Mol. Ecol. 26, 6317–6335. doi: 10.1111/mec.14361
Peterson, B. K., Weber, J. N., Kay, E. H., Fisher, H. S., and Hoekstra, H. E. (2012). Double digest RADseq: an inexpensive method for de novo SNP discovery and genotyping in model and non-model species. PLoS One 7:e37135. doi: 10.1371/journal.pone.0037135
Pramual, P., Khumkratok, S., and Wongpakam, K. (2011). Population genetics of invasive weed Mimosa pigra L. (Mimosoideae) in Thailand. Pak. J. Bot. 43, 2721–2726.
Radosavljević, I., Satovic, Z., and Liber, Z. (2015). Causes and consequences of contrasting genetic structure in sympatrically growing and closely related species. AoB Plants 7:plv106. doi: 10.1093/aobpla/plv106
R Core Team (2019). R: A Language and Environment for Statistical Computing. R Foundation for Statistical Computing.
Reed, D. H., and Frankham, R. (2003). Correlation between fitness and genetic diversity. Conserv. Biol. 17, 230–237. doi: 10.1046/j.1523-1739.2003.01236.x
Rhoads, E. A., Williams, P. K., and Krane, C. M. (2017). High inbreeding and low connectivity among Aambystoma texanum populations in fragmented Ohio forests. Ecol. Evol. 7, 11135–11147. doi: 10.1002/ece3.3637
Rice, W. R. (1989). Analyzing tables of statistical tests. Evolution 43, 223–225. doi: 10.1111/j.1558-5646.1989.tb04220.x
Rochette, N. C., Rivera-Colon, A. G., and Catchen, J. M. (2019). Stacks 2: analytical methods for paired-end sequencing improve RADseq-based population genomics. Mol. Ecol. 28, 4737–4754. doi: 10.1111/mec.15253
Rödin-Mörch, P., Luquet, E., Meyer-Lucht, Y., Richter-Boix, A., Höglund, J., and Laurilla, A. (2019). Latitudinal divergence in a widespread amphibian: contrasting patterns of neutral and adaptive genomic variation. Mol. Ecol. 28, 2996–3011. doi: 10.1111/mec.15132
Rodrigues, D. M., Turchetto, C., Lima, J. S., and Freitas, L. B. (2019). Diverse yet endangered: pollen dispersal and mating system reveal inbreeding in a narrow endemic plant. Plant Ecol. Divers. 12, 169–180. doi: 10.1080/17550874.2019.1610914
Rogalski, J. M., Drechsler-Santos, E. R., Locatelli, L., de Moraes, L. K., Groposo, C., Silva, C. V., et al. (2005). “Estrutura demográfica de Mimosa catharinensis Burk (Leguminosae), uma espécie endêmica da Ilha de Santa Catarina, Florianópolis, SC.” in Resumos do 56° Congresso Nacional de Botânica 2005.
Sales, E., Nebauer, S. G., Mus, M., and Segura, J. (2001). Population genetic study in the Balearic endemic plant species Digitalis minor (Scrophulariaceae) using RAPD markers. Am. J. Bot. 88, 1750–1759. doi: 10.2307/3558349
Santos, J. A., Trazzi, P. A., Rocha, L. F., Cunha, F. L., and Carvalho, D. (2019). “Cultura de tecidos vegetais na conservação da biodiversidade,” in Ciências biológicas campo promissor em pesquisa 2. ed. R. M. Freitas (Ponta Grossa: Atena), 243.
Schliep, K. P. (2011). Phangorn: phylogenetic analysis in R. Bioinformatics 27, 592–593. doi: 10.1093/bioinformatics/btq706
Seijo, J. G., and Neffa, V. G. S. (2004). The cytological origin of the polyads and their significance in the reproductive biology of Mimosa bimucronata. Bot. J. Linn. Soc. 144, 343–349. doi: 10.1111/j.1095-8339.2003.00253.x
Sękiewicz, K., Walas, Ł., Beridze, B., Fennane, M., and Dering, M. (2020). High genetic diversity and low future habitat suitability: will Cupressus atlantica, endemic to the high atlas, survive under climate change? Reg. Environ. Chang. 20:132. doi: 10.1007/s10113-020-01711-9
Sherif, N. A., Benjamin, J. H. F., Kumar, T. S., and Rao, M. V. (2018). Somatic embryogenesis, acclimatization and genetic homogeneity assessment of regenerated plantlets of Anoectochilus elatus Lindl., an endangered terrestrial jewel orchid. Plant Cell Tissue Organ Cult. 132, 303–316. doi: 10.1007/s11240-017-1330-4
Silva, L. A., Guimarães, E., Rossi, M. N., and Maimoni-Rodella, R. C. S. (2011). Reproduction biology of mimosa bimucronata: a ruderal species. Planta Daninha 29, 1011–1021. doi: 10.1590/S0100-83582011000500007
Silva, C. V., Martins, M., Gaio, M. F., Moraes, L. K. A., Silveira, C. I. G., Rogalski, J. M., et al. (2005). “Aspectos da biologia reprodutiva de Mimosa catharinensis Burk. (Leguminosae) no Parque Estadual do Rio Vermelho, Florianópolis, SC.” in Resumos do 56° Congresso Nacional de Botânica 2005.
Silva, A. R., Resende-Moreira, L. C., Carvalho, C. S., Lanes, E. C. M., Ortiz--Vera, M. P., Viana, P. L., et al. (2020). Range-wide neutral and adaptive genetic structure of an endemic herb from Amazonian Savannas. AoB Plants 12:plaa003. doi: 10.1093/aobpla/plaa003
Simmons, L., Mathieson, M. T., Lamont, R. W., and Shapcott, A. (2018). Genetic diversity of endangered orchid Phaius australis across a fragmented Australian landscape. Conserv. Genet. 19, 451–4665. doi: 10.1007/s10592-017-1022-y
Simon, M. F., and Hay, J. D. (2003). Comparison of a common and rare species of Mimosa (Mimosaceae) in Central Brazil. Austral Ecol. 28, 315–326. doi: 10.1046/J.1442-9993.2003.01291.X
Spielman, D., Brook, B. W., and Frankham, R. (2004). Most species are not driven to extinction before genetic factors impact them. Proc. Natl. Acad. Sci. 101, 15261–15264. doi: 10.1073/pnas.0403809101
Spoladore, J., Mansano, V. F., Lemes, M. R., Freitas, L. C. D., and Sebbenn, A. M. (2017). Genetic conservation of small populations of the endemic tree Swartzia glazioviana (Taub.) Glaz. (Leguminosae) in the Atlantic Forest. Conserv. Genet. 18, 1105–1117. doi: 10.1007/s10592-017-0962-6
Sujii, O. S., Tambarussi, E. V., Grando, C., Silvestre, E. A., Viana, J. P. G., Brancalion, P. H. S., et al. (2021). High gene flow through pollen partially compensates spatial limited gene fow by seeds for a Neotropical tree in forest conservation and restoration areas. Conserv. Genet. 22, 383–396. doi: 10.1007/s10592-021-01344-3
Taimanga,, and Fohouo, F. T. (2018). Pollination efficiency of Apis mellifera Linnaeus 1758 (hymenoptera, Apidae) on Mimosa pudica Linnaeus 1753 (Fabaceae) inflorescences at Yassa (Douala, Cameroon). J. Entomol. Zool. Stud. 6, 2027–2033.
Thomas, W. J. W., Anthony, J. M., Dobrowolski, M. P., and Krauss, A. L. (2021). Optimizing the conservation of genetic diversity of the last remaining population of a critically endangered shrub. AoB Plants 13:plab005. doi: 10.1093/aobpla/plab005
Toczydlowski, R. H., and Waller, D. M. (2019). Drift happens: molecular genetic diversity and differentiation among populations of jewelweed (Impatiens capensis Merrb.) reflect fragmentation of foodplain forests. Mol. Ecol. 28, 2495–2475. doi: 10.1111/mec.15072
Turchetto, C., Segatto, A. L. A., Mäder, G., Rodrigues, D. M., Bonatto, S. L., and Freitas, L. B. (2016). High levels of genetic diversity and population structure in an endemic and rare species: implications for conservation. AoB Plants 8:plw002. doi: 10.1093/aobpla/plw002
Turchetto-Zolet, A. C., Pinheiro, F., Salgueiro, F., and Palma-Silva, C. (2013). Phylogeographical patterns shed light on evolutionary process in South America. Mol. Ecol. 22, 1193–1213. doi: 10.1111/mec.12164
Vibrans, A. C., Sevegnani, L., Gasper, A. L., and Lingner, D. V. (2012). “Inventário Florístico Florestal de Santa Catarina,” in Diversidade e conservação dos remanescentes florestais. Vol. 1. eds.
Waits, L. P., Luikart, G., and Taberlet, P. (2001). Estimating the probability of identity among genotypes in natural populations: cautions and guidelines. Mol. Ecol. 10, 249–256. doi: 10.1046/j.1365-294X.2001.01185.x
Wang, S. (2020). Genetic diversity and population structure of the endangered species Paeonia decomposita endemic to China and implications for its conservation. BMC Plant Biol. 20:510. doi: 10.1186/s12870-020-02682-z
Ward, M., Dick, C. W., Gribel, R., and Lowe, A. J. (2005). To self, or not to self… a review of outcrossing pollen-mediated gene flow in neotropical trees. Heredity 95, 246–254. doi: 10.1038/sj.hdy.6800712
Wright, B. R., Farquharson, K. A., McLennan, E. A., Belov, K., and Hogg, C. J. (2020). A demonstration of conservation genomics for threatened species management. Mol. Ecol. Resour. 20, 1526–1541. doi: 10.1111/1755-0998.13211
Wright, S. I., Kalisz, S., and Slotte, T. (2013). Evolutionary consequences of self-fertilization in plants. Proc. R. Soc. B 280:20130133. doi: 10.1098/rspb.2013.0133
Zang, Y., Luan, P., Ren, G., Hu, G., and Yin, J. (2020). Estimating the inbreeding level and genetic relatedness in an isolated population of critically endangered Sichuan taimen (Hucho bleekeri) using genome-wide SNP markers. Ecol. Evol. 10, 1390–1400. doi: 10.1002/ece3.5994
Zimmerman, S. J., Aldridge, C. L., and Oyler-McCance, S. J. (2020). An empirical comparison of population genetic analyses using microsatellite and SNP data for a species of conservation concern. BMC Genomics 21:382. doi: 10.1186/s12864-020-06783-9
Zimmermann, B. L., Crivellaro, M. S., Hauschild, C. B., Bartholomei-Santos, M. L., Crandall, K. A., Pérez-Losada, M., et al. (2018). Phylogeographic reveals unexpected low genetic diversity in a widely distributed species: the case of the freshwater crab Aegla platensis (Decapoda: Anomura). Biol. J. Linn. Soc. 123, 578–592. doi: 10.1093/biolinnean/blx166
Keywords: demographic history, Fabaceae, Mimosa catharinensis , conservation genetics, genetic diversity
Citation: Teixeira TM and Nazareno AG (2021) One Step Away From Extinction: A Population Genomic Analysis of A Narrow Endemic, Tropical Plant Species. Front. Plant Sci. 12:730258. doi: 10.3389/fpls.2021.730258
Edited by:
Nina Rønsted, National Tropical Botanical Garden, United StatesReviewed by:
Mária Šurinová, Academy of Sciences of the Czech Republic (ASCR), CzechiaJeremie Fant, Chicago Botanic Garden, United States
Copyright © 2021 Teixeira and Nazareno. This is an open-access article distributed under the terms of the Creative Commons Attribution License (CC BY). The use, distribution or reproduction in other forums is permitted, provided the original author(s) and the copyright owner(s) are credited and that the original publication in this journal is cited, in accordance with accepted academic practice. No use, distribution or reproduction is permitted which does not comply with these terms.
*Correspondence: Alison G. Nazareno, YWxpc29uZ25AdWZtZy5icg==; bmF6YXJlbm9AdW1pY2guZWR1