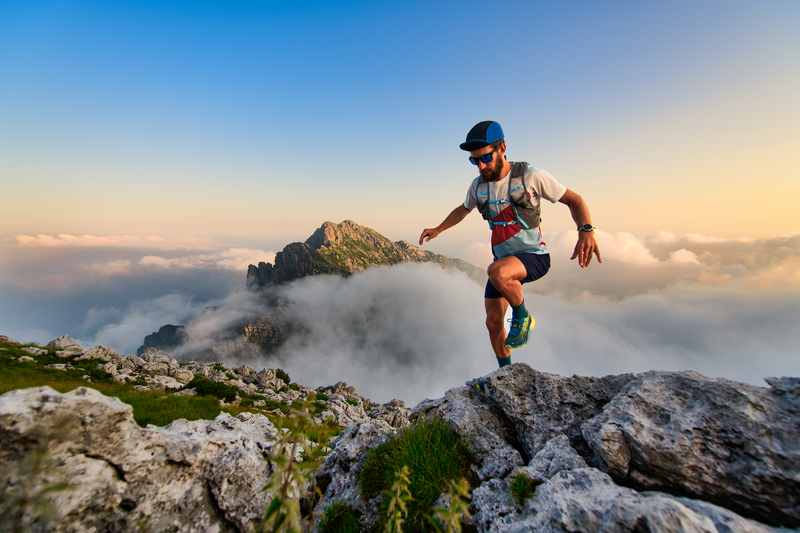
95% of researchers rate our articles as excellent or good
Learn more about the work of our research integrity team to safeguard the quality of each article we publish.
Find out more
ORIGINAL RESEARCH article
Front. Plant Sci. , 30 August 2021
Sec. Plant Nutrition
Volume 12 - 2021 | https://doi.org/10.3389/fpls.2021.723862
The limited availability of phosphorus (P) in soils causes a major constraint in the productivity of potatoes, which requires increased knowledge of plant adaptation responses in this condition. In this study, six potato cultivars, namely, Agria, Lady Claire, Milva, Lilly, Sieglinde, and Verdi, were assessed for their responses on plant growth, leaf physiology, P use efficiency (PUE), and tuber quality with three P levels (Plow, Pmed, and Phigh). The results reveal a significant variation in the cultivars in response to different P availabilities. P-efficient cultivars, Agria, Milva, and Lilly, possessed substantial plant biomass, tuber yield, and high P uptake efficiency (PUpE) under low P supply conditions. The P-inefficient cultivars, Lady Claire, Sieglinde, and Verdi, could not produce tubers under P deprivation conditions, as well as the ability to efficiently uptake P under low-level conditions, but they were efficient in P uptake under high soil P conditions. Improved PUpE is important for plant tolerance with limited P availability, which results in the efficient use of the applied P. At the leaf level, increased accumulations of nitrate, sulfate, sucrose, and proline are necessary for a plant to acclimate to P deficiency-induced stress and to mobilize leaf inorganic phosphate to increase internal PUE and photosynthesis. The reduction in plant biomass and tuber yield under P-deficient conditions could be caused by reduced CO2 assimilation. Furthermore, P deficiency significantly reduced tuber yield, dry matter, and starch concentration in Agria, Milva, and Lilly. However, contents of tuber protein, sugars, and minerals, as well as antioxidant capacity, were enhanced under these conditions in these cultivars. These results highlight the important traits contributing to potato plant tolerance under P-deficient conditions and indicate an opportunity to improve the P efficiency and tuber quality of potatoes under deficient conditions using more efficient cultivars. Future research to evaluate molecular mechanisms related to P and sucrose translocation, and minimize tuber yield reduction under limited P availability conditions is necessary.
Potatoes demand high phosphorus (P) availability in soils because their roots are relatively inefficient in P uptake with low soil P concentration (Sandaña, 2016; Wacker-Fester et al., 2019). P fertilizers have been applied to increase soil P and tuber yield. However, up to 80% of the applied P turns into insoluble complex and organically bound forms, which are not readily available for plants (Shi et al., 2019). The unavailable P tends to remain in the upper soil layers and tends to flow toward surface water, which causes eutrophication (King et al., 2015). Considering environmental concerns along with a decrease in globally reserved P resources (Cordell and White, 2014), improved agronomic practices that reduce P fertilizer input are necessary to enhance P efficiency for the production of potatoes.
For potatoes, P use efficiency (PUE) can be defined as the capability of the plants to produce biomass or tuber yield per unit of applied P (Veneklaas et al., 2012). Under low P availability conditions, PUE is strongly influenced by P uptake efficiency (PUpE), the amount of total plant P uptake per unit of applied P (Sandaña, 2016). Plants respond to limited P availability through morphological and physiological adjustments to acclimate to deficient-P conditions. The previous study reported increased root biomass compared with the shoots of potato (cv. Milva) under P-deficient conditions, which indicates preferential photoassimilate allocation to roots to enhance P uptake (Chea et al., 2021). However, root sugar concentrations were reduced by 50–80% under these conditions. Based on these results, additional investigation on leaf biochemical alterations under P-deficient conditions is required to explain the shortage in photoassimilates for translocation. Owing to the indispensable role of P in energy transfer, marginal P deficiency could reduce adenosine triphosphate (ATP) synthesis for consumption in the Calvin cycle, causing a reduction in total photosynthetic rates (Carstensen et al., 2018; Dixon et al., 2020). Electron transport and ATP synthesis mediation in the Calvin cycle is important to increase photosynthesis (Simkin et al., 2019). Furthermore, a large amount of inorganic P (Pi) in leaf tissues has to be generated through internal P recycling to maintain its concentration in the stroma for carbon fixation (Wissuwa et al., 2005). Therefore, efficient use of plant internal P for photosynthesis is essential to ensure sufficient photoassimilates for shoot growth and translocation. However, Wissuwa et al. (2005) and Fredeen et al. (1989) showed an accumulation of soluble sugars under P-deficient conditions, which suggested that the utilization of photoassimilates for plant growth was restricted. In maize, Plénet et al. (2000) reported a direct effect of P deficiency on growth rather than on leaf photosynthesis. Little is known about potatoes with respect to photosynthesis and leaf biochemical alteration induced by P deficiency. In addition, the stress caused by P deficiency triggers the accumulation of osmolytes, such as proline, for stress detoxification (Hayat et al., 2012) and greater uptake of essential minerals, such as nitrogen (N) and sulfur (S) (Chea et al., 2021). These adaptation mechanisms are necessary for plant viability under P-deficient conditions. Therefore, potato cultivars with enhanced P use efficiency and photosynthetic capacity, under limited P availability conditions, could be an alternative strategy to overcome situations of P deficiency.
P availability in soils highly influences potato tuber yield and quality (Naumann et al., 2020). However, besides the above-mentioned morphological and biochemical responses, the impact of limited P availability on potato tuber quality is less documented, except for a few reports on tuber yield, dry matter, starch, protein, and sugars (Öztürk et al., 2010; Fernandes et al., 2015; Leonel et al., 2017). Wang and Frei (2011) showed an increased concentration of micronutrients, protein, and antioxidant capacity in potato tubers and grains of various crops as a result of nutrient uptake alteration and modulation of key enzymes under abiotic stress conditions. Moreover, the recent discovery showed an increase in leaf minerals and antioxidant compounds, such as total flavonoids and total phenolics, in response to P deficiency (Chea et al., 2021); thus, we can hypothesize that P deficiency may also stimulate the uptake of minerals and the antioxidant capacity in tubers.
In this study, we sought to assess (I) the impact of P deficiency on plant growth, PUE, photosynthetic characteristics, and leaf biochemical properties, and (II) the effects of P deficiency on the quality of potato tubers. The goals of this study are to identify P-efficient cultivars and to provide further insights into the tolerant mechanisms of potatoes under P deficiency conditions. We also provided the first report on the implications of P deficiency on ascorbic acid and the antioxidant capacity of potato tubers.
Six potato cultivars were used, which consisted of four table potatoes, Agria, Milva, Lilly, and Sieglinde, and two processing potatoes, Lady Claire and Verdi. Lady Claire was obtained from Meijer Potato (Rilland, the Netherlands); Agria, Lilly, and Sieglinde were obtained from Kartoffel Mueller (Nersingen, Germany); Milva and Verdi were obtained from Europlant Pflanzenzucht GmbH (Lüneburg, Germany). These cultivars were selected based on their popularity for production in the respective regions, and their differences in morphological and yield characteristics. Additionally, Sieglinde is known for its limited biomass and tuber yield production, and it is a cultivar sensitive to nutrient deficiency (Mauromicale et al., 2006). The description of each cultivar is shown in Supplementary Table 1.
The experiment was performed under outdoor conditions using six cultivars and three P levels (0.02, 0.2, and 1.2 g kg−1 soil; designated as Plow, Pmed, and Phigh, respectively) with four replications. The average temperature during this period (June–September 2019) was 19 ± 6.5°C. Diurnal photosynthetic active radiation, temperature, relative humidity, and daily precipitation are shown in Supplementary Figure 1. The sandy soil used in the experiment had a pH of 4.8 and extractable calcium acetate lactate P (CAL-P) of 0.06 g kg−1 soil. To lower P concentration, the soil was mixed with equal amounts of medium-sized quartz sand, so that all the treatments had the same initial soil P. Afterward, the different P treatments were induced by applying Ca(H2PO4)2 basally as powder. The application of other nutrients is shown in Supplementary Table 2. Soil Ca concentration was balanced by the addition of CaCO3. After all the nutrients were applied, soil pH was in the range of 5.5–7. Then, the soil mixture, with a bulk density of 1.1 kg dm−3, was filled in 6 L pots (Mitscherlich, STOMA, Emmingen-Liptingen, Germany). Afterward, a single germ bud of 1–2 cm was taken from each potato seed using a ball shaper and was planted in each pot at a depth of 5 cm. These procedures were adapted from Koch et al. (2019) and Wacker-Fester et al. (2019). All the seedlings were germinated within 10 days after they were planted. Water was supplied regularly to maintain optimum soil moisture based on visual observation.
Plant height development was monitored during the experiment. Leaf gas exchange measurements were conducted on the terminal leaf of a fully developed leaflet at the fourth position from the top using a portable photosynthesis system (LI-6800, LI-COR Biosciences, Lincoln, NE, United States) after 35, 53, and 70 days after emergence (DAE). Prior to the measurements, each plant was adapted for 1 h under constant environmental conditions (temperature = 20°C, relative humidity = 60%, and photosynthetic photon flux density [PPFD] = 400 μmol m−2 s−1 at plant level) in a climate chamber. For the measurement, the CO2 concentration, relative humidity, and leaf temperature inside the cuvette (4 cm2) were adjusted to 400 ppm, 50%, and 25°C, respectively. Light response curves were generated by starting with a PPFD of 1,400 μmol m−2 s−1 in 13 steps (180 s/step) to zero. Based on light response curve measurements, the CO2 assimilation rate, stomatal conductance, and intercellular CO2 concentration measured at PPFD of 400 μmol m−2 s−1 were compared for different cultivars and P treatments at different stages of development.
The whole leaflet at the fourth position from the top was taken 53 DAE after leaf gas exchange measurements for mineral and biochemical analyses. A part of the sample was freshly ground with liquid nitrogen and stored at −20°C. The plants were mature, and the tubers were fully developed at 87 DAE; therefore, the whole plants were harvested and partitioned into shoots, roots, and tubers. Another part of the fresh tuber was used for ascorbic acid determination, immediately after cutting, as described below. The sub-samples of each plant part were freeze-dried in a freeze dryer (EPSILON 2-40, Christ, Osterode am Harz, Germany) for 4 days. The biomass of the plant was then calculated as a combination of total shoots (including sampled young leaves 53 DAE) and root dry matter (DM). Dry samples were ground in a hammer mill (DFH 48 Culatti, Kinematica, Malters, Switzerland) with a 0.5 mm sieve.
Minerals in the leaves, shoots, roots, and tubers were analyzed by extracting 100 mg of the freeze-dried sample according to Koch et al. (2019) to determine the concentration of P, S, potassium (K), calcium (Ca), magnesium (Mg), manganese (Mn), iron (Fe), and zinc (Zn). These mineral concentrations were determined by inductively coupled plasma optical emission spectrometry (ICP-OES) (Varian, Palo Alto, CA, United States). Shoot, root, and tuber P contents were calculated by multiplying the P concentration of the respective plant part with its DM content. Total P uptake of each plant was calculated as the combination of shoot, root, and tuber P contents. Afterward, P uptake efficiency (PUpE) and P use efficiency (PUE) was calculated based on Sandaña (2016) as follows:
The applied P of each treatment was determined by multiplication of the applied P concentration with the soil dry weight per pot. Carbon (C) and N concentration in the leaves and tubers were analyzed from 0.75 g of the freeze-dried samples using the dry combustion method with a Vario EL analyzer (Elementar, Langenselbold, Germany). Phosphate (), nitrate (), and sulfate () in the leaves and tubers were determined by extracting 20 mg of the freeze-dried samples with 1 ml of 0.1 M HCl, and the extracts were analyzed with an ion chromatography system (ECO IC, Metrohm, Herisau, Switzerland) in accordance with the procedures described by Koch et al. (2020). The concentration of each ion was expressed as mg of the respective mineral per unit of sample DM. After the harvest, available P in the soil was extracted following the Olsen method using a bicarbonate solution (Hartmann et al., 2019), and was determined in accordance with molybdenum blue procedures (Murphy and Riley, 1962) with a UV-Vis spectrophotometer (HP 8453, Hewlett Packard, Böblingen, Germany) at 882 nm absorbance.
Leaf chlorophyll and free proline were extracted by homogenizing 20 mg of freshly ground samples with 250 μl of 80% ethanol at 95°C. The mixture was then centrifuged at 10,600 g for 10 min to collect the supernatant. The procedures were sequentially repeated twice with 150 μl of 80% ethanol and 150 μl of 50% ethanol. The supernatants from each step were pooled and measured for leaf chlorophyll and free proline concentration according to Koch et al. (2019) and Chea et al. (2021), respectively. For ATP and protein measurements, 100 mg of the fresh leaf samples were extracted with 1 ml of cold 5% trichloroacetic acid for 5 min, and the mixture was centrifuged for 10 min at 13,000 g at 4°C to collect the supernatant. The ATP concentration in the supernatant was determined using an ATPlite assay kit (PerkinElmer, Waltham, MA, United States) in accordance with the instructions of the manufacturer. The pellet from the sample extract was resuspended with 400 μl of 0.1 M NaOH for 30 min at 95°C. After centrifugation for 10 min at 10,000 g, protein concentration was determined using a Bradford protein kit (Merck, Darmstadt, Germany) based on the modified methods of Zor and Selinger (1996). Bovine serum albumin was used as the standard.
Soluble sugars in the leaves were extracted by homogenizing 50 mg of the freeze-dried samples with 700 μl of 80% acetonitrile in a shaker at 420 rpm for 3 h. Then, 50 μl of 3.6% K4[Fe(CN)6]*3H2O and 50 μl of 7.2% 7H2O were subsequently added to precipitate proteins, followed by 30 min of centrifugation at 15,000 g to collect the supernatant, and it was stored at −20°C. For the tubers, 0.75 g of each freeze-dried sample was extracted with 3 ml of distilled water by shaking for 1 h at 420 rpm. Protein precipitation was executed by sequentially adding 0.5 mL of 3.6% K4[Fe(CN)6]*3H2O and 0.5 ml of 7.2% 7H2O in the mixture, which was then subjected to 20 min of centrifugation at 2,600 g to collect the supernatant. The extraction procedures were repeated two times without protein precipitation. The supernatants from each step were pooled and filled up to 10 ml with distilled water and were stored at −20°C. For measurement, the extract was thawed and centrifuged for 30 min at 15,000 g. The supernatant was then filtered through a 0.45-μm membrane with the help of a 13 mm syringe (VWR, Darmstadt, Germany). Finally, 20 μl of the filtered extract was used for the quantification of soluble sugars (sucrose, glucose, and fructose) by high-performance liquid chromatography (Jasco, Pfungstadt, Germany). As eluent, 80% acetonitrile was used through a 5 μm column (LiChrospher 100 NH2, Merck, Darmstadt, Germany) at 22°C and with a flow rate of 1 ml min−1.
The starch concentration of the tubers was determined by a polarimetric method according to the procedures of Koch et al. (2019). Tuber N concentration was converted to crude protein concentration with a factor of 6.25 (AOAC, 2005). The ascorbic acid content of the potato tubers was analyzed based on a 2,6-dichlorophenolindophenol (DIP) titrimetric method described in Sonntag et al. (2020).
To determine total phenolics (TPC), total flavonoids (TFC), and antioxidant capacity, 100 mg of the freeze-dried tuber sample was extracted two times with 1 ml of 99.9% methanol. The supernatants were combined and filled up to 2 ml with methanol. TPC and TFC of the extract were analyzed according to Chea et al. (2021). Antioxidant capacity was determined based on 2,2-diphenyl-1-picrylhydrazyl (DPPH) assay, and Trolox equivalent antioxidant capacity (TEAC) assay based on Kaur et al. (2013), with slight modifications. For the DPPH assay, 20 μl of the extract was suspended with 180 μl of 0.2 mM DPPH. After incubation for 30 min in the dark, the mixture was read with a plate reader (Synergy HTX, Biotek, Winooski, VT, United States) at 515 nm absorbance. The TEAC assay is based on the ability of antioxidants to scavenge 2,2′-azino-bis-3-ethylbenzothiazoline-6-sulphonic acid (ABTS) radical cations. For measurement, 10 μl of the extract was mixed with 150 μl of the ABTS working solution, containing 0.15 mM of ABTS and 0.5 mM of K2S2O8. The mixture was incubated at room temperature for 10 min in darkness, and the absorbance was read with a plate reader at 734 nm. In both the assays, 99.9% of methanol was used as a negative control, and radical scavenging capacity was determined based on the difference in the negative control and sample extract absorbance. The antioxidant capacity was calculated against the Trolox standard calibration curve and expressed as μmol Trolox equivalent (TE) g−1 DM.
Data of plant growth, mineral concentration, P efficiency, leaf biochemical properties, and tuber quality parameters were subjected to two-way ANOVA. Tukey's honestly significant difference (HSD) test at p < 0.05 was performed for pairwise comparisons among the P treatments when there were significant differences in ANOVA. The association among the observed traits was assessed by Pearson's correlation. These analyses were conducted following the methods of Gomez and Gomez (1984) using the Statistix 8.0 software (Analytical Software, Tallahassee, United States). Graphical presentations were prepared in Sigmaplot 12.5 (Systat Software, San Jose, CA, United States).
The P applications had a significant effect on plant height, plant biomass, and tuber yield for all the cultivars (Figure 1). The effects of the cultivars and the interaction among the cultivars and P levels were also significant (Supplementary Table 3). The plant height of each cultivar evolved at different rates during the growing period depending on the P application (Figures 1A,B). The plant biomass of Lady Claire, Lilly, Sieglinde, and Verdi was strongly inhibited with the Plow treatment (0.40–1.13 g plant−1), and it increased by 24- to 85-fold under higher P availability conditions. Agria and Milva had relatively greater plant biomass (6.16 and 9.70 g plant−1, respectively) than the other cultivars under Plow conditions, which also increased in response to higher P levels (Figure 1C). However, under Plow conditions, Lady Claire, Sieglinde, and Verdi were not able to produce tubers, while the tuber yield of Agria, Lilly, and Milva ranged from 45.90 to 79.42 g plant−1 (Figure 1D). The variations in mean values of plant height, plant biomass, and tuber yield among the cultivars and P treatments are shown in Supplementary Table 4.
Figure 1. (A) Shoot phenotype 40 days after emergence (DAE), (B) plant height development, (C) plant biomass, and (D) tuber yield of potato cultivars with the application of Plow, Pmed, and Phigh. Letters in lowercase indicate a significant difference between P treatments of each cultivar by Tukey's honestly significant difference (HSD) test at p < 0.05. Error bar represents SE of means (n = 4). FW, fresh weight.
Mineral analyses of leaves 53 DAE revealed low P concentrations under Plow conditions, which ranged from 0.82 (Sieglinde) to 1.19 mg g−1 (Agria) (Figure 2A; Supplementary Table 4). The application of Pmed and Phigh increased leaf P by 50–83% and 3- to 5-fold, respectively, compared with Plow. We also observed a reduction of leaf phosphate under Plow (Figure 2B), but it was at a lower magnitude than that of leaf P. The Plow treatment reduced shoot P concentrations, but there was no significant difference between Plow and Pmed in root and tuber P concentration (Figures 2C–E). Low P availability in the soil suppressed total P uptake, but it enhanced the PUpE of Agria and Milva by 44% (Figures 2F,G). In contrast, the PUpE of Lady Claire, Sieglinde, and Verdi was hampered by low soil P. Furthermore, PUE remained highest for Agria among the cultivars under Plow conditions (Figure 2H). We were unable to determine the PUE of Lady Claire, Sieglinde, and Verdi under Plow conditions due to the absence of tubers; thus, we assumed a very low PUE for these cultivars under low P availability conditions. The analysis of soil P after harvest, for which the Olsen extraction method was used, revealed that soil P concentration under Phigh conditions was 14–16 times and 7–9 times higher than that under Plow and Pmed conditions, respectively (Figure 2I).
Figure 2. (A–E) Phosphorus (P) concentration in different plant parts, (F) total P uptake, (G) P uptake efficiency, (H) P use efficiency, and (I) residual soil P concentration of potato cultivars with the application of Plow, Pmed, and Phigh. P uptake efficiency, amount of total P uptake per unit of applied P, and P use efficiency, tuber dry matter production per unit of P uptake. Phosphate concentration (B) is expressed as mg of mineral (P) per unit of DM. Different letters in lowercase indicate a significant difference between P treatments of each cultivar by Tukey's HSD test at p < 0.05. Error bar represents SE of means (n = 4). DM, dry matter; n.d., not determined because of no tuber production.
Furthermore, the Pearson's correlations of traits associated with plant P concentration and PUE show positive correlations among leaf P, shoot P, plant biomass, total P uptake, and applied P levels. However, these parameters negatively correlated with PUE, which was positively associated with PUpE (Figure 3).
Figure 3. Correlation among plant P concentration, biomass, total P uptake, P uptake efficiency (PUpE), P use efficiency (PUE), and P applications (applied P). Color gradients represent Pearson's correlation coefficient, and values in bold of each cell indicate p-values which are significant at < 0.05.
In response to the Plow treatment, leaf essential minerals and ions such as N, nitrate, S, and sulfate significantly increased compared with higher P availability (Table 1). Furthermore, there were significant correlations between leaf N and S with their respective ionic forms (Supplementary Figure 2). The impact of P deficiency on other leaf macro and micronutrients is shown in Supplementary Table 5.
Table 1. Leaf concentration (mg g−1 dry matter, DM) of nitrogen (N), nitrate, sulfur (S), and sulfate of potato cultivars with Plow, Pmed, and Phigh.
CO2 assimilation rate and stomatal conductance were significantly affected by the cultivars and P levels on all measurement dates (Figures 4A,B; Supplementary Table 3). In general, both parameters were reduced by 13–90% on all measurement dates under Plow conditions compared with Pmed. Across the measurement dates, the CO2 assimilation and stomatal conductance of Agria and Milva were relatively higher than those of the other cultivars under Plow conditions. We observed an increasing trend in the CO2 assimilation and stomatal conductance of Lady Claire, Sieglinde, and Verdi under Phigh conditions compared with Pmed, but they were either stable or reduced by 16–45% for Agria, Lilly, and Milva. The influence of P applications on intercellular CO2 concentration was to a variable extent in regard to cultivars and measurement dates (Figure 4C). For Lady Claire, Lilly, Sieglinde, and Verdi, the intercellular CO2 concentration was enhanced under Plow conditions on at least one of the measurement dates.
Figure 4. (A) CO2 assimilation, (B) stomatal conductance, and (C) intercellular CO2 concentration of potato cultivars with the application of Plow, Pmed, and Phigh. Different letters in lowercase indicate a significant difference between P treatments of each cultivar by Tukey's HSD test at p < 0.05. Error bar represents SE of means (n = 2–3); n.d., not determined because of too small leaf area to fit in the cuvette.
Furthermore, the analyses of leaves sampled 53 DAE show that leaf ATP ranged from 3.02 (Milva) to 22.87 nmol g−1 (Sieglinde) under Plow conditions and that it was enhanced by 2- to 12-fold under Phigh conditions (Figure 5A; Supplementary Table 6). Leaf protein and chlorophyll concentrations of each cultivar were less affected by P applications (Figures 5B,C). However, leaf proline under Plow conditions ranged from 5.10 (Agria) to 9.80 μmol g−1 (Sieglinde). At a higher P application (Phigh), leaf proline was reduced by 14–63% (Figure 5D; Supplementary Table 6). Even though P availability in the soil had less influence on leaf sucrose in all the cultivars, except for Lady Claire and Milva, reducing sugars (fructose and glucose) were significantly increased in response to higher P supply (Figures 5E,F). In addition, total soluble sugars positively correlated with ATP concentration and CO2 assimilation, although the correlation coefficient between the total soluble sugars and CO2 assimilation was moderate (Figure 6).
Figure 5. (A–F) Leaf biochemical characteristics of potato cultivars 53 DAE with Plow, Pmed, and Phigh. Different letters in lowercase indicate a significant difference between P treatments of each cultivar by Tukey's HSD test at p < 0.05. Error bar represents SE of means (n = 4). FW, fresh weight; DM, dry matter.
Figure 6. Correlation among leaf soluble sugars with (A) adenosine triphospate (ATP) concentration and (B) CO2 assimilation rate measured 53 DAE. FW, fresh weight; DM, dry matter; * and *** indicate significant correlation at p < 0.05 and p < 0.001, respectively.
Since Lady Claire, Sieglinde, and Verdi were unable to produce tubers under Plow conditions, the results are demonstrated only for Agria, Lilly, and Milva to assess the impact of Plow on tuber quality characteristics. The significant effects of the cultivars, P levels, and their interactions were observed in many quality parameters (Supplementary Table 7). Figure 7 shows that Plow reduced tuber DM by 22–32%, starch concentration by 14–23%, and ascorbic acid by 10–25% compared with both Pmed and Phigh. However, tuber protein was increased under Plow conditions by 27–64% and 71–85% compared with Pmed and Phigh, respectively. Tuber soluble sugars of Agria and Milva under Plow conditions were higher than those under higher P levels; however, the soluble sugars of Lilly were not significantly different between the P treatments. There was no significant difference in TPC and TFC among the P applications of Agria, but increasing the P application resulted in decreasing TPC and TFC for Lilly and Milva. Furthermore, the antioxidant capacity (DPPH and TEAC) of Plow was 11–57% higher than that of Pmed and Phigh. Besides these tuber quality characteristics, the P applications also affected mineral and ion concentrations to variable extents (Table 2). Although Plow reduced tuber C and phosphate concentration by 2–40%, it increased the concentrations of the other minerals and ions (K, Ca, Mg, S, Cu, Fe, Mn, Zn, nitrate and sulfate by 20–85% compared with Phigh.
Figure 7. (A–I) Tuber quality characteristics of potato cultivars with the application of Plow, Pmed, and Phigh. Different letters in lowercase indicate a significant difference between P treatments of each cultivar by Tukey's HSD test at p < 0.05. DM, dry matter; FW, fresh weight; TPC, total phenolic concentration; TFC, total flavonoid concentration; DPPH, antioxidant capacity by 2,2-diphenyl-1-picrylhydrazyl assay; TEAC, Trolox equivalent antioxidant capacity.
A pot experiment was conducted to elucidate the responses of the six potato cultivars to soil P levels (Plow, Pmed, and Phigh). Under pot conditions, the availability of applied P for the plants is less interfered with by P immobilization and buffering capacity of the soils, which usually occur under field conditions. The results provide a further understanding of the impacts of P deficiency and the tolerance mechanisms of potato cultivars, from plant to leaf levels.
The highest plant height, plant biomass, and tuber yield were seen under Phigh conditions, which indicates that in the experiments there was sufficient P in soils with P application at 1.2 g kg−1. All the cultivars exhibited deficiency under Plow conditions through stagnated height development, reduced plant biomass, and less or no tuber yield formation (Figure 1). The reduction in plant biomass under P-deficient conditions was at a magnitude greater than that reported in pot studies by Lee et al. (2013) and Wacker-Fester et al. (2019), which suggested that P-deficient conditions in this pot study were severe. The variation in plant biomass and tuber yield of potato cultivars was also documented (Lee et al., 2013; Soratto et al., 2015; Wacker-Fester et al., 2019), which allowed us to identify P-efficient cultivars. In potatoes, tuber yield is important for classifying the P responsiveness of cultivars (Soratto et al., 2015); thus, it is an excellent parameter that indicates the tolerance of cultivars to P deficiency. In this study, the P-efficient cultivars, Agria, Milva, and Lilly, produced substantial tuber yield, but the P-inefficient cultivars, Lady Claire, Sieglinde, and Verdi, were unable to produce tubers under P-deficient conditions. Furthermore, Lady Claire, Sieglinde, and Verdi maintained substantial tissue P concentration and plant biomass, and they had relatively high PUpE and PUE under P-deficient conditions (Figures 1, 2A–H). The ranges of PUpE and PUE were similar to those in Sandaña (2016) under field conditions. In contrast, although Lady Claire, Sieglinde, and Verdi had similar plant P concentrations compared with the other cultivars, they were characterized by very low plant biomass, low PUpE, and low PUE under P-deficient conditions. This indicates that these cultivars conserved the sparingly available P in the plant for viability, and they might lack traits associated with enhanced P uptake and allocation for growth and tuber formation. The separation of cultivars for their efficiency under limited P availability conditions is also confirmed based on the principal component analysis of traits associated with P efficiency, as shown in Supplementary Figure 3. Although residual P availability in soils under P-deficient conditions was similar among the cultivars, the enhanced P uptake of P-efficient cultivars may have been caused by improved root traits, which could modify the amount of P available for plants (Lee et al., 2013). Therefore, improved PUpE is important to enhance PUE under limited P supply conditions (Wang et al., 2010). The increased PUpE and PUE of Lady Claire, Sieglinde, and Verdi under high P availability conditions suggests that these cultivars are efficient in P uptake when soil P availability is not a limiting factor. Increased P availability in soil resulted in high plant P concentration, P uptake, and plant biomass (Figure 3), but it cannot give assurance for enhanced PUE if the PUpE is low. Consequently, high residual soil P, after harvest, implies inefficient use of P fertilizer, which, under field conditions, is a potential risk for the environment (Heuer et al., 2017).
In plants, P exists as inorganic orthophosphate forms ( or Pi) and organic phosphate esters (Veneklaas et al., 2012). The results reveal a lesser reduction in leaf Pi compared with leaf P under P-deficient conditions, which could be caused by an increase in a Pi fraction under these conditions. Under P-deficient conditions, plants sense cytosolic Pi shortage through recycling the entire P in the vacuole, to increase Pi concentration for efflux into cytosol and chloroplast (Shen et al., 2011; Long et al., 2019). Therefore, P translocation to active photosynthetic tissue and internal P recycling could be adaptations of potato cultivars under stress conditions induced by P deprivation. Furthermore, leaf nitrate also increased under P-deficient conditions. The mechanisms underlying nitrate accumulation have not been thoroughly understood; however, they could be caused by nitric oxide generation due to oxidative stress acclimation under P-deficient conditions (Fu et al., 2018). High nitric oxide production was also reported on soybean leaves (Ramos-Artuso et al., 2019) and Arabidopsis (Royo et al., 2015) under P deprivation conditions. In the complex nitrate cycle in plants, nitrate is required for nitric oxide biosynthesis, and the turnover of nitric oxide also produces nitrate. This complete cycle is regulated by nitrate reductase (Astier et al., 2018). Furthermore, nitrate assimilation is an energetically costly process (Nunes-Nesi et al., 2010), which could be inhibited under P-deficient conditions, resulting in an accumulation of nitrate in leaves. Additionally, the uptake and allocation of other N forms such as ammonium and amides may be altered under P-deficient conditions, as implied by the relationship between leaf N and nitrate shown in Supplementary Figure 2. Furthermore, leaf sulfate concentration may also increase to fulfill the S demand of sulfolipid generation for replacing phospholipid under P-deficient conditions and to balance leaf anion-to-cation ratio under low phosphate ion conditions (Misson et al., 2005; Rouached, 2011).
Besides the alterations in leaf minerals and ions, P deficiency reduced stomatal conductance and CO2 assimilation rate at different magnitudes (Figures 4A,B). Reduction in photosynthesis under P-deficient conditions was also observed in barley (Carstensen et al., 2018) and soybean (Singh and Reddy, 2015). P deficiency suppresses photosynthesis rate through disruption on electron transport and reduction in ATP and NADPH synthesis (Carstensen et al., 2018). In this study, although fast photosynthesis measurements were conducted at 180 s at each radiation level, it was sufficient to obtain steady-state results, because the PPFD inside the cuvette for the data used in this study and under ambient conditions, before the measurements, was the same. The results further reveal that at least two of the three P-efficient cultivars (viz. Agria and Milva) had relatively high CO2 assimilation rates and stomatal conductance, especially 35 and 70 DAE, which explained the substantial shoot biomass production and tuber yield of these cultivars under P starvation conditions. The minimal disruption in the photosynthesis of these cultivars under P-deficient conditions could be caused by improved P allocation to leaves to increase the leaf area. In soybean, Chaudhary et al. (2008) also showed the importance of P allocation to shoots to increase PUE and leaf area under P deprivation conditions. However, the reduction of CO2 assimilation in P-efficient cultivars under high P supply conditions might be linked to their high leaf area (Figure 1A). Leaves of the plants expand in response to high P supply, causing high leaf area (Shi et al., 2019), and eventually, become thinner. Therefore, photosynthetic machinery per unit of leaf area may be reduced. However, increased leaf area under high P supply conditions could compensate for the reduction in photosynthesis because of a greater light interception.
Although leaf photosynthesis was reduced by P deficiency, leaf protein and chlorophyll were less affected (Figures 5B,C). In leaves, the majority of N is present in chlorophyll and proteins of the thylakoids (Perchlik and Tegeder, 2018); therefore, in this study, the accumulation of leaf N may contribute to the maintenance of protein and chlorophyll in leaves under P-deficient conditions. A similar observation in maize indicated that leaves of P-deficient plants are less associated with a reduction in chlorophyll concentration because these leaves eventually become thicker and appear to be bluish-green (Plénet et al., 2000). However, the results reveal that P deficiency also modulates leaf ATP, sucrose, reducing sugars, and proline at different levels depending on the cultivar. Since the P applications did not significantly affect leaf protein concentration (Figures 5B,C), the effects of P levels on ATP and proline could be compared and discussed based on per leaf fresh weight. At the leaf level, P plays an essential role as a substrate for ATP synthesis in the chloroplast (Carstensen et al., 2018). Even though a chloroplast P transporter (AtPHT4;1) is proposed to mediate chloroplast Pi for ATP synthesis activities under limited P supply conditions in Arabidopsis (Karlsson et al., 2015), in this study, sufficient chloroplast Pi may have not been maintained under severe P-deficient conditions, which ultimately results in ATP reduction. ATP limitation under P-deficient conditions hampers the use of NADPH in the Calvin cycle (Carstensen et al., 2018); thus, CO2 assimilation is reduced for sugar production.
In this study, P deficiency also influenced sugar metabolism. There was no significant reduction in leaf sucrose concentration in many of the cultivars under P-deficient conditions (Figure 5E). This could be due to a high cleavage of sucrose to fructose and glucose under high P availability conditions, but under P-deficient conditions, the conversion of sucrose was inhibited. This resulted in a huge increase of these reducing sugars in response to increasing P applications, especially for Agria, Lilly, and Milva (Figure 5F). The conversion of sucrose to reducing sugars is regulated by enzymes such as invertase, sucrose phosphate synthase, and fructose 1,6-bisphosphatase, which are enhanced under sufficient P supply conditions (García-Caparrós et al., 2021). The less or no reduction of sucrose in the leaves under P-deficient conditions could also be caused by either limited sugar transport or the sugar conservation response of plants. Under P-deficient conditions, plants maintain large amounts of compatible non-toxic solutes such as sucrose and proline for cellular osmotic adjustment, stabilizing cell structure, and scavenging free radicals (Ashraf and Foolad, 2007; Hayat et al., 2012). In Arabidopsis, a high sucrose level is important to induce P starvation-responsive genes for mobilizing plant internal P (Lei et al., 2011). Under stress conditions induced by P deficiency, proline is synthesized from glutamate in the cytosol; however, depending on the recovery from stress, proline is rapidly oxidized into glutamate, while ATP is also generated during this oxidation process to maintain leaf viability (Launay et al., 2019). Among the cultivars, we found that Sieglinde had a relatively high ATP, proline, sucrose, and total reducing sugar (fructose and glucose) concentration compared with the other cultivars under P-deficient conditions. These results indicate a high stress intensity of this cultivar under P-deficient conditions, which may have inhibited sugar translocation. It also recommends further investigations on sucrose transporters that may explain molecular mechanisms underlying the relatively high sucrose of these cultivars under low P availability conditions. In this study, there was a positive correlation between the concentration of leaf total soluble sugars and the concentration of ATP (Figure 6A). The correlation between total soluble sugars and CO2 assimilation was also positively significant (Figure 6B), but it was to a lesser extent, which suggested that improved CO2 assimilation is important for sugar production, provided that there is a sufficient amount of ATP in the reaction to convert the assimilated CO2 into sugars.
We present the implications of P deficiency on the tuber quality of potato in addition to plant agronomic and biochemical characteristics as outlined above. P is involved in several key enzymes that regulate the starch synthesis, and it is also a key element of starch composition (Nielsen et al., 1994; Naumann et al., 2020). Although we did not observe a significant difference in tuber P concentration between Plow and Pmed, a reduction of P application resulted in a significant decrease in tuber DM and starch concentration (Figures 7A,B). This suggests a compromise between improving PUE and the quality parameters. The tuber DM of all the cultivars under P-deficient conditions was also below the range (16–18%) of many potatoes available in the market (Storey, 2007), which may have a significant impact on the market acceptability. However, protein concentration was enhanced under low P availability conditions (Figure 7C), which could be caused by increased N uptake as indicated by high leaf N concentration (Table 1). These results are similar to those reported by Leonel et al. (2017). However, Fernandes et al. (2015) and Öztürk et al. (2010) did not observe significant differences in tuber DM, starch, and protein concentrations between low and high soil P availability. The inconsistency of these results might be due to cultivar differences in DM and starch concentration. Furthermore, the P-deficient condition reported in those studies might be less severe, compared with this study, and therefore did not alter the DM, starch, and protein concentrations in the tubers. The sugar concentration of the tubers is also important for the fresh market (Storey, 2007). In this study, P deficiency enhanced sugar concentration in the tubers for Agria and Milva, but it had no significant effect on those of Lilly (Figure 7D). This indicates that under P efficiency conditions, the limited carbohydrates allocated into the tubers might be converted into sugars rather than starch. Similar to the findings of this study, Xing et al. (2020) reported a non-significant relationship between soil P availability and total soluble sugars in tubers, because some potato tubers grown under low soil P conditions also contain a relatively high sugar concentration.
The analyses of ascorbic acid, antioxidant capacity, and minerals of the potato tubers revealed a reduction of ascorbic acid in all the cultivars except for Milva, and an increase in TPC, TFC, DPPH, TEAC, minerals, and ions under P-deficient conditions (Figures 7E–I; Table 2). The concentration of ascorbic acid under different P treatments was within the ranges (0.10–0.25 mg g−1 FW) of potatoes in the markets (Storey, 2007), which implies that the reduction of ascorbic acid under P deficiency could be neglected. Ascorbic acid accounts for about 13% of the total antioxidant capacity of tubers (Storey, 2007). Therefore, about 20% reduction in ascorbic acid under P-deficient conditions could be compensated by an increase in other antioxidants, such as TPC and TFC, while increasing the total antioxidant capacity significantly under these conditions. Although tuber sample extraction for antioxidant capacity measurement was conducted using methanol, which aimed for hydrophilic antioxidants (Kaur et al., 2013), these antioxidants contribute the most to the total antioxidant capacity of potato tubers (Andre et al., 2007). The accumulation of these antioxidants is caused by oxidative stress induced by P deficiency, which triggers numerous plant response reactions for antioxidant systems (Wang and Frei, 2011). The increased concentration of minerals in the leaves also contributed to improving the concentration of these minerals and ions in tubers. However, nitrate concentration in tubers under P-deficient conditions exceeded the general range (<200 mg kg−1 FW) for potato, based on the classification of vegetables, according to nitrate concentration published by Santamaria (2006). High nitrate concentration could be caused by a higher proportion of tuber skin when the size of tubers is small under P-deficient conditions (data not shown). Increased concentrations of protein, minerals, and ions, except P and phosphate, under P-deficient conditions, could also be a result of tuber yield reduction; thus, the concentration is less diluted by the shortage of carbohydrates (Wang and Frei, 2011). The increased concentrations of tuber phytochemicals and minerals are valuable to promote the health and physical well-being of consumers (Andre et al., 2007; Wang and Frei, 2011).
There is a significant variation in plant responses to P deficiency that exists among the tested potato cultivars. We could identify the P-efficient cultivars, Agria, Milva, and Lilly, possessing substantial plant biomass, tuber yield, and high PUpE under low P supply conditions, and they may be suitable for production under limited P conditions. The P-inefficient cultivars, Lady Claire, Sieglinde, and Verdi, lacked the efficiency for P uptake and the ability to produce tubers under P-deficient conditions. However, these cultivars may be efficient in P uptake at high P availability in the soils, which leads to a reduction of P loss in the environment. In response to the low supply of P, potato plants attempted to maintain essential ions (such as phosphate, nitrate, and sulfate) and compatible solutes (such as proline and sucrose) to improve internal PUE and acclimate stress, induced by P deficiency. Leaf photosynthesis also decreased under P-deficient conditions, which was associated with ATP reduction, and further resulted in reduced plant biomass and tuber yield. Furthermore, even though P deficiency significantly reduced tuber DM, concentrations of sugars and minerals, and antioxidant capacity were enhanced for the P-efficient cultivars, which can contribute to better nutritional properties of potatoes. These results indicate a possibility to improve P efficiency and tuber quality of potatoes under P-deficient conditions using P-efficient cultivars. Therefore, in the future, focus on evaluating molecular mechanisms related to P and sucrose transporters will increase the knowledge of internal PUE under limited P availability conditions.
The original contributions presented in the study are included in the article/Supplementary Material, further inquiries can be directed to the corresponding author.
LC performed the experiment, analyzed the data, and drafted the manuscript. CM and AM contributed to photosynthetic measurements and data analyses and revised the manuscript. EP and MN supervised, designed the experiment, and revised the manuscript. All the authors read and approved the final version of the manuscript.
This research was funded by Research Grants for Doctoral Programs in Germany of the German Academic Exchange Service (DAAD), Grant No: 57381412.
The authors declare that the research was conducted in the absence of any commercial or financial relationships that could be construed as a potential conflict of interest.
All claims expressed in this article are solely those of the authors and do not necessarily represent those of their affiliated organizations, or those of the publisher, the editors and the reviewers. Any product that may be evaluated in this article, or claim that may be made by its manufacturer, is not guaranteed or endorsed by the publisher.
The authors would like to thank the German Academic Exchange Service (DAAD) for providing a scholarship to LC. We also would like to thank Kairan Zhang for providing numerous supports on data collection, Merle Tränkner for suggestions on photosynthesis data analyses, Heinz Coners for provisioning weather dataset, Susanne Koch for the support on soil P analyses, and the technical staff at the Division Quality of Plant Products for their support in sample preparation and analyses. We also acknowledge Meijer Potato, the Netherlands, and Europlant Pflanzenzucht GmbH, Germany, for the provision of potato seeds. We acknowledge the support by the Open Access Publication Funds of Göttingen University.
The Supplementary Material for this article can be found online at: https://www.frontiersin.org/articles/10.3389/fpls.2021.723862/full#supplementary-material
Andre, C. M., Ghislain, M., Bertin, P., Oufir, M., Herrera, M. R., Hoffmann, L., et al. (2007). Andean potato cultivars (Solanum tuberosum L.) as a source of antioxidant and mineral micronutrients. J. Agric. Food Chem. 55, 366–378. doi: 10.1021/jf062740i
AOAC (2005). “AOAC official method 990.30: Protein (crude) in animal feed,” in Official Methods of Analysis of AOAC International (Arlington, VA: AOAC International).
Ashraf, M., and Foolad, M. R. (2007). Roles of glycine betaine and proline in improving plant abiotic stress resistance. Environ. Exp. Bot. 59, 206–216. doi: 10.1016/j.envexpbot.2005.12.006
Astier, J., Gross, I., and Durner, J. (2018). Nitric oxide production in plants: an update. J. Exp. Bot. 69, 3401–3411. doi: 10.1093/jxb/erx420
Carstensen, A., Herdean, A., Schmidt, S. B., Sharma, A., Spetea, C., Pribil, M., et al. (2018). The Impacts of phosphorus deficiency on the photosynthetic electron transport chain. Plant Physiol. 177, 271–284. doi: 10.1104/pp.17.01624
Chaudhary, M. I., Adu-Gyamfi, J. J., Saneoka, H., Nguyen, N. T., Suwa, R., Kanai, S., et al. (2008). The effect of phosphorus deficiency on nutrient uptake, nitrogen fixation and photosynthetic rate in mashbean, mungbean and soybean. Acta Physiol. Plant 30, 537–544. doi: 10.1007/s11738-008-0152-8
Chea, L., Pfeiffer, B., Schneider, D., Daniel, R., Pawelzik, E., and Naumann, M. (2021). Morphological and metabolite responses of potatoes under various phosphorus levels and their amelioration by plant growth-promoting rhizobacteria. Int. J. Mol. Sci. 22:5162. doi: 10.3390/ijms22105162
Cordell, D., and White, S. (2014). Life's bottleneck: sustaining the world's phosphorus for a food secure future. Annu. Rev. Environ. Resour. 39, 161–188. doi: 10.1146/annurev-environ-010213-113300
Dixon, M., Simonne, E., Obreza, T., and Liu, G. (2020). Crop response to low phosphorus bioavailability with a focus on tomato. Agronomy 10:617. doi: 10.3390/agronomy10050617
Fernandes, A. M., Soratto, R. P., Moreno, L. d. A., and Evangelista, R. M. (2015). Effect of phosphorus nutrition on quality of fresh tuber of potato cultivars. Bragantia 74, 102–109. doi: 10.1590/1678-4499.0330
Fredeen, A. L., Rao, I. M., and Terry, N. (1989). Influence of phosphorus nutrition on growth and carbon partitioning in Glycine max. Plant Physiol. 89, 225–230. doi: 10.1104/pp.89.1.225
Fu, Y.-F., Zhang, Z.-W., and Yuan, S. (2018). Putative connections between nitrate reductase S-nitrosylation and NO synthesis under pathogen attacks and abiotic stresses. Front. Plant Sci. 9:474. doi: 10.3389/fpls.2018.00474
García-Caparrós, P., Lao, M. T., Preciado-Rangel, P., and Sanchez, E. (2021). Phosphorus and carbohydrate metabolism in green bean plants subjected to increasing phosphorus concentration in the nutrient solution. Agronomy 11:245. doi: 10.3390/agronomy11020245
Gomez, K. A., and Gomez, A. A. (1984). Statistical Procedures for Agricultural Research. Toronto ON: John Wiley and Sons.
Hartmann, T., Wollmann, I., You, Y., and Müller, T. (2019). Sensitivity of three phosphate extraction methods to the application of phosphate species differing in immediate plant availability. Agronomy 9:29. doi: 10.3390/agronomy9010029
Hayat, S., Hayat, Q., Alyemeni, M. N., Wani, A. S., Pichtel, J., and Ahmad, A. (2012). Role of proline under changing environments: a review. Plant Signal. Behav. 7, 1456–1466. doi: 10.4161/psb.21949
Heuer, S., Gaxiola, R., Schilling, R., Herrera-Estrella, L., López-Arredondo, D., Wissuwa, M., et al. (2017). Improving phosphorus use efficiency: a complex trait with emerging opportunities. Plant J. 90, 868–885. doi: 10.1111/tpj.13423
Karlsson, P. M., Herdean, A., Adolfsson, L., Beebo, A., Nziengui, H., Irigoyen, S., et al. (2015). The Arabidopsis thylakoid transporter PHT4;1 influences phosphate availability for ATP synthesis and plant growth. Plant J. 84, 99–110. doi: 10.1111/tpj.12962
Kaur, C., Walia, S., Nagal, S., Walia, S., Singh, J., Singh, B. B., et al. (2013). Functional quality and antioxidant composition of selected tomato (Solanum lycopersicon L) cultivars grown in Northern India. Food Sci. Technol. 50, 139–145. doi: 10.1016/j.lwt.2012.06.013
King, K. W., Williams, M. R., Macrae, M. L., Fausey, N. R., Frankenberger, J., Smith, D. R., et al. (2015). Phosphorus transport in agricultural subsurface drainage: a review. J. Environ. Qual. 44, 467–485. doi: 10.2134/jeq2014.04.0163
Koch, M., Busse, M., Naumann, M., Jákli, B., Smit, I., Cakmak, I., et al. (2019). Differential effects of varied potassium and magnesium nutrition on production and partitioning of photoassimilates in potato plants. Physiol. Plant. 166, 921–935. doi: 10.1111/ppl.12846
Koch, M., Winkelmann, M. K., Hasler, M., Pawelzik, E., and Naumann, M. (2020). Root growth in light of changing magnesium distribution and transport between source and sink tissues in potato (Solanum tuberosum L.). Sci. Rep. 10:8796. doi: 10.1038/s41598-020-72313-y
Launay, A., Cabassa-Hourton, C., Eubel, H., Maldiney, R., Guivarc'h, A., Crilat, E., et al. (2019). Proline oxidation fuels mitochondrial respiration during dark-induced leaf senescence in Arabidopsis thaliana. J. Exp. Bot. 70, 6203–6214. doi: 10.1093/jxb/erz351
Lee, W. C., Liu, G., and Alva, A. K. (2013). Potato cultivar evaluation for phosphorus-use efficiency. J. Crop Improv. 27, 617–626. doi: 10.1080/15427528.2013.814039
Lei, M., Liu, Y., Zhang, B., Zhao, Y., Wang, X., Zhou, Y., et al. (2011). Genetic and genomic evidence that sucrose is a global regulator of plant responses to phosphate starvation in Arabidopsis. Plant Physiol. 156, 1116–1130. doi: 10.1104/pp.110.171736
Leonel, M., do Carmo, E. L., Fernandes, A. M., Soratto, R. P., Ebúrneo, J. A. M., Garcia, É. L., et al. (2017). Chemical composition of potato tubers: the effect of cultivars and growth conditions. J. Food Sci. Technol. 54, 2372–2378. doi: 10.1007/s13197-017-2677-6
Long, L., Ma, X., Ye, L., Zeng, J., Chen, G., and Zhang, G. (2019). Root plasticity and Pi recycling within plants contribute to low-P tolerance in Tibetan wild barley. BMC Plant Biol. 19:341. doi: 10.1186/s12870-019-1949-x
Mauromicale, G., Ierna, A., and Marchese, M. (2006). Chlorophyll fluorescence and chlorophyll content in field-grown potato as affected by nitrogen supply, genotype, and plant age. Photosynt. 44:76. doi: 10.1007/s11099-005-0161-4
Misson, J., Raghothama, K. G., Jain, A., Jouhet, J., Block, M. A., Bligny, R., et al. (2005). A genome-wide transcriptional analysis usingArabidopsis thaliana Affymetrix gene chips determined plant responses to phosphate deprivation. Proc. Natl. Acad. Sci. U. S. A. 102, 11934–11939. doi: 10.1073/pnas.0505266102
Murphy, J., and Riley, J. P. (1962). A modified single solution method for the determination of phosphate in natural waters. Anal. Chim. Acta 27, 31–36. doi: 10.1016/S0003-2670(00)88444-5
Naumann, M., Koch, M., Thiel, H., Gransee, A., and Pawelzik, E. (2020). The Importance of nutrient management for potato production part II: plant nutrition and tuber quality. Potato Res. 63, 121–137. doi: 10.1007/s11540-019-09430-3
Nielsen, T. H., Wischmann, B., Enevoldsen, K., and Moller, B. L. (1994). Starch phosphorylation in potato tubers proceeds concurrently with de Novo biosynthesis of starch. Plant Physiol. 105, 111–117. doi: 10.1104/pp.105.1.111
Nunes-Nesi, A., Fernie, A. R., and Stitt, M. (2010). Metabolic and signaling aspects underpinning the regulation of plant carbon nitrogen interactions. Mol. Plant 3, 973–996. doi: 10.1093/mp/ssq049
Öztürk, E., Kavurmacı, Z., Kara, K., and Polat, T. (2010). The effects of different nitrogen and phosphorus rates on some quality traits of potato. Potato Res. 53, 309–312. doi: 10.1007/s11540-010-9176-8
Perchlik, M., and Tegeder, M. (2018). Leaf amino acid supply affects photosynthetic and plant nitrogen use efficiency under nitrogen stress. Plant Physiol. 178, 174–188. doi: 10.1104/pp.18.00597
Plénet, D., Etchebest, S., Mollier, A., and Pellerin, S. (2000). Growth analysis of maize field crops under phosphorus deficiency. Plant Soil 223, 119–132. doi: 10.1023/A:1004877111238
Ramos-Artuso, F., Galatro, A., Lima, A., Batthyány, C., and Simontacchi, M. (2019). Early events following phosphorus restriction involve changes in proteome and affects nitric oxide metabolism in soybean leaves. Environ. Exp. Bot. 161, 203–217. doi: 10.1016/j.envexpbot.2019.01.002
Rouached, H. (2011). Multilevel coordination of phosphate and sulfate homeostasis in plants. Plant Signal. Behav. 6, 952–955. doi: 10.4161/psb.6.7.15318
Royo, B., Moran, J. F., Ratcliffe, R. G., and Gupta, K. J. (2015). Nitric oxide induces the alternative oxidase pathway in Arabidopsis seedlings deprived of inorganic phosphate. J. Exp. Bot. 66, 6273–6280. doi: 10.1093/jxb/erv338
Sandaña, P. (2016). Phosphorus uptake and utilization efficiency in response to potato genotype and phosphorus availability. Eur. J. Agron. 76, 95–106. doi: 10.1016/j.eja.2016.02.003
Santamaria, P. (2006). Nitrate in vegetables: toxicity, content, intake and EC regulation. J. Sci. Food Agric. 86, 10–17. doi: 10.1002/jsfa.2351
Shen, J., Yuan, L., Zhang, J., Li, H., Bai, Z., Chen, X., et al. (2011). Phosphorus dynamics: from soil to plant. Plant Physiol. 156, 997–1005. doi: 10.1104/pp.111.175232
Shi, Q., Pang, J., Yong, J. W. H., Bai, C., Pereira, C. G., Song, Q., et al. (2019). Phosphorus-fertilisation has differential effects on leaf growth and photosynthetic capacity of Arachis hypogaea L. Plant Soil 447, 99–116. doi: 10.1007/s11104-019-04041-w
Simkin, A. J., López-Calcagno, P. E., and Raines, C. A. (2019). Feeding the world: improving photosynthetic efficiency for sustainable crop production. J. Exp. Bot. 70, 1119–1140. doi: 10.1093/jxb/ery445
Singh, S. K., and Reddy, V. R. (2015). Response of carbon assimilation and chlorophyll fluorescence to soybean leaf phosphorus across CO2: alternative electron sink, nutrient efficiency and critical concentration. J. Photochem. Photobiol. B. Biol. 151, 276–284. doi: 10.1016/j.jphotobiol.2015.08.021
Sonntag, F. E. H., Bunzel, D., Kulling, S., Porath, I., Pach, F., Pawelzik, E., et al. (2020). Effect of potassium fertilization on the contents of antioxidants in three cocktail tomato cultivars. J. Appl. Bot. Food Qual. 93, 34–43. doi: 10.5073/JABFQ.2020.093.005
Soratto, R. P., Pilon, C., Fernandes, A. M., and Moreno, L. A. (2015). Phosphorus uptake, use efficiency, and response of potato cultivars to phosphorus levels. Potato Res. 58, 121–134. doi: 10.1007/s11540-015-9290-8
Storey, M. (2007). “The harvested crop,” in Potato Biology and Biotechnology: Advances and Perspectives, eds D. Vreugdenhil and J. Bradshaw (Amsterdam, Boston: Elsevier), 441–470. doi: 10.1016/B978-044451018-1/50063-4
Veneklaas, E. J., Lambers, H., Bragg, J., Finnegan, P. M., Lovelock, C. E., Plaxton, W. C., et al. (2012). Opportunities for improving phosphorus-use efficiency in crop plants. New Phytol. 195, 306–320. doi: 10.1111/j.1469-8137.2012.04190.x
Wacker-Fester, K., Uptmoor, R., Pfahler, V., Dehmer, K. J., Bachmann-Pfabe, S., and Kavka, M. (2019). Genotype-specific differences in phosphorus efficiency of potato (Solanum tuberosum L.). Front. Plant Sci. 10:1029. doi: 10.3389/fpls.2019.01029
Wang, X., Shen, J., and Liao, H. (2010). Acquisition or utilization, which is more critical for enhancing phosphorus efficiency in modern crops? Plant Sci. 179, 302–306. doi: 10.1016/j.plantsci.2010.06.007
Wang, Y., and Frei, M. (2011). Stressed food – the impact of abiotic environmental stresses on crop quality. Agric. Ecosyst. Environ. 141, 271–286. doi: 10.1016/j.agee.2011.03.017
Wissuwa, M., Gamat, G., and Ismail, A. M. (2005). Is root growth under phosphorus deficiency affected by source or sink limitations? J. Exp. Bot. 56, 1943–1950. doi: 10.1093/jxb/eri189
Xing, Y., Niu, X., Wang, N., Jiang, W., Gao, Y., and Wang, X. (2020). The Correlation between soil nutrient and potato quality in loess plateau of china based on PLSR. Sustainability 12:1588. doi: 10.3390/su12041588
Keywords: ATP, cultivars, phosphorus deficiency, phosphorus efficiency, leaf, potato, sugars, tuber quality
Citation: Chea L, Meijide A, Meinen C, Pawelzik E and Naumann M (2021) Cultivar-Dependent Responses in Plant Growth, Leaf Physiology, Phosphorus Use Efficiency, and Tuber Quality of Potatoes Under Limited Phosphorus Availability Conditions. Front. Plant Sci. 12:723862. doi: 10.3389/fpls.2021.723862
Received: 11 June 2021; Accepted: 22 July 2021;
Published: 30 August 2021.
Edited by:
Haijun Gong, Northwest A&F University, ChinaReviewed by:
Mareike Kavka, University of Rostock, GermanyCopyright © 2021 Chea, Meijide, Meinen, Pawelzik and Naumann. This is an open-access article distributed under the terms of the Creative Commons Attribution License (CC BY). The use, distribution or reproduction in other forums is permitted, provided the original author(s) and the copyright owner(s) are credited and that the original publication in this journal is cited, in accordance with accepted academic practice. No use, distribution or reproduction is permitted which does not comply with these terms.
*Correspondence: Marcel Naumann, bWFyY2VsLm5hdW1hbm5AYWdyLnVuaS1nb2V0dGluZ2VuLmRl
Disclaimer: All claims expressed in this article are solely those of the authors and do not necessarily represent those of their affiliated organizations, or those of the publisher, the editors and the reviewers. Any product that may be evaluated in this article or claim that may be made by its manufacturer is not guaranteed or endorsed by the publisher.
Research integrity at Frontiers
Learn more about the work of our research integrity team to safeguard the quality of each article we publish.