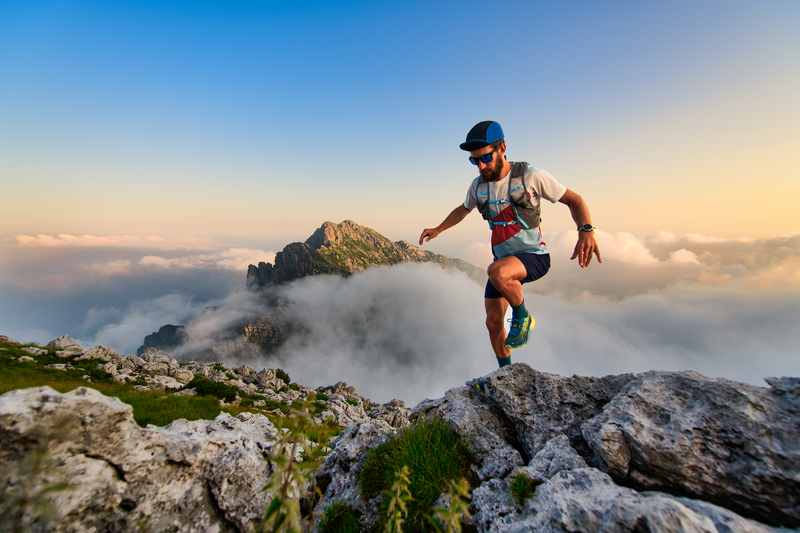
95% of researchers rate our articles as excellent or good
Learn more about the work of our research integrity team to safeguard the quality of each article we publish.
Find out more
MINI REVIEW article
Front. Plant Sci. , 20 October 2021
Sec. Plant Metabolism and Chemodiversity
Volume 12 - 2021 | https://doi.org/10.3389/fpls.2021.719003
This article is part of the Research Topic The Back-and-Forth between Metabolism and Photosystem I View all 7 articles
Plant productivity greatly relies on a flawless concerted function of the two photosystems (PS) in the chloroplast thylakoid membrane. While damage to PSII can be rapidly resolved, PSI repair is complex and time-consuming. A major threat to PSI integrity is acceptor side limitation e.g., through a lack of stromal NADP ready to accept electrons from PSI. This situation can occur when oscillations in growth light and temperature result in a drop of CO2 fixation and concomitant NADPH consumption. Plants have evolved a plethora of pathways at the thylakoid membrane but also in the chloroplast stroma to avoid acceptor side limitation. For instance, reduced ferredoxin can be recycled in cyclic electron flow or reducing equivalents can be indirectly exported from the organelle via the malate valve, a coordinated effort of stromal malate dehydrogenases and envelope membrane transporters. For a long time, the NADP(H) was assumed to be the only nicotinamide adenine dinucleotide coenzyme to participate in diurnal chloroplast metabolism and the export of reductants via this route. However, over the last years several independent studies have indicated an underappreciated role for NAD(H) in illuminated leaf plastids. In part, it explains the existence of the light-independent NAD-specific malate dehydrogenase in the stroma. We review the history of the malate valve and discuss the potential role of stromal NAD(H) for the plant survival under adverse growth conditions as well as the option to utilize the stromal NAD(H) pool to mitigate PSI damage.
Oxygenic photosynthesis started to evolve over 3 billion years ago (Blankenship, 2010; Sánchez-Baracaldo and Cardona, 2020). It allowed for CO2 fixation into carbohydrates and other energy rich compounds releasing O2 as a byproduct. This enabled oxygen-requiring metabolic and biosynthetic pathways which form the basis for higher multicellular life on earth (Schirrmeister et al., 2013). Although the conversion of light energy into chemical energy has fascinated scientists for centuries, many aspects of the underlying biochemistry are still not fully understood. Land plants represent the main food resource for humans, livestock, and wild animals. In addition, their importance as renewable energy carriers and sustainable building materials have increased drastically over the last decades. In a world undergoing climate change with more adverse weather conditions, humankind has to overcome many challenges to meet the food and energy demand of the growing global population (Popp et al., 2014). As part of this effort, a detailed understanding of the molecular mechanisms governing photosynthetic efficiency in the plant chloroplast is indispensable. This includes knowledge on how plants rapidly acclimate to the dynamic growth conditions they face in the field e.g., shifts in light intensity and temperature. Several molecular processes housed in the chloroplast have emerged as critical for acclimation, emphasizing the central importance of the organelle (Kleine et al., 2021). Insights from this research will inform the design of crop plants with improved tolerance against adverse growth conditions.
Recently, we reviewed the dynamic regulation of the proton motive force (pmf) across the chloroplast thylakoid membrane in response to light intensity shifts (Armbruster et al., 2017). In this mini review, we briefly revisit the electron transport in the photosynthetic machinery of C3 plants and the mechanisms that protect the two photosystems (PSII and PSI) at the level of the thylakoid membrane (Figure 1A). We then focus on the current understanding of the stromal redox pools downstream of PSI, plastid export of reducing equivalents, and new insights regarding the involved coenzymes (Figure 1B). We close by discussing future research directions.
Figure 1. Plastid electron (e−) flow and indirect export of reducing equivalents. (A) Cyclic (red) and linear (blue) e− flow/ transport (CEF and LEF, respectively) across the thylakoid membrane. Recycling e− from reduced FD back to PQ by CEF allows for ATP production without NADPH formation. The pmf generated by LEF and CEF drives ATP synthesis at the ATP synthase complex. An excess of reduced PQH2 can be avoided via PTOX activity (modified from Yamamoto and Shikanai, 2020). (B) Indirect export of reducing equivalents from the stroma to prevent PSI acceptor side limitation. The terminal PSI e− acceptor NADP is resupplied to FNR through NADPH oxidation in the CBBC and by export of surplus reducing equivalents out of the chloroplast. Fatty acid synthesis in the light may provide another e− sink. Reducing power is transferred to the cytosol via TPT in form of TP or through OMT as malate. 3-PGA can be reduced to TP by GapAB in the plastid and be regenerated in the cytosol by GapC or GapN. Reduction of oxaloacetate to malate is driven by plNAD-MDH or plNADP-MDH fueled by the respective redox pools. The CBBC interconnects the NAD(H) and NADP(H) redox pools. This is mediated by PGDH3. Stromal NADP+ synthesis relies on NAD+ import and phosphorylation by NADK2. 3-PGA, 3-Phosphoglyceric acid; ADP, adenosine diphosphate; ATP, adenosine triphosphate; CEF, cyclic electron flow; cy, cytosolic; Cyt b6f, cytochrome b6f; FAS, fatty acid synthase; FD, ferredoxin; FNR, ferredoxin:NADP(H) oxidoreductase; GapA/B, bispecific (NAD+/NADP+-dependent) glyceraldehyde 3-phosphate dehydrogenase; GapC, cytosolic glyceraldehyde 3-phosphate dehydrogenase; GapN, non-phosphorylating irreversible glyceraldehyde 3-phosphate dehydrogenase; H, hydrogen; LEF, linear electron flow; MDH, malate dehydrogenase; mt, mitochondrial; NAD(H), nicotinamide adenine dinucleotide; NADK2, nicotinamide adenine dinucleotide kinase 2; NADP(H), nicotinamide adenine dinucleotide phosphate; NDH, NADH dehydrogenase; NPQ, non-photochemical quenching; O2, molecular oxygen; OAA, oxaloacetate; OMT, oxaloacetate malate transporter; PC, plastocyanin; PDCp, pyruvate dehydrogenase complex; pe, peroxisomal; PEP, 2-phosphoenolpyruvate; PGR5, proton gradient regulation 5; PGRL1, PGR5-like photosynthetic phenotype 1; Pi, inorganic phosphate; pl, plastid; pmf, proton motive force; PQ, plastoquinone; PQH2, plastoquinol; PSI, photosystem I; PSII, photosystem II; PTOX, plastid terminal oxidase; TP, triose-phosphate; TPT, triose-phosphate/phosphate translocator (Created with BioRender.com).
Light energy absorbed by the light harvesting complexes (LHC) causes charge separation in the chlorophyll a (Chl a) molecules located at the P680 reaction center of PSII (Figure 1A). Subsequently, the excited P680* passes an electron (e−) on to the first e− acceptor of the electron transport chain (ETC). The e− gap in the oxidized P680+ molecule is filled through the splitting of water molecules which results in the release of e− and molecular O2 (McEvoy and Brudvig, 2006). From P680* e− are shuttled via plastoquinone (PQ) to plastoquinol (PHQ) reduction through the lipid matrix of the thylakoid membrane to the cytochrome b6f complex (Cyt b6f). Cyt b6f transfers the e− over to the luminal e− carrier protein plastocyanin (PC). Reduced PC travels the longest distance in the ETC by diffusion to the PSI donor side where it re-reduces P700+ (Höhner et al., 2020). Through a second light-driven process an excited P700* molecule is generated. At the PSI acceptor side, P700* passes its e− on to ferredoxin. Finalizing the linear e− flow (LEF) reaction steps, the stromal enzyme ferredoxin-NADP+ oxidoreductase (FNR) reduces NADP+ to NADPH (Mulo, 2011). In summary, LEF transfers light energy captured by the thylakoid membrane-bound PS complexes into biochemically stored energy in the form of stromal NADPH. Together with ferredoxin, NADPH is the primary driver of stromal redox reactions and thus critical for the chloroplast redox poise (Geigenberger and Fernie, 2014). During LEF, the water splitting reaction and Cyt b6f activity release H+ into the lumen. This sets up a proton gradient ΔpH across thylakoid membrane. ΔpH and Δψ, the thylakoid membrane potential, together constitute the pmf, which drives stromal ATP production via ATP synthase (Kramer et al., 2003). NADPH and ATP fuel the Calvin Bassham Benson Cycle (CBBC) resulting in CO2-fixation, synthesis of triose-phosphates, and regeneration of NADP (Scheibe, 2004; Kramer and Evans, 2011; Dietz et al., 2016). NADPH consumption by the CBBC and other pathways relies on the dynamic growth conditions of plants (Gururani et al., 2015). A shortage of NADP ready to accept e− results in PSI acceptor side limitation (Chaux et al., 2015; Kanazawa et al., 2017). In this situation O2 acts as an e− acceptor triggering reactive oxygen species (ROS) production and concomitant PSI damage (Shimakawa and Miyake, 2018). Differently from PSII, which is repaired so rapidly that plants voluntarily sacrifice it if needed, PSI's turnover time is extremely long (Rantala et al., 2020). Consequently, plants have evolved several PSI protective mechanisms at the thylakoids and in the stroma including transport processes across the envelope membrane which effectively mitigate the risk of PSI damage (Munekage et al., 2002; Alric and Johnson, 2017).
A significant role in PSI protection can be assigned to the acidity of the thylakoid lumen. During light phases, low luminal pH activates the PsbS protein, which in combination with carotenoid pigments participating in the xanthophyll cycle regulates non-photochemical quenching (NPQ) (Jahns and Holzwarth, 2012). NPQ mostly causes heat dissipation at the PSII antenna which decreases the energy flux into the ETC and the amount of e− rushing toward PSI via LEF. In addition, high ΔpH slows down PQH2 oxidation at Cyt b6f and therefore LEF e− transport. This process is called photosynthetic control (Foyer et al., 2012).
Plant chloroplasts can also uncouple ATP from NADPH production in a process designated cyclic electron flow (CEF) around PSI. CEF diverts energy into the ATP pool without NADPH production (Shikanai and Yamamoto, 2017). This allows to finetune the ATP-NADPH stoichiometry according to the CBBC requirements and to decrease PSI acceptor side limitation by providing an alternative route for reduced ferredoxin (Kramer and Evans, 2011). Two CEF pathways exist in parallel: (1) PGR5/PGRL1 and (2) the NADH dehydrogenase-like (NDH) complex. Both utilize ferredoxin for PQH2 oxidoreduction. Their exact in vivo function and relative contribution to CEF are still under debate and very actively investigated (Shikanai and Yamamoto, 2017). An overaccumulation of reduced PQH2 can be prevented via the thylakoid membrane bound plastid terminal oxidase (PTOX). PTOX oxidizes PQH2 by reducing O2 to H2O (Alric and Johnson, 2017).
Lastly, imbalances between PSII and PSI excitation especially under fluctuating light conditions need to be avoided to prevent e− excess at PSI (Grieco et al., 2012). Therefore, the mobile part of LHCII can switch between two states depending on reversible phosphorylation and either preferentially associate with PSII or PSI (state transition) (Pesaresi et al., 2011). LHCII phosphorylation in Arabidopsis is primarily catalyzed by the STN7 kinase. STN7 activity in turn, is partly coupled to the light dependent redox state of the PQ pool. Under low or ambient light conditions, the PQ pool remains oxidized. LHCII is docked to PSII and LEF prevails (State 1). Under light conditions that yield in a reduced PQ pool, i.e. buildup of PQH2 (such as fluctuating light), STN7 becomes active resulting in LHCII phosphorylation and migration to PSI (State 2). This situation supports CEF and overall protects PSI (Minagawa, 2011). Additionally, it was shown in Arabidopsis that acetylation of several photosynthesis components via the acetyltransferase NSI/ GNAT2 is also required for state transitions (Koskela et al., 2018, 2020).
Two export routes are known in C3 plant chloroplasts to indirectly provide reducing equivalents to other organelles to replenish stromal NADP (Figure 1B): the triose-phosphate/phosphate translocator (TPT) and the malate valve (aka malate-oxaloacetate (OAA) shuttle) (Dietz et al., 2016). Both contribute to the avoidance of PSI acceptor side limitation. Flux through the TPT is dependent on the prevailing Pi availability, which is coupled to the chloroplast's energy status, i.e., ATP availability (Flugge et al., 2011). The malate valve represents an independent alternative that connects chloroplast LEF with the cytosol, mitochondria, and peroxisomes via a process called malate circulation (Figure 1B) (Scheibe, 2004). Outside the plastid a plethora of dehydrogenases function to regenerated reducing equivalents in the respective organelles (Figure 1B). For detailed information on the characterization of these enzymes, we refer to several studies (Pracharoenwattana et al., 2007; Tomaz et al., 2010; Schneider et al., 2018; Liszka et al., 2020). The flux through the plastid malate valve will be the focus of the remaining article.
The plastid malate valve consists of an oxaloacetate/malate transporter (OMT1 or DiT1) in the inner envelope (Kinoshita et al., 2011) and two different malate dehydrogenases (MDH) to reduce stromal OAA to malate (Selinski and Scheibe, 2019). The two dehydrogenases differ in their coenzyme preference. The NADP-MDH is highly specific for NADP(H) (Scheibe, 1987), while the other isoform strongly prefers NAD(H) (NAD-MDH) (Berkemeyer et al., 1998). The co-existence of both MDHs has puzzled scientists for decades. The mainstream opinion has been that illuminated leaf chloroplasts use NAD merely to convert it into NADP via NAD kinase 2 (NADK2) (Figure 1B). NADH cannot be phosphorylated (Turner et al., 2004). Many reports have described FNR's strong coenzyme preference for NADP(H) (Correll et al., 1993; Piubelli et al., 2000; Medina et al., 2001; Hermoso et al., 2002). Therefore, photosynthesis and redox pathways should primarily depend on NADP(H) and not utilize NAD(H). It has also been assumed that transhydrogenase activity in the chloroplast stroma, i.e., enzymatic NADPH to NADH conversion, would affect photosynthesis by interfering with the carefully balanced NADP(H) production and consuming pathways, most importantly the CBBC (Krause and Heber, 1976). Until now, the vast majority of plastid biochemical pathways, especially the light-dependent and CO2 fixation reactions are depicted exclusively with NADP(H). However, if this were correct it seems hard to comprehend why C3 plant species possess NAD-dependent MDHs with confirmed enzyme activity in light and dark-incubated chloroplasts (Backhausen et al., 1998). Indeed, evidence suggesting a significant function for NAD(H) in illuminated chloroplasts has been growing over the last years (Zhao et al., 2018; Höhner et al., 2021).
The NADP-MDH contains conserved cysteine residues in its N and C-terminus that function as a redox switch to keep the enzyme active only in the light, when the stroma is reduced (high availability of NADPH). In vitro this effect is achieved by adding DTTred into extraction and assay buffers (Carr et al., 1999). Many experiments confirmed that NADP-MDH is highly active under reducing conditions but essentially inactive in the dark (Scheibe and Stitt, 1988). Surprisingly, several studies on chloroplast extracts from different C3 species reported substantial NAD-MDH activity regardless of the redox-state (Neuhaus et al., 1993; Backhausen et al., 1998; Selinski et al., 2014). This brought up the question: How can these two reactions coexist? One explanation is the different regulation of the two enzymes. NADP-MDH needs a reduced environment to function, while NAD-MDH isoforms, which lack several conserved cysteine residues, do not (Berkemeyer et al., 1998). Therefore, export of reducing equivalents in the light, especially in situations when the CBBC capacity and concomitant NADPH consumption drop, is facilitated via the NADP-MDH malate valve to ensure NADP for PSI (Okegawa and Motohashi, 2015; Thormählen et al., 2017; Selinski and Scheibe, 2019). However, at night NADP-MDH activity may create a futile cycle with the oxidative pentose phosphate pathway (OPPP) which produces NADPH (Kruger and von Schaewen, 2003; Sharkey and Weise, 2015). Therefore, NADP-MDH needs to stay inactive to avoid interference with the metabolism and risking plant survival during night phases.
Conversely, the physiologically assigned role for NAD-MDH has been participation in the chloroplast's dark metabolism, i.e., the incomplete glycolysis (Selinski et al., 2014). Under these conditions, stromal NADH level should be sufficiently high to drive the malate valve and decrease the reducing equivalent surplus while replenishing NAD for glycolysis. In other words, it was assumed that the absence of diurnal stromal NADH production would keep NAD-MDH inactive until dark metabolism takes over.
While all these assumptions have merit, several discoveries from the C3 model plant Arabidopsis hint at a more complex scenario, suggesting NAD(H) may also play a role in daytime chloroplast metabolism.
1) Loss of the sole NADP-MDH gene does not result in a noticeable phenotype (Hebbelmann et al., 2012; Heyno et al., 2014). If C3 plants relied heavily on the NADP dependent malate valve, shouldn't we expect more dramatic effects especially on photosynthesis and PSI function in NADP-mdh loss-of-function lines? To our knowledge, PSI acceptor side limitation in NADP-mdh mutants has not been reported. Moreover, expression of an NADP-MDH variant that is no longer controlled by the redox poise and thus active during the night did not affect the mutants' growth under long-day conditions. This indicates that the enzyme activity did not significantly affect the OPPP as originally assumed. Interestingly, the mutants were more susceptible to fluctuating light and extended night periods (Yokochi et al., 2021).
2) If a lack of NADP-MDH could be backed up by NAD-MDH, as increases in NAD-MDH transcript level suggest (Selinski and Scheibe, 2014), what enzymes could aid in diverting e− from NADPH into NADH so that it can function as a reducing agent to fuel the malate valve? Plants do not possess classic transhydrogenase enzymes as many bacteria do (Jackson, 2012).
3) By now we know a handful of NAD(H)-dependent stromal reactions. Is it really feasible that these strictly occur in the dark when according to the original hypothesis flux through plastid glycolysis would be sufficient to build up a decently sized stromal NADH pool? Disputing this idea, chloroplast fatty acid synthesis, which includes NAD(H)-dependent steps, is also active during the day (Browse et al., 1981).
4) What are the consequences of losing stromal NAD-MDH activity and the export of reducing equivalents via this route? How does it affect photosynthesis and PSI function? These questions have not been investigated but deserve research attention.
Recent findings on NAD-MDH mutants are highly intriguing but complicate future studies. Independent groups have shown that a homozygous NAD-MDH gene loss via T-DNA insertions is lethal (Beeler et al., 2014; Selinski et al., 2014). Also, a CRISPR/Cas9 approach to isolate loss of function NAD-mdh mutants was unsuccessful (Zhao et al., 2018). Posttranscriptional suppression of NAD-MDH mRNA via artificial microRNA resulted in severely underdeveloped and pale plant mutants (Beeler et al., 2014). Interestingly, NAD-mdh mutant lethality and visual phenotypes in T-DNA mutants were overcome by the expression of a non-functional NAD-MDH variant (Schreier et al., 2018). The authors showed that the developmental defects observed in NAD-mdh amiRNA lines are due to a second, non-enzymatic moonlighting function of the protein. Indeed, this and another study revealed protein-protein interactions between NAD-MDH and components of the heteromeric FtsH12-FtsHi AAA-ATPase complex (Kikuchi et al., 2018; Schreier et al., 2018). In theory, the non-functional enzyme complementing NAD-mdh line could serve as a stepping stone to dissect the NADH-dependent route via the malate valve. This could help to understand NAD-MDH's importance for the export of reducing equivalents and in replenishing NADP to PSI's acceptor side. However, these investigations are complicated because the lines were generated in a Ler-1 (Landsberg erecta) background. Several studies on different Arabidopsis accessions have shown that small genetic variations play a big part in the phenotypic response to light regimes (Maloof et al., 2001; Van Rooijen et al., 2017; Kaiser et al., 2020). Thus, investigating the significance of either plastid MDH-type, which ideally involves the attempt to generate double mutants, is not straightforward. All publicly available NADP-mdh mutants are in Col-0 (Columbia) background.
A promising option is to employ the recently isolated viable NAD-mdh point mutant som410 (suppressor of mosaic death 1). This non-functional NAD-MDH mutant resembles the wild type (Zhao et al., 2018). Interestingly, som410 accumulates less biomass when grown in 16/8 h day-night cycles (long-day light conditions) but more biomass than wild-type plants if grown under constant light (Zhao et al., 2018). This apparent sensitivity to the length of the light period could imply a disturbed stromal redox poise and decreased photosynthesis. The authors suggest that plastid NADP-MDH and NAD-MDH can indeed simultaneously generate malate for export from chloroplasts during the day (Zhao et al., 2020). This would also mean that differently from the previous assumption transhydrogenase activity in the stroma does not occur during this process or it does not affect photosynthesis as originally postulated (Krause and Heber, 1976). The relative contribution of each respective MDHs to the malate valve flux remains to be shown. som410 are Col-0 accession lines and thus may aid in resolving the flux question. This could further clarify the malate valve's importance for flawless PSI function.
An alternative approach to shine light on the importance of the NAD-MDH driven malate valve and stromal NADH is to pinpoint the production routes of NADH in illuminated mesophyll chloroplasts. So far, three different plastid reactions are known to prefer NAD(H) over NADP(H): (1) NAD-specific glyceraldehyde-3-phosphate (Gap) dehydrogenases (GapCp1 and GapCp2) which in Arabidopsis are expressed in heterotrophic tissues (Muñoz-Bertomeu et al., 2009). In chloroplasts, three additional Gap dehydrogenases (GapA1, GapA2, and GapB) exist. Interestingly, they possess dual co-enzyme specificity but only NAD(H)-dependent activity is light-independent (Baalmann et al., 1995; Berkemeyer et al., 1998; Sparla et al., 2002), (2) the plastid Pyruvate dehydrogenase complex (PDCp) which supplies NADH to the enoyl-acyl carrier protein (ACP) reductase (ENR) to initiate fatty acid synthesis (Camp and Randall, 1985), and (3) the phosphorylated pathway of serine biosynthesis (PPSB) (Ros et al., 2014).
PPSB is active in spinach leaves (Larsson and Albertsson, 1979) and therefore a good candidate for NADH production in illuminated chloroplasts. The Arabidopsis genome encodes three different Phosphoglycerate dehydrogenase (PGDH) isoforms (Benstein et al., 2013; Toujani et al., 2013). PGDH utilizes 3-PGA and NAD to catalyze the first of three steps to yield NADH and eventually serine in the stroma. Only PGDH1 and PGDH3 are expressed in leaves. Whereas PGDH3 is restricted to mesophyll cells, PGDH1 is found in autotrophic and heterotrophic tissues (Benstein et al., 2013; Toujani et al., 2013). Homozygous pgdh1 loss of function mutants are not viable (Benstein et al., 2013). Interestingly, PGDH1 and PGDH3 loci differ greatly in their co-expression patterns: PGDH1 clusters with PGDH2 and other components of amino acid metabolism; PGDH3 is co-expressed with subunits of the thylakoid NDH complex suggesting a potential link to PSI and photosynthesis (Casatejada-Anchel et al., 2021; Höhner et al., 2021).
In part, these distinct features encouraged two independent studies focusing on the function of PGDH3 (Casatejada-Anchel et al., 2021; Höhner et al., 2021). The experiments showed that the PGDH3 isoform is a highly specific NAD(H)-dependent dehydrogenase, which is active in mesophyll chloroplasts under reducing conditions as found in the light (Höhner et al., 2021). In line with this, two independent pgdh3 loss of function lines showed alteration in their photosynthetic performance. If grown under continuous long-day light conditions (16/8 h day-night cycles), pgdh3 mutants revealed an increased transient NPQ level under non-saturating actinic light compared to wild-type plants (Höhner et al., 2021). This subtle behavior often indicates delayed CBBC activation and decreased ATPase NADPH consumption. Consequently, CO2 fixation in pgdh3 mutants is lower than in wild-type plants (Casatejada-Anchel et al., 2021; Höhner et al., 2021).
Furthermore, pgdh3 plants have increased PSI acceptor side limitations whereas photosynthetic control seemed unchanged. The metabolome of subcellular enriched fractions collected from pgdh3 mutants suggested a shift in redox-related compounds. Stromal NADH level were lower, whereas the total NADPH pools increased. Interestingly, also the malate pools and thus malate circulation between organelles were subject to changes. Altogether the results point to a significant role of PGDH3 for C3 photosynthesis (Höhner et al., 2021). More importantly, the studies substantiate the evidence that stromal NADH is produced in mesophyll plastids during the day. Differently from PDCp, which works in tight conjunction with fatty acid metabolism, PGDH3 activity yields reduced NADH available for NAD-MDH and other stromal reactions. This provides a path to fuel the plastid malate valve with e− originating from the stromal NADH pool. More importantly, because PGDH3 withdraws 3-PGA and its e− from the CBBC, it represents an elegant indirect route to relay e− from reduced NADPH into the NADH pool (Höhner et al., 2021). This aids in resupplying oxidized NADP to the PSI acceptor side. The involvement of the tightly regulated CBBC allows the chloroplast to keep close tabs on the pathway's flux thereby avoiding the risk of uncontrolled transhydrogenase activity in the stroma that may affect photosynthesis (Höhner et al., 2021).
Arabidopsis upregulates PGDH3 transcription and protein level if plants are subjected to dynamic light stress (Schneider et al., 2019; Niedermaier et al., 2020). Indeed, pgdh3 loss-of-function mutants revealed progressing PSII damage (low Fv/Fm) when fluctuating growth light was applied. In contrast, pgdh3 plants were capable to adjust to constant highlight stress which suggests other mechanisms provide protection under such conditions. Altogether, the data indicate that the NADH-dependent pathway provides a well-needed alternative route to export reducing equivalents from the stroma (Höhner et al., 2021). This is critical during growth phases with sudden light intensity changes, which occur frequently in the field. Since PSI damage occurs when e− acceptors become limited (Miyake, 2020) it is likely that the lack of PGDH3 and decreased stromal NADH production also result in PSI damage. This needs to be tested in the future as it may explain why gene expression of PGDH3 and components of the NDH complex appears to be coordinated (Höhner et al., 2021). Although the structure suggests reduced ferredoxin is the electron donor to NDH (Laughlin et al., 2019; Schuller et al., 2019), in vitro experiments have successfully employed NADH and NADPH as the primary e− donors to drive NDH activity probably via FNR (Sazanov et al., 1998; Rumeau et al., 2005; Strand et al., 2016, 2017). Overall, a coordinated increase in NDH and PGDH3 activity in response to stromal NADPH buildup may be necessary to minimize the risks of PSI damage.
A full understanding of the stromal NAD(H)-dependent pathway in illuminated chloroplasts could inspire new strategies to secure plant productivity during light stress e.g., by protecting PSI through avoiding acceptor side limitations and concomitant damage to PSI. To reach this goal we suggest a few research directions and potential challenges.
Quantitative information of the alternative NADH yielding reactions is required. Since substantial amounts of stromal NADH remain in pgdh3 mutants (Höhner et al., 2021), it will be important to pinpoint how much if any is contributed by PGDH1 activity in mesophyll chloroplasts (PGDH2 is not expressed). Since PGDH1 loss of function is lethal (Höhner et al., 2021) this requires a knock-down approach in the pgdh3 background. In parallel, it needs to be determined to what degree PDCp generates a reduced coenzyme surplus, i.e. NADH unused in diurnal fatty acid synthesis available to drive the malate valve. GAPCp1 and GAPC2 are reportedly not expressed in Arabidopsis leaves (Muñoz-Bertomeu et al., 2009). This should be validated in wild-type and pgdh mutants to ensure no compensatory upregulation of the enzymes take place if stromal NADH production routes are blocked.
Regulatory aspects could also be further explored for PGDH enzymes. For instance, all three PGDHs were shown to bind thioredoxin (TRX). However, only PGDH1 seemed to be directly regulated by TRX according to in vitro studies (Yoshida et al., 2020). Additionally, product or feedback inhibition by serine was shown for PGDH1 and PGDH3 isoforms from Arabidopsis (Benstein et al., 2013; Okamura and Hirai, 2017). Protein-protein interactions between PGDH proteins and unknown binding partners may exert another level of regulation. PGDH1 and PGDH3 display distinct localization in leaf plastids. Although a strong UBQ10 promoter from Arabidopsis was used in these studies, PGDH1 appeared soluble in the stroma whereas PGDH3 remained in small punctured structures in the chloroplasts of Arabidopsis and tobacco (Höhner et al., 2021). Endogenous promoters driving expression of PGDH fluorescence protein fusions and immunoblotting of plastid sub-organellar fractions should settle this discussion. Lastly, a moonlighting function for PGDH3 cannot be excluded at this point.
It will be critical to further exploit assays that allow activity monitoring of either MDH type simultaneously. This could be realized through employing genetically encoded sensors. A number of studies from Arabidopsis report significant progress toward this goal (Okamura and Hirai, 2017; Lim et al., 2020; Steinbeck et al., 2020). Here, NAD(H) and NADP(H)-specific sensors were targeted to the cytosol, stroma, and mitochondria. The data not only confirmed a light-dependent redox shunt from the stroma into the cytosol but also showed clear evidence for stromal NADH reduction in response to light (Lim et al., 2020; Steinbeck et al., 2020). In the future, these measurements need to be realized in a single reporter line that expresses NAD(H) and NADP(H) sensors in the same organelle. Introgressing these sensors into loss and gain-of-function mutants of interest will allow to determine the relative contribution of specific enzymes, transporters etc.
A first step into this direction was realized through the design of an illumination platform synchronized imaging setup (Elsässer et al., 2020). Elsässer and colleagues managed to trigger dark-light transitions similar to conditions expected in the field while almost simultaneously recording cytosolic NAD(H), stromal ATP, and stromal pH changes via a genetically encoded sensors in wild-type and NADP-mdh Arabidopsis mutants. The experiments on true leaves documented a strong light-dependent NADH reduction in the wild type but also NADP-mdh mutant cytosol. They also found that dark, i.e., resting, cytosolic NADH/NAD ratios were higher in NADP-mdh than in wild-type plants. The authors concluded that a significant export of reducing equivalents from illuminated chloroplasts persists in the absence of stromal NADP-MDH. Interestingly, carbon and reductant fluxes between the organelles remain high during the night, which was underappreciated thus far. Altogether, these findings may indicate a more important role of the stromal NAD-dependent MDH flux via the chloroplast malate valve and the reactions that deliver reduced NADH then previously acknowledged.
Finally, we propose that characterization of (stromal) NAD(P) dehydrogenases should generally determine Vmax, Km, Kcat etc. for both coenzymes, NAD(H) and NADP(H). When we started our research, we were surprised how little information was available in the newer literature. Over 30 years ago, a dual coenzyme specificity for NAD(H) and NADP(H) was shown for the chloroplast GapA/B isoforms. Other NAD(H)-dependent reactions or enzymes with dual coenzyme specificity may have been overlooked. Regardless if additional NAD(H) or dual coenzyme specific dehydrogenases will emerge, the data will greatly improve our perspective of the stromal redox poise.
Understanding the relative light-dependent activity of NADP(H) vs. NAD(H)-coupled reactions in the stroma and their contribution to malate export from the chloroplast will yield a better understanding of C3 photosynthesis and the constraints their flux has on photosynthetic efficiency. Once this has been achieved, engineering these pathways could improve plant yields in the field, for instance through avoidance of PSI acceptor side limitations and irreversible PSI damage. Even decades after their first description, the chloroplast malate valve and its two MDH enzymes remain therefore highly interesting. Thorough future research employing a combination of new and more traditional tools such as in vivo imaging of genetically encoded sensors and chlorophyll fluorescence spectroscopy of plants exposed to standard and dynamic growth conditions may yield insights to unlock the full potential of the malate valve and its linked pathways for biotechnological applications.
H-HK wrote the manuscript. MK edited text, references, and designed the figure. Both authors contributed to the article and approved the submitted version.
H-HK was funded by the 3rd call ERA-CAPS grant (NSF IOS-1847382) and a National Science Foundation Career Award (IOS-1553506).
The authors declare that the research was conducted in the absence of any commercial or financial relationships that could be construed as a potential conflict of interest.
All claims expressed in this article are solely those of the authors and do not necessarily represent those of their affiliated organizations, or those of the publisher, the editors and the reviewers. Any product that may be evaluated in this article, or claim that may be made by its manufacturer, is not guaranteed or endorsed by the publisher.
We apologize to the authors whose relevant contributions and original articles could not be cited or discussed due to space limitations or a lack of awareness regarding their existence. We thank Drs. Bettina Bölter, Serena Schwenkert (both LMU Munich), and Stephan Krüger (University of Cologne) for constructive feedback.
Alric, J., and Johnson, X. (2017). Alternative electron transport pathways in photosynthesis: a confluence of regulation. Curr. Opin. Plant Biol. 37, 78–86. doi: 10.1016/j.pbi.2017.03.014
Armbruster, U., Correa Galvis, V., Kunz, H. H., and Strand, D. D. (2017). The regulation of the chloroplast proton motive force plays a key role for photosynthesis in fluctuating light. Curr. Opin. Plant Biol. 37, 56–62. doi: 10.1016/j.pbi.2017.03.012
Baalmann, E., Backhausen, J. E., Rak, C., Vetter, S., and Scheibe, R. (1995). Reductive modification and nonreductive activation of purified spinach chloroplast NADP-dependent glyceraldehyde-3-phosphate dehydrogenase. Arch. Biochem. Biophys. 324, 201–208. doi: 10.1006/abbi.1995.0031
Backhausen, J. E., Vetter, S., Baalmann, E., Kitzmann, C., and Scheibe, R. (1998). NAD-dependent malate dehydrogenase and glyceraldehyde 3-phosphate dehydrogenase isoenzymes play an important role in dark metabolism of various plastid types. Planta 205, 359–366. doi: 10.1007/s004250050331
Beeler, S., Liu, H. C., Stadler, M., Schreier, T., Eicke, S., Lue, W. L., et al. (2014). Plastidial NAD-dependent malate dehydrogenase is critical for embryo development and heterotrophic metabolism in arabidopsis. Plant Physiol. 164, 1175–1190. doi: 10.1104/pp.113.233866
Benstein, R. M., Ludewig, K., Wulfert, S., Wittek, S., Gigolashvili, T., Frerigmann, H., et al. (2013). Arabidopsis phosphoglycerate dehydrogenase1 of the phosphoserine pathway is essential for development and required for ammonium assimilation and tryptophan biosynthesis. Plant Cell 25, 5011–5029. doi: 10.1105/tpc.113.118992
Berkemeyer, M., Scheibe, R., and Ocheretina, O. (1998). A novel, non-redox-regulated NAD-dependent malate dehydrogenase from chloroplasts of Arabidopsis thaliana L. J. Biol. Chem. 273, 27927–27933. doi: 10.1074/jbc.273.43.27927
Blankenship, R. E. (2010). Early evolution of photosynthesis. Plant Physiol. 154, 434–438. doi: 10.1104/pp.110.161687
Browse, J., Roughan, P. G., and Slack, C. R. (1981). Light control of fatty acid synthesis and diurnal fluctuations of fatty acid composition in leaves. Biochem. J. 196, 347–354. doi: 10.1042/bj1960347
Camp, P. J., and Randall, D. D. (1985). Purification and characterization of the pea chloroplast pyruvate dehydrogenase complex. Plant Physiol. 77, 571–577. doi: 10.1104/pp.77.3.571
Carr, P. D., Verger, D., Ashton, A. R., and Ollis, D. L. (1999). Chloroplast NADP-malate dehydrogenase: structural basis of light- dependent regulation of activity by thiol oxidation and reduction. Structure 7, 461–475. doi: 10.1016/S0969-2126(99)80058-6
Casatejada-Anchel, R., Muñoz-Bertomeu, J., Rosa-Téllez, S., Anoman, A. D., Nebauer, S. G., Torres-Moncho, A., et al. (2021). Phosphoglycerate dehydrogenase genes differentially affect Arabidopsis metabolism and development. Plant Sci. 306:110863. doi: 10.1016/j.plantsci.2021.110863
Chaux, F., Peltier, G., and Johnson, X. (2015). A security network in PSI photoprotection: regulation of photosynthetic control, NPQ and O2 photoreduction by cyclic electron flow. Front. Plant Sci. 6, 1–7. doi: 10.3389/fpls.2015.00875
Correll, C. C., Ludwig, M. L., Bruns, C. M., and Karplus, P. A. (1993). Structural prototypes for an extended family of flavoprotein reductases: comparison of phthalate dioxygenase reductase with ferredoxin reductase and ferredoxin. Protein Sci. 2, 2112–2133. doi: 10.1002/pro.5560021212
Dietz, K. J., Turkan, I., and Krieger-Liszkay, A. (2016). Redox- and reactive oxygen species-dependent signaling into and out of the photosynthesizing chloroplast. Plant Physiol. 171, 1541–1550. doi: 10.1104/pp.16.00375
Elsässer, M., Feitosa-Araujo, E., Lichtenauer, S., Wagner, S., Fuchs, P., Giese, J., et al. (2020). Photosynthetic activity triggers pH and NAD redox signatures across different plant cell compartments. bioRxiv [Preprint]. doi: 10.1101/2020.10.31.363051
Flugge, U.-I., Hausler, R. E., Ludewig, F., and Gierth, M. (2011). The role of transporters in supplying energy to plant plastids. J. Exp. Bot. 62, 2381–2392. doi: 10.1093/jxb/erq361
Foyer, C. H., Neukermans, J., Queval, G., Noctor, G., and Harbinson, J. (2012). Photosynthetic control of electron transport and the regulation of gene expression. J. Exp. Bot. 63, 1637–1661. doi: 10.1093/jxb/ers013
Geigenberger, P., and Fernie, A. R. (2014). Metabolic control of redox and redox control of metabolism in plants. Antioxid. Redox Signal. 21, 1389–1421. doi: 10.1089/ars.2014.6018
Grieco, M., Tikkanen, M., Paakkarinen, V., Kangasjärvi, S., and Aro, E. M. (2012). Steady-state phosphorylation of light-harvesting complex II proteins preserves photosystem I under fluctuating white light. Plant Physiol. 160, 1896–1910. doi: 10.1104/pp.112.206466
Gururani, M. A., Venkatesh, J., and Tran, L. S. P. (2015). Regulation of photosynthesis during abiotic stress-induced photoinhibition. Mol. Plant 8, 1304–1320. doi: 10.1016/j.molp.2015.05.005
Hebbelmann, I., Selinski, J., Wehmeyer, C., Goss, T., Voss, I., Mulo, P., et al. (2012). Multiple strategies to prevent oxidative stress in Arabidopsis plants lacking the malate valve enzyme NADP-malate dehydrogenase. J. Exp. Bot. 63, 1445–1459. doi: 10.1093/jxb/err386
Hermoso, J. A., Mayoral, T., Faro, M., Gómez-Moreno, C., Sanz-Aparicio, J., and Medina, M. (2002). Mechanism of coenzyme recognition and binding revealed by crystal structure analysis of ferredoxin-NADP+ reductase complexed with NADP+. J. Mol. Biol. 319, 1133–1142. doi: 10.1016/S0022-2836(02)00388-1
Heyno, E., Innocenti, G., Lemaire, S. D., Issakidis-Bourguet, E., and Krieger-Liszkay, A. (2014). Putative role of the malate valve enzyme NADP-malate dehydrogenase in H2O2 signalling in Arabidopsis. Philos. Trans. R. Soc. B Biol. Sci. 369:20130228. doi: 10.1098/rstb.2013.0228
Höhner, R., Day, P. M., Zimmermann, S. E., Lopez, L. S., Krämer, M., Giavalisco, P., et al. (2021). Stromal NADH supplied by PHOSPHOGLYCERATE DEHYDROGENASE3 is crucial for photosynthetic performance. Plant Physiol. 186, 1–26. doi: 10.1093/plphys/kiaa117
Höhner, R., Pribil, M., Herbstová, M., Lopez, L. S., Kunz, H. H., Li, M., et al. (2020). Plastocyanin is the long-range electron carrier between photosystem II and photosystem I in plants. Proc. Natl. Acad. Sci. U. S. A. 117, 15354–15362. doi: 10.1073/pnas.2005832117
Jackson, J. B. (2012). A review of the binding-change mechanism for proton-translocating transhydrogenase. Biochim. Biophys. Acta Bioenerg. 1817, 1839–1846. doi: 10.1016/j.bbabio.2012.04.006
Jahns, P., and Holzwarth, A. R. (2012). The role of the xanthophyll cycle and of lutein in photoprotection of photosystem II. Biochim. Biophys. Acta Bioenerg. 1817, 182–193. doi: 10.1016/j.bbabio.2011.04.012
Kaiser, E., Walther, D., and Armbruster, U. (2020). Growth under fluctuating light reveals large trait variation in a panel of Arabidopsis accessions. Plants 9:316. doi: 10.3390/plants9030316
Kanazawa, A., Ostendorf, E., Kohzuma, K., Hoh, D., Strand, D. D., Sato-Cruz, M., et al. (2017). Chloroplast ATP synthase modulation of the thylakoid proton motive force: implications for photosystem I and photosystem II photoprotection. Front. Plant Sci. 8, 1–12. doi: 10.3389/fpls.2017.00719
Kikuchi, S., Asakura, Y., Imai, M., Nakahira, Y., Kotani, Y., Hashiguchi, Y., et al. (2018). A Ycf2-FtsHi heteromeric AAA-ATPase complex is required for chloroplast protein import. Plant Cell 30, 2677–2703. doi: 10.1105/tpc.18.00357
Kinoshita, H., Nagasaki, J., Yoshikawa, N., Yamamoto, A., Takito, S., Kawasaki, M., et al. (2011). The chloroplastic 2-oxoglutarate/malate transporter has dual function as the malate valve and in carbon/nitrogen metabolism. Plant J. 65, 15–26. doi: 10.1111/j.1365-313X.2010.04397.x
Kleine, T., Nägele, T., Neuhaus, H. E., Schmitz-Linneweber, C., Fernie, A. R., Geigenberger, P., et al. (2021). Acclimation in plants – the Green Hub consortium. Plant J. 106, 23–40. doi: 10.1111/tpj.15144
Koskela, M. M., Brünje, A., Ivanauskaite, A., Grabsztunowicz, M., Lassowskat, I., Neumann, U., et al. (2018). Chloroplast acetyltransferase NSI is required for state transitions in Arabidopsis thaliana. Plant Cell 30, 1695–1709. doi: 10.1105/tpc.18.00155
Koskela, M. M., Brünje, A., Ivanauskaite, A., Lopez, L. S., Schneider, D., DeTar, R. A., et al. (2020). Comparative analysis of thylakoid protein complexes in state transition mutants nsi and stn7: focus on PSI and LHCII. Photosynth. Res. 145:15. doi: 10.1007/s11120-020-00711-4
Kramer, D. M., Cruz, J. A., and Kanazawa, A. (2003). Balancing the central roles of the thylakoid proton gradient. Trends Plant Sci. 8, 27–32. doi: 10.1016/S1360-1385(02)00010-9
Kramer, D. M., and Evans, J. R. (2011). The importance of energy balance in improving photosynthetic productivity. Plant Physiol. 155, 70–78. doi: 10.1104/pp.110.166652
Krause, G., and Heber, U. (1976). “Energetics of intact chloroplasts,” in The Intact Chloroplast, ed J. Barber (Amsterdam: Elsevier), 171–214.
Kruger, N., and von Schaewen, A. (2003). The oxidative pentose phosphate pathway: structure and organisation. Curr. Opin. Plant Biol. 6, 236–246. doi: 10.1016/S1369-5266(03)00039-6
Larsson, C., and Albertsson, E. (1979). Enzymes related to serine synthesis in spinach chloroplasts. Physiol. Plant. 45, 7–10. doi: 10.1111/j.1399-3054.1979.tb01654.x
Laughlin, T. G., Bayne, A. N., Trempe, J. F., Savage, D. F., and Davies, K. M. (2019). Structure of the complex I-like molecule NDH of oxygenic photosynthesis. Nature 566, 411–414. doi: 10.1038/s41586-019-0921-0
Lim, S. L., Voon, C. P., Guan, X., Yang, Y., Gardeström, P., and Lim, B. L. (2020). In planta study of photosynthesis and photorespiration using NADPH and NADH/NAD+ fluorescent protein sensors. Nat. Commun. 11:3238. doi: 10.1038/s41467-020-17056-0
Liszka, A., Schimpf, R., Zaruma, K. I. C., Buhr, A., Seidel, T., Walter, S., et al. (2020). Three cytosolic NAD-malate dehydrogenase isoforms of Arabidopsis thaliana: on the crossroad between energy fluxes and redox signaling. Biochem. J. 477:3673. doi: 10.1042/BCJ20200240
Maloof, J. N., Borevitz, J. O., Dabi, T., Lutes, J., Nehring, R. B., Redfern, J. L., et al. (2001). Natural variation in light sensitivity of Arabidopsis. Nat. Genet. 29, 441–446. doi: 10.1038/ng777
McEvoy, J. P., and Brudvig, G. W. (2006). Water-splitting chemistry of photosystem II. Chem. Rev. 106, 4455–4483. doi: 10.1021/cr0204294
Medina, M., Luquita, A., Tejero, J., Hermoso, J., Mayoral, T., Sanz-Aparicio, J., et al. (2001). Probing the determinants of coenzyme specificity in ferredoxin-NADP + reductase by site-directed mutagenesis. J. Biol. Chem. 276, 11902–11912. doi: 10.1074/jbc.M009287200
Minagawa, J. (2011). State transitions-the molecular remodeling of photosynthetic supercomplexes that controls energy flow in the chloroplast. Biochim. Biophys. Acta Bioenerg. 1807, 897–905. doi: 10.1016/j.bbabio.2010.11.005
Miyake, C. (2020). Molecular mechanism of oxidation of P700 and suppression of ROS production in photosystem I in response to electron-sink limitations in C3 plants. Antioxidants 9:230. doi: 10.3390/antiox9030230
Mulo, P. (2011). Chloroplast-targeted ferredoxin-NADP+ oxidoreductase (FNR): structure, function and location. Biochim. Biophys. Acta Bioenerg. 1807, 927–934. doi: 10.1016/j.bbabio.2010.10.001
Munekage, Y., Hojo, M., Meurer, J., Endo, T., Tasaka, M., and Shikanai, T. (2002). PGR5 is involved in cyclic electron flow around photosystem I and is essential for photoprotection in Arabidopsis. Cell 110, 361–371. doi: 10.1016/S0092-8674(02)00867-X
Muñoz-Bertomeu, J., Cascales-Miñana, B., Mulet, J. M., Baroja-Fernández, E., Pozueta-Romero, J., Kuhn, J. M., et al. (2009). Plastidial glyceraldehyde-3-phosphate dehydrogenase deficiency leads to altered root development and affects the sugar and amino acid balance in Arabidopsis. Plant Physiol. 151, 541–558. doi: 10.1104/pp.109.143701
Neuhaus, H. E., Batz, O., Thom, E., and Scheibe, R. (1993). Purification of highly intact plastids from various heterotrophic plant tissues: analysis of enzymic equipment and precursor dependency for starch biosynthesis. Biochem. J. 296, 395–401. doi: 10.1042/bj2960395
Niedermaier, S., Schneider, T., Bahl, M. O., Matsubara, S., and Huesgen, P. F. (2020). Photoprotective acclimation of the Arabidopsis thaliana leaf proteome to fluctuating light. Front. Genet. 11:154. doi: 10.3389/fgene.2020.00154
Okamura, E., and Hirai, M. Y. (2017). Novel regulatory mechanism of serine biosynthesis associated with 3-phosphoglycerate dehydrogenase in Arabidopsis thaliana. Sci. Rep. 7, 1–7. doi: 10.1038/s41598-017-03807-5
Okegawa, Y., and Motohashi, K. (2015). Chloroplastic thioredoxin m functions as a major regulator of Calvin cycle enzymes during photosynthesis in vivo. Plant J. 84, 900–913. doi: 10.1111/tpj.13049
Pesaresi, P., Pribil, M., Wunder, T., and Leister, D. (2011). Dynamics of reversible protein phosphorylation in thylakoids of flowering plants: the roles of STN7, STN8 and TAP38. Biochim. Biophys. Acta Bioenerg. 1807, 887–896. doi: 10.1016/j.bbabio.2010.08.002
Piubelli, L., Aliverti, A., Arakaki, A. K., Carrillo, N., Ceccarelli, E. A., Andrew Karplus, P., et al. (2000). Competition between C-terminal tyrosine and nicotinamide modulates pyridine nucleotide affinity and specificity in plant ferredoxin-NADP+ reductase. J. Biol. Chem. 275, 10472–10476. doi: 10.1074/jbc.275.14.10472
Popp, J., Lakner, Z., Harangi-Rákos, M., and Fári, M. (2014). The effect of bioenergy expansion: food, energy, and environment. Renew. Sustain. Energy Rev. 32, 559–578. doi: 10.1016/j.rser.2014.01.056
Pracharoenwattana, I., Cornah, J. E., and Smith, S. M. (2007). Arabidopsis peroxisomal malate dehydrogenase functions in β-oxidation but not in the glyoxylate cycle. Plant J. 50, 381–390. doi: 10.1111/j.1365-313X.2007.03055.x
Rantala, S., Lempiäinen, T., Gerotto, C., Tiwari, A., Aro, E. M., and Tikkanen, M. (2020). PGR5 and NDH-1 systems do not function as protective electron acceptors but mitigate the consequences of PSI inhibition. Biochim. Biophys. Acta Bioenerg. 1861:148154. doi: 10.1016/j.bbabio.2020.148154
Ros, R., Muñoz-Bertomeu, J., and Krueger, S. (2014). Serine in plants: bio- synthesis, metabolism, and functions. Trends Plant Sci. 19:564. doi: 10.1016/j.tplants.2014.06.003
Rumeau, D., Bécuwe-Linka, N., Beyly, A., Louwagie, M., Garin, J., and Peltier, G. (2005). New subunits NDH-M, -N, and -O, encoded by nuclear genes, are essential for plastid Ndh complex functioning in higher plants. Plant Cell 17:219. doi: 10.1105/tpc.104.028282
Sánchez-Baracaldo, P., and Cardona, T. (2020). On the origin of oxygenic photosynthesis and cyanobacteria. New Phytol. 225, 1440–1446. doi: 10.1111/nph.16249
Sazanov, L. A., Burrows, P. A., and Nixon, P. J. (1998). The plastid ndh genes code for an NADH-specific dehydrogenase: isolation of a complex I analogue from pea thylakoid membranes. Proc. Natl. Acad. Sci. U. S. A. 95, 1319–1324. doi: 10.1073/pnas.95.3.1319
Scheibe, R. (1987). NADP+-malate dehydrogenase in C3-plants: regulation and role of a light-activated enzyme. Physiol. Plant. 71, 393–400. doi: 10.1111/j.1399-3054.1987.tb04362.x
Scheibe, R. (2004). Malate valves to balance cellular energy supply. Physiol. Plant. 120, 21–26. doi: 10.1111/j.0031-9317.2004.0222.x
Scheibe, R., and Stitt, M. (1988). Comparison of NADP-malate dehydrogenase activation, QA reduction and O2 evolution in spinach leaves. Plant Physiol. Biochem. 26, 473–481.
Schirrmeister, B. E., De Vos, J. M., Antonelli, A., and Bagheri, H. C. (2013). Evolution of multicellularity coincided with increased diversification of cyanobacteria and the great oxidation event. Proc. Natl. Acad. Sci. U. S. A. 110, 1791–1796. doi: 10.1073/pnas.1209927110
Schneider, M., Knuesting, J., Birkholz, O., Heinisch, J. J., and Scheibe, R. (2018). Cytosolic GAPDH as a redox-dependent regulator of energy metabolism. BMC Plant Biol. 18:184. doi: 10.1186/s12870-018-1390-6
Schneider, T., Bolger, A., Zeier, J., Preiskowski, S., Benes, V., Trenkamp, S., et al. (2019). Fluctuating light interacts with time of day and leaf development stage to reprogram gene expression. Plant Physiol. 179, 1632–1657. doi: 10.1104/pp.18.01443
Schreier, T. B., Cléry, A., Schläfli, M., Galbier, F., Stadler, M., Demarsy, E., et al. (2018). Plastidial NAD-dependent malate dehydrogenase: a moonlighting protein involved in early chloroplast development through its interaction with an ftsh12-ftshi protease complex. Plant Cell 30, 1745–1769. doi: 10.1105/tpc.18.00121
Schuller, J. M., Birrell, J. A., Tanaka, H., Konuma, T., Wulfhorst, H., Cox, N., et al. (2019). Structural adaptations of photosynthetic complex I enable ferredoxin-dependent electron transfer. Science (80-.) 363, 257–260. doi: 10.1126/science.aau3613
Selinski, J., König, N., Wellmeyer, B., Hanke, G. T., Linke, V., Ekkehard Neuhaus, H., et al. (2014). The plastid-localized NAD-dependent malate dehydrogenase is crucial for energy homeostasis in developing Arabidopsis thaliana seeds. Mol. Plant 7, 170–186. doi: 10.1093/mp/sst151
Selinski, J., and Scheibe, R. (2014). Lack of malate valve capacities lead to improved n-assimilation and growth in transgenic A. Thaliana plants. Plant Signal. Behav. 9:e29057. doi: 10.4161/psb.29057
Selinski, J., and Scheibe, R. (2019). Malate valves: old shuttles with new perspectives. Plant Biol. 21, 21–30. doi: 10.1111/plb.12869
Sharkey, T. D., and Weise, S. E. (2015). The glucose 6-phosphate shunt around the Calvin-Benson cycle. J. Exp. Bot. 67, 4067–4077. doi: 10.1093/jxb/erv484
Shikanai, T., and Yamamoto, H. (2017). Contribution of cyclic and pseudo-cyclic electron transport to the formation of proton motive force in chloroplasts. Mol. Plant 10, 20–29. doi: 10.1016/j.molp.2016.08.004
Shimakawa, G., and Miyake, C. (2018). Oxidation of P700 ensures robust photosynthesis. Front. Plant Sci. 871, 1–15. doi: 10.3389/fpls.2018.01617
Sparla, F., Pupillo, P., and Trost, P. (2002). The C-terminal extension of glyceraldehyde-3-phosphate dehydrogenase subunit B acts as an autoinhibitory domain regulated by thioredoxins and nicotinamide adenine dinucleotide*. J. Biol. Chem. 277, 44946–44952. doi: 10.1074/jbc.M206873200
Steinbeck, J., Fuchs, P., Negroni, Y. L., Elsässer, M., Lichtenauer, S., Stockdreher, Y., et al. (2020). In vivo nadh/nad1 biosensing reveals the dynamics of cytosolic redox metabolism in plants. Plant Cell 32, 3324–3345. doi: 10.1105/tpc.20.00241
Strand, D. D., Fisher, N., Davis, G. A., and Kramer, D. M. (2016). Redox regulation of the antimycin A sensitive pathway of cyclic electron flow around photosystem I in higher plant thylakoids. Biochim. Biophys. Acta Bioenerg. 1857, 1–6. doi: 10.1016/j.bbabio.2015.07.012
Strand, D. D., Fisher, N., and Kramer, D. M. (2017). The higher plant plastid NAD(P)H dehydrogenase-like complex (NDH) is a high efficiency proton pump that increases ATP production by cyclic electron flow. J. Biol. Chem. 292, 11850. doi: 10.1074/jbc.M116.770792
Thormählen, I., Zupok, A., Rescher, J., Leger, J., Weissenberger, S., Groysman, J., et al. (2017). Thioredoxins play a crucial role in dynamic acclimation of photosynthesis in fluctuating light. Mol. Plant 10, 168–182. doi: 10.1016/j.molp.2016.11.012
Tomaz, T., Bagard, M., Pracharoenwattana, I., Lindén, P., Lee, C. P., Carroll, A. J., et al. (2010). Mitochondrial malate dehydrogenase lowers leaf respiration and alters photorespiration and plant growth in Arabidopsis. Plant Physiol. 154, 1143–1157. doi: 10.1104/pp.110.161612
Toujani, W., Muñoz-Bertomeu, J., Flores-Tornero, M., Rosa-Téllez, S., Anoman, A. D., Alseekh, S., et al. (2013). Functional characterization of the plastidial 3-phosphoglycerate dehydrogenase family in Arabidopsis. Plant Physiol. 163, 1164–1178. doi: 10.1104/pp.113.226720
Turner, W. L., Waller, J. C., Vanderbeld, B., and Snedden, W. A. (2004). Cloning and characterization of two NAD kinases from arabidopsis. Identification of a calmodulin binding isoform. Plant Physiol. 135, 1243–1255. doi: 10.1104/pp.104.040428
Van Rooijen, R., Kruijer, W., Boesten, R., Van Eeuwijk, F. A., Harbinson, J., and Aarts, M. G. M. (2017). Natural variation of YELLOW SEEDLING1 affects photosynthetic acclimation of Arabidopsis thaliana. Nat. Commun. 8:1421. doi: 10.1038/s41467-017-01576-3
Yamamoto, H., and Shikanai, T. (2020). Does the Arabidopsis proton gradient regulation5 mutant leak protons from the thylakoid membrane?1. Plant Physiol. 184, 421–427. doi: 10.1104/pp.20.00850
Yokochi, Y., Yoshida, K., Hahn, F., Miyagi, A., Wakabayashi, K. I., Kawai-Yamada, M., et al. (2021). Redox regulation of NADP-malate dehydrogenase is vital for land plants under fluctuating light environment. Proc. Natl. Acad. Sci. U. S. A. 118, 1–12. doi: 10.1073/pnas.2016903118
Yoshida, K., Ohtaka, K., Hirai, M. Y., and Hisabori, T. (2020). Biochemical insight into redox regulation of plastidial 3-phosphoglycerate dehydrogenase from arabidopsis thaliana. J. Biol. Chem. 295, 14906–14915. doi: 10.1074/jbc.RA120.014263
Zhao, Y., Luo, L., Xu, J., Xin, P., Guo, H., Wu, J., et al. (2018). Malate transported from chloroplast to mitochondrion triggers production of ROS and PCD in Arabidopsis thaliana. Cell Res. 28, 448–461. doi: 10.1038/s41422-018-0024-8
Keywords: C3 photosynthesis, redox, chloroplast, coenzymes, acclimation
Citation: Krämer M and Kunz H-H (2021) Indirect Export of Reducing Equivalents From the Chloroplast to Resupply NADP for C3 Photosynthesis—Growing Importance for Stromal NAD(H)? Front. Plant Sci. 12:719003. doi: 10.3389/fpls.2021.719003
Received: 01 June 2021; Accepted: 23 September 2021;
Published: 20 October 2021.
Edited by:
Corina M. Fusari, Centro de Estudios Fotosintéticos y Bioquímicos (CEFOBI), ArgentinaReviewed by:
Kaori Kohzuma, Tohoku University, JapanCopyright © 2021 Krämer and Kunz. This is an open-access article distributed under the terms of the Creative Commons Attribution License (CC BY). The use, distribution or reproduction in other forums is permitted, provided the original author(s) and the copyright owner(s) are credited and that the original publication in this journal is cited, in accordance with accepted academic practice. No use, distribution or reproduction is permitted which does not comply with these terms.
*Correspondence: Hans-Henning Kunz, a3VuekBsbXUuZGU=
Disclaimer: All claims expressed in this article are solely those of the authors and do not necessarily represent those of their affiliated organizations, or those of the publisher, the editors and the reviewers. Any product that may be evaluated in this article or claim that may be made by its manufacturer is not guaranteed or endorsed by the publisher.
Research integrity at Frontiers
Learn more about the work of our research integrity team to safeguard the quality of each article we publish.