- 1Biotechnology Research Institute, Chinese Academy of Agricultural Sciences, Beijing, China
- 2College of Life Science, Shanxi University, Taiyuan, China
- 3Institute for Agricultural Biosciences, Oklahoma State University, Ardmore, OK, United States
- 4Department of Plant and Soil Sciences, Oklahoma State University, Stillwater, OK, United States
Plant height is an important agronomic trait that is closely related to biomass yield and crop production. Despite legumes comprise one of the largest monophyletic families that are second only to grasses in terms of economic and nutritional values, due to an ancient genome duplication event, most legume plants have complex genomes, thus the molecular mechanisms that determine plant height are less known in legumes. Here, we report the identification and characterization of MAIN STEM DWARF1 (MSD1), which is required for the plant height in the model legume Medicago truncatula. Loss of function of MSD1 leads to severely reduced main stem height but normal lateral branch elongation in M. truncatula. Histological analysis revealed that the msd1-1 main stem has shorter internodes with reduced cell size and number compared with the wild type, indicating that MSD1 affects cell elongation and cell proliferation. MSD1 encodes a putative GA 20-oxidase that is expressed at significantly higher levels in the main shoot apex than in the lateral shoot apices, suggesting that MSD1 expression is associated with its effect on the main stem elongation. UPLC-MS/MS analysis showed that GA9 and GA4, two identified products of the GA 20-oxidase, were severely reduced in msd1-1, and the dwarf phenotype of msd1-1 could be rescued by supplementation with gibberellic acid GA3, confirming that MSD1 functions as a biologically active GA 20-oxidase. Moreover, we found that disruption of either MtGA20ox7 or MtGA20ox8, homologs of MSD1, has little effects on the elongation of the main stem, while the msd1-1 mtga20ox7-1 mtga20ox8 triple mutants exhibits a severe short main shoot and lateral branches, as well as reduced leaf size, suggesting that MSD1 and its homologs MtGA20ox7 and MtGA20ox8, redundantly regulate M. truncatula shoot elongation and leaf development. Taken together, our findings demonstrate the molecular mechanism of MSD1-mediated regulation of main stem elongation in M. truncatula and provide insights into understanding the functional diversity of GA 20-oxidases in optimizing plant architecture in legumes.
Introduction
Plant height, mainly confined by stem elongation, is not only a decisive factor that affects plant architecture but also an important agronomic trait that contributes to crop yield (Wang and Li, 2008). Gibberellins (GAs) are a large family of tetracyclic diterpenoid plant hormones that play important roles in multiple plant growth and developmental progresses, including promoting seed germination, stem elongation, flowering, pollen development, as well as fruit growth and firmness (Hedden and Sponsel, 2015; Li et al., 2020). The stem elongation function of GA contributed to the Green Revolution in which mutations in GA biosynthesis or signaling are the basis for semidwarf rice and wheat, respectively (Peng et al., 1999; Sasaki et al., 2002).
The biosynthesis of GAs is a multi-step process divided into three stages (Yamaguchi, 2008; Hedden and Thomas, 2012). In the first stage, biosynthesis of ent-kaurene is restricted to plastids and catalyzed successively by ent-copalyl diphosphate synthase (CPS) and ent-kaurene synthase (KS). In the second stage, ent-kaurene is converted to GA12 by ent-kaurene oxidase (KO) and ent-kaurenoic acid oxidase (KAO), both of which are cytochrome P450 enzymes (Magome et al., 2013; Nomura et al., 2013; Regnault et al., 2014). In the final stage, GA12 is a substrate for cytoplasm-located gibberellin 20-oxidase (GA20ox) multi-family enzymes and follows a non-13-hydroxylation pathway leading to GA9 via GA15 and GA24, and then GA9 is converted to bioactive GA4 and GA7 by GA 3-hydroxylase (GA3ox) (Lange et al., 1994; Chiang et al., 1995; Xu et al., 1995b; Israelsson et al., 2004).
Impaired GA biosynthesis caused by defects in early-step genes, CPS, KS, and KO, leads to typical GA-deficient mutant phenotypes, including severe dwarfism with greatly impaired fertility (Sun and Kamiya, 1994; Helliwell et al., 1998; Ueguchi-Tanaka et al., 2007; Yamaguchi, 2008; Chen et al., 2014; Regnault et al., 2014; Guo et al., 2020). By contrast, mutation of late-step genes of GA biosynthesis leads to semi-dwarf phenotypes because of the functional redundancy of homologous genes, such as genes coding for GA3ox in Arabidopsis, rice (Oryza sativa), Medicago truncatula and alfalfa (Medicago sativa) (Itoh et al., 2001; Dalmadi et al., 2008; Hu et al., 2008; Zhang et al., 2020). Notably, the semi-dwarf “green revolution” phenotype in rice resulted from a mutation in the sd1 gene, which encodes a GA 20-oxidase (OsGA20ox2) (Sasaki et al., 2002; Spielmeyer et al., 2002). Overexpression of OsGA20ox1 causes a tall and GA-overproduction phenotype; RNAi-mediated suppression of OsGA20ox1 results in phenotypes that are similar to those of sd1, indicating that these two genes probably have complementary function in GA synthesis (Oikawa et al., 2004). In Arabidopsis, GA20ox1 and GA20ox2 act partially redundantly to promote plant growth (Rieu et al., 2008). These studies indicate that genes in the GA20ox family are of great value for optimizing plant architecture in agricultural species.
Legumes are second only to grasses in terms of economic and nutritional values, and are the major sources of plant proteins and oils for humans and animals (Graham and Vance, 2003). Studies on several dwarf mutants in pea (Pisum sativum), soybean (Glycine max), and the model legume M. truncatula have suggested that the GA pathway plays a conserved role in controlling plant height in legumes (Yaxley et al., 2001; Li et al., 2018; Guo et al., 2020; Zhang et al., 2020). Notably, despite the M. truncatula GA20ox family genes exhibited functional redundancy (Ma et al., 2019), overexpression of GA20ox can increase M. truncatula plant height and biomass (Wang et al., 2020), suggesting an application potential of the GA20ox family genes for biomass improvement in legumes. Nevertheless, due to the ancient genome duplication event, most legume plants undergo gene duplication and subsequent functional diversification (Shoemaker et al., 2006), thus the biological functions of the GA20ox family genes in legumes remain largely unclear.
In this study, we reported the identification and characterization of a distinct dwarf mutant, main stem dwarf1-1 (msd1-1), in M. truncatula, which is defective in the main stem elongation. MSD1 encodes a putative GA 20-oxidase, catalyzing the late step of GA biosynthesis. Our results demonstrated that MSD1 specifically controls the main stem elongation in M. truncatula, while MSD1’s homologs genes MtGA20ox7 or MtGA20ox8 show little effects on the elongation of the main stem. However, the msd1-1 mtga20ox7-1 mtga20ox8 triple mutant exhibits a severely short main shoot and lateral branches, as well as reduced leaf size, suggesting that MSD1 and its homologs MtGA20ox7 and MtGA20ox8 redundantly regulate M. truncatula shoot elongation and leaf development.
Materials and Methods
Plant Materials and Growth Conditions
Medicago truncatula strain R108 was used as the wild type for all experiments described in this study. msd1-1 (NF5514), msd1-2 (NF12848), msd1-3 (NF10524), msd1-4 (NF21287), mtga20ox7-1 (NF1343), mtga20ox7-2 (NF18196), and mtga20ox8 (NF19184) were identified from a Tnt1 retrotransposon-tagged mutant collection of M. truncatula R108 (Tadege et al., 2008). The M. truncatula seeds were scarified with sandpaper and germinated at 4°C for 1 week, then the germinated seeds were planted and grown in soil mix (soil:vermiculite = 1:1) in the greenhouse under the following conditions: 24°C day/22°C night temperature, 16-h day/8-h night photoperiod, and 60–70% relative humidity.
The msd1-1 mtga20ox7-1, msd1-1 mtga20ox8, mtga20ox7-1 mtga20ox8 double mutants, and the msd1-1 mtga20ox7-1 mtga20ox8 triple mutants were generated through genetic crosses and identified on the basis of PCR genotyping in the F2 or F3 segregating population. The primers used to identify the Tnt1 insertions are listed in Supplementary Table 1.
Morphological Analysis
For the measurement of the internode length, 20 individual plants of both wild type and msd1-1 mutant were grown simultaneously in the same greenhouse, and the seventh internode beneath the shoot apex of each plant (8-week-old plants) was measured to calculate the average length. Six internodes of each genotype were randomly selected from the above 20 samples, fresh M. truncatula internodes from wild type and mutant plants were fixed in 3.5% (v/v) glutaraldehyde in 25 mM phosphate buffer (pH 7) for 48 h, followed by 1% (w/v) osmium tetroxide in 25 mM phosphate buffer (pH 7) for 2 h, and dehydrated in a graded ethanol series (50, 70, 90, 95, 100%), critical-point dried in liquid CO2, mounted on aluminum stubs and sputter coated with gold. The internodes were observed using scanning electron microscope by SU8010 (Hitachi, Japan).
In order to measure the length of internode epidermal cells, 15 cells were randomly selected from the SEM images for both wild type and msd1-1, and the lengths were measured by using Image J. The cell number was calculated by the ratio of the average internode length (calculated from a total of 20 internodes) to the average cell length (evaluated from 15 cells). Projected areas of leaves were measured by scanning to generate digital images, followed by analysis using the Image J software.
Phylogenetic Analysis and Sequences Alignment
Multiple sequences alignment was performed using ClustalW.1 Bootstrap values of 1000 permutations for the neighbor-joining phylogenetic tree were performed using MEGA 7.0 software.2 Accession numbers used in this study are listed in Supplementary Table 2.
Quantification of Endogenous GAs
Wild type and msd1-1 plants were grown in soil for 5 weeks in the greenhouse. The main stems (∼0.3 g) from 10 plants of each genotype were collected and mixed together for GA quantification. The GA contents were determined by the Wuhan Greensword Creation Technology Company, and the analysis was performed as described previously (Chen et al., 2012). Three independent biological replicates and technical replicates were measured for each sample.
Exogenous GA3 Application
Bioactive GA3 (SIGMA, Lot: BCBR3974V) was dissolved in ethanol (0.1 M) and diluted with ddH2O to 2 mM. The first spray was applied at 7-day-old seedlings after sowing, and the later sprays were performed twice a week for four weeks in total. An equivalent group (n = 16) of msd1-1 mutant plants was treated similarly with a solution without GA3 (MOCK) at each time. All msd1-1 mutants with the treatments (+GA3 and MOCK) were grown simultaneously in the same greenhouse. Experiments were repeated twice independently with similar results.
RNA Extraction and Gene Expression Analysis
Total RNA was extracted from plant tissues using TRIzol Reagent (Invitrogen). cDNA was synthesized by reverse transcription with SuperScript (Invitrogen). Reverse transcription PCR (RT-PCR) was performed using a 2xTaq PCR Master Mix (UPTECH) using MtActin as a control. Quantitative RT-PCR was performed as previously described (Wang et al., 2017) with at least three biological and three technical replicates for both the treatment samples and controls. All primers used are listed in Supplementary Table 1.
Plasmid Construction and Plant Transformation
To generate the constructs used for complementation, an 1134-bp of full-length MSD1 coding sequence was amplified from M. truncatula R108 plant and ligated to the pEarlyGate203 vector to generate p35S:MSD1 construct. The construct were introduced into Agrobacterium tumefaciens by chemical transformation. A. tumefaciens strain AGL1 was used for M. truncatula transformation (Tadege et al., 2011). All primers used are listed in Supplementary Table 1.
Results
Identification of the M. truncatula msd1 Mutant
To investigate the molecular mechanism underlying the regulation of plant height in the model legume M. truncatula, we identified a distinct dwarf mutant named main stem dwarf1-1 (msd1-1) from a forward genetic screen of the Tnt1 retrotransposon-tagged M. truncatula mutant population (Yarce et al., 2013). By contrast with the wild type, the msd1-1 mutant exhibits dwarfed main stem, while side branches were normal (Figures 1A–C,E–G,I). There were no difference in internode number between the msd1-1 mutant and the wild type, but the length of every internode in the msd1-1 was significant shorter than that in wild type (Figures 1D,H,J).
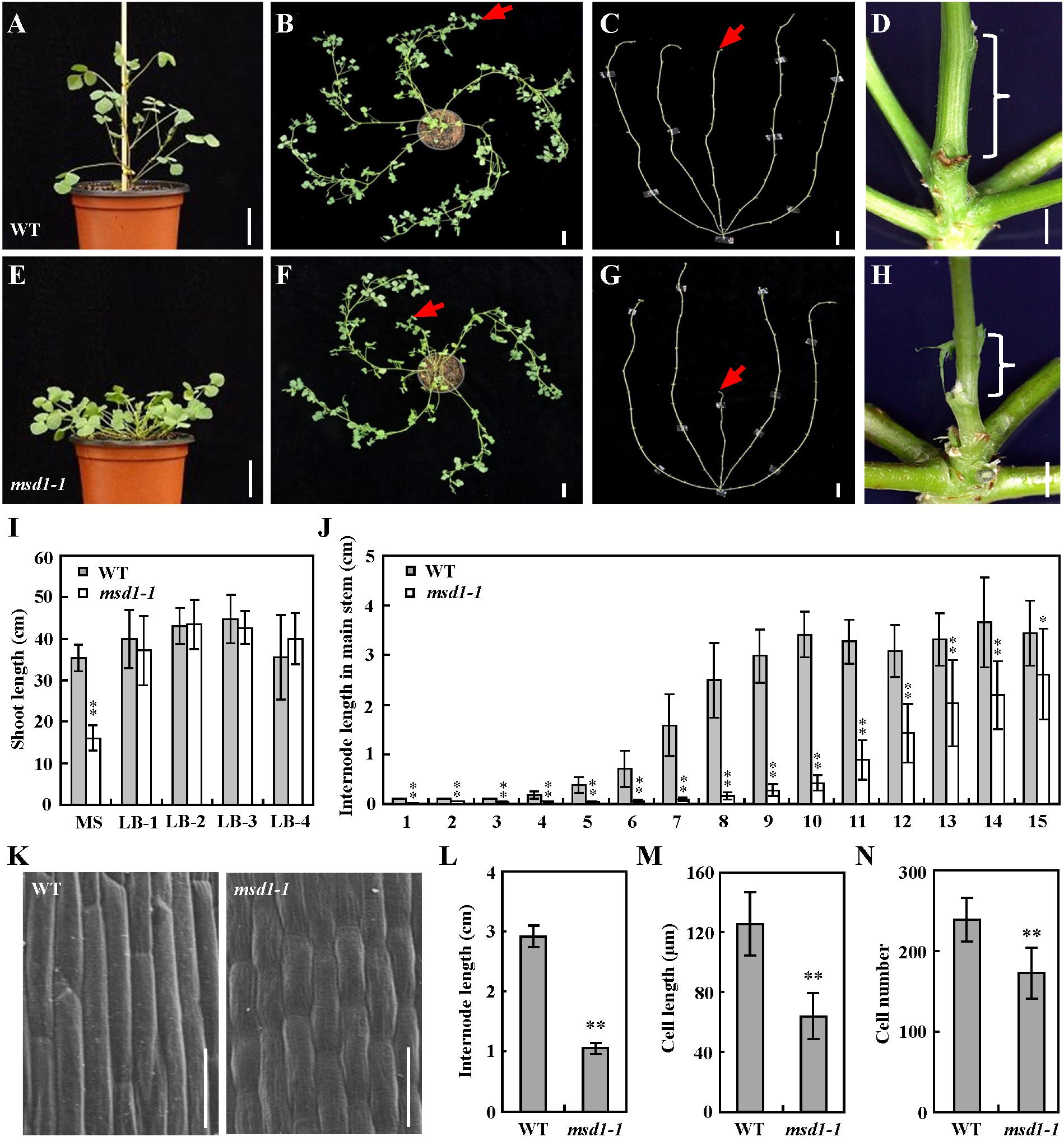
Figure 1. Morphological comparison of the wild type and msd1-1 plants. (A–H) Phenotypic comparison of seedling and stem between wild type (WT) and msd1-1. Seedlings of WT (A,B) and msd1-1 (E,F) at 5-week-old and 8-week-old, respectively, and 8-week-old bald stems without leaves in WT (C) and msd1-1 (G) plants. Bars = 3 cm; panels (D,H) are magnifications of shoot base in panels (C,G), respectively. Bars = 2 mm. Red arrows indicate main stem, braces indicate main stem internode. (I) Comparison of shoot length between 8-week-old WT and msd1-1 plants. MS, main stem; LB-1, the first lateral branch; LB-2, the second lateral branch; LB-3, the third lateral branch; LB-4, the fourth lateral branch. Bars represent means ± SD (n = 20); asterisks indicate significant differences from the WT (∗∗P < 0.01, Student’s t-test). (J) Comparison of internode length between 8-week-old WT and msd1-1 plants. The x-axis represents corresponding node numbers. Bars represent means ± SD (n = 20); asterisks indicate significant differences from the WT (∗∗P < 0.01, Student’s t-test). (K) Scanning electron microscope images of the seventh internode of WT and msd1-1 plants. Bars = 50 μm. (L) Comparison of the seventh internode length between 8-week-old WT and msd1-1 plants. Bars represent means ± SD (n = 10); asterisks indicate significant differences from the WT (∗∗P < 0.01, Student’s t-test). (M,N) Comparison of cell length (M) and cell number (N) in the seventh internode between 8-week-old WT and msd1-1 plants. Bars represent means ± SD (n = 15); asterisks indicate significant differences from the WT (∗∗P < 0.01, Student’s t-test).
To determine the reason of the reduced length in the main stem, we examined the cell numbers and lengths using the seventh main stem internode, which exhibited significant difference, and found that cells in the msd1-1 mutant were ∼50% the length of those in the wild type (Figures 1K,M). The total epidermal cell number was calculated as internode length/epidermal cell length, and this indicated that the number of cells in the msd1-1 mutant was ∼72% that of the wild type (Figures 1L–N). These results revealed that the main stem dwarf phenotype in the msd1-1 mutant resulted from both decreased length and number of internode cells, with the decreases in cell length accounting for the main effect.
Molecular Cloning of the MSD1 Gene
The msd1-1 mutant phenotype segregates as a single recessive mutation, in which heterozygous parents produce progeny that segregated 3:1 (35:10) for the wild-type-like and mutant plants. To identify the gene associated with the mutant phenotype, thermal asymmetric interlaced-PCR was performed to recover the flanking sequences of Tnt1 from msd1-1 (Tadege et al., 2008). Based on the genotyping results, one Tnt1 insertion segregating with the mutant phenotype was identified. Further genotyping analyses confirmed that all msd1-1 mutant plants harbored homozygous insertion for the particular flanking sequence tag (FST). The full length gene sequence corresponding to this FST was recovered and sequence alignment showed that the candidate gene encodes a putative GA 20-oxidase, which is identical to previously reported MtGA20ox1/Medtr1g102070 (Ma et al., 2019). Genomic PCR analysis showed that the Tnt1 was inserted in the second exon of MSD1, resulting in abolished transcription of the full-length MSD1 (Figures 2A,B). To confirm that the msd1-1 mutant phenotype is caused by disruption of MSD1, we obtained three additional Tnt1 insertion lines (NF12848, NF10524, and NF21287). Analysis of flanking sequences showed that NF12848, NF10524, and NF21287 contained Tnt1 insertions at different locations in exon 1 of MSD1; we therefore named these lines msd1-2, msd1-3, and msd1-4, respectively (Figure 2A). RT-PCR analysis revealed that the transcripts of MSD1 were abolished in these four mutants (Figure 2B), and msd1-2, msd1-3, and msd1-4 showed similar phenotype as observed in msd1-1 (Figure 2C). The identity of MSD1 was further confirmed by genetic complementation. We introduced the 1134-bp of full-length coding sequence of MSD1 driven by cauliflower mosaic virus (CaMV) 35S promoter into msd1-1 plants by A. tumefaciens-mediated transformation. The main stem elongation was rescued in the complemented transgenic msd1-1 plants (Figure 2C). Collectively, these data confirmed that disrupting MSD1 function leads to the main stem dwarf but normal side branches in the msd1 mutants.
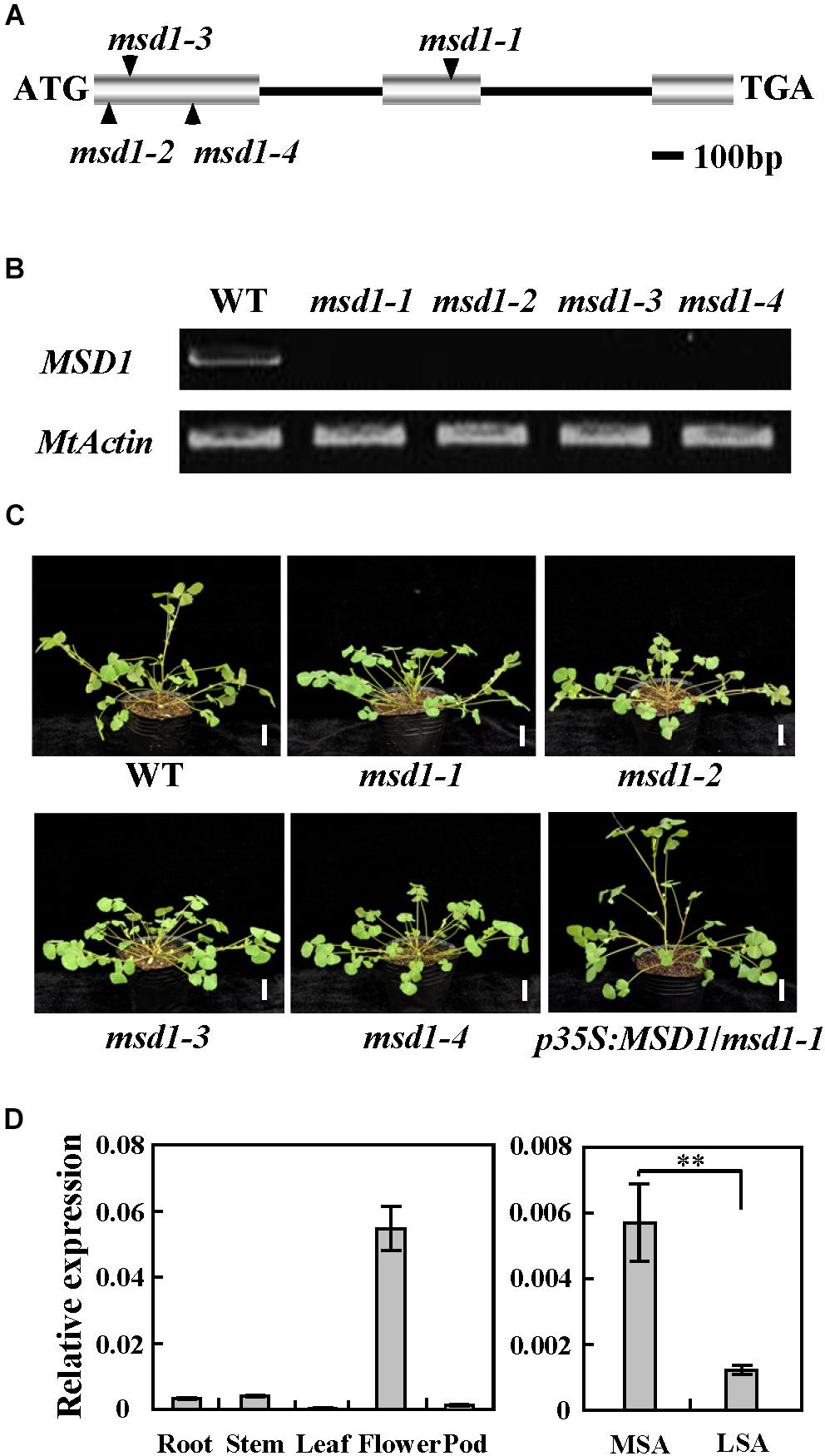
Figure 2. Molecular cloning of the MSD1 gene. (A) Schematic representation of the gene structure of MSD1 and the Tnt1 insertion sites in msd1-1, msd1-2, msd1-3, and msd1-4. (B) RT-PCR analysis of MSD1 expression in wild type (WT) and various msd1 alleles. MtActin was used as the loading control. (C) Phenotypic analysis of WT, msd1-1, msd1-2, msd1-3, msd1-4, and msd1-1 plants complemented with p35S:MSD1. Bars = 2 cm. (D) MSD1 transcript levels in different tissues, as revealed by quantitative RT-PCR. MtActin was used as an internal control. MSA, main shoot apices. LSA, lateral shoot apices. Values are means ± SD of three technical replicates; asterisks indicate significant differences (∗∗P < 0.01, Student’s t-test). Three independent experiments were performed, with similar results.
To explain why the main stem was specifically shortened while the side branches were no significant changes in msd1-1, quantitative RT-PCR was conducted in different tissues. The results revealed that MSD1 was expressed in flowers, axillary buds, stem, root, pods, leaf, and shoot apices (Figure 2D). It is worth mentioning that MSD1 was expressed significantly higher in main shoot apices than in lateral shoot apices, although the MSD1 expression level is low in both tissues (Figure 2D), which supports its function in controlling main stem elongation in M. truncatula.
MSD1 Affects Internode Elongation via Affecting GA Biosynthesis
Given that GA20ox is a key enzyme of the later steps in the GA biosynthesis pathway (Qin et al., 2013), we speculated that MSD1 might possess the conserved catalyzing function of GA20ox during the synthesis of bioactive GAs in M. truncatula. It has reported that GA20oxs use C20-GAs as substrates to produce immediate precursors, GA9 and GA20, then GA9 and GA20 are respectively converted to bioactive GA4 and GA1 by GA3ox (Yamaguchi, 2008; Figure 3A). We therefore analyzed the contents of GA9, GA20, GA1 and GA4 in msd1-1. UPLC-MS/MS analysis showed that the concentration of GA20 was slightly reduced in the msd1-1 mutant while GA9 was only 16.8% of that in the wild type (Figure 3B). Meanwhile, GA1 and GA4 in the msd1-1 mutant were respectively reduced to 83.8 and 51.6% of wild type (Figure 3B). To investigate whether the GA deficiency is related to the msd1 mutant phenotype, we treated 5-week-old msd1-1 mutant plants with 2 mM GA3 for a month, and found that the height of the plants was restored compared with the control (Figures 3C–E), indicating exogenous application of GA could restore the dwarf phenotype of the msd1-1 mutant. Taken together, our results demonstrated that MSD1 regulates the biosynthesis of bioactive GAs, which control plant height in M. truncatula.
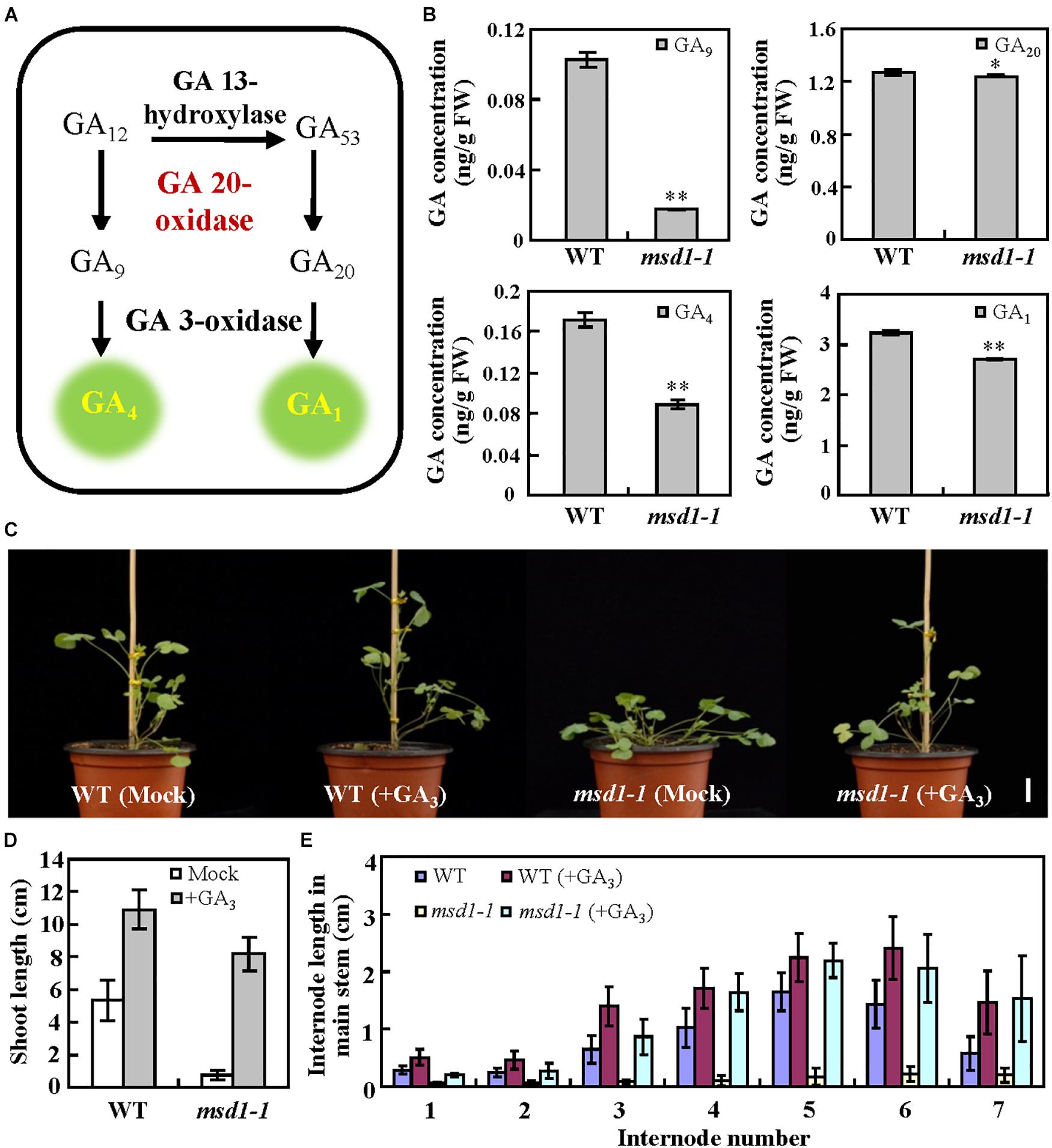
Figure 3. MSD1 was involved in GA biosynthesis pathway. (A) Simplified scheme of the later steps in GA biosynthesis pathway. (B) Concentrations of endogenous GA9, GA20, GA4, and GA1 in 5-week-old WT and msd1-1 plants. Bars represent means ± SD (n = 3); asterisks indicate significant differences from the WT (∗P < 0.05, ∗∗P < 0.01, Student’s t-test). (C) Phenotypes of wild type (WT) and msd1-1 mutant treated with 2 mM GA3 for 5 weeks in comparison with untreated controls (mock). Bars = 2 cm. (D) The main shoot height of plants in the experiment shown in panel (C). Bars represent means ± SD (n = 16). (E) The internode length in main stem in the experiment shown in panel (C). Bars represent means ± SD (n = 16).
Phylogenetic Analysis of M. truncatula GA 20-Oxidase Family Genes
MSD1 belongs to the 2-oxoglutarate-dependent dioxygenase (2-ODDs) family, which contains two conserved domains: DIOX_N and 2OG-FeII_Oxy (Pan et al., 2017; Tenreira and Lange, 2017; Supplementary Figure 1). The protein BLAST analysis revealed that MSD1 has seven homologs in the M. truncatula genome. Consistent with the previous report (Ma et al., 2019), phylogenetic analyses suggested MSD1, MtGA20ox7/Medtr6g464620 and MtGA20ox8/Medtr8g033380 were clustered to close groups, with MSD1 and MtGA20ox7 falling within the same clade, which is close to AtGA20ox1-4 (Figure 4A). Quantitative RT-PCR revealed that MtGA20ox7 and MtGA20ox8 were both expressed in flowers, axillary buds, stem, root, pods, leaf as well as shoot apices (Figures 4B,C), suggesting that MtGA20ox7 and MtGA20ox8 may serve similar function as MSD1 in the regulation of plant height via affecting GA biosynthesis.
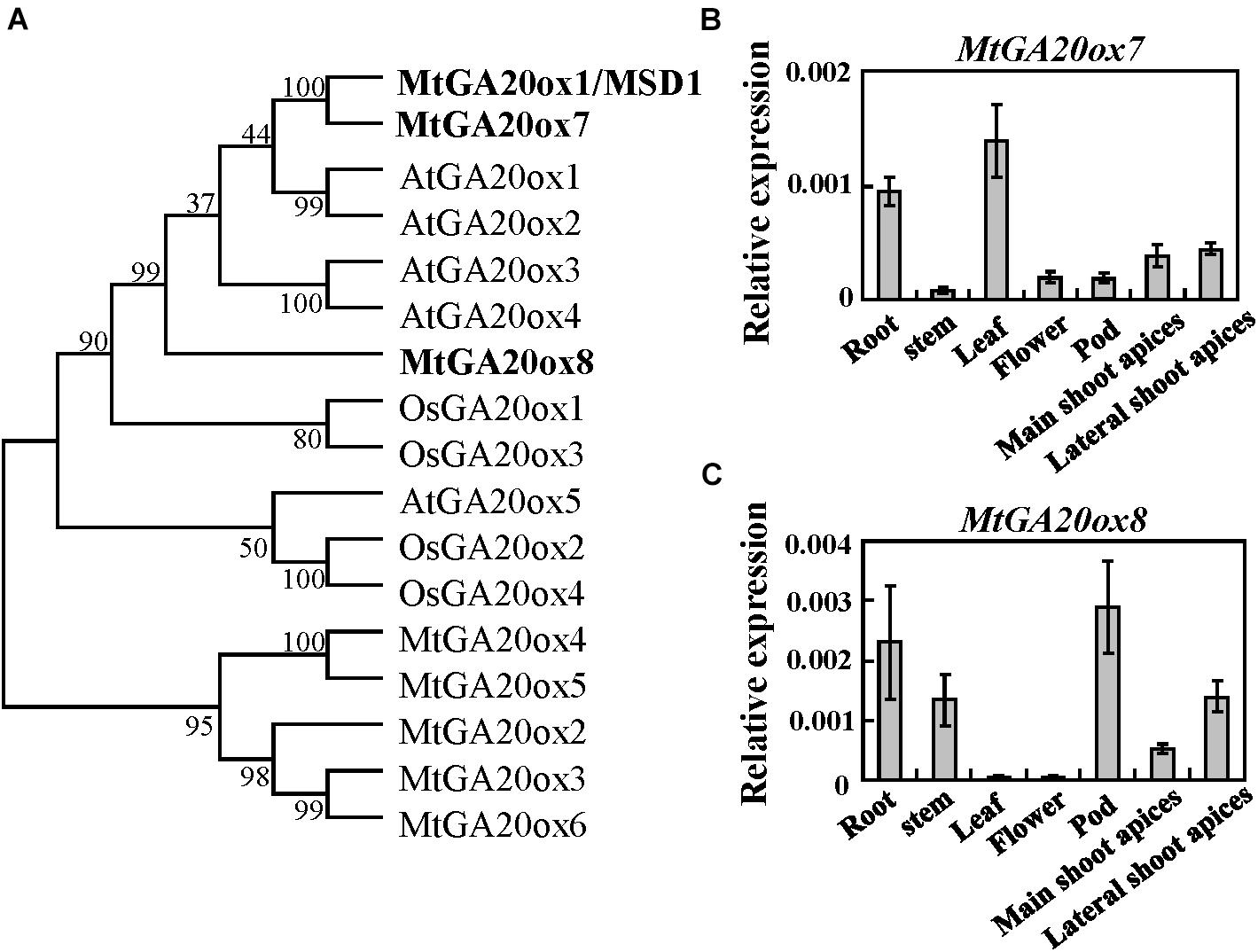
Figure 4. Phylogenetic analysis of MSD1 and its closely related homologs. (A) Phylogenetic analysis of MSD1 and its homologs in M. truncatula (Mt), Arabidopsis (At) and rice (Os). Numbers on branches indicate bootstrap percentages for 1,000 replicates. (B,C) MtGA20ox7-1 and MtGA20ox8 transcript levels in different tissues, as revealed by quantitative RT-PCR. MtActin was used as an internal control. Values are means ± SD of three technical replicates. Three independent experiments were performed, with similar results.
Genetic Analyses of MSD1, MtGA20ox7, and MtGA20ox8 in the Regulation of M. truncatula Shoot Development
To investigate the roles of MtGA20ox7 and MtGA20ox8 with respect to MSD1 function in the regulation of shoot elongation, we first identified two mutant lines (NF1343 and NF18196) harboring the Tnt1 insertion in the MtGA20ox7 locus (Ma et al., 2019). Analysis of flanking sequences showed that NF1343 and NF18196 contained Tnt1 insertions in exon 1 and exon 2 of MtGA20ox7, respectively (Supplementary Figure 2A). We therefore named these two lines mtga20ox7-1 and mtga20ox7-2. RT-PCR analysis revealed that the transcripts of MtGA20ox7 were abolished in above two mutant lines (Supplementary Figure 2B). Meanwhile, a loss-of-function mutant line (NF19184, named mtga20ox8) harboring the Tnt1 insertion in MtGA20ox8 was also identified (Ma et al., 2019; Supplementary Figure 3). In agree to previous findings, no obvious plant height defects were observed in mtga20ox7-1, mtga20ox7-2, and mtga20ox8 compared to the wild-type plants (Ma et al., 2019; Figure 5A; Supplementary Figures 2C, 3C). Next, we generated double and triple mutants with combinations of msd1-1, mtga20ox7-1, and mtga20ox8 to investigate the genetic relationship among MSD1, MtGA20ox7, and MtGA20ox8 in M. truncatula shoot elongation. Consistent with the notion that MSD1 acts as an essential regulator in M. truncatula main stem elongation, the msd1-1 showed the most severe phenotype, with significantly reduced main stem elongation relative to the mtga20ox7-1 and mtga20ox8 single mutants, which are comparable to wild type. However, the mtga20ox7-1 mtga20ox8 double mutants exhibited reduced main stem height, indicating that the contributions of MtGA20ox7 and MtGA20ox8 to M. truncatula main stem elongation are secondary and redundant (Figures 5A,B). Notably, the msd1-1 mtga20ox7-1 and msd1-1 mtga20ox8 double mutants and the msd1-1 mtga20ox7-1 mtga20ox8 triple mutant exhibited drastically reduced main stem height, showing an additive defect on main stem elongation (Figures 5A,B). In addition, phenotypic observations showed lateral shoot length and leaf size was reduced in both msd1-1 mtga20ox7-1 and msd1-1 mtga20ox8 double mutants, but the msd1-1 mtga20ox7-1 mtga20ox8 triple mutant showed drastically reduced lateral shoot length and leaf size relative to wild type plants (Figures 5C, 6). Collectively, these results indicated that MSD1 and its homologs MtGA20ox7 and MtGA20ox8 coordinately regulate shoot elongation and leaf development in M. truncatula.
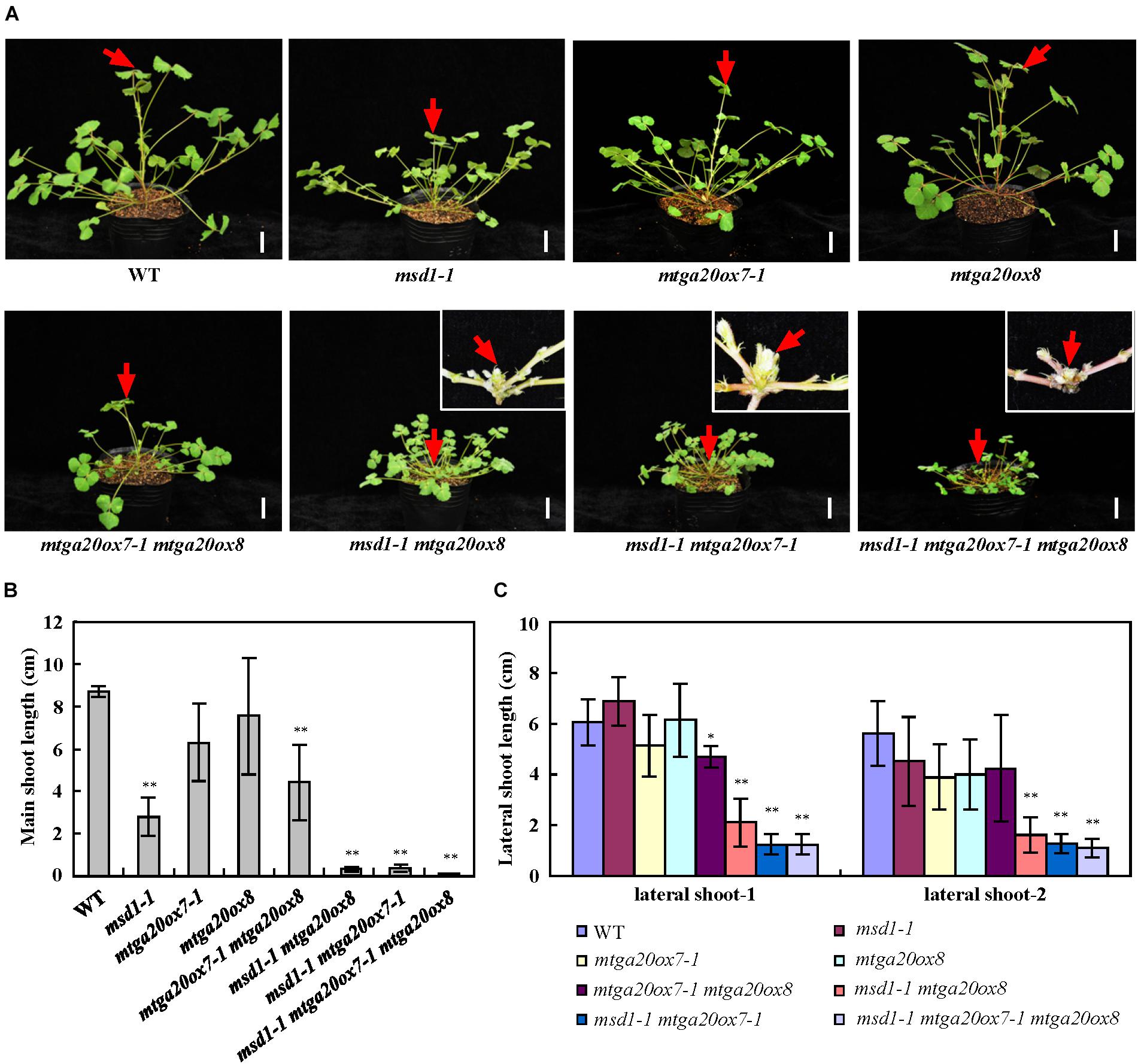
Figure 5. Genetic analyses of MSD1, MtGA20ox7 and MtGA20ox8 in regulating M. truncatula shoot elongation. (A) Phenotypic comparison of 4-week-old wild type (WT) and mtga20 mutants. Red arrows indicate main stem. The insets show close-up views of the main stem. Bars = 2 cm. (B) Comparison of the main shoot length in 4-week-old WT and mtga20 mutants. Bars represent means ± SD (n = 16); asterisks indicate significant differences from the WT (∗∗P < 0.01, Student’s t-test). (C) Comparison of the lateral shoot length in 4-week-old WT and mtga20 mutants. Bars represent means ± SD (n = 16); asterisks indicate significant differences from the WT (∗P < 0.05, ∗∗P < 0.01, Student’s t-test).
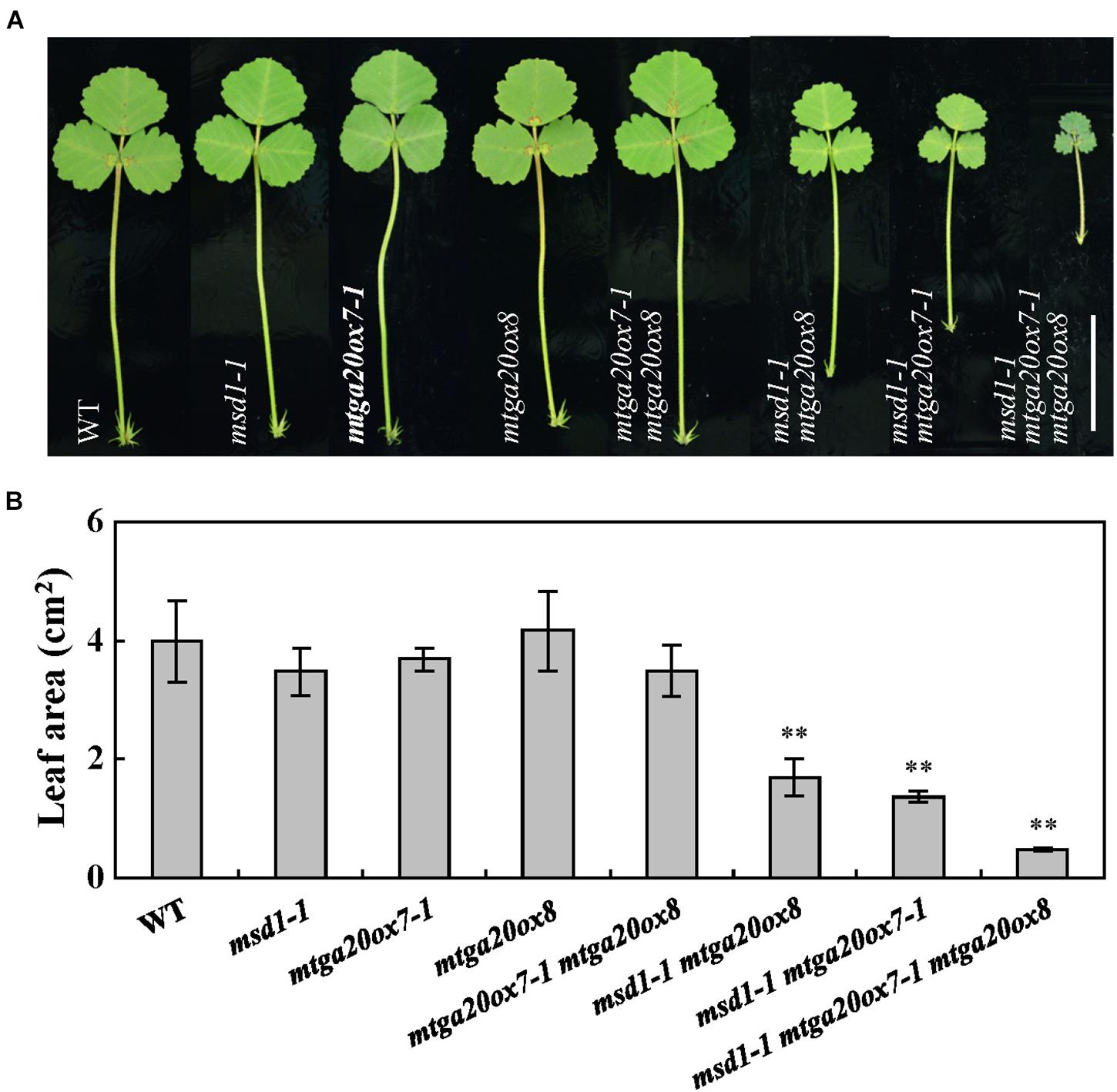
Figure 6. Comparison of leaves in wild type and mtga20ox mutants. (A) Phenotype of leaves in 4-week-old wild type (WT) and diverse mtga20ox mutants. Bars = 2 cm. (B) Comparison of leaf size in WT and mtga20ox mutants. Bars represent means ± SD (n = 16); asterisks indicate significant differences from the WT (∗∗P < 0.01, Student’s t-test).
Discussion
Plant height is one of the most important agricultural traits that determines biomass production and grain yield (Salas Fernandez et al., 2009). Despite the genes encoding GA20ox have been identified and characterized in several plant species (Rieu et al., 2008; Asano et al., 2011; Plackett et al., 2012; Chen et al., 2019), relatively little progress regarding the biological function of the GA20ox family genes is demonstrated in legumes with complex genomes (Igielski and Kepczynska, 2017). In this study, we reported that the disruption of MSD1, a putative GA 20-oxidase, by Tnt1 retrotransposon insertion resulted in severely reduced main stem elongation, which is associated with reduced content of GA in the model legume M. truncatula (Figures 2A,B), suggesting the functional conservation of the GA20ox family genes in the regulation of plant height in legumes. The contents of the bioactive GA1 and GA4 were both reduced in the msd1 mutant compared with the wild type (Figure 3B), indicating that MSD1 catalyzed the synthesis of bioactive GAs. Nevertheless, in contrast to GA20 and GA1, which show a relatively small reduction, the concentrations of GA9 and GA4 were significantly reduced in the msd1 mutant, suggesting that MSD1 may have a much greater effect on the biosynthesis of non-13-hydroxylated GAs in M. truncatula. Histological analysis showed the main shoot dwarf phenotype of msd1 is caused by the decrease of the cell elongation and cell division in the main stem (Figures 1K–N). This is consistent with previous studies demonstrating that GAs enhance cell elongation and proliferation (de Lucas et al., 2008; Hedden and Thomas, 2012; Lee et al., 2012; Tong et al., 2014). It has been reported that the cell elongation is regulated by cell wall-loosening protein expansin (EXP) and xyloglucan endo-transglycosylases (XET), which have been shown to be specifically upregulated by GAs in Arabidopsis and rice (Xu et al., 1995a; Lee and Kende, 2001, 2002). In addition, GAs can also induce cell elongation via upregulating the transcription levels of cell division-related genes including cell cycle genes CYCA1;1 and CDC2Os-3 in deepwater rice (Lee and Kende, 2002). Nevertheless, so far, the underlying mechanism by which GA regulates the expression of these genes remains to be elucidated in legume plants. Thus, the identification of msd1 mutant may provide a model system to further investigate the regulation mechanism of GA in determining cell proliferation and elongation in M. truncatula and other legumes.
It is worth noting that the msd1 plant showed a severely reduced main stem height but with normal lateral branch elongation (Figures 1A–J), which is rarely observed in GA20ox mutants identified in other plant species, suggesting the functional diversity of GA20ox in regulating plant height in legumes. The specific function of MSD1 in controlling main stem elongation may be explained by its spatial expression profile. Quantitative RT-PCR analysis revealed that MSD1 is expressed at significantly higher levels in the main shoot apex than in the lateral shoot apices, suggesting that tissue specificity appears to be important for the functional diversification among GA20ox gene family. Nevertheless, the finding that differential expression of MSD1 in main and lateral branches leads to significant different shoot length may provide a cue for further investigating the regulation mechanism of GA in M. truncatula shoot development and may be valuable in plant breeding.
The M. truncatula genome contains eight GA20ox family members and phylogenetic analyses showed that MSD1, MtGA20ox7 and MtGA20ox8 are close to AtGA20ox1-4, OsGA20ox1 and OsGA20ox3, which are involved in regulating plant height in Arabidopsis and rice (Figure 4A; Igielski and Kepczynska, 2017). Phenotypic analysis in single mutant of msd1-1, mtga20ox7-1 and mtga20ox8 suggested that MSD1 plays an essential role in controlling main shoot height, while MtGA20ox7 and MtGA20ox8 showed no obvious influence in regulating main shoot height in M. truncatula (Figures 5A,B and Supplementary Figures 2C, 3C). Nevertheless, the double mutants of msd1-1 mtga20ox7-1 and msd1-1 mtga20ox8 both exhibit more severe dwarf in main shoot height compared to msd1-1 (Figures 5A,B). The triple mutant msd1-1 mtga20ox7-1 mtga20ox8 has the most seriously decreased main shoot height and leaf size (Figures 5A,B, 6), suggesting that MtGA20ox7 and MtGA20ox8 are functionally redundant to MSD1 in the regulation of shoot elongation and leaf development. Therefore, further elucidating the actions of diverse MtGA20ox members and investigating their genetic interactions will enlighten our understanding of biological function of MtGA20oxs in controlling M. truncatula growth and development.
Taken together, our studies reveal the molecular mechanism of MSD1-mediated regulation of main stem elongation and demonstrate the coordination of MSD1 and its homologs MtGA20ox7 and MtGA20ox8 in controlling M. truncatula shoot elongation and leaf development, which provide insights into understanding the functional diversity of GA 20-oxidases in optimizing plant architecture in legumes.
Data Availability Statement
The original contributions presented in the study are included in the article/Supplementary Material, further inquiries can be directed to the corresponding author/s.
Author Contributions
WL, QM, PY, LN, and HL designed the research and analyzed the data. WL, QM, and PY performed the experiments. JW and YP contributed the analytical tools. WL, LN, and HL wrote the manuscript. All authors contributed to the article and approved the submitted version.
Funding
This work was supported by grants from the National Natural Science Foundation of China (32071864), the Fundamental Research Funds for Central Non-profit Scientific Institution (Y2020YJ12 and No. 1610392020005), and the Agricultural Science and Technology Innovation Program of CAAS (CAAS-ZDRW202009 and CAAS-ZDXT2019004).
Conflict of Interest
The authors declare that the research was conducted in the absence of any commercial or financial relationships that could be construed as a potential conflict of interest.
Publisher’s Note
All claims expressed in this article are solely those of the authors and do not necessarily represent those of their affiliated organizations, or those of the publisher, the editors and the reviewers. Any product that may be evaluated in this article, or claim that may be made by its manufacturer, is not guaranteed or endorsed by the publisher.
Supplementary Material
The Supplementary Material for this article can be found online at: https://www.frontiersin.org/articles/10.3389/fpls.2021.709625/full#supplementary-material
Supplementary Figure 1 | Amino acid sequence alignment of MSD1 and its close homologs in Arabidopsis and M. truncatula.
Supplementary Figure 2 | Identification of the mtga20ox7 mutant.
Supplementary Figure 3 | Identification of the mtga20ox8 mutant.
Supplementary Table 1 | Primers used in this study.
Supplementary Table 2 | Accession numbers used in this study.
Footnotes
References
Asano, K., Yamasaki, M., Takuno, S., Miura, K., Katagiri, S., Ito, T., et al. (2011). Artificial selection for a green revolution gene during japonica rice domestication. Proc. Natl. Acad. Sci. U.S.A. 108, 11034–11039. doi: 10.1073/pnas.1019490108
Chen, M. L., Fu, X. M., Liu, J. Q., Ye, T. T., Hou, S. Y., Huang, Y. Q., et al. (2012). Highly sensitive and quantitative profiling of acidic phytohormones using derivatization approach coupled with nano-LC-ESI-Q-TOF-MS analysis. J. Chromatogr. B Analyt. Technol. Biomed. Life Sci. 905, 67–74. doi: 10.1016/j.jchromb.2012.08.005
Chen, X., Tian, X., and Xue, L. (2019). CRISPR-based assessment of gene specialization in the gibberellin metabolic pathway in rice. Plant Physiol. 180, 2091–2105. doi: 10.1104/pp.19.00328
Chen, Y., Hou, M., Liu, L., Wu, S., Shen, Y., Ishiyama, K., et al. (2014). The maize DWARF1 encodes a gibberellin 3-oxidase and is dual localized to the nucleus and cytosol. Plant Physiol. 166, 2028–2039. doi: 10.1104/pp.114.247486
Chiang, H. H., Hwang, I., and Goodman, H. M. (1995). Isolation of the Arabidopsis GA4 locus. Plant cell 7, 195–201. doi: 10.1105/tpc.7.2.195
Dalmadi, A., Kaló, P., Jakab, J., Saskoi, A., Petrovics, T., Deák, G., et al. (2008). Dwarf plants of diploid Medicago sativa carry a mutation in the gibberellin 3-beta-hydroxylase gene. Plant Cell Rep. 27, 1271–1279. doi: 10.1007/s00299-008-0546-5
de Lucas, M., Davière, J. M., Rodríguez-Falcón, M., Pontin, M., Iglesias-Pedraz, J. M., Lorrain, S., et al. (2008). A molecular framework for light and gibberellin control of cell elongation. Nature 451, 480–484. doi: 10.1038/nature06520
Graham, P. H., and Vance, C. P. (2003). Legumes: importance and constraints to greater use. Plant Physiol. 131, 872–877. doi: 10.1104/pp.017004
Guo, S., Zhang, X., Bai, Q., Zhao, W., Fang, Y., Zhou, S., et al. (2020). Cloning and functional analysis of dwarf gene mini plant 1 (MNP1) in Medicago truncatula. Int. J. Mol. Sci. 2:4968. doi: 10.3390/ijms21144968
Hedden, P., and Sponsel, V. (2015). A century of Gibberellin research. J. Plant Growth Regul. 34, 740–760. doi: 10.1007/s00344-015-9546-1
Hedden, P., and Thomas, S. G. (2012). Gibberellin biosynthesis and its regulation. Biochemical. J. 444, 11–25. doi: 10.1042/BJ20120245
Helliwell, C. A., Sheldon, C. C., Olive, M. R., Walker, A. R., Zeevaart, J. A., Peacock, W. J., et al. (1998). Cloning of the Arabidopsis ent-kaurene oxidase gene GA3. Proc. Natl. Acad. Sci. U.S.A. 95, 9019–9024. doi: 10.1073/pnas.95.15.9019
Hu, J., Mitchum, M. G., Barnaby, N., Ayele, B. T., Ogawa, M., Nam, E., et al. (2008). Potential sites of bioactive gibberellin production during reproductive growth in Arabidopsis. Plant Cell 20, 320–336. doi: 10.1105/tpc.107.057752
Igielski, R., and Kepczynska, E. (2017). Gene expression and metabolite profiling of gibberellin biosynthesis during induction of somatic embryogenesis in Medicago truncatula Gaertn. PLoS One 12:e0182055. doi: 10.1371/journal.pone.0182055
Israelsson, M., Mellerowicz, E., Chono, M., Gullberg, J., and Moritz, T. (2004). Cloning and overproduction of gibberellin 3-oxidase in hybrid aspen trees. effects on gibberellin homeostasis and development. Plant Physiol. 135, 221–230. doi: 10.1104/pp.104.038935
Itoh, H., Ueguchi-Tanaka, M., Sentoku, N., Kitano, H., Matsuoka, M., and Kobayashi, M. (2001). Cloning and functional analysis of two gibberellin 3 beta -hydroxylase genes that are differently expressed during the growth of rice. Proc. Natl. Acad. Sci. U.S.A. 98, 8909–8914. doi: 10.1073/pnas.141239398
Lange, T., Hedden, P., and Graebe, J. E. (1994). Expression cloning of a gibberellin 20-oxidase, a multifunctional enzyme involved in gibberellin biosynthesis. Proc. Natl. Acad. Sci. U.S.A. 91, 8552–8556. doi: 10.1073/pnas.91.18.8552
Lee, L. Y., Hou, X., Fang, L., Fan, S., Kumar, P. P., and Yu, H. (2012). STUNTED mediates the control of cell proliferation by GA in Arabidopsis. Development 139, 1568–1576. doi: 10.1242/dev.079426
Lee, Y., and Kende, H. (2001). Expression of beta-expansins is correlated with internodal elongation in deepwater rice. Plant Physiol. 127, 645–654.
Lee, Y., and Kende, H. (2002). Expression of alpha-expansin and expansin-like genes in deepwater rice. Plant Physiol. 130, 1396–1405. doi: 10.1104/pp.008888
Li, R., Sun, S., Wang, H., Wang, K., and Yu, H. (2020). FIS1 encodes a GA2-oxidase that regulates fruit firmness in tomato. Nat. Commun. 11, 5844. doi: 10.1038/s41467-020-19705-w
Li, Z., Guo, Y., Ou, L., Hong, H., Wang, J., Liu, Z., et al. (2018). Identification of the dwarf gene GmDW1 in soybean (Glycine max L.) by combining mapping-by-sequencing and linkage analysis. Theor. Appl. Genet. 131, 1001–1016. doi: 10.1007/s00122-017-3044-8
Ma, Q., Yin, P., Lin, H., Jin, Z., Wang, H., Yang, J., et al. (2019). Functional analysis of gibberellin oxidase gene MtGA20ox in Medicago truncatula. Curr. Biotechnol. 9, 161–168.
Magome, H., Nomura, T., Hanada, A., Takeda-Kamiya, N., Ohnishi, T., Shinma, Y., et al. (2013). CYP714B1 and CYP714B2 encode gibberellin 13-oxidases that reduce gibberellin activity in rice. Proc. Natl. Acad. Sci. U.S.A. 110, 1947–1952. doi: 10.1073/pnas.1215788110
Nomura, T., Magome, H., Hanada, A., Takeda-Kamiya, N., Mander, L. N., Kamiya, Y., et al. (2013). Functional analysis of Arabidopsis CYP714A1 and CYP714A2 reveals that they are distinct gibberellin modification enzymes. Plant Cell Physiol. 54, 1837–1851. doi: 10.1093/pcp/pct125
Oikawa, T., Koshioka, M., Kojima, K., Yoshida, H., and Kawata, M. (2004). A role of OsGA20ox1, encoding an isoform of gibberellin 20-oxidase, for regulation of plant stature in rice. Plant Mol. Biol. 55, 687–700. doi: 10.1007/s11103-004-1692-y
Pan, C., Tian, K., Ban, Q., Wang, L., Sun, Q., He, Y., et al. (2017). Genome-wide analysis of the biosynthesis and deactivation of gibberellin-dioxygenases gene family in Camellia sinensis (L.) O. Kuntze. Genes 8:235. doi: 10.3390/genes8090235
Peng, J., Richards, D. E., Hartley, N. M., Murphy, G. P., Devos, K. M., Flintham, J. E., et al. (1999). ‘Green revolution’ genes encode mutant gibberellin response modulators. Nature 400, 256–261. doi: 10.1038/22307
Plackett, A. R., Powers, S. J., Fernandez-Garcia, N., Urbanova, T., Takebayashi, Y., Seo, M., et al. (2012). Analysis of the developmental roles of the Arabidopsis gibberellin 20-oxidases demonstrates that GA20ox1, -2, and -3 are the dominant paralogs. Plant Cell 24, 941–960. doi: 10.1105/tpc.111.095109
Qin, X., Liu, J. H., Zhao, W. S., Chen, X. J., Guo, Z. J., and Peng, Y. L. (2013). Gibberellin 20-oxidase gene OsGA20ox3 regulates plant stature and disease development in rice. Mol. Plant. Microb. Interact. 26, 227–239. doi: 10.1094/MPMI-05-12-0138-R
Regnault, T., Davière, J. M., Heintz, D., Lange, T., and Achard, P. (2014). The gibberellin biosynthetic genes AtKAO1 and AtKAO2 have overlapping roles throughout Arabidopsis development. Plant J. 80, 462–474. doi: 10.1111/tpj.12648
Rieu, I., Ruiz-Rivero, O., Fernandez-Garcia, N., Griffiths, J., Powers, S. J., Gong, F., et al. (2008). The gibberellin biosynthetic genes AtGA20ox1 and AtGA20ox2 act, partially redundantly, to promote growth and development throughout the Arabidopsis life cycle. Plant J. 53, 488–504. doi: 10.1111/j.1365-313X.2007.03356.x
Salas Fernandez, M. G., Becraft, P. W., Yin, Y., and Lübberstedt, T. (2009). From dwarves to giants? Plant height manipulation for biomass yield. Trends Plant Sci. 14, 454–461. doi: 10.1016/j.tplants.2009.06.005
Sasaki, A., Ashikari, M., Ueguchi-Tanaka, M., Itoh, H., Nishimura, A., Swapan, D., et al. (2002). Green revolution: a mutant gibberellin-synthesis gene in rice. Nature 416, 701–702. doi: 10.1038/416701a
Shoemaker, R. C., Schlueter, J., and Doyle, J. J. (2006). Paleopolyploidy and gene duplication in soybean and other legumes. Curr. Opin. Plant Biol. 9, 104–109. doi: 10.1016/j.pbi.2006.01.007
Spielmeyer, W., Ellis, M. H., and Chandler, P. M. (2002). Semidwarf (sd-1), “green revolution” rice, contains a defective gibberellin 20-oxidase gene. Proc. Natl. Acad. Sci. U.S.A. 99, 9043–9048. doi: 10.1073/pnas.132266399
Sun, T. P., and Kamiya, Y. (1994). The Arabidopsis GA1 locus encodes the cyclase ent-kaurene synthetase a of gibberellin biosynthesis. Plant cell 6, 1509–1518. doi: 10.1105/tpc.6.10.1509
Tadege, M., Lin, H., Bedair, M., Berbel, A., Wen, J., Rojas, C. M., et al. (2011). STENOFOLIA regulates blade outgrowth and leaf vascular patterning in Medicago truncatula and Nicotiana sylvestris. Plant Cell 23, 2125–2142. doi: 10.1105/tpc.111.085340
Tadege, M., Wen, J., He, J., Tu, H., Kwak, Y., Eschstruth, A., et al. (2008). Large-scale insertional mutagenesis using the Tnt1 retrotransposon in the model legume Medicago truncatula. Plant J. 54, 335–347. doi: 10.1111/j.1365-313X.2008.03418.x
Tenreira, T., and Lange, M. J. P. (2017). A specific gibberellin 20-oxidase dictates the flowering-runnering decision in diploid strawberry. Plant Cell 29, 2168–2182. doi: 10.1105/tpc.16.00949
Tong, H., Xiao, Y., Liu, D., Gao, S., Liu, L., Yin, Y., et al. (2014). Brassinosteroid regulates cell elongation by modulating gibberellin metabolism in rice. Plant Cell 26, 4376–4393. doi: 10.1105/tpc.114.132092
Ueguchi-Tanaka, M., Nakajima, M., Motoyuki, A., and Matsuoka, M. (2007). Gibberellin receptor and its role in gibberellin signaling in plants. Annu. Rev. Plant Biol. 58, 183–198. doi: 10.1146/annurev.arplant.58.032806.103830
Wang, H., Jiang, H., Xu, Y., Wang, Y., Zhu, L., Yu, X., et al. (2020). Systematic analysis of gibberellin pathway components in medicago truncatula reveals the potential application of gibberellin in biomass improvement. Int. J. Mol. Sci 21:7180. doi: 10.3390/ijms21197180
Wang, H., Niu, L., Fu, C., Meng, Y., Sang, D., Yin, P., et al. (2017). Overexpression of the WOX gene STENOFOLIA improves biomass yield and sugar release in transgenic grasses and display altered cytokinin homeostasis. PLoS Genet. 13:e1006649. doi: 10.1371/journal.pgen.1006649
Wang, Y., and Li, J. (2008). Molecular basis of plant architecture. Annu. Rev. Plant Biol. 59, 253–279. doi: 10.1146/annurev.arplant.59.032607.092902
Xu, W., Purugganan, M. M., Polisensky, D. H., Antosiewicz, D. M., Fry, S. C., and Braam, J. (1995a). Arabidopsis TCH4, regulated by hormones and the environment, encodes a xyloglucan endotransglycosylase. Plant Cell 7, 1555–1567. doi: 10.1105/tpc.7.10.1555
Xu, Y. L., Li, L., Wu, K., Peeters, A. J., Gage, D. A., and Zeevaart, J. A. (1995b). The GA5 locus of Arabidopsis thaliana encodes a multifunctional gibberellin 20-oxidase: molecular cloning and functional expression. Proc. Natl. Acad. Sci. U.S.A. 92, 6640–6644. doi: 10.1073/pnas.92.14.6640
Yamaguchi, S. (2008). Gibberellin metabolism and its regulation. Annu. Rev. Plant Biol. 59, 225–251. doi: 10.1146/annurev.arplant.59.032607.092804
Yarce, J. C., Lee, H. K., Tadege, M., Ratet, P., and Mysore, K. S. (2013). Forward genetics screening of Medicago truncatula Tnt1 insertion lines. Methods Mol. Biol. 1069, 93–100. doi: 10.1007/978-1-62703-613-9_8
Yaxley, J. R., Ross, J. J., Sherriff, L. J., and Reid, J. B. (2001). Gibberellin biosynthesis mutations and root development in pea. Plant Physiol. 125, 627–633. doi: 10.1104/pp.125.2.627
Keywords: GA 20-oxidase, MSD1, main stem elongation, functional diversification, Medicago truncatula
Citation: Li W, Ma Q, Yin P, Wen J, Pei Y, Niu L and Lin H (2021) The GA 20-Oxidase Encoding Gene MSD1 Controls the Main Stem Elongation in Medicago truncatula. Front. Plant Sci. 12:709625. doi: 10.3389/fpls.2021.709625
Received: 14 May 2021; Accepted: 25 June 2021;
Published: 04 August 2021.
Edited by:
Yuling Jiao, Institute of Genetics and Developmental Biology, Chinese Academy of Sciences, ChinaReviewed by:
Peter Hedden, Peter Hedden, CzechiaJoanna Putterill, The University of Auckland, New Zealand
Copyright © 2021 Li, Ma, Yin, Wen, Pei, Niu and Lin. This is an open-access article distributed under the terms of the Creative Commons Attribution License (CC BY). The use, distribution or reproduction in other forums is permitted, provided the original author(s) and the copyright owner(s) are credited and that the original publication in this journal is cited, in accordance with accepted academic practice. No use, distribution or reproduction is permitted which does not comply with these terms.
*Correspondence: Hao Lin, linhao@caas.cn; Lifang Niu, niulifang@caas.cn
†These authors have contributed equally to this work