- 1College of Life Science and Food Engineering, Inner Mongolia Minzu University, Tongliao, China
- 2Horqin Plant Stress Biology Research Institute of Inner Mongolia Minzu University, Tongliao, China
- 3U.S. Department of Agriculture-Agricultural Research Service, Plant Genetics Research Unit, Donald Danforth Plant Science Center, Saint Louis, MO, United States
Glutathione peroxidases (GPXs) protect cells against damage caused by reactive oxygen species (ROS) and play key roles in regulating many biological processes. Here, five GPXs were identified in the Ricinus communis genome. Phylogenetic analysis displayed that the GPXs were categorized into five groups. Conserved domain and gene structure analyses showed that the GPXs from different plant species harbored four highly similar motifs and conserved exon-intron arrangement patterns, indicating that their structure and function may have been conserved during evolution. Several abiotic stresses and hormone-responsive cis-acting elements existed in the promoters of the RcGPXs. The expression profiles indicated that the RcGPXs varied substantially, and some RcGPXs were coordinately regulated under abiotic stresses. Overexpression of RcGPX4 in Arabidopsis enhanced cold tolerance at seed germination but reduced freezing tolerance at seedlings. The expression of abscisic acid (ABA) signaling genes (AtABI4 and AtABI5), ABA catabolism genes (AtCYP707A1 and AtCYP707A2), gibberellin acid (GA) catabolism gene (AtGA2ox7), and cytokinin (CTK)-inducible gene (AtARR6) was regulated in the seeds of transgenic lines under cold stress. Overexpression of RcGPX4 can disturb the hydrogen peroxide (H2O2) homeostasis through the modulation of some antioxidant enzymes and compounds involved in the GSH-ascorbate cycle in transgenic plants. Additionally, RcGPX4 depended on the MAPK3-ICE1-C-repeat-binding factor (CBF)-COR signal transduction pathway and ABA-dependent pathway to negatively regulate the freezing tolerance of transgenic plants. This study provides valuable information for understanding the potential function of RcGPXs in regulating the abiotic stress responses of castor beans.
Introduction
Plants are often exposed to diverse abiotic stresses, which significantly constrain the spatial distribution and yield of crops (Agarwal et al., 2006). These abiotic stresses can induce the accumulation of reactive oxygen species (ROS) (Choudhury et al., 2017). Although ROS can act as signaling molecules to activate the stress responses, high concentrations of ROS can damage various cellular components, leading to the death of cells (You and Chan, 2015). To survive, plants have developed antioxidant mechanisms for scavenging ROS, such as non-enzymatic and enzymatic antioxidants, to protect cells from uncontrolled oxidative damage (Blokhina et al., 2003). As a key ROS scavenger, glutathione peroxidases (GPXs, EC 1.11.1.9) belong to non-heme PODs and use reduced glutathione (GSH) or thioredoxin (Trx) as a reductant to convert organic hydroperoxides or hydrogen peroxide (H2O2) to the corresponding alcohols and water (Ursini et al., 1995; Bela et al., 2015; Passaia and Margispinheiro, 2015). Plant GPXs carry cysteine residue in the active site and have a preference for Trx as electron donors (Navrot et al., 2006; Koua et al., 2009).
A body of evidence has demonstrated that plant GPXs are responsive to abiotic stresses, such as drought, cold, salt, and oxidative stresses (Roxas et al., 2000; Yoshimura et al., 2004; Navrot et al., 2006; Passaia et al., 2013; Kim et al., 2014; Islam et al., 2015a). For instance, the transcript level of Nelumbo nucifera NnGPX was dramatically increased under cold, heat, salt treatments, and mechanical damage. Overexpression of NnGPX in rice has markedly improved salt tolerance (Diao et al., 2014). Overexpression of an endogenous GSH S-transferase with GPX activity in tobacco has enhanced seedling growth under cold stress compared with the control seedlings (Roxas et al., 2000). Two wheat GPXs were overexpressed in Arabidopsis and displayed higher salt and H2O2 tolerance compared to wild type (WT) (Zhai et al., 2013). Rhodiola crenulata RcGPX5 conferred drought tolerance in transgenic plants by reducing the production of malondialdehyde (MDA) and increasing antioxidant enzyme activities (Zhang et al., 2019). Five GPXs were identified in rice and showed different responses to stress treatments (Passaia et al., 2013). In Arabidopsis, overexpression of chloroplastic GPXs increased tolerance to abiotic and biotic stresses. The reduced expression of both GPX1 and GPX7 resulted in severe morphological alterations in the leaves and chloroplasts of Arabidopsis (Chang et al., 2009). These findings revealed that GPXs regulated plant growth, development, and various abiotic stress responses.
To date, GPX genes from various plant species, such as Panax ginseng (Kim et al., 2014), O. sativa (Passaia et al., 2013), Triticum aestivum (Zhai et al., 2013), Arabidopsis thaliana (Miao et al., 2006; Chang et al., 2009; Gaber et al., 2012), Populus trichocarpa (Koh et al., 2007; Selles et al., 2012), and Thellungiella salsuginea (Gao et al., 2014), have been cloned and functionally characterized. Investigation of GPX families in A. thaliana (Gaber et al., 2012), Lotus japonicus (Ramos et al., 2009), P. trichocarpa (Navrot et al., 2006), O. sativa (Islam et al., 2015a), Gossypium hirsutum (Chen et al., 2017), and Cucumis sativus (Zhou et al., 2018) showed that the plant GPX family not only contains numerous GPXs with different subcellular localizations and physicochemical properties but also displays diverse abiotic stress responses. Thus, these studies emphasized the significance of GPX family studies on the genome-wide level.
Ricinus communis L. (R. communis) is an important non-edible oilseed crop, and its seed oil is widely used for pharmaceutical and industrial applications due to its high level of ricinoleic acid (Akpan et al., 2006; Ogunniyi, 2006; Scholza and da Silva, 2008). However, unfavorable environments, such as low temperature, severely inhibit the growth and development of the castor beans (Wang et al., 2019). Considering the increasing industry demands for castor bean oil, great effort is made to generate stress-tolerant and broadly adapted varieties by traditional breeding and genetic engineering techniques. It is imperative to reveal the molecular mechanism underlying the resistance of castor beans to inferior growth conditions. In this study, we identified GPX family genes in R. communis and analyzed their expression profiles under various stress conditions. Furthermore, RcGPX4 was functionally characterized. Overexpression of RcGPX4 in Arabidopsis decreased sensitivity to cold stress at seed germination but showed hypersensitivity to freezing treatment at seedlings compared with the WT. This study laid a foundation for developing new cold-tolerant castor cultivars.
Materials and Methods
Identification of RcGPXs and Protein Properties Analysis
To systematically identify the putative GPX in castor bean, the published GPX protein sequences, such as five in O. sativa (Islam et al., 2015a), eight in A. thaliana (Rodriguez Milla et al., 2003), six in L. japonicus (Ramos et al., 2009), six in C. sativus (Zhou et al., 2018), and 13 in G. hirsutum (Chen et al., 2017), were blasted against R. communis database.1 Furthermore, the GPX domain (PF00255)2 was used to search the R. communis database with HMMER software. The redundant sequences of GPXs were screened and removed. The remaining genes were confirmed by the existence of the GPX domain by Pfam. The molecular weights (kDa) and isoelectric points (PIs) were estimated using the ProtParam tool (Gasteiger et al., 2005). Subcellular localization was predicted by CELLO (Yu et al., 2006), WoLF PROST (Horton et al., 2007), and TargetP-2.0 (Armenteros et al., 2019).
Analyses of Glutathione Peroxidases Genes/Proteins
Sequences of GPXs from rice, cucumber, Arabidopsis, and castor bean were aligned by Clustal W software (Larkin et al., 2007). The conserved motifs of GPXs were analyzed using the online MEME tool with the following parameters: the maximum number of motifs was set to 5 and the width of the motif was between 6 and 50 (Bailey et al., 2009). The exon-intron arrangement was analyzed by alignment of coding sequences (CDS) and genomic sequences using the online Gene Structure Display Server (GSDS) analysis tool (Hu et al., 2015). Seventy-five GPX protein sequences from R. communis, C. sativus, O. sativa, A. thaliana, Vitis vinifera, T. salsuginea, Solanum lycopersicum, Phaseolus vulgaris Linn, P. trichocarpa, Phoenix dactylifera, Medicago sativa Linn, Citrullus lanatus, and Brassica napus were used to perform comprehensive phylogenetic analysis using the Bayesian method. Briefly, a multiple sequence alignment of the selected amino acid sequences was automatically performed using Muscle (Edgar, 2004). The region used for generating the phylogenetic tree was obtained by excluding sites with alignment gaps (where gaps were found in over 5% of each alignment site) and missing data, leaving 166 positions in the final dataset. The best model of evolution was selected using Modelgenerator V.851 (Keane et al., 2006) following the corrected Akaike Information Criterion with four discrete gamma categories and used to construct the phylogenetic tree. The best model of evolution identified by the model generator was Le Gascuel (LG) + G (Le and Gascuel, 2008). But there were only 10 amino acid modes implemented excluding LG + G in MrBayes, the suboptimal available model Jones Taylor Thornton (JTT) + G (implemented under the name “Jones model” in MrBayes) was used (Jones et al., 1992). Finally, the phylogenetic analysis was inferred with MrBayes V.3.2.1 (Huelsenbeck et al., 2001) using the following parameters: 15 million generations, sampling every 1,000 generations, temperature = 0.2. The phylogenetic tree was decorated with Interactive Tree Of Life (iTOL) V5 (Letunic and Bork, 2021). The protein IDs and sequences of GPXs were listed in Supplementary Table 1. Total 1,500 bp sequences upstream from the translational start site of each gene were surveyed to predict cis-acting regulatory elements against the PlantCare database (Lescot et al., 2002).
Plasmid Construction and Arabidopsis Transformation of RcGPX4
The CDS of RcGPX4 was amplified from the castor bean leaves by real-time (RT)-PCR and inserted into the pCambia1300 expression vector under the control of cauliflower mosaic virus 35S promoter (CaMV 35S) with KpnI and XbaI linkers using the primers GGGGTACCATGCTTTGTAGTTCTTCAACTAGAT, and GCTCTAGATCACGCAACCCCCAGCAGTTTCTTA. WT flowers of Arabidopsis (ecotype Columbia) were transformed with Agrobacterium tumefaciens GV3101 harboring CaMV35S:RcGPX4 via the floral dip method (Clough and Bent, 1998). Transformants were selected in a media supplemented with 25 mg/L hygromycin and then confirmed by PCR. The homozygous T3 lines were used for further phenotypic investigation.
Phenotypic Analysis of Transgenic Arabidopsis Plants
Seeds of transgenic plants and WT were sterilized with 5% NaClO and washed five times with distilled water. The sterilized seeds were stratified by dark incubation at 4°C for 3 days. For germination assay, the seeds were sown on 1/2 Murashige and Skoog (MS) and transferred into a growth chamber at 22°C as control, whereas grown at 4°C for cold treatment. Germinated seeds with protruded radicals were recorded for 13 consecutive days. The freezing tolerance assay was performed using 14-day-old seedlings, which were pre-treated at 4°C for 3 days, exposed to − 10°C for 5 h, thawed at 4°C for 12 h, and then recovered at 22°C for 5 days before counting survival rate.
Measurement of Physiological Parameters Between Wild Type and Overexpressed Plants
Fourteen-day-old seedlings of overexpressed lines (OE) and WT grew on 1/2 MS were treated at 4°C for 0, 1, and 2 days for physiological indices assays. The content of H2O2, MDA, reduced GSH, oxidized GSH (GSSG), activities of L-ascorbate peroxidase (APX), superoxide dismutase (SOD), catalase (CAT), peroxidase (POD) was measured using reagent kits from Nanjing Jiancheng Bioengineering Institute of Jiangsu Province, China (Cat. nos. A064-1, A003-3, A061-1, A123-1-1, A001-1, A007-1-1, and A084-3-1). The activities of glutathione reductase (GR) and dehydroascorbate reductase (DHAR) were assayed by Suzhou Comin Biotechnology Co., Ltd., kits (Cat. nos. GR-2-W, DHAR-2-W).
RNA Extraction and Expression Pattern Analysis
Three-week-old castor seedlings (genotype Tongbi5) planted in a growth chamber were subjected to different stresses, such as salt (300 mM), cold (4°C), and drought [15% Polyethylene glycol (PEG)] treatments. Total RNAs were extracted from leaves, stems, and roots at 0 and 8 h of stress treatments. The seeds of WT and OE lines imbibed for 1 day at 22°C and 8 days at 4°C were harvested and used for key genes expression involved in seed germination. The leaves of 4-week-old plants exposed to 4°C for 0, 30 min, 1 h, and 3 h were utilized for comparing the expression of cold stress-induced marker genes between WT and OE lines. Total RNA extraction, first-strand cDNA synthesis, and qRT-PCR were performed as described by Wang et al. (2019). The relative expression levels were calculated according to the 2–ΔΔ Ct method (Livak and Schmittgen, 2001). RcActin (XM_002522148) and RcGAPDH (XM_002513282) were used as reference genes. The values are the means ± SD. The P-value < 0.05 of the Student’s t-test was considered to be statistically significant. The primers used in this study are presented in Supplementary Table 2.
Results
Identification of RcGPXs in the Ricinus communis Genome
Based on an extensive search in the R. communis genome database using the GPX domain and known GPXs of other plant species as Protein BLAST (BLASTp) queries, five GPXs were identified and designated as RcGPX1 to RcGPX5 according to the order of their gene locus (Table 1). The length of the RcGPXs was varied among members, ranging from 167 to 265 amino acids with an average of 201. The deduced molecular weights (MWs) and PIs of the five RcGPXs were 18.81–29.48 kDa and 4.97–9.20, respectively. Subcellular localization prediction indicated that RcGPX3 and RcGPX4 were localized in chloroplast/mitochondria, RcGPX2 and RcGPX5 were located in the cytoplasm, and RcGPX1 was located in cytoplasm/extracellular.
To analyze the properties of the five RcGPXs, the RcGPXs were aligned to identify the conserved residues within the families from the castor bean, cucumber, Arabidopsis, and rice using ClustalW. The results showed that three conserved Cys residues were identified in the members of the GPX family, and three conserved domains, GK(R)V(A/T/P)L(M)L(I)V(I)VNVASR(K/Q/E)CG, ILAFPCNQF and WNFT(E/S/A)KF were found in the GPXs of most plants. Several highly conserved residues, namely, Cys (C, known as a catalytic site), Gln (Q), Trp (W), and Asn (N), were identified in all of the aligned sequences, which might form a potential redox center (Figure 1).
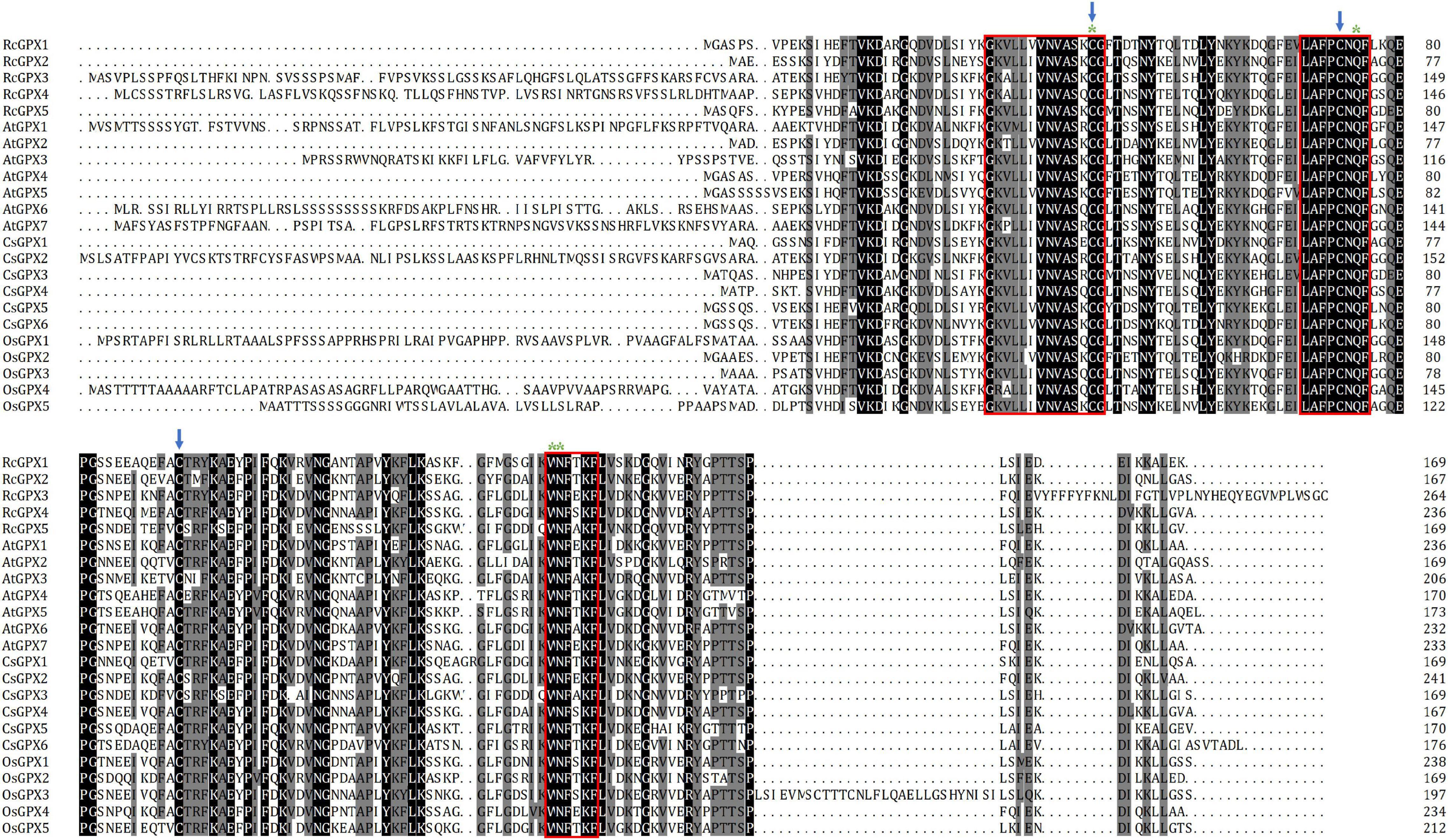
Figure 1. Multiple sequence alignments of the GPXs from the castor bean, cucumber, Arabidopsis, and rice. The three conserved domains found in most animal and plant GPXs were indicated with red boxes; the three conserved Cys residues of the plant GPX proteins were indicated with blue arrows. The amino acid residues that formed a potential redox center were indicated with asterisks. GPXs, glutathione peroxidases.
Gene Structures and Evolutionary Relationships Analyses of Glutathione Peroxidases in Castor Bean and Other Plant Species
To further investigate the evolutionary relationships among the GPXs, MEME analysis was used to identify the conserved motifs of GPXs from the castor bean, Arabidopsis, and rice. A total of five conserved motifs were found (Figure 2A and Supplementary Table 3). Motifs 1–4 were 50 amino acid residues, of which motifs 1–3 contained three conserved domains which are presented in Figure 1 separately, whereas motif 5 was 6 residues. Motifs 1 and 2 were associated with the GPX domain (PF00255) and existed in all tested GPX homologs. Motif 5, which was located in the N-terminal region, was present in all GPXs but absent in AtGPX3, AtGPX6-7, and RcGPX3. Interestingly, motif 4 was specific to AtGPX1, AtGPX6, and RcGPX3. Although some GPXs contained additional motifs, most interspecies GPXs shared similar motifs, which suggested that the structure and function of most GPXs may have been conserved in diverse plant species during evolution.
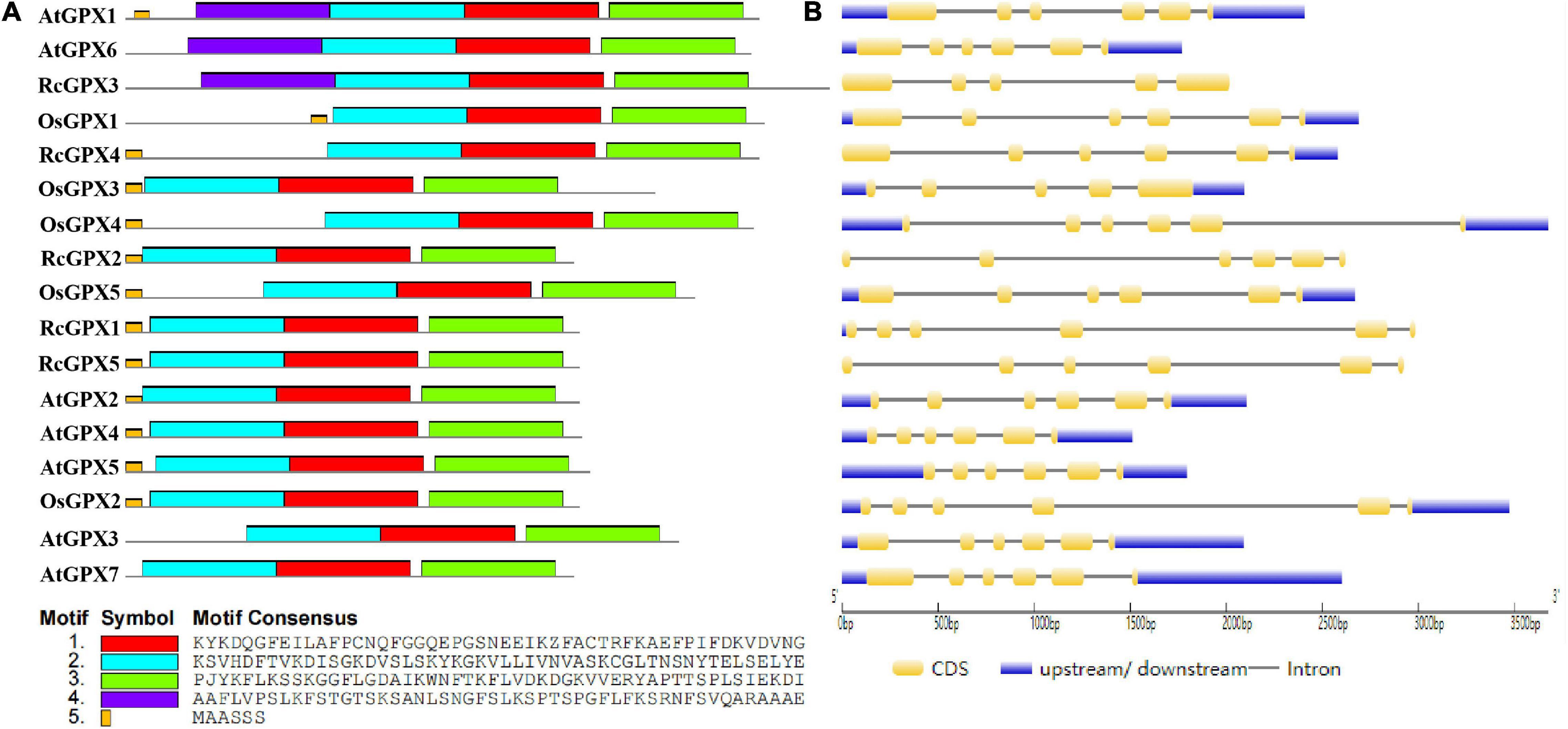
Figure 2. Conserved motifs and gene structures of GPXs from the castor bean, rice, and Arabidopsis. (A) Conserved motifs were identified by MEME, and their distribution was presented by different colors. (B) Exon/-intron organization was analyzed by GSDS software. The yellow round-corner rectangles indicated exons, the black lines indicated introns, and the blue rectangles indicated untranslated regions. GPXs, glutathione peroxidases; GSDS, Gene Structure Display Server.
To determine gene structure diversity, alignments of genomic sequences against CDS were carried out by the online GSDS analysis tool (Figure 2B). All of the open reading frames (ORFs) of the five RcGPXs contained six exons and five introns except RcGPX3, which harbored five exons and four introns. Although the lengths of introns were variable, all RcGPXs showed the same lengths in exons 2–5 except RcGPX3 that showed identical sizes in exons 2–4 with other RcGPXs. Notably, Some GPXs in the three species had a similar gene structure and comparable ORF lengths although they had variable lengths in 5′ or 3′ untranslated regions. Such features were also reported in C. sativus, G. hirsutum, and T. salsuginea (Gao et al., 2014; Chen et al., 2017; Zhou et al., 2018). These results revealed a significant degree of conservation of the GPXs family in the exon-intron arrangement in diverse plant species.
The Bayesian method was constructed from thirteen 13 plant species, including such as 75 GPX homologs to explore their phylogenetic relationship (Figure 3). Combined with the prediction of CELLO, WoLF PSORT, and TargetP subcellular localization servers, plant GPXs were clustered into five groups: mitochondria and chloroplast localized GPXs, cytoplasm localized GPXs, cytoplasm/extracellular/plasma membrane localized GPXs, cytoplasm/mitochondria/chloroplast localized GPXs, and cytoplasm/extracellular/nucleus localized GPXs (Supplementary Table 4). RcGPXs were clustered into five phylogenetic groups and did not display a conserved clustering pattern along with the GPXs of diverse plant species. RcGPX1 was clustered within the subgroup of S. lycopersicum SlGPX4, RcGPX2 was clustered within the P. trichocarpa PtGPX4, RcGPX3 was categorized into the subgroup of P. dactylifera PdGPX4, RcGPX4 was classified along with PdGPX2/5, and RcGPX5 was clustered within the subgroup of ClGPX1 and CsGPX3.
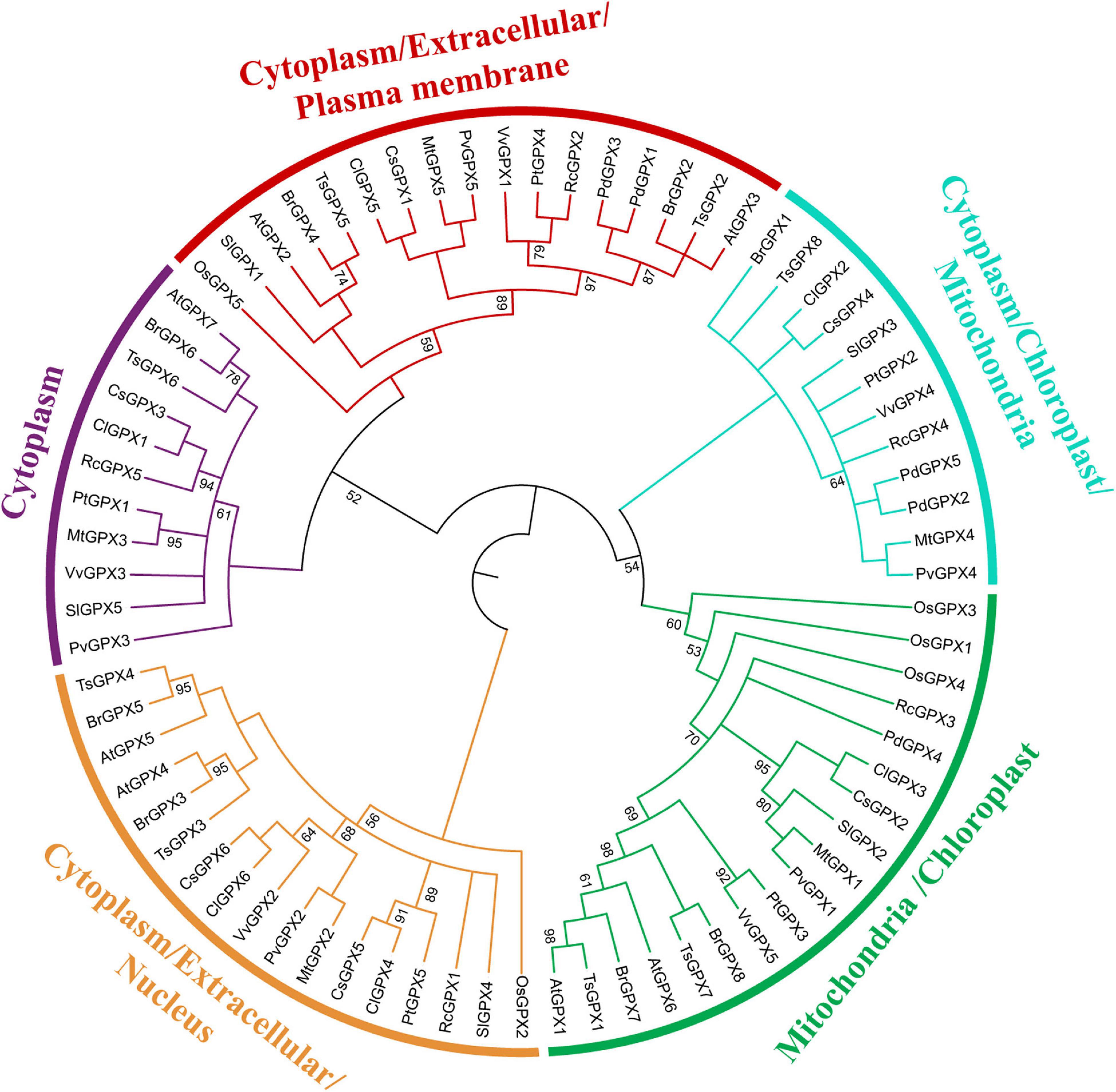
Figure 3. Phylogenetic tree of 75 GPX protein sequences based on 166 positions. The numbers at some branches indicated the posterior probability (posterior probability > 99 was not displayed). The tree topology as shown here was identical in the Bayesian inference (BI) analyses. The different colors of each branch indicated the different groups. GPXs, glutathione peroxidases.
Cis-Acting Regulatory Elements Analysis of RcGPX Promoters
Numerous studies have revealed that GPXs are responsive to a variety of environmental stresses and play critical roles in protecting plants from environmental stresses (Miao et al., 2006; Gaber et al., 2012). Therefore, 1,500 bp sequences upstream from the translational start site of each gene were analyzed to identify cis-regulatory elements potentially important for differential transcriptional regulation of the RcGPXs. Diverse cis-acting regulatory elements were discovered and their quantity differed in each RcGPX promoter (Supplementary Table 5). Nearly all RcGPX promoters contained cis-acting elements responsive to abscisic acid (ABA) and drought and anaerobic stresses; some RcGPX promoters included elements responsive to salicylic acid (SA) and gibberellin acid (GA), whereas a few were related to low temperature, methyl jasmonate (MeJA), wound, auxin, and defense and stress responsiveness (Table 2). These findings suggested that the RcGPX genes might be associated with responses to different abiotic stresses and plant hormones.
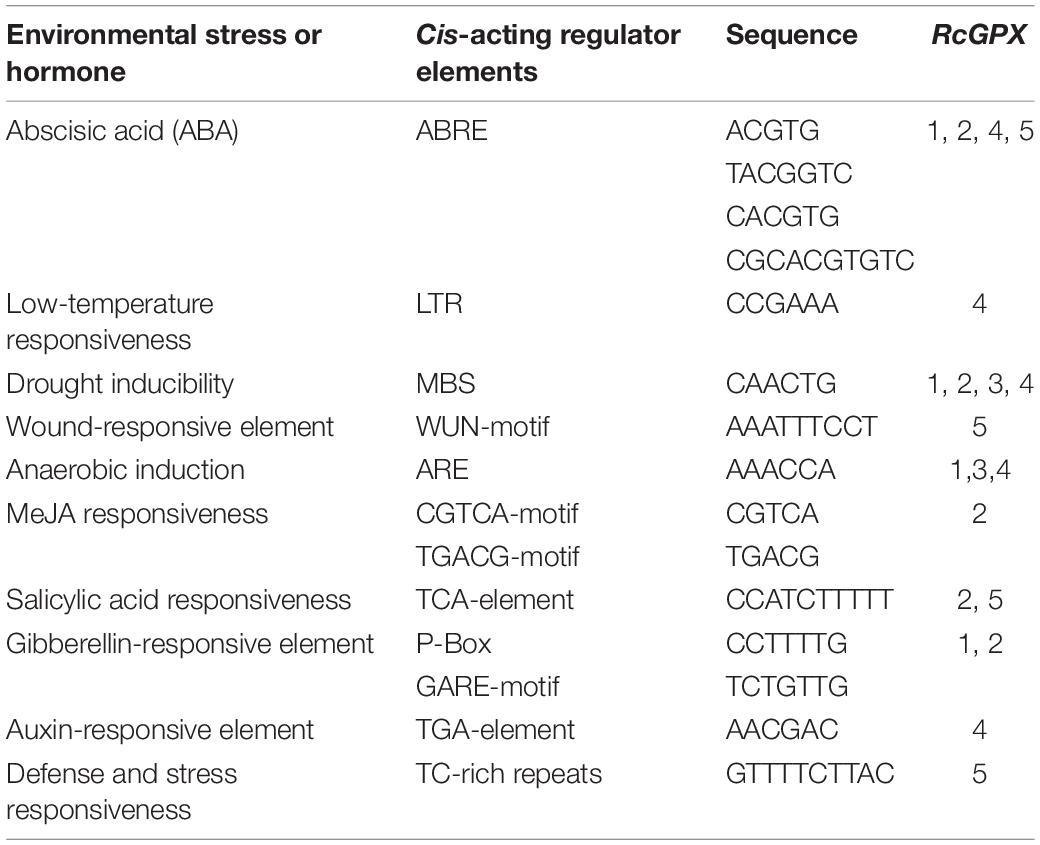
Table 2. Putative cis-acting regulatory elements associated with stress and hormone responses in RcGPX promoters.
Expression Analyses of the RcGPXs in Response to Various Abiotic Stresses
A growing body of evidence indicated that GPXs can protect the cells from damage caused by abiotic stresses (Roxas et al., 2000; Islam et al., 2015b; Zhang et al., 2019). Since stress-responsive cis-acting elements existed in the promoters of the RcGPXs, qRT-PCR was performed to investigate the expression change of RcGPXs under cold, drought, and salt stresses (Figure 4). Short-term exposure of R. communis plants to cold stress suppressed or induced the expression of some RcGPXs, and the effect differed among tissues, such as leaves, roots, and stems. Compared to the unstressed samples, the expression of all RcGPXs was not significantly changed under cold stress in leaves, while that of the only RcGPX5 was significantly downregulated in roots, and RcGPX2 and RcGPX4 were dramatically upregulated in stems after cold treatment. The expression of RcGPXs was also regulated by salt and drought stresses. Salt stress (300 mM NaCl) highly increased the transcript level of RcGPX5 and decreased the expression of RcGPX1 and RcGPX3 in leaves. In salt-treated stems, the expression of RcGPX2 was downregulated and that of RcGPX3 was upregulated. No obvious change in the abundance of all RcGPXs was observed in roots after salt stress. Drought stress induced the expression of RcGPX3 and RcGPX4 in leaves and stems, respectively. However, the transcript level of RcGPX2 was decreased in roots after drought treatment.
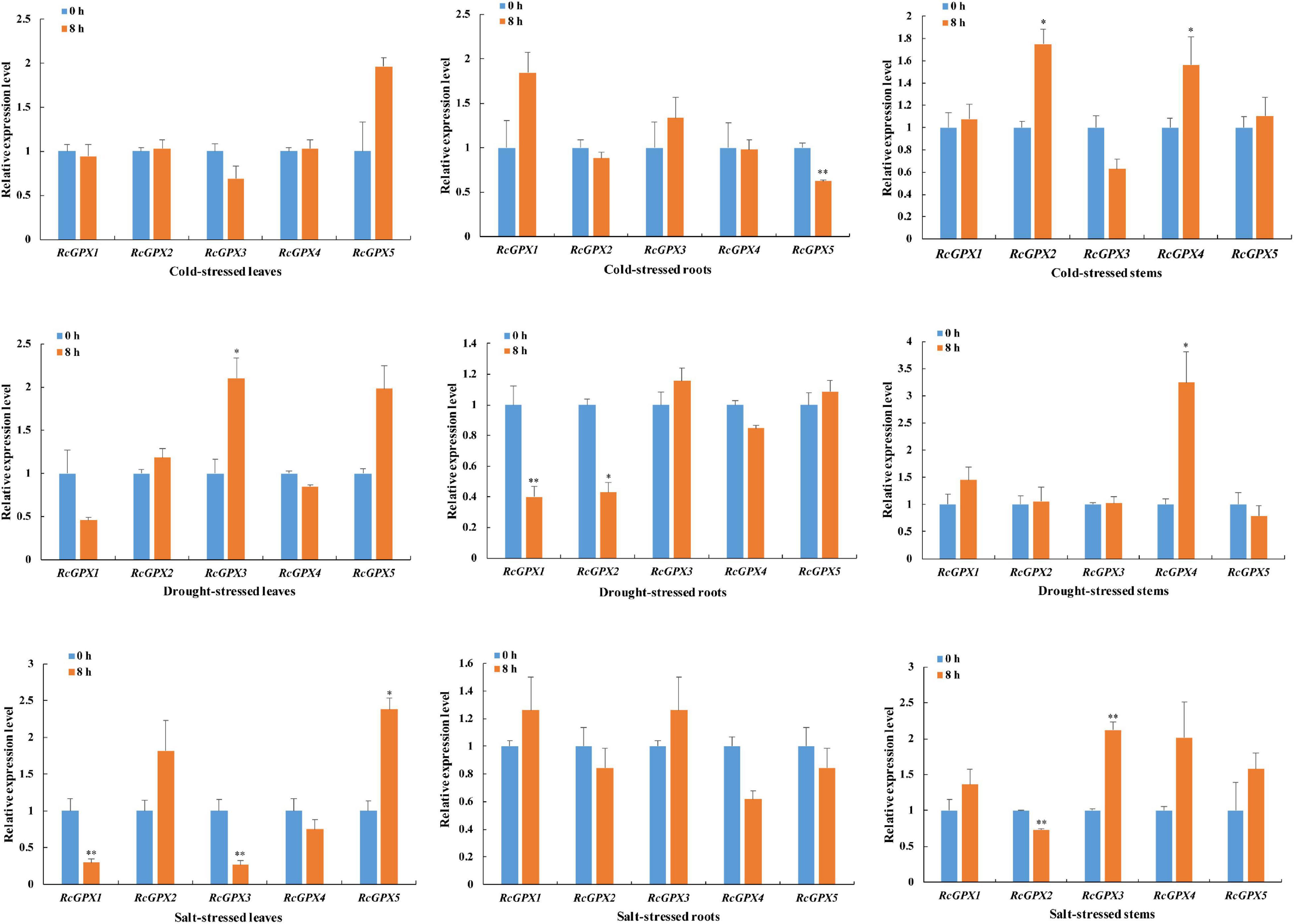
Figure 4. Effect of different stresses on the expression of the RcGPXs in leaves, roots, and stems. Total RNA was extracted from the leaves, stems, and roots of 3-week-old castor seedlings subjected to salt (300 mM), cold (4°C), and drought (15% PEG) treatments at 0 and 8 h. 0 h treated plants were used as controls. Data represent the means ± SD of three independent biological replicates. ∗P < 0.05, ∗∗P < 0.01 by Student’s t-test.
Overexpression of RcGPX4 Enhanced Cold Tolerance at Seed Germination but Reduced Freezing Tolerance at Seedlings
Our previous study showed that the protein abundance of RcGPX4 was increased in cold-stressed imbibed seeds (Wang et al., 2019). To test whether RcGPX4 might be associated with cold tolerance, three T3 homozygous transgenic lines were selected to investigate the effect on the phenotype. No difference in seed germination was observed between transgenic plants and WT under normal conditions (Supplementary Figure 1). When the seeds sown on 1/2 MS were exposed to cold stress, transgenic seeds began to germinate at day 7 whereas WT seeds were delayed 1 day. Moreover, the germination rate of OE lines was significantly higher than that of WT, which indicated that RcGPX4 positively regulated seed germination of transgenic plants under cold stress (Figure 5). The freezing tolerance assay was done using 14-day-old seedlings. Surprisingly, after freezing stress, a higher survival rate was observed in WT compared with transgenic plants. The survival rate of WT was about 80% whereas that of OE lines was between 40 and 52%, which suggested that RcGPX4 negatively mediated seedlings of transgenic plants in response to freezing treatment (Figure 6).
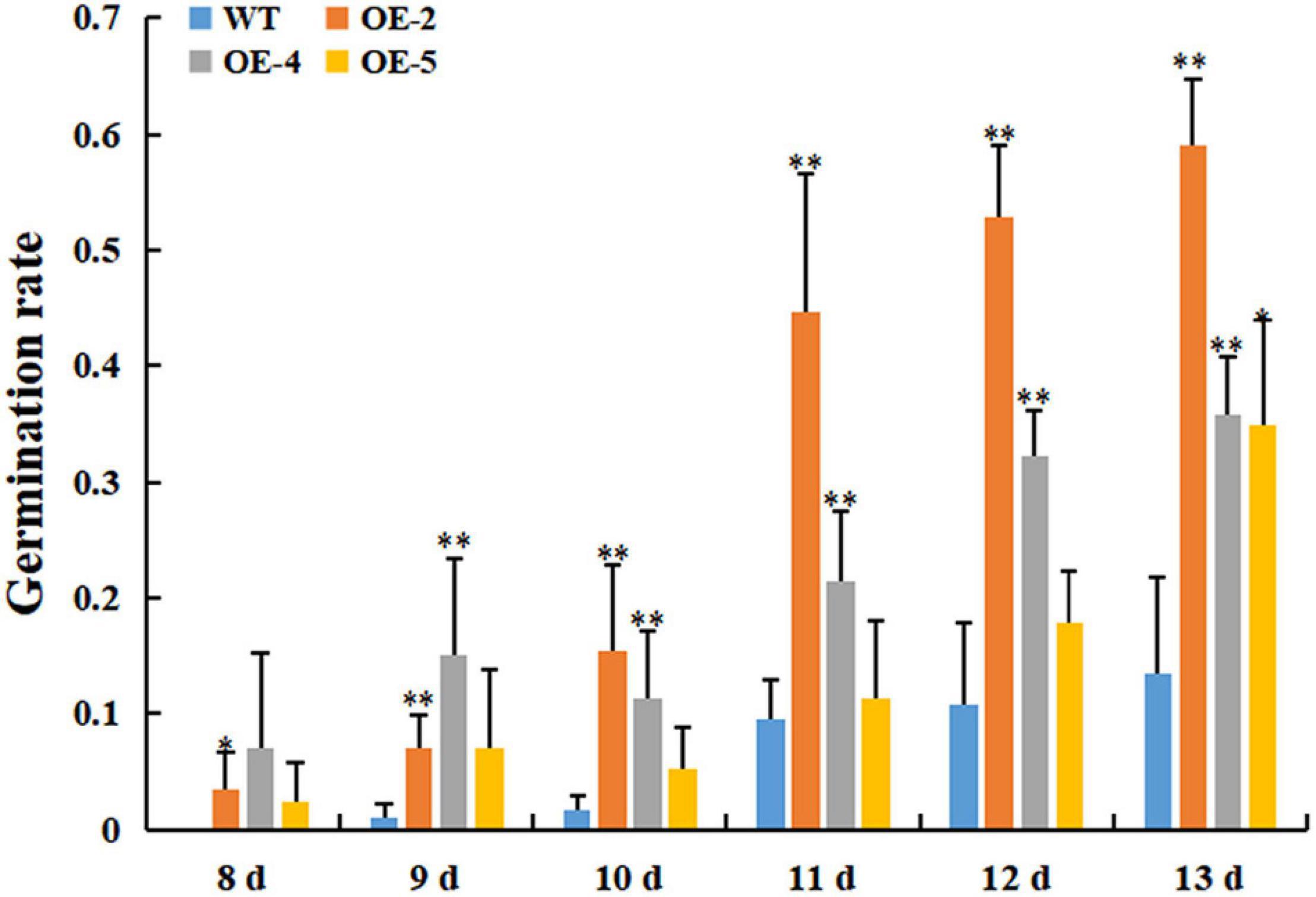
Figure 5. Overexpression of RcGPX4 increased seed germination in transgenic plants under cold stress. The surface-sterilized seeds of wild type and transgenic plants were sown on 1/2 MS media and grown at 4°C for 13 consecutive days. Data represent the mean ± SD of three independent biological replicates (n = 42 seeds for each replicate). Asterisks indicate a significant difference between wild type and overexpressed lines (∗P < 0.05, ∗∗P < 0.01, student’s t-test). WT: wild-type; OE-2, OE-4, and OE-5: three T3 homozygous transgenic lines.
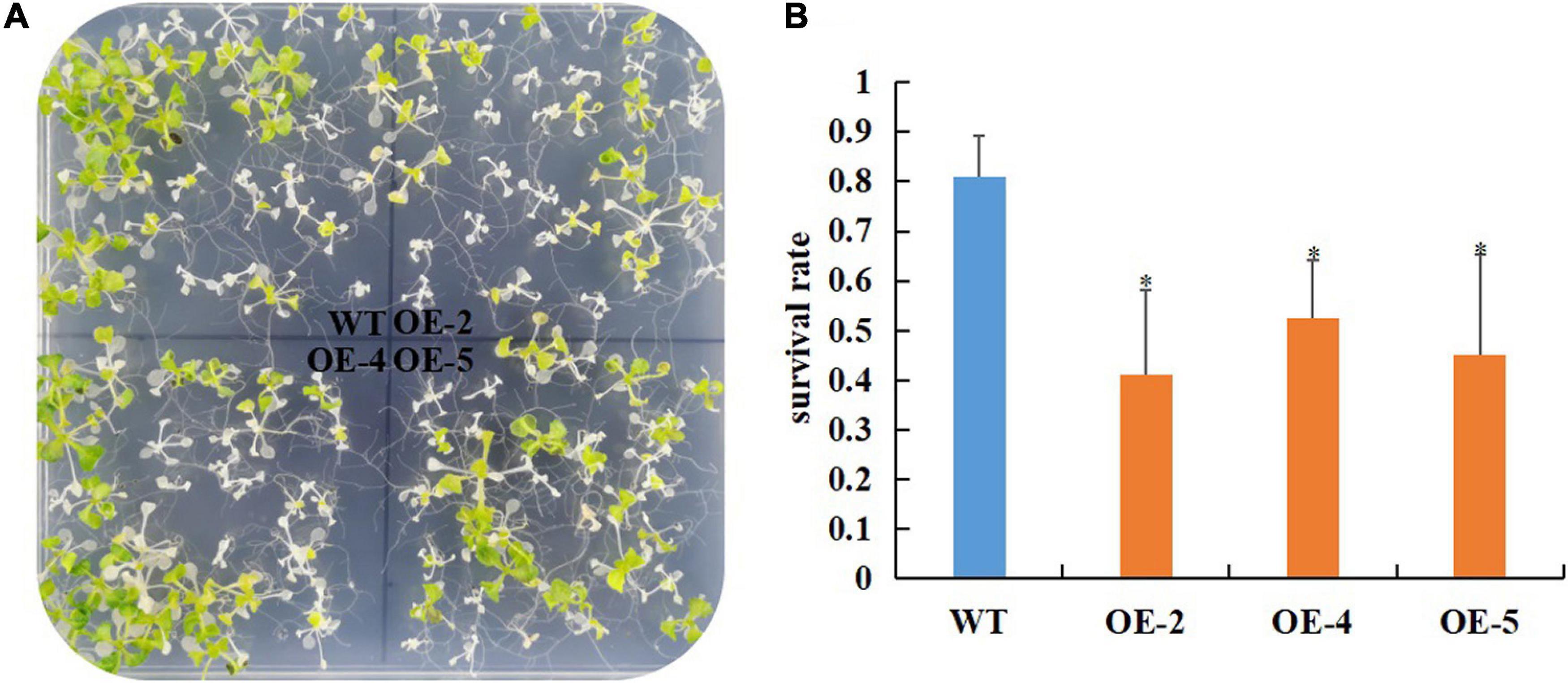
Figure 6. Overexpression of RcGPX4 reduced freezing tolerance in transgenic plants. Freezing phenotype (A) and survival rate (B) of RcGPX4 overexpressing transgenic plants. Fourteen-day-old seedlings grown 1/2 MS at 22°C were pre-treated at 4°C for 3 days, subjected to freezing stress at –10°C for 5 h, and then recovered at 22°C for 5 days before counting survival rate. Data represent the mean ± SD of three independent biological replicates (n = 42 seedlings for each replicate). Asterisks indicate a significant difference between wild type and overexpressed lines (∗P < 0.05, student’s t-test). WT: wild-type; OE-2, OE-4, and OE-5: three T3 homozygous transgenic lines.
Transgenic Plants Produced More H2O2 and Lipid Peroxidation Compared With Wild Type
Since GPX can act as an important detoxifier of H2O2, the amount of H2O2 was assayed between WT and transgenic lines. The content of H2O2 was significantly higher in transgenic lines than in WT under normal and cold stress conditions (Figure 7A). The alteration in the production of lipid hydroperoxide triggered by ROS can be determined by the level of MDA. The transgenic plants showed more accumulation of MDA compared with WT under cold stress, which suggested a higher degree of lipid peroxidation in transgenic plants (Figure 7B). Considering the surprising discovery that transgenic plants can accumulate more H2O2, we hypothesized that overexpression of RcGPX4 might disturb the H2O2 homeostasis through the modulation of other antioxidant enzymes. In normal conditions, the activities of SOD and POD were not significantly different between WT and OE plants (Figures 7C,D). However, the activities of CAT and APX were obviously decreased and increased separately as compared to WT (Figures 7E,F). Under cold stress, the activity of SOD was significantly enhanced, the activities of POD and CAT were obviously decreased, while APX activity did not change noticeably in OE plants as compared to WT. To further investigate whether overexpression of RcGPX4 can affect the antioxidant enzymes and compounds involved in the GSH-ascorbate cycle, we assayed the content of GSH and GSSG in WT and OE plants (Figures 7G,H). Under cold-stressed conditions, the content of reduced GSH and GSH/GSSG ratios was higher in transgenic plants than in WT (Figures 7G,I). The activity of GR significantly increased in OE lines in both conditions, while the activity of DHAR decreased after cold stress but did not display the obvious difference in the control condition (Figures 7J,K).
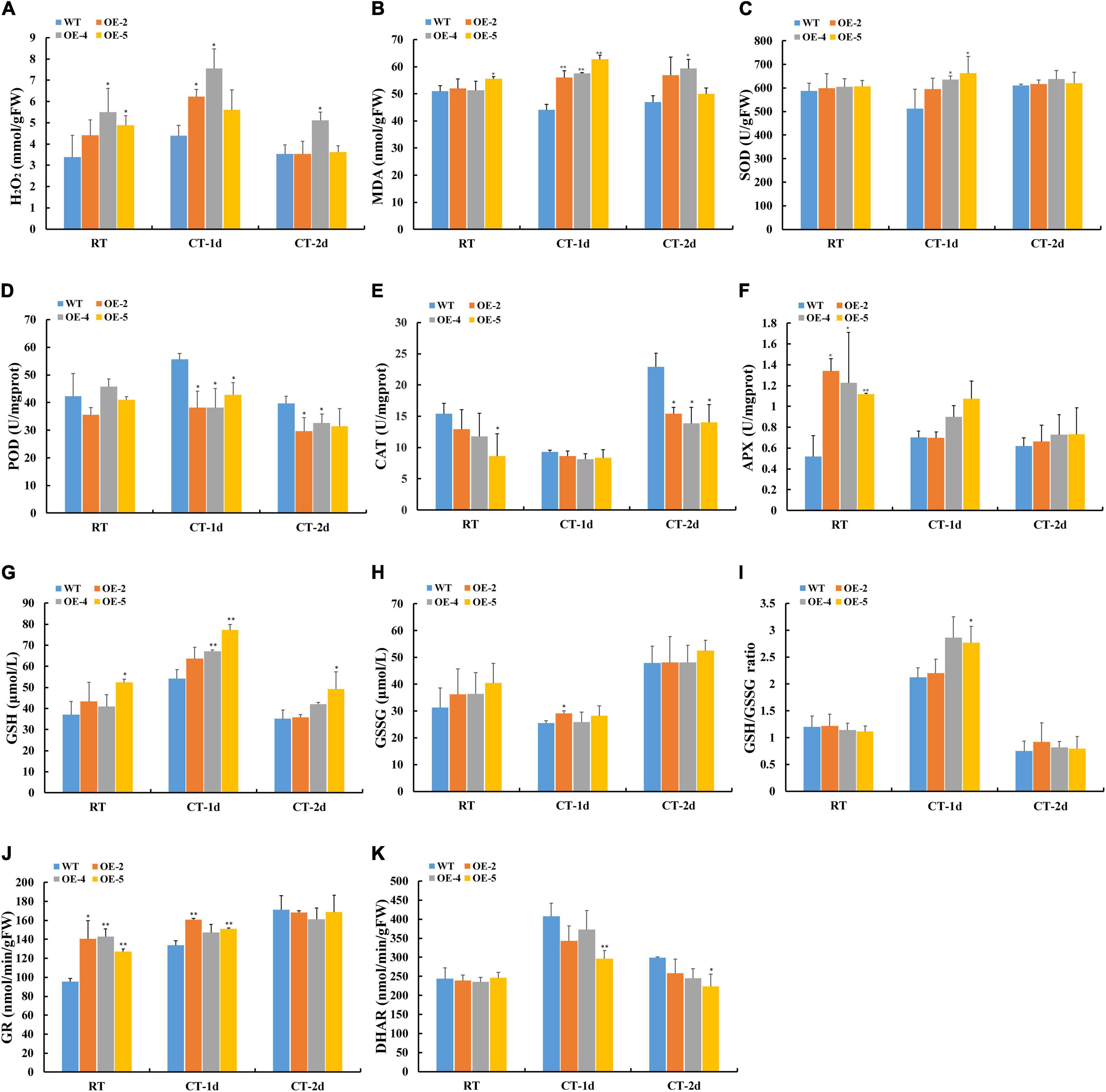
Figure 7. Effect of RcGPX4 overexpression on metabolic content and the activities of antioxidant enzymes between WT and transgenic plants. (A) H2O2 content; (B) MDA content; (C) SOD activity; (D) POD activity; (E) CAT activity; (F) APX activity; (G) reduced GSH content; (H) GSSG content; (I) GSH/GSSG ratio; (J) GR activity; and (K) DHAR activity. RT: room temperature (22°C); CT-1: cold temperature (4°C) for 1 day; CT-2: cold temperature (4°C) for 2 days. FW: fresh weight. Data represent the mean ± SD of three independent biological replicates. Asterisks indicate a significant difference between wild type and overexpressed lines (∗P < 0.05, ∗∗P < 0.01, student’s t-test). WT, wild type; H2O2, hydrogen peroxide; MDA, malondialdehyde; SOD, superoxide dismutase; CAT, catalase; POD, peroxidase; GSSG, oxidized GSH; APX, activities of L-ascorbate peroxidase; GSH, glutathione; GR, glutathione reductase; DHAR, dehydroascorbate reductase.
Effect of RcGPX4 Overexpression on the Expression of ABA/GA/CTK Pathway-Related Genes
Since RcGPX4 positively regulated the seed germination of transgenic lines under cold stress, we examined the expression of ABA/GA/CTK pathway-related genes in imbibed seeds of OE lines and WT under control condition and cold treatment. The results showed that the transcript of AtABI4 in OE lines was significantly lower than in the WT under cold stress, whereas no obvious alteration was observed under the control condition. The expression level of AtABI5 and AtGA2ox7 was lower in OE lines compared with WT under both conditions. AtCYP707A1 of OE lines showed a higher expression level than that of WT under both conditions. Compared with WT, a decrease in AtCYP707A2 expression of OE lines was observed in both conditions, although it exhibited no significant difference in the presence of cold stress. Interestingly, the transcriptional expression of AtARR6 in OE lines was decreased under control conditions but was increased under cold stress compared with WT (Figure 8). These results demonstrated that RcGPX4 can confer cold tolerance of transgenic lines through the alteration of ABA/GA/CTK pathway-related genes under cold stress.
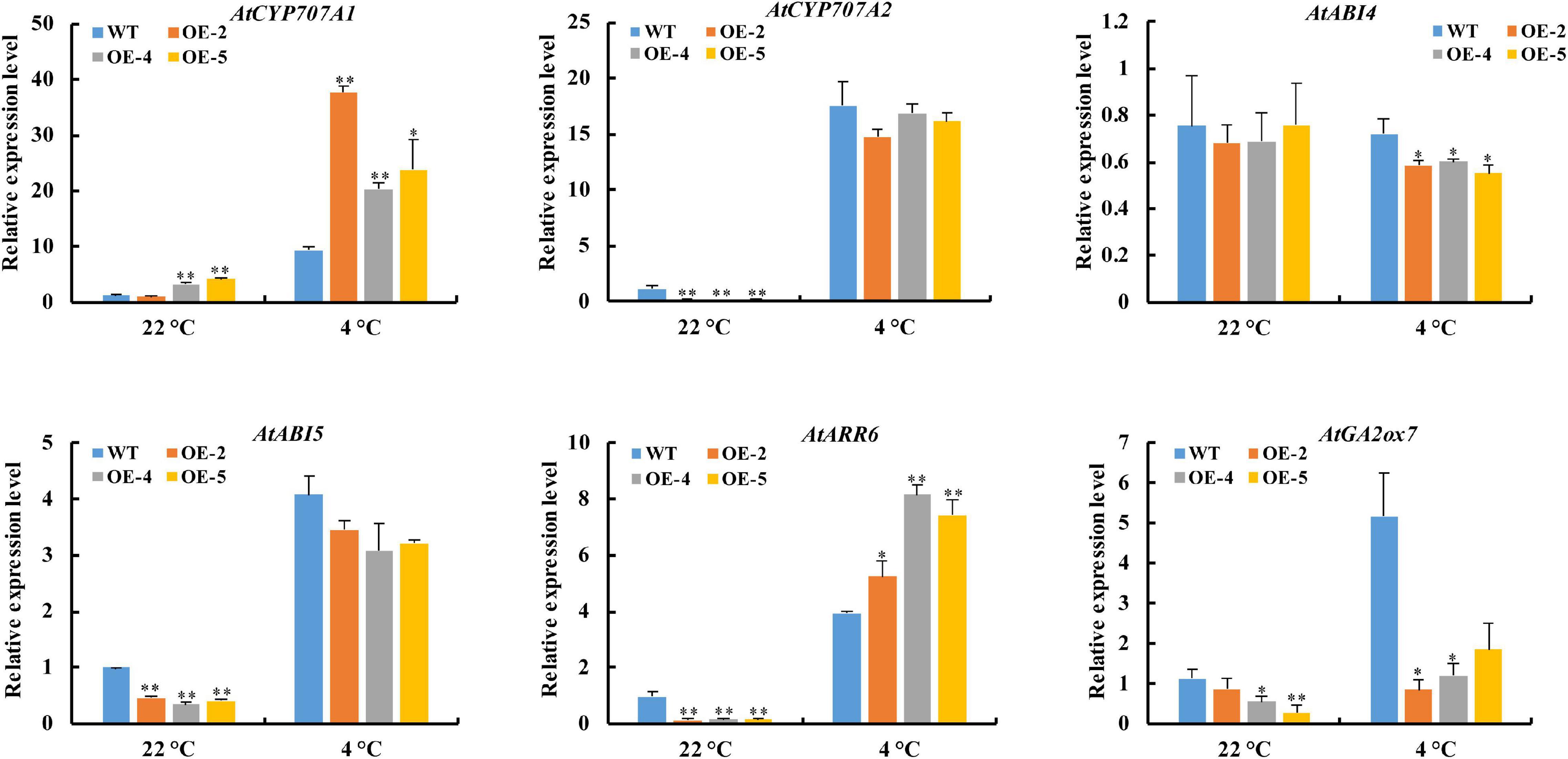
Figure 8. Expression pattern of ABA/GA/CTK pathway-related genes regulated by RcGPX4. The seeds of WT and transgenic lines imbibed for 1 day at 22°C and 8 days at 4°C were harvested for RNA extraction and qRT-PCR analysis. Data represent the mean ± SD of three independent biological replicates. Asterisks indicate a significant difference between wild type and overexpressed lines (∗P < 0.05, ∗∗P < 0.01, student’s t-test). ABA, abscisic acid; GA, gibberellin acid; CTK, cytokinin.
Effect of RcGPX4 Overexpression on the Expression of C-Repeat-Binding Factor-Dependent and ABA-Dependent Pathway Genes
To further explore the molecular mechanism of the reduced freezing tolerance regulated by RcGPX4 in transgenic seedlings, we investigated the expression level of cold-responsive genes involved in CBF-dependent and ABA-dependent pathways. After cold treatment, the expression of AtMAPK3 was significantly upregulated in OE lines compared with WT. Meanwhile, the expression of AtICE1, AtCBF1, AtCBF2, AtCOR47A, AtKIN, AtRD29A, AtABI1, AtABI2, and AtRAB18 in transgenic plants was strikingly lower than in WT. These results suggested that RcGPX4 played a negative role in freezing tolerance by suppressing the genes expression of the ICE1-CBF-COR cascade3 and ABA signaling pathways (Figure 9).
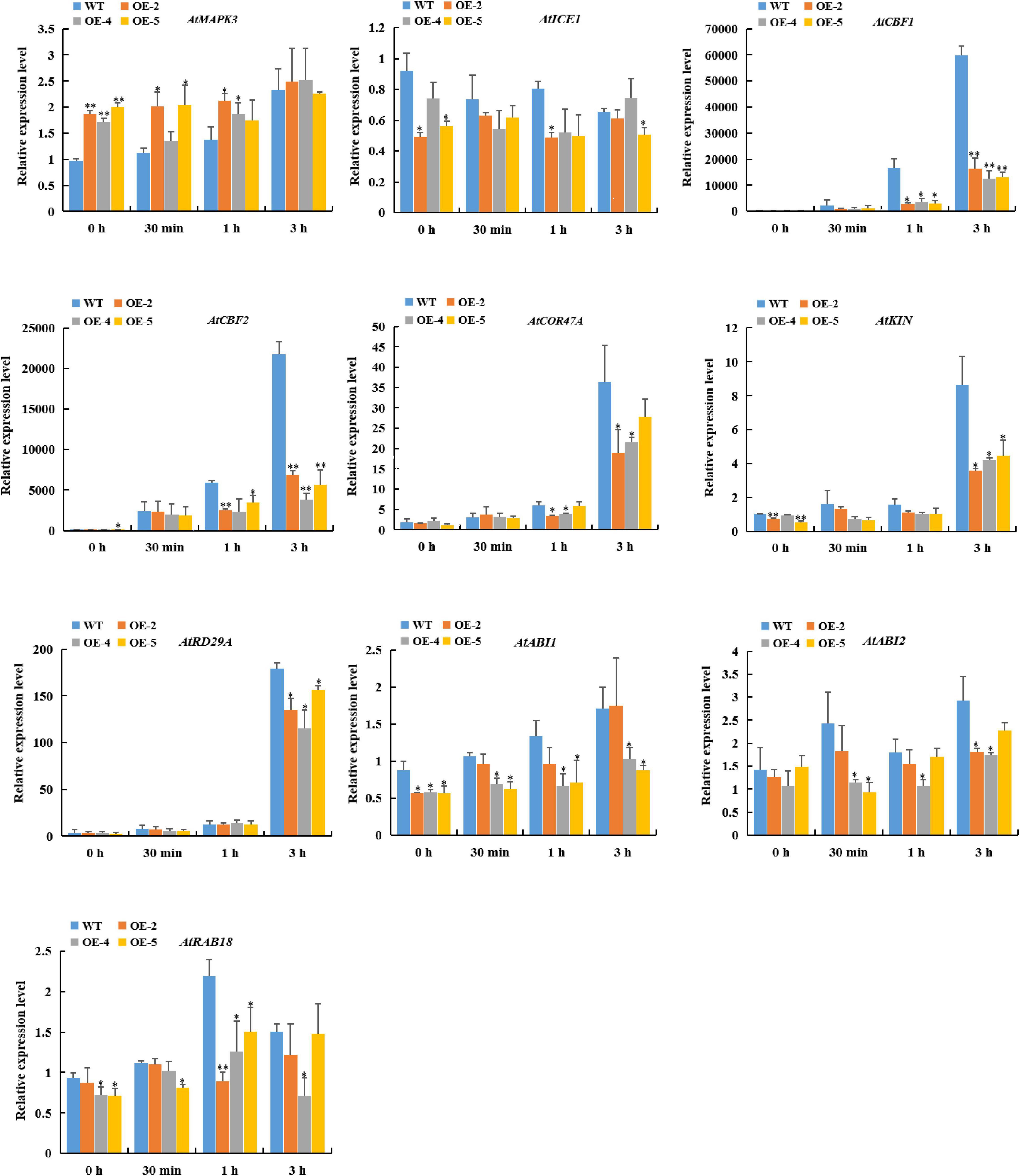
Figure 9. Expression pattern of cold-induced marker genes in WT and transgenic lines subjected to cold stress (4°C) for the indicated times. Data represent the mean ± SD of three independent biological replicates. Asterisks indicate a significant difference between wild type and overexpressed lines (∗P < 0.05, ∗∗P < 0.01, student’s t-test). WT, wild type.
Discussion
Unfavorable environmental stimulation can alter cellular redox homeostasis, which causes the excessive generation of ROS. Plants have evolved enzymatic and non-enzymatic antioxidants to neutralize excess ROS. GPXs, as efficient ROS scavengers, can defend plants against oxidative injury. Herein, a total of five GPXs were identified from the current R. communis genome. The number of RcGPXs was comparable to that in rice (five members), L. japonicus (six members), cucumber (six members), and A. thaliana (eight members). In our study, one tandem duplication event (RcGPX4 and RcGPX5) was observed, which might be conducive to the expansion of the RcGPX gene family. The five RcGPXs harbored four highly similar motifs, which were also identified in all GPXs of other plant species (Zhou et al., 2018; Jana and Yaish, 2020). This indicates that the structure and function of GPXs from different plant species may have been conserved during evolution. A few conserved motifs were categorized into specific groups of GPXs, indicating that these motifs might be associated with the specific functions of the GPXs. Gene structure also showed that the GPXs from different species were highly conserved due to similar exon-intron organization and ORF lengths, which suggested that exon-intron arrangement played a critical role in the evolution of multigene families (Cao et al., 2016). The phylogenetic tree showed that GPXs from 13 plant species were classified into five groups based on predicted subcellular compartment, which was supported by the classification in other plants (Ozyigit et al., 2016). Margis et al. (2008) indicated that GPX clustered into the same subcellular compartments may be more associated with each other than GPXs with different localization from the same organism. Our results also showed that cytosolic localized GPXs were clustered together while chloroplast/mitochondria localized GPXs were categorized together. Ozyigit et al. (2016) suggested that the presence or absence or position of transit peptide sequences located in the N-terminal site of GPXs mainly determine the phylogenetic distribution. However, it will be needed to investigate whether GPXs, clustered into the same subcellular compartment branches, are positioned to predicted location and performed a similar function. Five RcGPXs were distributed into five phylogenetic groups, and each RcGPX was closer to GPX either in dicots or monocots, such as RcGPX3 and PdGPX4, RcGPX1 and SlGPX4, which indicated that GPX may not evolve separately in monocots and dicots. This observation was supported by phylogenetic analysis of P. dactylifera PdGPXs, which showed that PdGPX2, 4, 5 were highly related to homologs in G. hirsutum (Jana and Yaish, 2020).
A body of studies suggested that plant GPXs acted as crucial regulatory roles in response to diverse environmental factors. For example, the expression of rice OsGPX2 and OsGPX4 was upregulated after drought and oxidative stresses but downregulated under salt and cold stresses (Islam et al., 2015a). In cucumber, the transcript level of most CsGPXs was downregulated under salt and drought stresses (Zhou et al., 2018). Analysis of RcGPX promoter sequences showed that diverse cis-acting regulatory elements, such as environmental stress binding sites, were discovered and their quantity differed in each RcGPX promoter (Supplementary Table 5), which implied their potential roles in abiotic stresses. Therefore, we explored the responses of the RcGPXs to diverse stresses, such as cold, drought, and salt, in different tissues. The expression of RcGPXs varied largely under these stresses. At least one member of RcGPXs was significantly regulated in at least one tissue under specific environmental conditions except that all RcGPXs remained unaltered in cold-stressed leaves and salt-stressed roots. In addition, RcGPX2 was upregulated in response to salt stress in leaves, whereas the transcript level for RcGPX3 and RcGPX5 was downregulated. This indicated that these members might act antagonistically to avoid a decrease in the reduced GSH or Trx pool.
Seed imbibition can rapidly generate H2O2 due to high respiratory activity and O2 depletion (Cakmak et al., 1993). Our proteomics study showed that the abundance of RcGPX4 was up-accumulated in the cold-stressed imbibed castor seeds compared to unstressed control, which might contribute to detoxify overproduction of H2O2 (Wang et al., 2019). This study displayed that overexpression of RcGPX4 was insensitive to cold stress in the germination stage. It has been reported that H2O2 can interplay with the phytohormones, such as ABA and GA, to regulate abiotic stress responses in plants (Jubany-Mari et al., 2009; Ishibashi et al., 2012). ABA, GA, and CTKs function antagonistically to modulate seed germination and dormancy (Piskurewicz et al., 2008; Guan et al., 2014). AtABI4 acts as a positive regulator of primary seed dormancy, which can inhibit the transcripts of AtCYP707A1 and AtCYP707A2 to promote ABA biosynthesis while contributing to GA degradation via the upregulation of AtGA2ox7 (Shkolnik-Inbar and Bar-Zvi, 2010; Shu et al., 2013, 2016). A recent study unraveled that AtABI4 can repress the transcription of type-A ARRs, one of CTK signaling components, and inhibit seed germination (Huang et al., 2017). In this study, the expression of AtABI4 and AtGA2ox7 was significantly downregulated while the transcription of AtCYP707A1 and AtARR6 was noticeably upregulated in cold-stressed imbibed OE seeds compared to unstressed control. Thus, we speculated that the improved seed germination of OE lines under cold stress was associated with the altered expression of ABA/GA/CTK pathway-related genes.
Cold stress can produce an increase in the amount of ROS, such as H2O2. The plant has evolved important physiological mechanisms, such as the GSH-ascorbate pathway, to cope with the damage caused by excessive H2O2. The accumulation and detoxification can be directly regulated by several enzymatic antioxidants. In our study, we surprisingly found that OE lines had higher H2O2 contents as compared to WT, which was not consistent with previous findings. After cold treatment, the transgenic plants had higher SOD activity but lower POD and CAT activities than WT. It was previously observed that transgenic plants overexpressed GPX had higher activities of SOD, CAT, and APX under drought and salinity stresses (Islam et al., 2015b; Zhang et al., 2019). APX activity was higher in transgenic seedlings than in WT under normal condition while was similar in both genotypes after cold stress. Roxas et al. (2000) showed that the elevated APX activity cannot substantially influence the general peroxide scavenging capacity. It seems to be a general phenomenon that overexpression of GPX can affect the activities of other antioxidant enzymes under abiotic stresses. However, based on our observation, the altered trend of antioxidant enzyme activities may depend on the function of different GPX family members. Therefore, an elevation of H2O2 in transgenic plants was mainly due to the decrease of CAT and POD activities and increase of SOD activity to cause the higher accumulation of MAD leading to lipid peroxidation and reduce tolerance to freezing stress. Moreover, we boldly speculated that maintenance of the high level of H2O2 regulated by the activities of different antioxidant enzymes may be needed as a signaling molecule to mediate the expression of cold-tolerant marker genes.
Most studies considered that plant GPX used Trxs as a reductant rather than GSH, which indicated that they were not relevant with GSH oxidation (Iqbal et al., 2006; Navrot et al., 2006). GSH can be oxidized to GSSG by DHAR, and GSSG can be recycled to GSH by GR (Rahantaniaina et al., 2017). Our results revealed that relatively high levels of GSH and GSH/GSSG in transgenic plants were controlled by the increased GR activity and the decreased DHAR activity, which might rebuild the new cellular redox balance in OE plants.
It is well-known that the ICE1-CBF-COR signaling pathway plays a critical role in plant cold acclimation. ICE1 can activate the transcription of CBF genes under cold stress, and CBF subsequently can induce the gene expression of CORs by directly binding their promoters, which contribute to plant freezing tolerance (Stockinger et al., 1997; Liu et al., 1998; Chinnusamy et al., 2003). However, this best characterized cold stress signaling pathway was regulated by diverse components, such as protein kinases, and phosphatases (Ding et al., 2019). Cold-activated mitogen-activated protein kinases MPK3 and MPK6 reduced ICE1 stability and transcriptional activity by phosphorylating ICE1 protein, which eventually decreased the CBF expression and plant freezing tolerance (Li et al., 2017). Besides the capacity to induce oxidative stress, H2O2 also can act as an important signaling molecule, which modulates the abiotic stresses tolerance by various pathways (Saxena et al., 2016). H2O2 can induce MPK3 and MPK6 via the activation of ANP1 (Kovtun et al., 2000). In this study, the expression level of MPK3 was significantly higher in OE plants compared to WT in both conditions. However, the transcriptional level of ICE1, CBFs, and downstream CORs were lower in OE lines than in WT under cold stress. These results indicated that RcGPX4 depended on the MAPK3-ICE1-CBF-COR signal transduction pathway to negatively modulate freezing tolerance in transgenic plants. A previous study unraveled that ATGPX3 might regulate ABA and oxidative signaling by interacting with ABI1 and ABI2 (Miao et al., 2006). ABI1/2 is involved in ABA signal transduction, and RAB18 is the ABA-induced marker gene (Yao et al., 2020). Yao et al. (2020) showed that CsbZIP18 reduced cold tolerance by suppressing the expression of AtABI1/2 and AtRAB18. Our results found that the AtABI1/2 and AtRAB18 were downregulated in OE lines under normal conditions and cold stress, which suggested that RcGPX4 impaired freezing tolerance in transgenic plants partly via the ABA-dependent pathway.
Conclusion
In conclusion, a total of five GPXs were identified in castor beans through genome-wide analysis. Their physicochemical characteristics, subcellular prediction, and cis-acting elements were investigated. A comparison of phylogenetic relationships, protein motifs, and gene structures revealed that the structure and function of most GPXs may have been conserved in diverse plant species during evolution. The expression of the RcGPXs varied largely, and some RcGPXs were coordinately regulated under cold, drought, and salt stresses. Overexpression of RcGPX4 in Arabidopsis decreased sensitivity to cold stress at the germination stage but showed hypersensitivity to freezing stress at seedlings. As compared to WT, transgenic plants can produce more H2O2 and lipid peroxidation, which was mainly due to the decrease of CAT and POD activities and the increase of SOD activity. The relatively high level of GSH and GSH/GSSG in transgenic plants was controlled by the increased GR activity and the decreased DHAR activity, which might rebuild the new cellular redox balance in OE plants. The improved seed germination in transgenic lines was associated with the alteration of ABA/GA/CTK pathway-related genes under cold stress. In addition, RcGPX4 played a negative role in freezing tolerance by suppressing the genes expression of ICE1-CBF-COR cascade and ABA signaling pathways.
Data Availability Statement
The original contributions presented in the study are included in the article/Supplementary Material, further inquiries can be directed to the corresponding authors.
Author Contributions
XW and JZ conceived and designed the experiments. XW and XL performed the experiments. XW, XL, Y-qA, HZ, DM, YJ, and HH analyzed the data. XW wrote the manuscript. JZ, Y-qA, HZ, DM, and LY reviewed and edited the manuscript. All authors contributed to the article and approved the submitted version.
Funding
This study was supported by the National Natural Science Foundation of China (Grant Nos. 32060493, 31701467, and 31760399), the Program for Young Talents of Science and Technology in the University of Inner Mongolia Autonomous Region (Grant No. NJYT-20-B25), and the Innovation and Entrepreneurship Startup Project for Overseas Scholar (Grant No. 2020QNCXRC07).
Conflict of Interest
The authors declare that the research was conducted in the absence of any commercial or financial relationships that could be construed as a potential conflict of interest.
Publisher’s Note
All claims expressed in this article are solely those of the authors and do not necessarily represent those of their affiliated organizations, or those of the publisher, the editors and the reviewers. Any product that may be evaluated in this article, or claim that may be made by its manufacturer, is not guaranteed or endorsed by the publisher.
Acknowledgments
We acknowledged Professor Ming Chen from the Zhejiang University for his assistance in the phylogenetic analysis.
Supplementary Material
The Supplementary Material for this article can be found online at: https://www.frontiersin.org/articles/10.3389/fpls.2021.707127/full#supplementary-material
Footnotes
- ^ https://phytozome.jgi.doe.gov/pz/portal.html
- ^ http://pfam.xfam.org/
- ^ ICE1 = inducer of CBF expression 1; COR = cold-responsive.
References
Agarwal, P. K., Agarwal, P., Reddy, M. K., and Sopory, S. K. (2006). Role of DREB transcription factors in abiotic and biotic stress tolerance in plants. Plant Cell Rep. 25, 1263–1274. doi: 10.1007/s00299-006-0204-8
Akpan, U., Jimoh, A., and Mohammed, A. (2006). Extraction characterization and modification of castor seed oil. Leonardo J. Sci. 8, 43–52. doi: 10.1074/jbc.M204802200
Armenteros, J. J. A., Salvatore, M., Emanuelsson, O., Winther, O., Heijne, G. V., Elofsson, A., et al. (2019). Detecting sequence signals in targeting peptides using deep learning. Life Sci. Alliance 2:e201900429. doi: 10.26508/lsa.201900429
Bailey, T. L., Boden, M., Buske, F. A., Frith, M., Grant, C. E., Clementi, L., et al. (2009). MEME SUITE: tools for motif discovery and searching. Nucleic Acids Res. 37, W202–W208. doi: 10.1093/nar/gkp335
Bela, K., Horvath, E., Galle, A., Szabados, L., Tari, I., and Csiszar, J. (2015). Plant glutathione peroxidases: emerging role of the antioxidant enzymes in plant development and stress responses. J. Plant Physiol. 176, 192–201. doi: 10.1016/j.jplph.2014.12.014
Blokhina, O., Virolainen, E., and Fagerstedt, K. (2003). Antioxidants oxidative damage and oxygen deprivation stress: a review. Ann. Bot. 91, 179–194. doi: 10.1093/aob/mcf118
Cakmak, I., Strbac, D., and Marschner, H. (1993). Activities of hydrogen peroxide scavenging enzymes in germinating wheat seeds. J. Exp. Bot. 44, 127–132. doi: 10.1093/jxb/44.1.127
Cao, Y., Han, Y., Jin, Q., Lin, Y., and Cai, Y. (2016). Comparative genomic analysis of the GRF genes in Chinese pear (Pyrus bretschneideri Rehd), poplar (Populous), grape (Vitis vinifera), Arabidopsis and rice (Oryza sativa). Front. Plant Sci. 7:1750. doi: 10.3389/fpls.2016.01750
Chang, C. C. C., Ślesak, I., Jordá, L., Sotnikov, A., Melzer, M., Miszalski, Z., et al. (2009). Arabidopsis chloroplastic glutathione peroxidases play a role in cross talk between photooxidative stress and immune responses. Plant Physiol. 150, 670–683. doi: 10.1104/pp.109.135566
Chen, M. Y., Li, K., Li, H. P., Song, C. P., and Miao, Y. C. (2017). The glutathione peroxidase gene family in Gossypium hirsutum: genome-wide identification classification gene expression and functional analysis. Sci. Rep. 7:44743. doi: 10.1038/srep44743
Chinnusamy, V., Ohta, M., Kanrar, S., Lee, B. H., Hong, X., Agarwal, M., et al. (2003). ICE1: a regulator of cold-induced transcriptome and freezing tolerance in Arabidopsis. Gene Dev. 17, 1043–1054. doi: 10.1101/gad.1077503
Choudhury, F. K., Rivero, R. M., Blumwald, E., and Mittler, R. (2017). Reactive oxygen species abiotic stress and stress combination. Plant J. 90, 856–867. doi: 10.1111/tpj.13299
Clough, S. J., and Bent, A. F. (1998). Floral dip: a simplified method for Agrobacterium-mediated transformation of Arabidopsis thaliana. Plant J. 16, 735–743. doi: 10.1046/j.1365-313x.1998.00343.x
Diao, Y., Xu, H., Li, G., Yu, A., Yu, X., Hu, W., et al. (2014). Cloning a glutathione peroxidase gene from Nelumbo nucifera and enhanced salt tolerance by overexpressing in rice. Mol. Biol. Rep. 41, 4919–4927. doi: 10.1007/s11033-014-3358-4
Ding, Y. L., Shi, Y. T., and Yang, S. H. (2019). Tansley review Advances and challenges in uncovering cold tolerance regulatory mechanisms in plants. New Phytol. 222, 1690–1704. doi: 10.1111/nph.15696
Edgar, R. C. (2004). MUSCLE: a multiple sequence alignment method with reduced time and space complexity. BMC Bioinform. 5:113. doi: 10.1186/1471-2105-5-113
Gaber, A., Ogata, T., Maruta, T., Yoshimura, K., Tamoi, M., and Shigeoka, S. (2012). The involvement of Arabidopsis glutathione peroxidase 8 in the suppression of oxidative damage in the nucleus and cytosol. Plant Cell Physiol. 53, 1596–1606. doi: 10.1093/pcp/pcs100
Gao, F., Chen, J., Ma, T. T., Li, H. Y., Wang, N., Li, Z. L., et al. (2014). The glutathione peroxidase gene family in Thellungiella salsuginea: genome-wide identification classification, and gene and protein expression analysis under stress conditions. Int. J. Mol. Sci. 15, 3319–3335. doi: 10.3390/ijms15023319
Gasteiger, E., Hoogland, C., Gattiker, A., Duvaud, S., Wilkins, M. R., Appel, R. D., et al. (2005). “Protein identification and analysis tools on the ExPASy server,” in The Proteomics Protocols Handbook, ed. J. M. Walker (Totowa, NJ: Humana), 571–607.
Guan, C., Wang, X., Feng, J., Hong, S., Liang, Y., Ren, B., et al. (2014). Cytokinin antagonizes abscisic acid-mediated inhibition of cotyledon greening by promoting the degradation of abscisic acid insensitive5 protein in Arabidopsis. Plant Physiol. 164, 1515–1526. doi: 10.1104/pp.113.234740
Horton, P., Park, K. J., Obayashi, T., Fujita, N., Harada, H., Adams-Collier, C. J., et al. (2007). WoLF PSORT: protein localization predictor. Nucleic Acids Res. 35, W585–W587. doi: 10.1093/nar/gkm259
Hu, B., Jin, J., Guo, A. Y., Zhang, H., Luo, J., and Gao, G. (2015). GSDS 2.0: an upgraded gene feature vpisualization server. Bioinformatics 31, 1296–1297. doi: 10.1093/bioinformatics/btu817
Huang, X. Z., Zhang, X. Y., Gong, Z. Z., Yang, S. H., and Shi, Y. T. (2017). ABI4 represses the expression of type-A ARRs to inhibit seed germination in Arabidopsis. Plant J. 89, 354–365. doi: 10.1111/tpj.13389
Huelsenbeck, J. P., Ronquist, F., and Hall, B. (2001). MrBayes: bayesian inference of phylogeny. Bioinformatics 17, 754–755.
Iqbal, A., Yabuta, Y., Takeda, T., Nakano, Y., and Shigeoka, S. (2006). Hydroperoxide reduction by thioredoxin-specific glutathione peroxidase isoenzymes of Arabidopsis thaliana. FEBS J. 273, 5589–5597. doi: 10.1111/j.1742-4658.2006.05548.x
Ishibashi, Y., Tawaratsumida, T., Kondo, K., Kasa, S., Sakamoto, M., Aoki, N., et al. (2012). Reactive oxygen species are involved in gibberellin/abscisic acid signaling in barley aleurone cells. Plant Physiol. 158, 1705–1714. doi: 10.1104/pp.111.192740
Islam, T., Manna, M., Kaul, T., Pandey, S., Reddy, C. S., and Reddy, M. K. (2015a). Genome-wide dissection of Arabidopsis and rice for the identification and expression analysis of glutathione peroxidases reveals their stress-specifc and overlapping response patterns. Plant Mol. Biol. Rep. 33, 1413–1427. doi: 10.1007/s11105-014-0846-6
Islam, T., Manna, M., and Reddy, M. K. (2015b). Glutathione peroxidase of Pennisetum glaucum (PgGPx) is a functional Cd2+ dependent peroxiredoxin that enhances tolerance against salinity and drought stress. PLoS One 10:e0143344. doi: 10.1371/journal.pone.0143344
Jana, G. J., and Yaish, M. W. (2020). Genome-wide identification and functional characterization of glutathione peroxidase genes in date palm (Phoenix dactylifera L.) under stress conditions. Plant Gene 23:100237. doi: 10.1016/j.plgene.2020.100237
Jones, D. T., Taylor, W. R., and Thornton, J. M. (1992). The rapid generation of mutation data matrices from protein sequences. Bioinformatics 8, 275–282. doi: 10.1093/bioinformatics/8.3.275
Jubany-Mari, T., Munne-Bosch, S., Lopez-Carbonell, M., and Alegre, L. (2009). Hydrogen peroxide is involved in the acclimation of the mediterranean shrub, Cistus albidus L., to summer drought. J. Exp. Bot. 60, 107–120. doi: 10.1007/s10815-007-9182-1
Keane, T. M., Creevey, C. J., Pentony, M. M., Naughton, T. J., and McInerney, J. O. (2006). Assessment of methods for amino acid matrix selection and their use on empirical data shows that ad hoc assumptions for choice of matrix are not justified. BMC Evol. Biol. 6:29. doi: 10.1186/1471-2148-6-29
Kim, Y. J., Jang, M. G., Noh, H. Y., Lee, H. J., Sukweenadhi, J., Kim, J. H., et al. (2014). Molecular characterization of two glutathione peroxidase genes of Panax ginseng and their expression analysis against environmental stresses. Gene 535, 33–41. doi: 10.1016/j.gene.2013.10.071
Koh, C. S., Didierjean, C., Navrot, N., Panjikar, S., Mulliert, G., Rouhier, N., et al. (2007). Crystal structures of a poplar thioredoxin peroxidase that exhibits the structure of glutathione peroxidases: insights into redox-driven conformational changes. J. Mol. Biol. 370, 512–529. doi: 10.1016/j.jmb.2007.04.031
Koua, D., Cerutti, L., Falquet, L., Sigrist, C. J. A., Theiler, G., Hulo, N., et al. (2009). PeroxiBase: a database with new tools for peroxidase family classification. Nucleic Acids Res. 37, D261–D266. doi: 10.1093/nar/gkn680
Kovtun, Y., Chiu, W. L., Tena, G., and Sheen, J. (2000). Functional analysis of oxidative stress-acti-vated mitogen-activated protein kinase cascade in plants. Proc. Natl. Acad. Sci. U.S.A. 97, 2940–2945. doi: 10.1073/pnas.97.6.2940
Larkin, M. A., Blackshields, G., Brown, N. P., Chenna, R., McGettigan, P. A., McWilliam, H., et al. (2007). Clustal W and Clustal X version 20. Bioinformatics 23, 2947–2948. doi: 10.1093/bioinformatics/btm404
Le, S. Q., and Gascuel, O. (2008). An improved general amino acid replacement matrix. Mol. Biol. Evol. 25, 1307–1320. doi: 10.1093/molbev/msn067
Lescot, M., Dehais, P., Thijs, G., Marchal, K., Moreau, Y., Van de Peer, Y., et al. (2002). PlantCARE, a database of plant cis-acting regulatory elements and a portal to tools for in silico analysis of promoter sequences. Nucleic Acids Res. 30, 325–327. doi: 10.1093/nar/30.1.325
Letunic, I., and Bork, P. (2021). Interactive Tree Of Life (iTOL) v5: an online tool for phylogenetic tree display and annotation. Nucleic Acids Res. 49, W293–W296. doi: 10.1093/nar/gkab301
Li, H., Ding, Y. L., Shi, Y. T., Zhang, X. Y., Zhang, S. Q., Gong, Z. Z., et al. (2017). MPK3- and MPK6-mediated ICE1 phosphorylation negatively regulates ICE1 stability and freezing tolerance in Arabidopsis. Dev. Cell 43:5. doi: 10.1016/j.devcel.2017.09.025
Liu, Q., Kasuga, M., Sakuma, Y., Abe, H., Miura, S., Yamaguchi-Shinozaki, K., et al. (1998). Two transcription factors, DREB1 and DREB2, with an EREBP/AP2 DNA binding domain separate two cellular signal transduction pathways in drought-and low-temperature-responsive gene expression, respectively, in Arabidopsis. Plant Cell 10, 1391–1406. doi: 10.1105/tpc.10.8.1391
Livak, K. J., and Schmittgen, T. D. (2001). Analysis of relative gene expression data using real-time quantitative PCR and the 2–ΔΔCt method. Methods 25, 402–408. doi: 10.1006/meth.2001.1262
Margis, R., Dunand, C., Teixeira, F. K., and Margis-Pinheiro, M. (2008). Glutathione peroxidase family-an evolutionary overview. Febs J. 275:3959. doi: 10.1111/j.1742-4658.2008.06542.x
Miao, Y. C., Lv, D., Wang, P. C., Wang, X. C., Chen, J., Miao, C., et al. (2006). An Arabidopsis glutathione peroxidase functions as both a redox transducer and a scavenger in abscisic acid and drought stress responses. Plant Cell 18, 2749–2766. doi: 10.1105/tpc.106.044230
Navrot, N., Collin, V., Gualberto, J., Gelhaye, E., Hirasawa, M., Rey, P., et al. (2006). Plant glutathione peroxidases are functional peroxiredoxins distributed in several subcellular compartments and regulated during biotic and abiotic stresses. Plant Physiol. 142, 1364–1379. doi: 10.1104/pp.106.089458
Ogunniyi, D. S. (2006). Castor oil: a vital industrial raw material. Bioresource Technol. 97, 1086–1091. doi: 10.1016/j.biortech.2005.03.028
Ozyigit, I. I., Filiz, E., Vatansever, R., Kurtoglu, K. Y., Koc, I., Münir, X., et al. (2016). Identification and comparative analysis of H2O2-Scavenging enzymes (Ascorbate Peroxidase and Glutathione Peroxidase) in selected plants employing bioinformatics approaches. Front. Plant Sci. 7:420. doi: 10.3389/fpls.2016.00301
Passaia, G., Fonini, L. S., Caverzan, A., Jardim-Messeder, D., Christof, A. P., Gaeta, M. L., et al. (2013). The mitochondrial glutathione peroxidase GPX3 is essential for H2O2 homeostasis and root and shoot development in rice. Plant Sci. 208, 93–101. doi: 10.1016/j.plantsci.2013.03.017
Passaia, G., and Margispinheiro, M. (2015). Glutathione peroxidases as redox sensor proteins in plant cells. Plant Sci. 234, 22–26. doi: 10.1016/j.plantsci.2015.01.017
Piskurewicz, U., Jikumaru, Y., Kinoshita, N., Nambara, E., Kamiya, Y., and Lopez-Molina, L. (2008). The gibberellic acid signaling repressor RGL2 inhibits Arabidopsis seed germination by stimulating abscisic acid synthesis and ABI5 activity. Plant Cell 20, 2729–2745. doi: 10.2307/25224377
Rahantaniaina, M. S., Li, S. C., Chatel-Innocenti, G., Tuzet, A., Mhamdi, A., Vanacker, H., et al. (2017). Glutathione oxidation in response to intracellular H2O2: key but overlapping roles for dehydroascorbate reductases. Plant Signal Behav. 12:8. doi: 10.1080/15592324.2017.1356531
Ramos, J., Matamoros, M. A., Naya, L., James, E. K., Rouhier, N., Sato, S., et al. (2009). The glutathione peroxidase gene family of Lotus japonicus: characterization of genomic clones, expression analyses and immunolocalization in legumes. New Phytol. 181, 103–114. doi: 10.1111/j.1469-8137.2008.02629.x
Rodriguez Milla, M. A., Maurer, A., Rodriguez Huete, A., and Gustafson, J. P. (2003). Glutathione peroxidase genes in Arabidopsis are ubiquitous and regulated by abiotic stresses through diverse signaling pathways. Plant J. 36, 602–615. doi: 10.1046/j.1365-313X.2003.01901.x
Roxas, V., Lodhi, S., Garrett, D. K., Mahan, J. R., and Allen, R. D. (2000). Stress tolerance in transgenic tobacco seedlings that overexpress glutathione S-transferase/glutathione peroxidase. Plant Cell Physiol. 41, 1229–1234. doi: 10.1093/pcp/pcd051
Saxena, I., Srikanth, S., and Chen, Z. (2016). Cross talk between H2O2 and interacting signal molecules under plant stress response. Front. Plant Sci. 7:22. doi: 10.3389/fpls.2016.00570
Scholza, V., and da Silva, J. N. (2008). Prospects and risks of the use of castor oil as a fuel. Biomass Bioenerg. 32, 95–100. doi: 10.1016/j.biombioe.2007.08.004
Selles, B., Hugo, M., Trujillo, M., Srivastava, V., Wingsle, G., Jacquot, J. P., et al. (2012). Hydroperoxide and peroxynitrite reductase activity of poplar thioredoxin-dependent glutathione peroxidase 5: kinetics catalytic mechanism and oxidative inactivation. Biochem. J. 442, 369–380. doi: 10.1042/BJ20111378
Shkolnik-Inbar, D., and Bar-Zvi, D. (2010). ABI4 mediates abscisic acid and cytokinin inhibition of lateral root formation by reducing polar auxin transport in Arabidopsis. Plant Cell 22, 3560–3573. doi: 10.4161/psb.6.5.14978
Shu, K., Chen, Q., Wu, Y., Liu, R., Zhang, H., Wang, P., et al. (2016). ABI4 mediates antagonistic effects of abscisic acid and gibberellins at transcript and protein levels. Plant J. 85, 348–361. doi: 10.1111/tpj.13109
Shu, K., Zhang, H., Wang, S., Chen, M., Wu, Y., Tang, S., et al. (2013). ABI4 regulates primary seed dormancy by regulating the biogenesis of abscisic acid and gibberellins in Arabidopsis. PLoS Genet. 9:e1003577. doi: 10.1371/journal.pgen.1003577
Stockinger, E. J., Gilmour, S. J., and Thomashow, M. F. (1997). Arabidopsis thaliana CBF1 encodes an AP2 domain-containing transcriptional activator that binds to the Crepeat/DRE, a cis-acting DNA regulatory element that stimulates transcription in response to low temperature and water deficit. Proc. Natl. Acad. Sci. U.S.A. 94, 1035–1040. doi: 10.1073/pnas.94.3.1035
Ursini, F., Maiorino, M., Brigelius-Flohe, R., Aumann, K. D., Roveri, A., Schomburg, D., et al. (1995). Diversity of glutathione peroxidases. Methods Enzymol. 252, 38–53. doi: 10.1016/0076-6879(95)52007-4
Wang, X. Y., Li, M., Liu, X. M., Zhang, L. X., Duan, Q., and Zhang, J. X. (2019). Quantitative proteomic analysis of castor (Ricinus communis L) seeds during early imbibition provided novel insights into cold stress response. Int. J. Mol. Sci. 20:355. doi: 10.3390/ijms20020355
Yao, L., Hao, X. Y., Gao, H. L., Ding, C. Q., Yang, Y. J., Wang, L., et al. (2020). ABA-dependent bZIP transcription factor, CsbZIP18, from Camellia sinensis negatively regulates freezing tolerance in Arabidopsis. Plant Cell Rep. 39, 553–565. doi: 10.1007/s00299-020-02512-4
Yoshimura, K., Miyao, K., Gaber, A., Takeda, T., Kanaboshi, H., Miyasaka, H., et al. (2004). Enhancement of stress tolerance in transgenic tobacco plants overexpressing Chlamydomonas glutathione peroxidase in chloroplasts or cytosol. Plant J. 37, 21–33. doi: 10.1046/j.1365-313X.2003.01930.x
You, J., and Chan, Z. (2015). ROS regulation during abiotic stress responses in crop plants. Front. Plant Sci. 6:1092. doi: 10.3389/fpls.2015.01092
Yu, C. S., Chen, Y. C., Lu, C. H., and Hwang, J. K. (2006). Prediction of protein subcellular localization. Proteins: Struct. Funct. Bioinform. 64, 643–651. doi: 10.1002/prot.21018
Zhai, C. Z., Zhao, L., Yin, L. J., Chen, M., Wang, Q. Y., Li, L. C., et al. (2013). Two wheat glutathione peroxidase genes whose products are located in chloroplasts improve salt and H2O2 tolerances in Arabidopsis. PLoS One 8:e73989. doi: 10.1371/journal.pone.0073989
Zhang, L. P., Wu, M., Teng, Y. J., Jia, S. H., Yu, D. S., Wei, T., et al. (2019). Overexpression of the Glutathione Peroxidase 5 (RcGPX5) Gene From Rhodiola crenulata Increases Drought Tolerance in Salvia miltiorrhiza. Front. Plant Sci. 9:1950. doi: 10.3389/FPLS.2018.01950
Keywords: Ricinus communis L., genome-wide identification, RcGPX4, functional analysis, cold tolerance
Citation: Wang X, Liu X, An Y-qC, Zhang H, Meng D, Jin Y, Huo H, Yu L and Zhang J (2021) Identification of Glutathione Peroxidase Gene Family in Ricinus communis and Functional Characterization of RcGPX4 in Cold Tolerance. Front. Plant Sci. 12:707127. doi: 10.3389/fpls.2021.707127
Received: 09 May 2021; Accepted: 30 September 2021;
Published: 05 November 2021.
Edited by:
Gisele Passaia Prietsch, Independent Researcher, Vancouver, BC, CanadaReviewed by:
Andreia Carina Turchetto Zolet, Federal University of Rio Grande do Sul, BrazilJoao Braga De Abreu Neto, Federal University of Rio Grande do Sul, Brazil
Andréia Caverzan, Independent Researcher, Passo Fundo, Brazil
Copyright © 2021 Wang, Liu, An, Zhang, Meng, Jin, Huo, Yu and Zhang. This is an open-access article distributed under the terms of the Creative Commons Attribution License (CC BY). The use, distribution or reproduction in other forums is permitted, provided the original author(s) and the copyright owner(s) are credited and that the original publication in this journal is cited, in accordance with accepted academic practice. No use, distribution or reproduction is permitted which does not comply with these terms.
*Correspondence: Xiaoyu Wang, eGlhb3l1d2FuZzE5ODdAaG90bWFpbC5jb20=; Jixing Zhang, emp4LTcxMTIwOUAxNjMuY29t