- 1Institut de biologie moléculaire des plantes, CNRS, Université de Strasbourg, Strasbourg, France
- 2Aquatic and Crop Resource Development Research Centre, National Research Council Canada, Saskatoon, SK, Canada
The extreme chemical and physical recalcitrance of sporopollenin deems this biopolymer among the most resilient organic materials on Earth. As the primary material fortifying spore and pollen cell walls, sporopollenin is touted as a critical innovation in the progression of plant life to a terrestrial setting. Although crucial for its protective role in plant reproduction, the inert nature of sporopollenin has challenged efforts to determine its composition for decades. Revised structural, chemical, and genetic experimentation efforts have produced dramatic advances in elucidating the molecular structure of this biopolymer and the mechanisms of its synthesis. Bypassing many of the challenges with material fragmentation and solubilization, insights from functional characterizations of sporopollenin biogenesis in planta, and in vitro, through a gene-targeted approach suggest a backbone of polyhydroxylated polyketide-based subunits and remarkable conservation of biochemical pathways for sporopollenin biosynthesis across the plant kingdom. Recent optimization of solid-state NMR and targeted degradation methods for sporopollenin analysis confirms polyhydroxylated α-pyrone subunits, as well as hydroxylated aliphatic units, and unique cross-linkage heterogeneity. We examine the cross-disciplinary efforts to solve the sporopollenin composition puzzle and illustrate a working model of sporopollenin’s molecular structure and biosynthesis. Emerging controversies and remaining knowledge gaps are discussed, including the degree of aromaticity, cross-linkage profiles, and extent of chemical conservation of sporopollenin among land plants. The recent developments in sporopollenin research present diverse opportunities for harnessing the extraordinary properties of this abundant and stable biomaterial for sustainable microcapsule applications and synthetic material designs.
Introduction
A seminal innovation in plant evolution was the fortification of mobile reproductive cells with sporopollenin. Amidst novel stresses, reinforcement of spore cell walls with a desiccation-resistant, durable exterior of sporopollenin likely provided an adaptive advantage to plant terrestrial reproduction. Lauded as the “diamond of the plant world,” sporopollenin is an organic material distinguished by its extraordinary stability and recalcitrance when challenged by mechanical, thermal, hydrostatic, non-oxidative chemical, and biological pressures (Kesseler and Harley, 2004; Barrier, 2008; Montgomery et al., 2016). The inert nature of sporopollenin is perhaps best highlighted by its chemical preservation in fossil spores an estimated 450 million years old, providing the earliest record of plant life on land (Brooks and Shaw, 1971; Brown and Lemmon, 2011). The ubiquitous appearance of sporopollenin in the outer shell of land plant spores and pollen grains, and strong evolutionary conservation of genes encoding key enzymes in the biosynthesis of sporopollenin among current land plants, support a critical function for this biopolymer in the conquest and proliferative success of plants on dry land (Wallace et al., 2011; Gómez et al., 2015; Quilichini et al., 2015; Shi et al., 2015).
The definition of sporopollenin as the organic residue remaining after acetolysis conveys the nebulous understanding of this biomacromolecule that has prevailed (Zetzsche and Vicari, 1931; Brown, 1960; Hemsley et al., 2009). This is in stark contrast to other major and more widely known biopolymers that fortify plant cell walls, including cutin, suberin, and lignin, for which chemical composition and linkage data are extensive (Mackenzie et al., 2015). Much of the uncertainty surrounding sporopollenin is due to the material’s extreme inertness, preventing solubilization under harsh treatments and yielding partial, modified or degraded fragments of the native polymer for biochemical characterization (Hemsley et al., 2009; Li et al., 2019a). From early models of a carotenoid-based polymer (Brooks and Shaw, 1968), the tools available for elucidating sporopollenin’s composition have grown rapidly, supporting substantial revision of molecular structure models. Circumventing challenges of sporopollenin solubilization and breakdown, genetic tools predominantly in model flowering plants have uncovered a core set of biosynthetic enzymes required for sporopollenin biosynthesis. Mounting evidence from non-model species supports strong conservation of a sporopollenin metabolic pathway across the plant kingdom. Mutant analyses, in vitro enzyme assays, labeling studies, and chemical breakdown analyses have facilitated informed inferences on sporopollenin’s composition, supporting a polyhydroxylated aliphatic backbone of polyketides, an aromatic fraction, and extensive coupling of subunits through ester and ether cross-linkages (Quilichini et al., 2015). Until recently however, genetic studies could not fully explain models from biochemical analyses and vice versa.
A recent surge in the analytical characterization of sporopollenin has produced significant breakthroughs in the elucidation of molecular structure, complimenting and challenging prevailing theories. Aided by the development of treatment approaches for interrogating the biopolymer, and the large quantities of spores/pollen available from non-flowering species, mass spectrometry, solid-state NMR, and infrared spectroscopy techniques have uncovered novel structural and compositional data on sporopollenin. Among these discoveries, subunits of varying phobicity coupled by linkages with different solvent stabilities offer plausible explanations for the unique recalcitrance of sporopollenin. While a clearer picture for sporopollenin is emerging, new discrepancies between studies from disparate plant groups and methodologies raise questions about the extent of chemical conservation versus heterogeneity of sporopollenin among land plants. Here, we highlight the latest breakthroughs and emerging controversies in sporopollenin research, and the value of solving the elusive structure of sporopollenin for biotechnological advances.
Conserved Metabolic Pathways for Sporopollenin Biosynthesis
In flowering plants, sporopollenin is synthesized in tapetal cells that line the inner surface of the anther. Following its synthesis, sporopollenin constituents must exit the tapetum, traverse a fluid-filled locule, and assemble into the structured outer pollen wall or exine surrounding immature pollen grains (or microspores). Although questions surrounding the mechanisms of sporopollenin delivery from the sporophytic tapetum to the developing male gametophyte remain largely unanswered and beyond the scope of this article, evidence from several species supports a role for transport proteins, including ATP-binding cassette transporters and lipid transfer proteins, in sporopollenin export and/or shuttling from the tapetum (Liang et al., 2010; Quilichini et al., 2010, 2014; Choi et al., 2011; Huang et al., 2013; Niu et al., 2013; Qin et al., 2013; Zhu et al., 2013; Zhao et al., 2015; Chang et al., 2016, 2018; Zaidi et al., 2020). Similarly, little is known about the polymerization of sporopollenin, but recent studies implicate reactive oxygen species (ROS) and the environment of the locule in the biopolymer’s assembly (see section entitled “Cross-linkages and Assembly of Sporopollenin” below).
In contrast to sporopollenin traffic and assembly, more is known about sporopollenin biosynthesis, with a suite of tapetum-expressed genes including MALE STERILITY2 (MS2; Aarts et al., 1997; Chen et al., 2011), CYTOCHROME P450 (CYP) 703A2 (Morant et al., 2007), CYP704B1 (Dobritsa et al., 2009), ACYL-COA SYNTHTASE5 (ACOS5; Souza et al., 2009), POLYKETIDE SYNTHASE A/LESS ADHESIVE POLLEN6 (PKSA/LAP6), PKSB/LAP5 (Dobritsa et al., 2010; Kim et al., 2010), and TETRAKETIDE α-PYRONE REDUCTASE1/DIHYDROFLAVONOL 4-REDUCTASE-LIKE1 (TKPR1/DRL1; Tang et al., 2009; Grienenberger et al., 2010) encoding enzymes required for sporopollenin biosynthesis in Arabidopsis thaliana (Arabidopsis; Table 1). Loss-of-function mutations in these genes affect male fertility and disrupt exine deposition, supporting functions for the encoded enzymes in synthesizing crucial sporopollenin constituents. Enzyme assays characterizing recombinant MS2, CYP703A2, CYP704B1, ACOS5, PKSA/LAP6, PKSB/LAP5, and TKPR1/DRL1 support a major role for lipid metabolism in sporopollenin production. In vitro, medium- to long-chain fatty acids with varying degrees of hydroxylation are accepted by Arabidopsis ACOS5, forming activated fatty acyl-CoA esters that can be condensed with malonyl-CoAs to form tri- and tetraketide α-pyrones by PKSA/LAP6 and PKSB/LAP5 enzymes (reviewed by Quilichini et al., 2015). Subsequent ketone reduction by TKPR1/DRL1 produces polyhydroxylated aliphatic α-pyrones that are proposed to form core monomers of sporopollenin (Figure 1, blue shading). In addition, the production of fatty alcohols by reduction of long-chain fatty acyl-ACP by MS2 (Doan et al., 2009; Chen et al., 2011) supports a hypothesized secondary pathway yielding aliphatic sporopollenin precursors that could link with the aliphatic α-pyrone units (Figure 1, green shading). Two CYTOCHROME P450 enzymes, CYP703A2 and CYP704B1, essential for exine synthesis, catalyze in-chain and ω-hydroxylation of medium- to long-chain fatty acids (Morant et al., 2007; Dobritsa et al., 2009). These P450 enzymes could act prior to ACOS5 to produce polyhydroxylated polyketides or in the production of hydroxylated aliphatic precursors with MS2 (Figure 1). These highly oxygenated potential sporopollenin precursors are conducive to covalent linkage within the polymer.
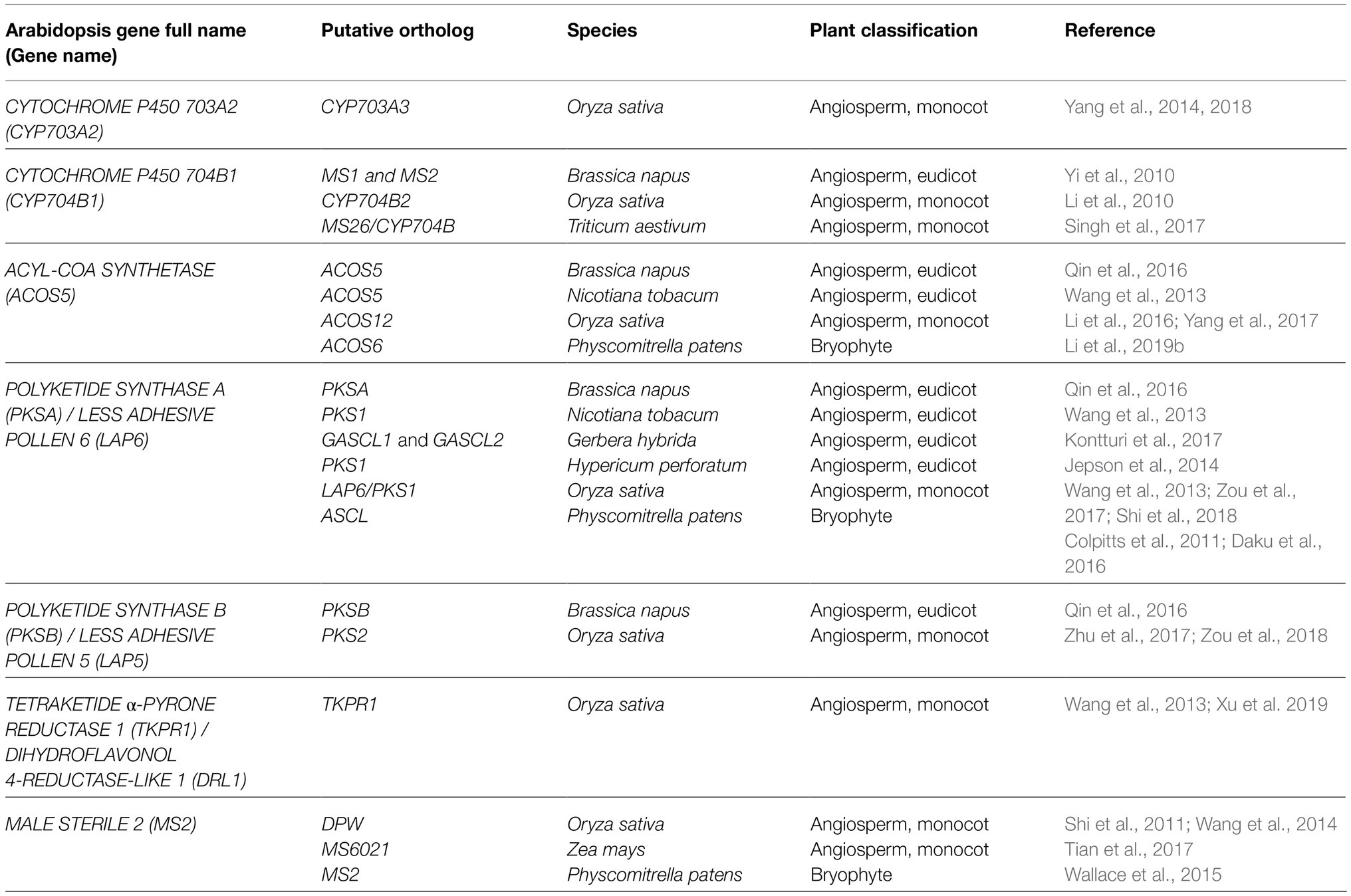
Table 1. Summary of genes and putative orthologs encoding core enzymes required for sporopollenin biosynthesis.
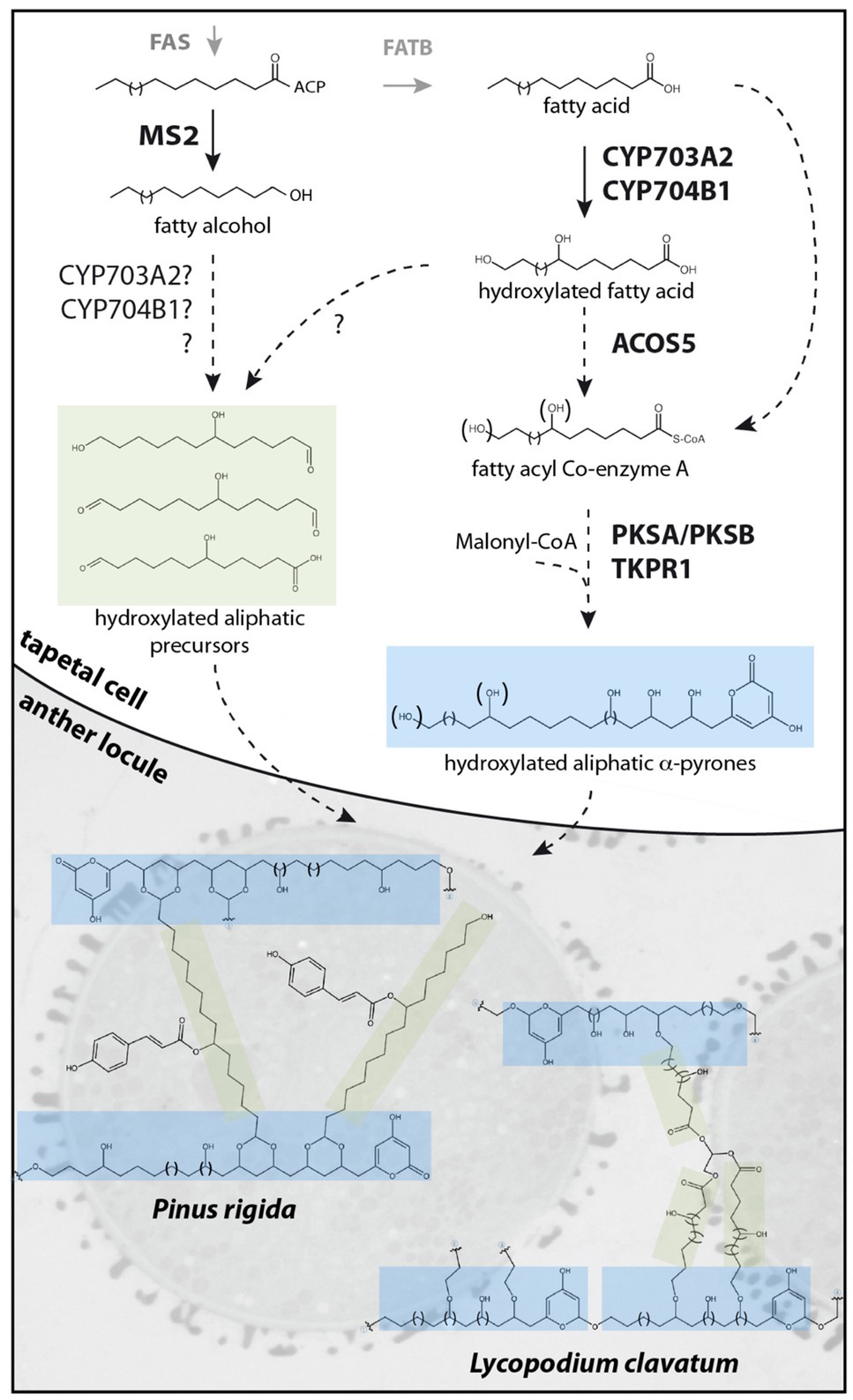
Figure 1. Proposed sporopollenin metabolic pathway and simplified molecular structures of Pinus rigida and Lycopodium clavatum sporopollenin. Fatty acyl-ACP esters, synthesized de novo by the FAS complex, are reduced by MS2 to produce fatty alcohols or hydrolyzed by FATB to produce free fatty acids. After hydroxylation by CYP703A2 and CYP704B1, free fatty acids may be reduced by unknown reductases to fatty alcohols or aldehydes, forming putative hydroxylated aliphatic sporopollenin precursors. Alternatively, hydroxylation by CYP703A2 and CYP704B1 of the fatty alcohol produced by MS2 may occur. In the case of Pinus rigida, these aliphatic precursors might be acylated with phenolics by an unknown transferase. Hydroxylated or non-hydroxylated fatty acids are esterified to CoA by ACOS5 prior to several cycles of condensation by PKSs and reduction by TKPRs to form polyhydroxylated α-pyrone precursors. The precursors could be exported to the anther locule and polymerized on the surface of developing microspores by an unknown mechanism. In Pinus rigida, a simplified sporopollenin is proposed to predominantly contain hydroxylated aliphatic α-pyrone units, crosslinked at one end through an ester group and linked through a dioxane moiety to other units by a hydroxylated aliphatic chain bearing a coumaroyl moiety. In Lycopodium clavatum, the sporopollenin structure is proposed to contain a rigid macrocyclic backbone of several hydroxylated aliphatic α-pyrone units coupled through ether bonds to hydroxylated aliphatic networks linked together by glycerol.
In addition to a critical role for lipid metabolism, there is support for the inclusion of hydroxylated aromatics in sporopollenin (Guilford et al., 1988; Ahlers et al., 1999; Domínguez et al., 1999; Ahlers et al., 2003; Li et al., 2019a; Lutzke et al., 2020; Xue et al., 2020), although no metabolic genes have been shown to be directly involved in the addition of aromatics to the biopolymer. One of the earliest studies to identify a putative genetic link between phenylpropanoid metabolism and sporopollenin production was in Arabidopsis, in which modulated expression of FERULIC ACID 5-HYDROXYLASE (F5H) and CAFFEIC ACID O-METHYLTRANSFERASE (COMT) to form unusual lignin with elevated 5-hydroxyguaiacyl units produced male sterility and abnormal exine (Weng et al., 2010). In rice, the Defective Pollen Wall 2 (DPW2) BAHD acyltransferase catalyzing the transfer of hydroxycinnamoyl moieties to ω-hydroxy fatty acids has been shown to be essential for pollen wall formation (Xu et al., 2017), although it is unclear whether the DPW2 product is directly incorporated into sporopollenin. Further, the expression of Arabidopsis phenylpropanoid pathway genes in the tapetum, alongside ACOS5, PKSA/LAP6, PKSB/LAP5, TKPR, MS2, CYP703A2, and CYP704B1 supports their involvement in sporopollenin production (Xue et al., 2020).
Over the last decade, the expansion of studies characterizing sporopollenin synthesis-related genes and their encoded enzymes in a variety of species has revealed crucial and largely conserved functionalities in spore/pollen wall formation among land plants. In rice, orthologous genes for each Arabidopsis sporopollenin biosynthesis-related gene have been characterized, including DPW (Shi et al., 2011), CYP703A3 (Yang et al., 2014, 2018), CYP704B2 (Li et al., 2010), ACOS12 (Li et al., 2016; Yang et al., 2017), PKS1/LAP6 (Wang et al., 2013; Zou et al., 2017; Shi et al., 2018), PKS2 (Zhu et al., 2017; Zou et al., 2018), and TKPR1 (Xu et al., 2019), and exhibit largely redundant gene product activities (Table 1). These findings, together with the characterization of orthologs required for sporopollenin biosynthesis in monocots Zea mays (Tian et al., 2017) and Triticum aestivum (Singh et al., 2017), and eudicots Brassica napus (Yi et al., 2010; Qin et al., 2016), Nicotiana tobacum (Wang et al., 2013), Gerbera hybrida (Kontturi et al., 2017), and Hypericum perforatum (Jepson et al., 2014) support strong conservation of a core sporopollenin metabolic pathway in angiosperms (Table 1). Despite a deficiency in the study of sporopollenin-related orthologs in non-flowering species, characterization of MS2 (Wallace et al., 2015), ACOS6 (Li et al., 2019b), and ASCL (Colpitts et al., 2011; Daku et al., 2016) in the early emerging moss Physcomitrella patens supports substantial overlap in gene product activities, lending further support to the conservation of sporopollenin biosynthetic machinery among land plants (Table 1). Further, ACOS, PKS, and TKPR appear to function cooperatively as a metabolon, forming an ER-localized multienzyme complex in the tapetum of Arabidopsis, Brassica napus, and Nicotiana tobacum (Lallemand et al., 2013; Wang et al., 2013; Qin et al., 2016), or dually present in the tapetum and locule of rice anthers (Wang et al., 2018; Xu et al., 2019). Altogether, the hypothesis that the synthesis of core sporopollenin constituents involves a highly conserved pathway has gained broad support, reinforcing earlier phylogenetic and genomic findings (Souza et al., 2008; Colpitts et al., 2011; Wang et al., 2013; Gómez et al., 2015; Shi et al., 2015; Zhang et al., 2016) and discoveries made in Arabidopsis. Although understanding of sporopollenin’s chemical composition remains incomplete, molecular genetics have provided a valued mechanism for making informed inferences on sporopollenin’s composition.
Putative Heterogeneity of Sporopollenin in the Plant Kingdom
Evidence from genetic and biochemical studies indicates polyhydroxylated aliphatic derivatives form major constituents of sporopollenin, which appear to be produced by conserved and ancient biochemical pathways. Yet, the extent of chemical conservation of the biopolymer among land plants remains unclear. With the growth of experimental evidence for sporopollenin-related putative orthologs and their encoded enzymes from diverse plant species (Table 1), data from several studies are challenging the notion of a single definition for sporopollenin. In the case of ACOS orthologs, Arabidopsis, rice, tobacco, and canola ACOS enzymes accept similar medium- to long-chain fatty acid substrates, indicating general conservation of enzymatic activities among flowering plants (Souza et al., 2009; Wang et al., 2013; Li et al., 2016; Qin et al., 2016; Yang et al., 2017). Interestingly however, amidst strong conservation of orthologous genes from disparate plant groups, examples of variation in substrate preferences and functional complementation capabilities for select sporopollenin-related enzymes have emerged. Notably, the abnormal exine in Arabidopsis and other dicot sporopollenin biosynthetic mutants commonly manifests as dual abnormalities in rice and other monocots, disrupting exine and anther cuticle formation. The incomplete complementation of the Arabidopsis acos5 mutant exine phenotype by OsACOS12, driven by the Arabidopsis ACOS5 promoter, suggests some functional divergence between dicot and monocot ACOS enzymes (Li et al., 2016). In bryophytes, investigations of sporopollenin synthesis-related genes, including ACOS6 (Li et al., 2019b), ASCL, a PKSA/LAP6 ortholog (Colpitts et al., 2011; Daku et al., 2016), and MS2 in Physcomitrella patens (Pp; Wallace et al., 2015), support general conservation of function, with knock-out mutations in each producing defective spore walls. However, complementation analyses using PpACOS6 or PpMS2 driven by their respective Arabidopsis promoters failed to recover acos5 and ms2 phenotypes (Wallace et al., 2015; Li et al., 2019b). In support of mixed complementation data, unique substrate preferences for ACOS5 and its orthologs in Physcomitrella and rice suggest sporopollenin fatty acid chain lengths may vary among species (Li et al., 2019b). The breadth of substrates accepted by PpACOS6 also appears reduced relative to AtACOS5 and OsACOS12, suggesting a degree of functional divergence in sporopollenin biosynthetic machinery that may translate into biochemical heterogeneity in the biopolymer across the plant kingdom.
The Molecular Structure of Sporopollenin
Despite its incredible properties and perhaps because of its resilience, the molecular structure of sporopollenin has not yet been fully elucidated. Recent works, however, report important progress in defining the molecular structure of sporopollenin. Li et al. developed and optimized chemical degradation protocols in association with state-of-the-art NMR techniques to study sporopollenin from the gymnosperm Pinus rigida (Li et al., 2019a). With the large amounts of pollen that can be obtained from pine and an optimized thioacidolysis method, sporopollenin was partially solubilized. Analysis of the degradation products by HPLC-UV-MS and NMR detected p-coumaroylated 7-OH-C16 aliphatic chains and the flavonoid naringenin as a minor element. Acetylation of the remaining insoluble fraction allowed partial solubilization, with further NMR analysis identifying mostly polyhydroxylated aliphatic chains, polyvinylacetate (PVA)-like units, flanked at one end by an α-pyrone moiety and crosslinked at the other end through an ester group. These units were found crosslinked with coumaroylated 7-OH-C16 fatty acids through a newly described dioxane moiety (Figure 1). Glycerol-like units, and to a lesser extent naringenin, were linked to PVA-like units through ester bonds. It is suggested that the α-pyrone PVA-like units could be obtained through extension of a hydroxylated fatty acyl precursor with several cycles of malonyl-CoA condensation by type III polyketide synthases (such as PKSA/PKSB) followed by a reduction of the ketone, possibly by TKPR1/DRL1. These units would then be crosslinked with p-coumaroylated 7-OH-C16 aliphatics, flanked on one or both ends by aldehydes, to form the dioxane moiety. In this model, sporopollenin crosslinking involves acetal linkages (forming the dioxane moieties) and ester linkages, having distinct chemical stability. The authors suggest that this chemical linkage heterogeneity explains the superior stability of sporopollenin relative to other biopolymers that are predominantly crosslinked via only one linkage type.
In another recent study, Mikhael et al. report novel insights on the molecular structure of sporopollenin from Lycopodium clavatum non-sexual spores (Mikhael et al., 2020). Using a combination of TOF-SIMS and CID-MS/MS, MALDI-TOF-MS and CID-TOF/TOF-MS/MS and solid-state NMR, they describe two main units that form the sporopollenin exine in this pteridophyte. The backbone is reported to contain a unique macrocyclic oligomeric monomer of polyhydroxylated aliphatic α-pyrone units, linked through ether bonds, to form a ring-like structure (Figure 1, blue shading). The second unit is composed of polyhydroxylated aliphatic chains with glycerol as a core component, which forms a dendrimer-like structure (Figure 1, green shading) and is reported to be covalently attached by ether bonds to the macrocyclic backbone to form sporopollenin. In contrast to Li et al., an absence of aromaticity was reported for L. clavatum sporopollenin. Using infrared spectroscopy, Lutzke et al. report analysis of isolated Pinus ponderosa sporopollenin (Lutzke et al., 2020). In agreement with known or hypothesized constituents of sporopollenin, they show that the biopolymer consists of distinct aliphatic and aromatic domains, but in contrast with models proposed by Li et al. and Mikhael et al., α-pyrones were not detected in FTIR spectra. They also report that exine morphology is largely retained after removal of all aromatics, suggesting phenolic compounds are not critical structural components. Similarly, Xue et al. report NMR analysis of sporopollenin from a variety of seed plants, pteridophytes and bryophytes (Xue et al., 2020). Their results indicate the presence of aromatics in all tested sporopollenins, including Lycopodium clavatum, contrasting with data presented by Mikhael et al. (2020).
Cross-Linkages and Assembly of Sporopollenin
To date, no enzyme is known to be involved in the polymerization and deposition of sporopollenin on the microspore surface. It has however been suggested that oxidative polymerization involving ROS takes place, similar to what is known for lignin (Matveyeva et al., 2012; Jacobowitz et al., 2019), and that sporopollenin deposition into an elaborately patterned and sculptured cell wall is guided by a cellulosic (primexine) scaffold and the physico-chemical properties of modulated phases surrounding developing microspores (Scott, 1994; Paxson-Sowders et al., 1997; Gabarayeva et al., 2019; Radja et al., 2019). The application of ROS scavenger to developing moss spores compromised spore wall formation and structural integrity in a dose-dependent manner, suggesting the involvement of ROS and peroxidases in sporopollenin polymerization and/or deposition (Rabbi et al., 2020). In Arabidopsis, tapetum-expressed peroxidases PER9 and PER40 are reported to be mostly involved in tapetal cell wall formation. Primary or secondary effects on pollen wall formation are however also described in the null mutants (Jacobowitz et al., 2019), supporting the oxidative polymerization of sporopollenin.
Discussion
Through the advancement of tools for solubilizing and fractionating sporopollenin, alongside expansion of studies characterizing sporopollenin biosynthesis in diverse plant species, immense strides toward structurally elucidating sporopollenin and the mechanisms of its biosynthesis have been made. Genetic and biochemical efforts to characterize sporopollenin are converging on similar findings, with extensive overlap in the core subunits and biosynthetic enzyme activities identified, collectively supporting a backbone of covalently coupled polyhydroxylated α-pyrones and hydroxylated aliphatic chains. Although much uncertainty surrounding this complex, resilient organic material remains, advancements in elucidating the molecular structure and biosynthetic mechanisms that form sporopollenin are enabling comparative analyses among land plants and raising new questions about the extent of sporopollenin’s chemical conservation (Xue et al., 2020). Further, mechanisms of assembly, higher-order structure, and the plasticity of the polymer remain largely unexplored topics of basic and applied interest.
Recent biochemical studies reporting polymer analysis describe the presence of hydroxylated, aliphatic α-pyrones in sporopollenin (Li et al., 2019a; Mikhael et al., 2020), upholding composition predictions based on in vitro activities of enzymes required for sporopollenin formation. Although biochemical and genetic approaches are forming a more consistent picture of sporopollenin, significant gaps remain between models of sporopollenin proposed by biochemists and what is known of its biosynthetic pathway. In Lycopodium and pine sporopollenin models, aliphatic precursors have in-chain and terminal hydroxyl groups. It is not clear whether the known cytochrome P450 oxygenases (CYP703A2 and CYP704B1) produce these hydroxylations or if other, yet-to-be-discovered oxygenases are involved. In Pinus rigida, it is hypothesized that aliphatic precursors bear aldehyde groups at one or both ends to form acetal bonds with the aliphatic α-pyrone. This production would require the action of unknown alcohol dehydrogenases or reductases from alcohol or carboxylic acid precursors, respectively. Similarly, the presence of coumaroyl and naringenin moieties in pine sporopollenin suggests the involvement of not-yet-discovered acyltransferases. Thus, genetic studies have the potential to bridge the remaining gaps in our knowledge of sporopollenin biosynthesis with insights from molecular structure analyses.
The molecular structure of sporopollenin has been a mystery for decades with numerous studies only providing glimpses of functional groups or fragments of the polymer constituents, such as lipids or phenol rings. It is now suggested that some earlier discoveries identified technical artifacts arising from the harsh conditions used for sporopollenin analysis (Gonzalez-Cruz et al., 2018; Mikhael et al., 2020). Using different models, recent studies present significant progress in understanding sporopollenin’s molecular composition. Li et al. describe a principal structure of hydroxylated aliphatic α-pyrone units linked by coumaroylated 7-OH-C16 aliphatic in pine. Lutzke et al. report aliphatic and aromatic domains but no polyketide-derived elements in pine sporopollenin. Mikhael et al. describe a macrocyclic backbone structure composed of hydroxylated aliphatic α-pyrone units linked by dendrimer-like, hydroxylated fatty acid units in L. clavatum with no aromatics, while Xue et al. report the presence of aromatics for the same plant. Thus, despite recent advances, inconsistencies remain in the available sporopollenin molecular structure data, depending on the techniques used or organism studied.
General consensus describes sporopollenin with a main polyhydroxylated aliphatic component and polyketide-derived aliphatic α-pyrone elements. This emerging picture reconciles for the first time the work of biochemists with that of geneticists that described the involvement of fatty acid oxygenase cytochrome P450s, polyketide synthases, and polyketide reductases in the biosynthesis of sporopollenin. Genetic studies have uncovered remarkable conservation of the core sporopollenin biosynthetic pathway in the plant kingdom. However, differences found recently question the extent of sporopollenin chemical conservation between evolutionary clusters. It is possible that the backbone of sporopollenin between plant clusters is conserved but differs in the way the units are assembled or “decorated” with phenolic-derived elements. Continued efforts to chemically and structurally characterize sporopollenin from flowering and early emerging plant species will help to resolve current discrepancies and provide evolutionary context for resolving sporopollenin’s compositional homogeneity within the plant kingdom.
The recent acceleration of long-term efforts to uncover the mysteries of sporopollenin, particularly with solid-state methods, has provided novel understanding of the molecular features of the biopolymer that confer extreme stability. Harnessing the resilience of sporopollenin offers diverse opportunities for synthetic material design in medical science, space exploration, and environmental remediation. Further, the use of sporopollenin-based microparticles as a vector for oral vaccines and a range of macromolecules offers potential for sustainable applications of this abundant and stable biomaterial.
Author Contributions
All authors listed have made a substantial, direct and intellectual contribution to the work, and approved it for publication.
Funding
Funding provided by the Aquatic and Crop Resource Development Research Centre, National Research Council of Canada, ACRD #58289.
Conflict of Interest
The authors declare that the research was conducted in the absence of any commercial or financial relationships that could be construed as a potential conflict of interest.
Publisher’s Note
All claims expressed in this article are solely those of the authors and do not necessarily represent those of their affiliated organizations, or those of the publisher, the editors and the reviewers. Any product that may be evaluated in this article, or claim that may be made by its manufacturer, is not guaranteed or endorsed by the publisher.
Acknowledgments
This work was supported by the Aquatic and Crop Resource Development Research Division of the National Research Council of Canada. The micrograph presented was prepared in the laboratory of A. L. Samuels and the BioImaging Facility at the University of British Columbia.
References
Aarts, M. G. M., Hodge, R., Kalantidis, K., Florack, D., Wilson, Z. A., Mulligan, B. J., et al. (1997). The Arabidopsis MALE STERILITY 2 protein shares similarity with reductases in elongation/condensation complexes. Plant J. 12, 615–623. doi: 10.1046/j.1365-313X.1997.d01-8.x
Ahlers, F., Lambert, J., and Wiermann, R. (2003). Acetylation and silylation of piperidine solubilized sporopollenin from pollen of Typha angustifolia L. Z. Naturforsch., C. J. Biosci. 58, 807–811. doi: 10.1515/znc-2003-11-1210
Ahlers, F., Thom, I., Lambert, J., Kuckuk, R., and Wiermann, R. (1999). 1H NMR analysis of sporopollenin from Typha angustifolia. Phytochemistry 50, 1095–1098. doi: 10.1016/S0031-9422(98)00225-8
Barrier, S. (2008). Physical and Chemical Properties of Sporopollenin Exine Particles. Doctoral dissertation. University of Hull.
Brooks, J., and Shaw, G. (1968). Chemical structure of the exine of pollen walls and a new function for carotenoids in nature. Nature 219, 532–533. doi: 10.1038/219532a0
Brooks, J., and Shaw, G. (1971). Evidence for life in the oldest known sedimentary rocks—the onverwacht series chert, Swaziland system of Southern Africa. Grana 11, 1–8. doi: 10.1080/00173137109427403
Brown, R. C., and Lemmon, B. E. (2011). Spores before sporophytes: hypothesizing the origin of sporogenesis at the algal–plant transition. New Phytol. 190, 875–881. doi: 10.1111/j.1469-8137.2011.03709.x
Chang, Z., Chen, Z., Yan, W., Xie, G., Lu, J., Wang, N., et al. (2016). An ABC transporter, OsABCG26, is required for anther cuticle and pollen exine formation and pollen-pistil interactions in rice. Plant Sci. 253, 21–30. doi: 10.1016/j.plantsci.2016.09.006
Chang, Z., Jin, M., Yan, W., Chen, H., Qiu, S., Fu, S., et al. (2018). The ATP-binding cassette (ABC) transporter OsABCG3 is essential for pollen development in rice. Rice 11:58. doi: 10.1186/s12284-018-0248-8
Chen, W., Yu, X. H., Zhang, K., Shi, J., De Oliveira, S., Schreiber, L., et al. (2011). MALE STERILE2 encodes a plastid-localized fatty acyl carrier protein reductase required for pollen exine development in Arabidopsis. Plant Physiol. 157, 842–853. doi: 10.1104/pp.111.181693
Choi, H., Jin, J.-Y., Choi, S., Hwang, J.-U., Kim, Y.-Y., Suh, M. C., et al. (2011). An ABCG/WBC-type ABC transporter is essential for transport of sporopollenin precursors for exine formation in developing pollen. Plant J. 65, 181–193. doi: 10.1111/j.1365-313X.2010.04412.x
Colpitts, C. C., Kim, S. S., Posehn, S. E., Jepson, C., Kim, S. Y., Wiedemann, G., et al. (2011). PpASCL, a moss ortholog of anther-specific chalcone synthase-like enzymes, is a hydroxyalkylpyrone synthase involved in an evolutionarily conserved sporopollenin biosynthesis pathway. New Phytol. 192, 855–868. doi: 10.1111/j.1469-8137.2011.03858.x
Daku, R. M., Rabbi, F., Buttigieg, J., Coulson, I. M., Horne, D., Martens, G., et al. (2016). PpASCL, the Physcomitrella patens anther-specific chalcone synthase-like enzyme implicated in sporopollenin biosynthesis, is needed for integrity of the moss spore wall and spore viability. PLoS One 11:e0146817. doi: 10.1371/journal.pone.0146817
Doan, T. T. P., Carlsson, A. S., Hamberg, M., Bülow, L., Stymne, S., and Olsson, P. (2009). Functional expression of five Arabidopsis fatty acyl-CoA reductase genes in Escherichia coli. J. Plant Physiol. 166, 787–796. doi: 10.1016/j.jplph.2008.10.003
Dobritsa, A. A., Lei, Z., Nishikawa, S.-I., Urbanczyk-Wochniak, E., Huhman, D. V., Preuss, D., et al. (2010). LAP5 and LAP6 encode anther-specific proteins with similarity to chalcone synthase essential for pollen exine development in Arabidopsis. Plant Physiol. 153, 937–955. doi: 10.1104/pp.110.157446
Dobritsa, A. A., Shrestha, J., Morant, M., Pinot, F., Matsuno, M., Swanson, R., et al. (2009). CYP704B1 is a long-chain fatty acid omega-hydroxylase essential for sporopollenin synthesis in pollen of Arabidopsis. Plant Physiol. 151, 574–589. doi: 10.1104/pp.109.144469
Domínguez, E., Mercado, J. A., Quesada, M. A., and Heredia, A. (1999). Pollen sporopollenin: degradation and structural elucidation. Sex. Plant Reprod. 12, 171–178. doi: 10.1007/s004970050189
Gabarayeva, N. I., Grigorjeva, V. V., and Shavarda, A. L. (2019). Mimicking pollen and spore walls: self-assembly in action. Ann. Bot. 123, 1205–1218. doi: 10.1093/aob/mcz027
Gómez, J. F., Talle, B., and Wilson, Z. A. (2015). Anther and pollen development: a conserved developmental pathway. J. Integr. Plant Biol. 57, 876–891. doi: 10.1111/jipb.12425
Gonzalez-Cruz, P., Uddin, M. J., Atwe, S. U., Abidi, N., and Gill, H. S. (2018). Chemical treatment method for obtaining clean and intact pollen shells of different species. ACS Biomater Sci. Eng. 4, 2319–2329. doi: 10.1021/acsbiomaterials.8b00304
Grienenberger, E., Kim, S. S., Lallemand, B., Geoffroy, P., Heintz, D., Souza, C. d. A., et al. (2010). Analysis of TETRAKETIDE α-PYRONE REDUCTASE function in Arabidopsis thaliana reveals a previously unknown, but conserved, biochemical pathway in sporopollenin monomer biosynthesis. Plant Cell 22, 4067–4083. doi: 10.1105/tpc.110.080036
Guilford, W. J., Schneider, D. M., Labovitz, J., and Opella, S. J. (1988). High resolution solid state C NMR spectroscopy of sporopollenins from different plant taxa. Plant Physiol. 86, 134–136. doi: 10.1104/pp.86.1.134
Hemsley, A. R., Barrie, P. J., Chaloner, W. G., and Scott, A. C. (2009). The composition of sporopollenin and its use in living and fossil plant systematics. Grana 32, 2–11. doi: 10.1080/00173139309427446
Huang, M.-D., Chen, T.-L. L., and Huang, A. H. C. (2013). Abundant type III lipid transfer proteins in Arabidopsis tapetum are secreted to the locule and become a constituent of the pollen exine. Plant Physiol. 163, 1218–1229. doi: 10.1104/pp.113.225706
Jacobowitz, J. R., Doyle, W. C., and Weng, J.-K. (2019). PRX9 and PRX40 are extensin peroxidases essential for maintaining tapetum and microspore cell wall integrity during Arabidopsis anther development. Plant Cell 31, 848–861. doi: 10.1105/tpc.18.00907
Jepson, C., Karppinen, K., Daku, R. M., Sterenberg, B. T., and Suh, D.-Y. (2014). Hypericum perforatum hydroxyalkylpyrone synthase involved in sporopollenin biosynthesis – phylogeny, site-directed mutagenesis, and expression in nonanther tissues. FEBS J. 281, 3855–3868. doi: 10.1111/febs.12920
Kesseler, R., and Harley, M. (2004). Pollen, the Hidden Sexuality of Flowers Papadakis London: Papa-dakis Publisher.
Kim, S. S., Grienenberger, E., Lallemand, B., Colpitts, C. C., Kim, S. Y., Souza, C. d. A., et al. (2010). LAP6/POLYKETIDE SYNTHASE A and LAP5/POLYKETIDE SYNTHASE B encode hydroxyalkyl α-pyrone synthases required for pollen development and sporopollenin biosynthesis in Arabidopsis thaliana. Plant Cell 22, 4045–4066. doi: 10.1105/tpc.110.080028
Kontturi, J., Osama, R., Deng, X., Bashandy, H., Albert, V. A., and Teeri, T. H. (2017). Functional characterization and expression of GASCL1 and GASCL2, two anther-specific chalcone synthase like enzymes from Gerbera hybrida. Phytochemistry 134, 38–45. doi: 10.1016/j.phytochem.2016.11.002
Lallemand, B., Erhardt, M., Heitz, T., and Legrand, M. (2013). Sporopollenin biosynthetic enzymes interact and constitute a metabolon localized to the endoplasmic reticulum of tapetum cells. Plant Physiol. 162, 616–625. doi: 10.1104/pp.112.213124
Li, Y., Li, D., Guo, Z., Shi, Q., Xiong, S., Zhang, C., et al. (2016). OsACOS12, an orthologue of Arabidopsis acyl-CoA synthetase5, plays an important role in pollen exine formation and anther development in rice. BMC Plant Biol. 16:256. doi: 10.1186/s12870-016-0943-9
Li, F.-S., Phyo, P., Jacobowitz, J., Hong, M., and Weng, J.-K. (2019a). The molecular structure of plant sporopollenin. Nat Plants 5, 41–46. doi: 10.1038/s41477-018-0330-7
Li, H., Pinot, F., Sauveplane, V., Werck-Reichhart, D., Diehl, P., Schreiber, L., et al. (2010). Cytochrome P450 family member CYP704B2 catalyzes the {omega}-hydroxylation of fatty acids and is required for anther cutin biosynthesis and pollen exine formation in rice. Plant Cell 22, 173–190. doi: 10.1105/tpc.109.070326
Li, Y.-L., Zhang, Y.-F., Li, D.-D., Shi, Q. S., Lou, Y., Yang, Z.-N., et al. (2019b). Acyl-CoA synthetases from Physcomitrella, rice and Arabidopsis: different substrate preferences but common regulation by MS188 in sporopollenin synthesis. Planta 250, 535–548. doi: 10.1007/s00425-019-03189-0
Liang, W., Yin, C., Zong, J., Gu, F., and Zhang, D. (2010). OsC6, encoding a lipid transfer protein, is required for postmeiotic anther development In rice. Plant Physiol. 154, 149–162. doi: 10.1104/pp.110.158865
Lutzke, A., Morey, K. J., Medford, J. I., and Kipper, M. J. (2020). Detailed characterization of Pinus ponderosa sporopollenin by infrared spectroscopy. Phytochemistry 170:112195. doi: 10.1016/j.phytochem.2019.112195
Mackenzie, G., Boa, A. N., Diego-Taboada, A., Atkin, S. L., and Sathyapalan, T. (2015). Sporopollenin, the least known yet toughest natural biopolymer. Front. Mater. 2:66. doi: 10.3389/fmats.2015.00066
Matveyeva, N. P., Polevova, S. V., Smirnova, A. V., and Yermakov, I. P. (2012). Sporopollenin accumulation in Nicotiana tabacum L. microspore wall during its development. Cell. Tiss. Biol. 6, 293–301. doi: 10.1134/S1990519X12030078
Mikhael, A., Jurcic, K., Schneider, C., Karr, D., Fisher, G. L., Fridgen, T. D., et al. (2020). Demystifying and unravelling the molecular structure of the biopolymer sporopollenin. Rapid Commun. Mass Spectrom. 34:e8740. doi: 10.1002/rcm.8740
Montgomery, W., Potiszil, C., Watson, J. S., and Sephton, M. A. (2016). Sporopollenin, a natural copolymer, is robust under high hydrostatic pressure. Macromol. Chem. Phys. 217, 2494–2500. doi: 10.1002/macp.201600142
Morant, M., Jørgensen, K., Schaller, H., Pinot, F., Møller, B. L., Werck-Reichhart, D., et al. (2007). CYP703 is an ancient cytochrome P450 in land plants catalyzing in-chain hydroxylation of lauric acid to provide building blocks for sporopollenin synthesis in pollen. Plant Cell 19, 1473–1487. doi: 10.1105/tpc.106.045948
Niu, B.-X., He, F.-R., He, M., Ren, D., Chen, L.-T., and Liu, Y.-G. (2013). The ATP-binding cassette transporter OsABCG15 is required for anther development and pollen fertility in rice. J. Integr. Plant Biol. 55, 710–720. doi: 10.1111/jipb.12053
Paxson-Sowders, D. M., Owen, H. A., and Makaroff, C. A. (1997). A comparative ultrastructural analysis of exine pattern development in wild-type Arabidopsis and a mutant defective in pattern formation. Protoplasma 198, 53–65. doi: 10.1007/BF01282131
Qin, M., Tian, T., Xia, S., Wang, Z., Song, L., Yi, B., et al. (2016). Heterodimer formation of BnPKSA or BnPKSB with BnACOS5 constitutes a multienzyme complex in tapetal cells and is involved in male reproductive development in Brassica napus. Plant Cell Physiol. 57, 1643–1656. doi: 10.1093/pcp/pcw092
Qin, P., Tu, B., Wang, Y., Deng, L., Quilichini, T. D., Li, T., et al. (2013). ABCG15 encodes an ABC transporter protein, and is essential for post-meiotic anther and pollen exine development in rice. Plant Cell Physiol. 54, 138–154. doi: 10.1093/pcp/pcs162
Quilichini, T. D., Friedmann, M. C., Samuels, A. L., and Douglas, C. J. (2010). ATP-binding cassette transporter G26 is required for male fertility and pollen exine formation in Arabidopsis. Plant Physiol. 154, 678–690. doi: 10.1104/pp.110.161968
Quilichini, T. D., Grienenberger, E., and Douglas, C. J. (2015). The biosynthesis, composition and assembly of the outer pollen wall: a tough case to crack. Phytochemistry 113, 170–182. doi: 10.1016/j.phytochem.2014.05.002
Quilichini, T. D., Samuels, A. L., and Douglas, C. J. (2014). ABCG26-mediated polyketide trafficking and hydroxycinnamoyl spermidines contribute to pollen wall exine formation in Arabidopsis. Plant Cell 26, 4483–4498. doi: 10.1105/tpc.114.130484
Rabbi, F., Renzaglia, K. S., Ashton, N. W., and Suh, D.-Y. (2020). Reactive oxygen species are required for spore-wall formation in Physcomitrella patens. Botany 98, 575–587. doi: 10.1139/cjb-2020-0012
Radja, A., Horsley, E. M., Lavrentovich, M. O., and Sweeney, A. M. (2019). Pollen cell wall patterns form from modulated phases. Cell 176, 856–868. doi: 10.1016/j.cell.2019.01.014
Scott, R. J. (1994). “Pollen exine: the sporopollenin enigma and the physics of pattern,” in Molecular and Cellular Aspects of Plant Reproduction. eds. R. J. Scott and A. D. Stead (Cambridge, UK: Cambridge University Press), 49–81.
Shi, J., Cui, M., Yang, L., Kim, Y.-J., and Zhang, D. (2015). Genetic and biochemical mechanisms of pollen wall development. Trends Plant Sci. 20, 741–753. doi: 10.1016/j.tplants.2015.07.010
Shi, J., Tan, H., Yu, X.-H., Liu, Y., Liang, W., Ranathunge, K., et al. (2011). Defective pollen wall is required for anther and microspore development in rice and encodes a fatty acyl carrier protein reductase. Plant Cell 23, 2225–2246. doi: 10.1105/tpc.111.087528
Shi, Q. S., Wang, K.-Q., Li, Y.-L., Zhou, L., Xiong, S. X., Han, Y., et al. (2018). OsPKS1 is required for sexine layer formation, which shows functional conservation between rice and Arabidopsis. Plant Sci. 277, 145–154. doi: 10.1016/j.plantsci.2018.08.009
Singh, M., Kumar, M., Thilges, K., Cho, M.-J., and Cigan, A. M. (2017). MS26/CYP704B is required for anther and pollen wall development in bread wheat (Triticum aestivum L.) and combining mutations in all three homeologs causes male sterility. PLoS One 12:e0177632. doi: 10.1371/journal.pone.0177632
Souza, C. d. A., Barbazuk, B., Ralph, S. G., Bohlmann, J., Hamberger, B., and Douglas, C. J. (2008). Genome-wide analysis of a land plant-specific acyl:coenzyme A synthetase (ACS) gene family in Arabidopsis, poplar, rice and Physcomitrella. New Phytol. 179, 987–1003. doi: 10.1111/j.1469-8137.2008.02534.x
Souza, C. d. A., Kim, S. S., Koch, S., Kienow, L., Schneider, K., McKim, S. M., et al. (2009). A novel fatty Acyl-CoA Synthetase is required for pollen development and sporopollenin biosynthesis in Arabidopsis. Plant Cell 21, 507–525. doi: 10.1105/tpc.108.062513
Tang, L. K., Chu, H., Yip, W. K., Yeung, E. C., and Lo, C. (2009). An anther-specific dihydroflavonol 4-reductase-like gene (DRL1) is essential for male fertility in Arabidopsis. New Phytol. 181, 576–587. doi: 10.1111/j.1469-8137.2008.02692.x
Tian, Y., Xiao, S., Liu, J., Somaratne, Y., Zhang, H., Wang, M., et al. (2017). MALE STERILE6021 (MS6021) is required for the development of anther cuticle and pollen exine in maize. Sci. Rep. 7:16736. doi: 10.1038/s41598-017-16930-0
Wallace, S., Chater, C. C., Kamisugi, Y., Cuming, A. C., Wellman, C. H., Beerling, D. J., et al. (2015). Conservation of Male Sterility 2 function during spore and pollen wall development supports an evolutionarily early recruitment of a core component in the sporopollenin biosynthetic pathway. New Phytol. 205, 390–401. doi: 10.1111/nph.13012
Wallace, S., Fleming, A., Wellman, C. H., and Beerling, D. J. (2011). Evolutionary development of the plant and spore wall. AoB Plants 2011:plr027. doi: 10.1093/aobpla/plr027
Wang, K., Guo, Z.-L., Zhou, W.-T., Zhang, C., Zhang, Z.-Y., Lou, Y., et al. (2018). The regulation of sporopollenin biosynthesis genes for rapid pollen wall formation. Plant Physiol. 178, 283–294. doi: 10.1104/pp.18.00219
Wang, Y., Lin, Y.-C., So, J., Du, Y., and Lo, C. (2013). Conserved metabolic steps for sporopollenin precursor formation in tobacco and rice. Physiol Plantarum 149, 13–24. doi: 10.1111/ppl.12018
Wang, W., Ma, Y., Suo, Y., Yan, L., Zhang, D., and Miao, C. (2014). Crystallization and preliminary crystallographic analysis of defective pollen wall (DPW) protein from Oryza sativa. Acta Cryst. 70, 758–760. doi: 10.1107/S2053230X14008486
Weng, J.-K., Mo, H., and Chapple, C. (2010). Over-expression of F5H in COMT-deficient Arabidopsis leads to enrichment of an unusual lignin and disruption of pollen wall formation. Plant J. 64, 898–911. doi: 10.1111/j.1365-313X.2010.04391.x
Xu, D., Qu, S., Tucker, M. R., Zhang, D., Liang, W., and Shi, J. (2019). Ostkpr1 functions in anther cuticle development and pollen wall formation in rice. BMC Plant Biol. 19, 1–13. doi: 10.1186/s12870-019-1711-4
Xu, D., Shi, J., Rautengarten, C., Yang, L., Qian, X., Uzair, M., et al. (2017). Defective Pollen Wall 2 (DPW2) encodes an acyl transferase required for rice pollen development. Plant Physiol. 173, 240–255. doi: 10.1104/pp.16.00095
Xue, J.-S., Zhang, B., Zhan, H., Lv, Y.-L., Jia, X.-L., Wang, T., et al. (2020). Phenylpropanoid derivatives are essential components of sporopollenin in vascular plants. Mol. Plant 13, 1644–1653. doi: 10.1016/j.molp.2020.08.005
Yang, X., Liang, W., Chen, M., Zhang, D., Zhao, X., and Shi, J. (2017). Rice fatty acyl-CoA synthetase OsACOS12 is required for tapetum programmed cell death and male fertility. Planta 246, 105–122. doi: 10.1007/s00425-017-2691-y
Yang, X., Wu, D., Shi, J., He, Y., Pinot, F., Grausem, B., et al. (2014). Rice CYP703A3, a cytochrome P450 hydroxylase, is essential for development of anther cuticle and pollen exine. J. Integr. Plant Biol. 56, 979–994. doi: 10.1111/jipb.12212
Yang, Z., Zhang, Y., Sun, L., Zhang, P., Liu, L., Yu, P., et al. (2018). Identification of cyp703a3-3 and analysis of regulatory role of CYP703A3 in rice anther cuticle and pollen exine development. Gene 649, 63–73. doi: 10.1016/j.gene.2018.01.058
Yi, B., Zeng, F., Lei, S., Chen, Y., Yao, X., Zhu, Y., et al. (2010). Two duplicate CYP704B1-homologous genes BnMs1 and BnMs2 are required for pollen exine formation and tapetal development in Brassica napus. Plant J. 63, 925–938. doi: 10.1111/j.1365-313X.2010.04289.x
Zaidi, M. A., O’Leary, S. J. B., Gagnon, C., Chabot, D., Wu, S., Hubbard, K., et al. (2020). A triticale tapetal non-specific lipid transfer protein (nsLTP) is translocated to the pollen cell wall. Plant Cell Rep. 39, 1185–1197. doi: 10.1007/s00299-020-02556-6
Zetzsche, F., and Vicari, H. (1931). Untersuchungen über die Membran der Sporen und Pollen. Helv. Chim. Acta 14, 58–78. doi: 10.1002/hlca.19310140104
Zhang, D., Shi, J., and Yang, X. (2016). “Role of Lipid Metabolism in Plant Pollen Exine Development,” in Lipids in Plant and Algae Development. Vol 86. eds. Y. Nakamura and Y. Li-Beisson (Cham: Springer), 315–337.
Zhao, G., Shi, J., Liang, W., Xue, F., Luo, Q., Zhu, L., et al. (2015). Two ATP Binding cassette G transporters, rice ATP binding cassette G26 and ATP binding cassette G15 collaboratively regulate rice male reproduction. Plant Physiol. 169, 2064–2079. doi: 10.1104/pp.15.00262
Zhu, L., Shi, J., Zhao, G., Zhang, D., and Liang, W. (2013). Post-meiotic deficient anther1 (PDA1) encodes an ABC transporter required for the development of anther cuticle and pollen exine in rice. J. Plant Biol. 56, 59–68. doi: 10.1007/s12374-013-0902-z
Zhu, X., Yu, J., Shi, J., Tohge, T., Fernie, A. R., Meir, S., et al. (2017). The polyketide synthase OsPKS2 is essential for pollen exine and Ubisch body patterning in rice. J. Integr. Plant Biol. 59, 612–628. doi: 10.1111/jipb.12574
Zou, T., Liu, M., Xiao, Q., Wang, T., Chen, D., Luo, T., et al. (2018). OsPKS2 is required for rice male fertility by participating in pollen wall formation. Plant Cell Rep. 37, 759–773. doi: 10.1007/s00299-018-2265-x
Keywords: sporopollenin, biopolymer, spore, pollen, exine cell wall, polyketide, α-pyrone, molecular structure
Citation: Grienenberger E and Quilichini TD (2021) The Toughest Material in the Plant Kingdom: An Update on Sporopollenin. Front. Plant Sci. 12:703864. doi: 10.3389/fpls.2021.703864
Edited by:
David Honys, Institute of Experimental Botany, Academy of Sciences of the Czech Republic, CzechiaReviewed by:
Ravishankar Palanivelu, University of Arizona, United StatesGwyneth Ingram, Université de Lyon, France
Copyright © 2021 Grienenberger and Quilichini. This is an open-access article distributed under the terms of the Creative Commons Attribution License (CC BY). The use, distribution or reproduction in other forums is permitted, provided the original author(s) and the copyright owner(s) are credited and that the original publication in this journal is cited, in accordance with accepted academic practice. No use, distribution or reproduction is permitted which does not comply with these terms.
*Correspondence: Teagen D. Quilichini, VGVhZ2VuLlF1aWxpY2hpbmlAbnJjLWNucmMuZ2MuY2E=
†ORCID: Etienne Grienenberger orcid.org/0000-0002-0555-3208
Teagen D. Quilichini orcid.org/0000-0003-3311-3776