- 1School of Biological Sciences, University of Utah, Salt Lake City, UT, United States
- 2Department of Biology, Utah State University, Logan, UT, United States
- 3Henry Eyring Center for Cell and Genome Science, University of Utah, Salt Lake City, UT, United States
Maize (Zea mays subsp. mays) yield loss from arthropod herbivory is substantial. While the basis of resistance to major insect herbivores has been comparatively well-studied in maize, less is known about resistance to spider mite herbivores, which are distantly related to insects and feed by a different mechanism. Two spider mites, the generalist Tetranychus urticae, and the grass-specialist Oligonychus pratensis, are notable pests of maize, especially during drought conditions. We assessed resistance (antibiosis) to both mites of 38 highly diverse maize lines, including several previously reported to be resistant to one or the other mite species. We found that line B96, as well as its derivatives B49 and B75, were highly resistant to T. urticae. In contrast, neither these three lines, nor any others included in our study, were notably resistant to the specialist O. pratensis. Quantitative trait locus (QTL) mapping with replicate populations from crosses of B49, B75, and B96 to susceptible B73 identified a QTL in the same genomic interval on chromosome 6 for T. urticae resistance in each of the three resistant lines, and an additional resistance QTL on chromosome 1 was unique to B96. Single-locus genotyping with a marker coincident with the chromosome 6 QTL in crosses of both B49 and B75 to B73 revealed that the respective QTL was large-effect; it explained ∼70% of the variance in resistance, and resistance alleles from B49 and B75 acted recessively as compared to B73. Finally, a genome-wide haplotype analysis using genome sequence data generated for B49, B75, and B96 identified an identical haplotype, likely of initial origin from B96, as the source of T. urticae resistance on chromosome 6 in each of the B49, B75, and B96 lines. Our findings uncover the relationship between intraspecific variation in maize defenses and resistance to its major generalist and specialist spider mite herbivores, and we identified loci for use in breeding programs and for genetic studies of resistance to T. urticae, the most widespread spider mite pest of maize.
Introduction
While cereal crops including maize (Zea mays subsp. mays) provide more than half of human calories, yield losses to both abiotic and biotic factors are substantial, and can be synergistic (Deutsch et al., 2018). Among abiotic factors, drought stress, which is often associated with elevated temperatures, is especially important, and may become more so in many regions under current climate change projections (Snowdon et al., 2020). Further, in spite of extensive pesticide use, as much as ∼20% of global maize production is lost due to herbivory by arthropod pests (Oerke, 2006). Taking into account anticipated climate change impacts on herbivores, which include increased metabolic rates and winter survival, yield losses from herbivory by insects for maize may increase as much as ∼30% given an average global surface temperature increase of 2°C (Deutsch et al., 2018).
Grasses are attacked by insect herbivores of diverse feeding guilds, such as leaf chewing (e.g., caterpillars and grasshoppers), stem mining (e.g., corn borers), and phloem-feeding (e.g., aphids) (Meihls et al., 2012). Additionally, spider mites (Arthropoda: Chelicerata: Arachnida: Acari), which belong to a sister taxon to insects, have long been recognized as field pests of maize and related cereals (Owens et al., 1976). As opposed to the major insect herbivores of maize, spider mites feed on individual leaf mesophyll cells with specialized mouth parts (needle-like stylets) (Bensoussan et al., 2016; Bui et al., 2018). Although only ∼0.6 mm in length, a single spider mite female can lay dozens of eggs, and the generation time can be as little as 7 days at high temperatures (Owens et al., 1976; Grbic et al., 2007). Population sizes on single plants may therefore reach tens of thousands during a growing season, and can result in nearly complete yield loss under favorable environmental conditions to spider mites (Owens et al., 1976).
Two spider mite species, the generalist two-spotted spider mite (Tetranychus urticae), and the grass-specialist Banks grass mite (Oligonychus pratensis), are significant pests on maize (Owens et al., 1976; Archer and Bynum, 1993; Peairs, 2010). While the impact of these herbivores on maize has been most studied in the United States, T. urticae is globally distributed, and O. pratensis has been reported on multiple continents (Migeon et al., 2011; Migeon and Dorkeld, 2021). In arid regions of the Western United States, both species can be economically damaging field pests of maize. In particular, yield losses from spider mite herbivory typically occur during hot and dry conditions as a result of rapid mite generation times at high temperatures, altered relationships with natural pathogens or predators, or potentially changes in the physiology of drought-stressed plants that are beneficial to mites (Peairs, 2010; Gill et al., 2020). In less arid regions of Eastern North America, T. urticae is the principal spider mite pest of maize. While this species causes little damage in years of average climatic conditions, during summers of abnormally high heat and low precipitation, populations of T. urticae can increase rapidly, and this species can become a dominant arthropod pest of maize, as occurred during the severe drought of 2012 in the Iowa state, United States (Varenhorst et al., 2012). Such outbreaks are very difficult to control, as spider mites, especially the generalist T. urticae, have among the highest known rates of pesticide resistance evolution (Van Leeuwen et al., 2010).
The inability to effectively control spider mites with pesticides, coupled with their vigorousness as pests during hot and dry conditions, has generated interest in understanding the extent and magnitude of spider mite resistance in maize germplasm, and variation in resistance has been observed for both T. urticae and O. pratensis in multiple studies (Owens et al., 1976; Archer, 1987; Kamali et al., 1989; Mansour and Bar-Zur, 1992; Mansour et al., 1993; Tadmor et al., 1999; Bynum et al., 2004a,b). It should be noted that while some of these older studies refer to T. cinnabarinus, T. cinnabarinus is now thought to be the red color form of T. urticae (Auger et al., 2013). Previous efforts to assess maize resistance to spider mites have varied in several ways, including in the stage of maize plants used for screening, in whether whole plants or detached leaves were employed, in the genetic composition of both the maize lines and mite strains, and in how resistance was measured. With respect to the latter, resistance arising from antibiosis (impaired herbivore growth or reproduction), or from tolerance (the ability of a plant to support high herbivore populations without detrimental effects), has been assessed. For T. urticae, several maize lines, including Oh43 and B96 (formerly called 41.2504B), have been reported to exhibit strong antibiosis (Kamali et al., 1989; Mansour and Bar-Zur, 1992; Tadmor et al., 1999). Resistance arising from antibiosis, but also tolerance, has been reported for O. pratensis (Bynum et al., 2004a), and in some cases the mechanism of O. pratensis resistance in maize was not specifically determined (Owens et al., 1976).
Although variation in maize resistance to both spider mite species has been reported, the underlying molecular-genetic basis of mite resistance is largely unknown, as is whether mechanisms of maize resistance to mites are the same as those that confer resistance to insect herbivores. To begin to address these questions, Bui et al. (2018) examined transcriptional responses to spider mites using B73, a comparatively spider mite susceptible maize line that is the source of the reference maize genome. B73 responses to both T. urticae and O. pratensis resembled that of mechanical wounding (tissue disruption is a component of feeding by all herbivores), and were similar to responses reported for herbivory by Spodoptera exigua, a lepidopteran caterpillar (Tzin et al., 2017; Bui et al., 2018). In particular, genes associated with jasmonic acid, a phytohormone known to mediate plant responses to many herbivores (Howe and Jander, 2008), were upregulated. Moreover, genes for the synthesis of benzoxazinoids, like 2,4-dihydroxy-7-methoxy-1,4-benzoxazin-3-one (DIMBOA), and its derivative compounds, were upregulated by both mite species. Benzoxazinoids are defensive compounds that are widespread in grasses (family Poaceae) and are stored as glucoside conjugates; upon tissue disruption during feeding, the aglucones are generated, and have detrimental effects on susceptible herbivores, including many lepidopteran larvae (Wouters et al., 2016). In maize, benzoxazinoids are synthesized by 14 enzymes encoded by the BX1-BX14 genes. Using maize bx1 and bx2 mutants that lack these compounds, Bui et al. (2018) found that on maize seedlings reproduction of T. urticae, but not O. pratensis, increased in the absence of benzoxazinoids. Although this finding suggests that some molecular components of maize resistance to insects may be the same as those that confer resistance to spider mites, whether variation in benzoxazinoid content among maize lines accounts for natural variation in resistance to T. urticae is unknown (Bui et al., 2018), as is the basis of resistance to O. pratensis.
To address these outstanding questions, we surveyed 38 inbred lines for antibiosis to both T. urticae and O. pratensis. We included lines previously reported to be resistant to spider mites, as well as lines reported to be resistant to lepidopteran herbivores, or to have high DIMBOA content. Further, to facilitate follow-up genetic studies, we included the diverse founders of the maize Nested Association Mapping (NAM) population, a high-resolution genetic mapping resource (McMullen et al., 2009). We identified only a small number of maize lines that were highly resistant to T. urticae, and no lines in our study exhibited strong antibiosis to O. pratensis. QTL mapping using three highly T. urticae resistant lines revealed two prominent loci as underpinning antibiosis to T. urticae. Our findings inform studies of plant resistance to spider mite herbivores of varying host breadth, and identify loci for use in breeding programs – as well as candidate genes – for resistance to T. urticae in maize.
Materials and Methods
Selection of Maize Lines and Crosses
Seeds for maize inbred B73 were kindly provided by G. Drews (University of Utah, Salt Lake City, UT, United States), while those for 37 other maize inbred lines were obtained from the North Central Regional Plant Introduction Station (Ames, IA, United States). Apart from B73, these included 24 additional parents of the NAM recombinant inbred line population (McMullen et al., 2009). We failed to propagate one NAM line founder, Ki3, which was therefore excluded from our study. The remaining 13 maize lines were selected because they met one or more of the following criteria: (1) were previously reported to have moderate to high resistance to either T. urticae or O. pratensis (B49, B64, B96, Oh43, Tx202, and TAM-MITE1, with TAM-MITE1 reported to exhibit antibiosis to both mite species; Oh43 is also a NAM line founder) (Kamali et al., 1989; Mansour and Bar-Zur, 1992; Tadmor et al., 1999; Bynum et al., 2004a; and phenotypic data reported in the U.S. National Plant Germplasm System, GRIN); (2) were parents (B14 and B52) or resultant lines (B49, B64, B68, and B86) in breeding programs involving crosses with B96 or Oh43, which have been reported to be highly T. urticae resistant (pedigree data is available at the Maize Genetics and Genomics Database, or MaizeGDB) (Portwood et al., 2019); (3) are widely used maize inbred lines with extensive genetic and genomic resources (Mo17 and W22) (Portwood et al., 2019); (4) have been reported to be resistant to Ostrinia nubilalis, the European corn borer, in previously published studies or in MaizeGDB (B49, B64, B52, B75, B86, B96, and CI31A) (Kamali et al., 1989; Barry et al., 1994; Portwood et al., 2019); or (5) have been reported to have high levels of DIMBOA in previous studies, or are labeled with the phenotype descriptor “DIMBOA content high” in MaizeGDB (B75, B96, and CI31A) (Bing et al., 1990b; Barry et al., 1994; Portwood et al., 2019). Crosses of B73 to B49, B75, and B96 were performed in greenhouses at the University of Utah, and F2 seeds for mapping studies were subsequently produced by selfing F1 progeny.
Spider Mite Stocks and Propagation
A T. urticae strain, W-GR, and an O. pratensis strain, were maintained at high population sizes (several thousand mites) on B73 maize plants in isolated laboratory rooms as previously described at the University of Utah and Utah State University (Logan, UT, United States) (Bui et al., 2018). The origin of these strains, neither of which is inbred, was described by Bui et al. (2018). Briefly, WG-R was derived from T. urticae mites collected at several sites, including from maize plants in a greenhouse, and the O. pratensis strain was isolated from field-grown maize. Both stocks originated from Utah, United States. We also used five additional strains of T. urticae collected in Utah, United States: Catnip (collected from a catnip plant, Nepeta cataria), NightS (collected from a bittersweet nightshade plant, Solanum dulcamara), ShCo (collected from a morning glory plant, Ipomoea purpurea) and strains KH and WGDel (collected from populations on multiple adjacent plant species, not including maize; KH was collected from house plants and WGDel was collected from a greenhouse). These five strains were inbred by mother-son crosses for five generations (Catnip, KH and ShCo) or eight generations (NightS and WGDel) on detached common bean (Phaseolus vulgaris) leaves maintained on wet cotton as previously described (Bryon et al., 2017). In the study by Bryon et al. (2017), genomic sequencing of multiple T. urtiace strains inbred for 5–8 generations suggested that this number of inbreeding generations can produce strains that are largely, albeit not necessarily completely, isogenic. While two of these inbred strains – ShCo and WGDel – were reported previously (Wybouw et al., 2019), the inbred Catnip, KH, and NightS strains were produced in the current study. Unless otherwise noted, the five inbred T. urticae strains were maintained by serial passage on detached bean leaves at the University of Utah as described previously (Bryon et al., 2017).
Screen of 38 Maize Lines for Spider Mite Resistance
Seeds for the 38 maize inbred lines were sown 1–2 cm deep in 5 × 5 cm plastic pots, and resulting seedlings were transplanted to 20 cm diameter pots 10 days after sowing. Germination and subsequent propagation at University of Utah greenhouses used a 16h-light/8h-dark photoperiod, approximate temperature of 25°C, and Metro-Mix® 900 growing mix soil (Sun Gro® Horticulture, Fillmore, UT, United States). After transplanting, plants were kept in trays and watered from below; the plants were fertilized weekly with 200 ppm NutriCulture Cal-Mag Special 16N-3P-16K (Plant Marvel Laboratories, Chicago Heights, IL, United States). At 8 weeks, barriers were applied on the 8th leaf using Tanglefoot, a non-phytotoxic wax (The Scotts Miracle-Gro Company, Marysville, OH, United States) as previously described (Bui et al., 2018; Gill et al., 2020). For each plant, three barriers were established perpendicular to the length of the leaf blades to define two adjacent enclosures of 6.5 cm in length. For each maize inbred line and each spider mite species, three plants were used, resulting in a total of six enclosures.
Twenty-four hours after enclosure establishment, 1- to 2-day-old adult females of T. urticae (strain W-GR) or O. pratensis were introduced into each enclosure. Briefly, the females were collected from mite populations synchronized on detached B73 maize leaves as described by Bui et al. (2018). Ten O. pratensis or eight T. urticae W-GR females were sucked into barrier pipet tips by vacuum (given the small size of mites, and their density on leaves, occasionally several additional mites were captured in tips, but see “effective female” correction below). The mites were then tapped to the bottom of the tips against the barriers, the tops of the tips were cut off to allow mites to escape, and tips were taped to the underside of the leaves between two Tanglefoot barriers (one tip per enclosure) as previously described (Bui et al., 2018; Gill et al., 2020). It takes a few hours for mites to exit tips and start feeding, and some may fail to exit tips, or fall off while exiting. Therefore, the number of females in each enclosure was assessed by visual inspection 1 day after release, and also at the end of the experiment (at 6 days). The number was assessed twice for reproducibility, and to ensure that a mite was not overlooked at day one by visual inspection, which is a challenge given spider mites’ small size. The greater of these counts was considered to be the number of females that had successfully entered a given enclosure (hereafter effective females). Six days after adding tips with mites to enclosures, individual enclosures were collected by cutting immediately adjacent to Tanglefoot barriers, and the resulting leaf sections were transferred to 4°C (which arrests mite reproduction and development). The total number of progeny (eggs and viable mites, all stages) in each enclosure was then determined under a dissecting microscope and normalized on a per enclosure basis by the count of effective females. The timepoint of 6 days was selected for sample collection because the 6-day time interval allowed mites to produce many progeny on susceptible maize plants, but not so many that accurate counts of mites within enclosures became impracticable.
The scope of screening 38 maize lines for resistance against two spider mite species imposed several constrains on the experimental design. Screening was performed in two batches (batches 1 and 2, see Figure 1); moreover, for logistical reasons we did not use a randomized design for the survey of the 38 lines (although within each batch, resistance to both mite species was assessed simultaneously for each maize line). To allow inter-batch reproducibility to be assessed, however, four maize lines were included in both batches (Figure 1 and section “Results”).
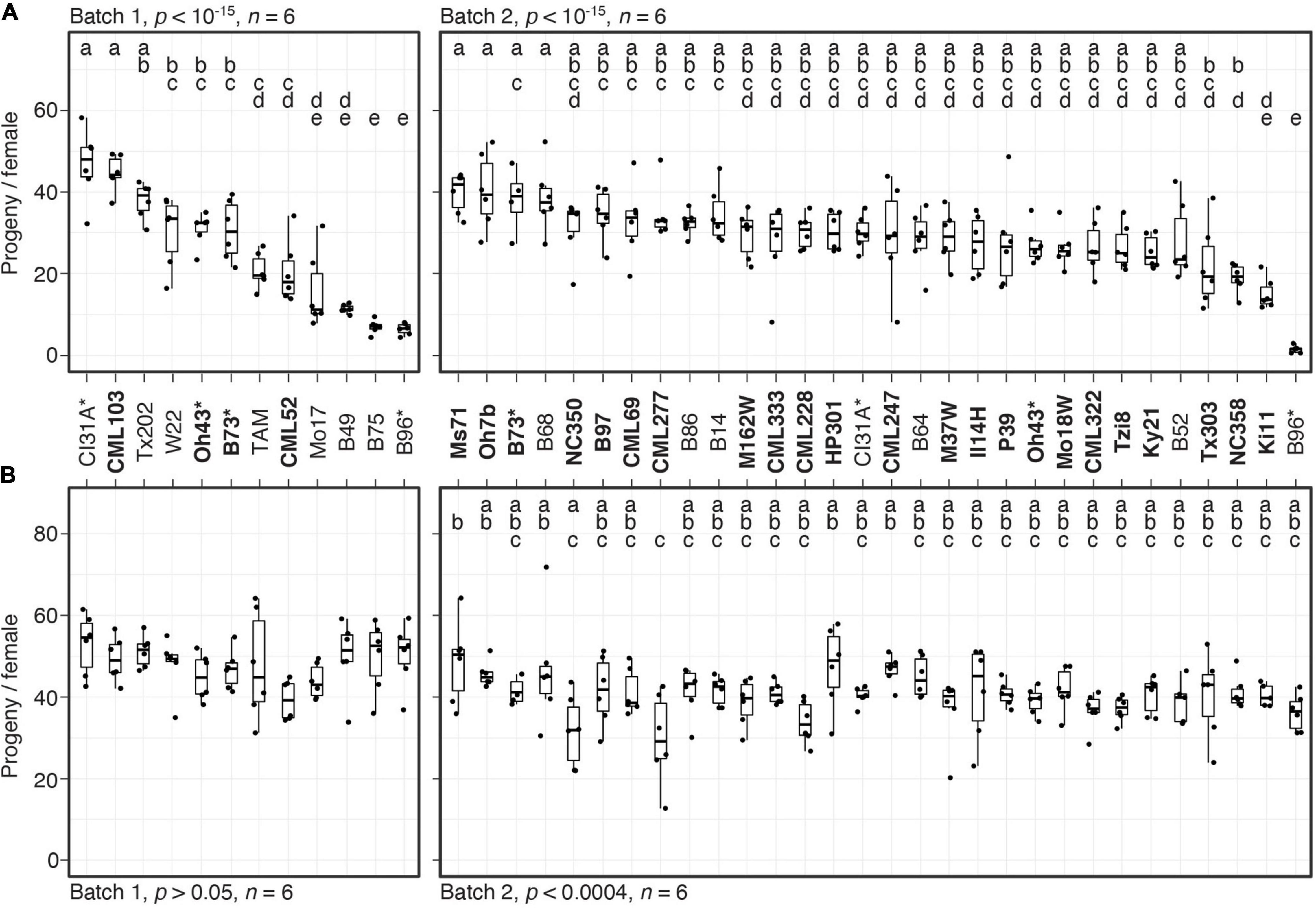
Figure 1. Variation in resistance among 38 maize inbred lines to T. urticae and O. pratensis. Indicated is the number of progeny per adult T. urticae female (A) and O. pratensis female (B) by maize line after 6 days of feeding in enclosures on the 8th leaves (boxplots with overlay of data points are shown). The screen was performed in two batches, 1 and 2 (left and right in each panel, respectively). In (A), maize lines are ranked by median resistance – least to most, left to right – within each batch; to facilitate comparisons between mite species, the same ranking is used in (B). Where significant ANOVA results (p < 0.05) were obtained by batch, pairwise significance was assessed with correction for multiple testing using the Hochberg method (different letters denote significant differences at p < 0.05). Maize line founders of the NAM population are in bold, and four lines included in both batches are indicated by asterisks. Sample size, n (enclosures per maize line, see section “Materials and Methods”), are as indicated except for maize lines B73 (A,B), B52 (B), or Ki11 (B) in batch 2, where either four or five enclosures were successfully established. TAM, TAM-MITE1.
Validation of Spider Mite Resistance
Spider mite resistance for lines B49, B73, B75, and B96 was subsequently assessed at a second site (a Utah State University greenhouse) using four replicates per maize line per mite species in a completely randomized design. Seeds were sown in 22.5 cm pots using Sunshine 3 soil (Sun Gro® Horticulture, Fillmore, UT, United States). Each pot was automatically fertigated (irrigation + fertilizer) at the rate of 0.3 L/day using 21–5–20 water soluble fertilizer mixture (4.8 kg/100 L of water, Peters Excel supplied by ICL Specialty Fertilizers, Summerville, SC, United States). Plants were kept at approximately 25°C with a 16 h-light/8 h-dark photoperiod cycle. When plants were 8-weeks old, Tanglefoot enclosures of length 15 cm were established on the 8th and 9th leaves (the enclosures were centered on leaf midpoints). Twenty adult female mites from either the T. urticae W-GR strain or the O. pratensis strain collected from whole B73 plants (see Materials and Methods section “Spider mite stocks and propagation”) were then introduced into the enclosures. Mite collection into barrier tips, and placement on leaves, was as for the initial survey of the 38 lines. After 6 days of mite infestation, the leaf samples inside each Tanglefoot enclosure were collected and frozen (to stop mite development and reproduction). The number of progeny (eggs and mites of any stage) in each enclosure were subsequently counted after leaf samples were thawed; progeny per female was then calculated on a per plant basis (the sums of the progeny in enclosures on the 8th and 9th leaves were divided by 40).
Performance of Five Inbred T. urticae Strains on Maize Inbreds
The productivity of five additional T. urticae strains (inbred strains Catnip, KH, NightS, ShCo, and WGDel) on maize lines B49, B73, B75, and B96 was assessed in greenhouse bays at the University of Utah. To allow a comparison to earlier findings, the T. urticae W-GR strain was also included. Prior to collecting females for use in resistance screening, mites from the five inbred strains, for which stocks were maintained on detached bean leaves, were passaged for ∼2 generations on detached B73 maize leaves for physiological adaptation. Subsequently, the experimental design was identical to that used for the initial survey of the 38 maize lines, except that single enclosures per plant were established on the 8th leaves, six replicates (single plants) were used per T. urticae strain and maize inbred line, and a completely randomized design was employed.
Design for Resistance Mapping, Phenotyping, and Sample Preparation
To localize maize resistance loci to T. urticae in F2 populations derived from B49 × B73, B75 × B73, and B96 × B73 crosses, we used bulked segregant analysis (BSA) genetic mapping (Zou et al., 2016; Kurlovs et al., 2019). For phenotyping, in sum 400 plants of each F2 population were sown to evaluate T. urticae resistance. However, to provide independent trials, and given the complexity of the experimental set up (establishing leaf enclosures, mite collections, and phenotyping), experiments by cross were performed with two subsets of 200 plants each sown and phenotyped at different times (hereafter termed replicates); these replicates were grown in greenhouse bays at the University of Utah. For each of the three crosses each pair of replicates was planted within 4 months of each other. For each replicate by cross, seeds were sown in 5 × 5 cm plastic pots with Metro-Mix 900 growing mix soil. Ten days after sowing, the seedlings were transplanted into 20 cm diameter pots and watered and fertilized as described previously. Tanglefoot enclosures of 6.25 cm in length were established on the 4th leaves of 3-week-old plants. Forty adult females of the T. urticae W-GR strain, collected from B73 plants, were released into each enclosure using barrier pipet tips as already described. Six days after infestation, the extent of mite feeding damage in each enclosure was scored using a visual scale (one for the least damage, seven for the heaviest damage). Each plant was scored by at least two project participants to produce an average score. If, in a given enclosure, more than 10 mites (25% of the released mites) were observed to have been trapped in a pipet tip, the sample was excluded from further analyses. Subsequently, damage scores were sorted to identify the 50 most sensitive plants (heavier damage, higher score) and 50 most resistant plants (lesser damage, lower score). For DNA preparation, single 4 mm leaf punches from all plants in a given pool were collected, combined, and DNA was extracted using the DNeasy PowerPlant Pro kit (Qiagen, Germantown, MD, United States) following the manufacturer’s recommendations. Illumina DNA-seq libraries of 550 bp average insert sizes were then constructed at the High Throughput Genomics Core Facility at the University of Utah and 125-bp paired-end DNA reads were generated on an Illumina HiSeq 2500 sequencer to generate between 26.8 and 59.3 million read pairs per sample (∼2.8 to 6.2-fold coverage depth for the maize genome).
Read Alignment, Variant Calling, and BSA Scans
We used the QTLseqr program (version 0.7.2) (Mansfeld and Grumet, 2018) which implements the G’ measure of genetic differentiation (Magwene et al., 2011) to detect differences in allele frequencies between pools that, when assessed with a false discovery rate (FDR) generated by QTLseqr, identifies QTL regions. The input for the G’ method is genotypic information at single nucleotide polymorphism (SNP) sites that is assessed from respective allele depths of aligned sequence reads. Reads from pooled samples were aligned to the B73 maize reference genome version AGPv4 (Jiao et al., 2017) using the default settings of BWA (version 0.7.15-r1140) (Li, 2013), and sorted by position using SAMtools 1.3.1 (Li et al., 2009). SNP detection was performed with the Genome Analysis Toolkit (GATK) version 3.6-0-g89b7209 (McKenna et al., 2010); in accordance with GATK best practices recommendations (Van der Auwera et al., 2013), duplicate reads were marked using Picard Tools 2.6.0-SNAPSHOT1 prior to indel realignment with GATK. Because QTLseqr only allows the specification of two samples for a given analysis (“HighBulk” and “LowBulk,” respectively), SAMtools was used to merge the resistant and sensitive replicates for analyses using both replicates by maize line. SNPs were called using the GATK UnifiedGenotyper tool for each bulked-segregant experiment, with SNPs for the individual and combined replicates called separately for each cross. The GATK VariantsToTable tool was used to generate tab-delimited table files (with the following fields: CHROM, POS, REF, ALT, AD, DP, GQ, and PL) from each of the respective UnifiedGenotyper variant call format (VCF) files to produce the required input for QTLseqr.
For each of the six resultant input table files by replicate (i.e., two replicates for each of the three crosses), reads were filtered by adapting QTLseqr recommendations (Mansfeld and Grumet, 2018). Briefly, depending on sequencing depth by sample, the following SNP read coverage requirements were used: a minimum depth across both pools in a replicate of 6–8, a maximum depth of 24–36, a maximum depth difference between the resistant and sensitive pools of 6–8, and minimum sample depths of 3–4 (these parameters resulted in filtered SNP counts of between 1.90 and 4.78 million among replicates across the three crosses). In combining replicates by cross, the respective parameters were doubled, resulting in filtered SNP counts by cross of between 3.15 and 6.58 million. Further, in all cases, a reference allele frequency cutoff of 0.2 and a minimum genotype quality of 20 were used. Following SNP filtering, G’ analyses with window sizes of 10 million bp were used, with outliers filtered using Hampel’s method, to identify QTL regions (Mansfeld and Grumet, 2018). In all cases, a FDR of 0.01 was applied to identify QTL.
Haplotype Analyses
To assess haplotype similarities between B49, B75, and B96, we generated between 255.2 to 319.2 million Illumina 125-bp paired-end reads for these three maize inbred lines (∼26.6 to 33.2-fold coverage depth), aligned the reads to the B73 genome, and performed variant detection (the same methods used for the BSAs were employed). Regions of haplotype similarity between B49, B75, and B96 were then detected in pairwise comparisons of SNPs using 5 Mb sliding windows with 500 kb offsets. For this analysis, 5.69 million high-confidence SNPs identified across all three lines were selected with a filtering scheme modified from GATK documentation specifying guidelines for hard-filtering. SNPs included in the analysis had to have: (1) read coverage depth (which is described by the AD field in the variant call format v. 4.2) between 0.25- and 1.5-fold of the genome wide average (to select against copy variable regions), (2) quality score normalized by allele coverage depth (QD field) of at least two, (3) maximum strand odds ratio (SOR) of three, (4) minimum mean mapping quality score (MQ) of at least 50, (5) minimum mapping quality rank sum score (MQRankSum) of –8, and (6) rank sum test for relative positioning of reference versus alternative alleles within reads (ReadPosRankSumTest) of at least –8. For each sliding window haplotype comparison between pairs of lines, 20% of SNPs had to pass the above-listed quality control criteria in the genomic window in a given pair of lines, and greater than 20 variable sites had to be present.
QTL Confirmation by Single-Locus Genotyping
A QTL interval for T. urticae resistance was characterized by genotyping F2 individuals for which phenotypic data was available (BSA replicate two for cross B49 × B73, and replicate one for cross B75 × B73). For genotyping, a co-dominate PCR marker at 73.4 Mb on chromosome 6 that distinguished a small indel between B73 and both B49 and B75 was selected by inspection of Illumina read data. DNA was isolated from 2 mm leaf punches from frozen leaf tissue collected from individual plants using the Extracta DNA Prep kit and protocol (Quantabio, Beverly, MA, United States). PCR was performed with forward (5′-GCAGCCAGCAAGAAGAAGTCC-3′) and reverse (5′-CACAGGTCGTAGTTAGTATTCC-3′) primers using Taq polymerase, dNTPs and standard buffer (New England BioLabs, Ipswich, MA, United States) with 35 cycles of 95°C for 30 s, 52°C for 30 s, and 68°C for 60 s. Genotypes were assessed following resolution of PCR products on 4% agarose gels stained with ethidium bromide. The use of frozen tissue with the Quantabio DNA isolation method did not lead to successful amplification from all samples, and where amplified bands were faint (especially for heterozygotes), genotype calls were not attempted (genotype calls were performed blindly to knowledge of the plant phenotype, however).
Meta-Analysis of a Resistance QTL Interval
To further confirm and resolve the location of a T. urticae resistance QTL in an interval of a shared haplotype between B49, B75, and B96 on chromosome 6 (see section “Results”), read alignments for all BSA sensitive pools (two replicates each for each of the three crosses of B49, B75, and B96 to B73) were combined using SAMtools (Li et al., 2009). The respective merging of alignments was also performed for the resistant pools separately. From each resulting alignment file, the Python module “pysam” (version 0.15.0)2 (Li et al., 2009) was used to extract the base counts from reads overlying 34,756 SNP positions that defined a shared haplotype extending from 7,895,601 to 109,684,471 bp on chromosome 6 (the SNPs used are from the analysis described in section “Haplotype analyses”). Briefly, at each variable position, bases in aligned reads corresponding to mite sensitive B73 were counted as sensitive, and those from B49, B75, and B96 were counted as resistant. In a sliding window analysis using 5 Mb windows with 1 Mb offsets, the difference in the frequency of resistant counts between the two pools (resistant pool compared to the sensitive pool) was then assessed. In a resulting plot of the allele frequency differences, the chromosome 6 centromere was indicated using the location reported in Schnable et al. (2009).
Statistical Analyses
Statistical analyses of phenotypic data were performed by analysis of variance (ANOVA) or regression (see section “Results”) using the R language (R Core Team, 2016). Where ANOVA analyses were significant, pair-wise t-tests were performed with correction for multiple testing using the Hochberg method. Where boxplots were used for data visualization, the jitter option in the R package ggplot2 (Wickham, 2016) was employed (a small unit of random variation was added per data point to avoid the plotting of overlapping points). Display items produced with R, or those output from the QTLseqr program, were adjusted using Adobe Illustrator (Adobe, San Jose, CA, United States).
Results
Variation in Mite Resistance in Maize
We evaluated mite resistance by surveying the number of progeny per female produced on enclosures on the 8th leaves of 38 inbred maize lines that included founders of the maize NAM population (McMullen et al., 2009), as well as lines previously reported to be resistant to T. urticae, O. pratensis, or both (NAM founders are indicated in bold in Figure 1; see section “Materials and Methods”). Given the scope of the screen, resistance tests were performed in two batches (batches 1 and 2), and four lines were included in both batches to assess reproducibility (B73, B96, CI31A, and Oh43). Significant variation in resistance to T. urtiace strain W-GR was observed in both batches (ANOVA, p < 10–15 for each batch, Figure 1A; for display by batch, lines are ordered from least to most resistant). In each batch, B96 was the most resistant maize line when assessed by median progeny per female of T. urticae, and in general progeny per female for lines replicated in both batches were similar. In batch 1, three other lines, B75, B49, and Mo17, were not significantly different from B96 (t-tests, p < 0.05, Hochberg correction for multiple testing). Apart from B96 and B49, resistance levels to W-GR for other maize lines previously reported to exhibit antibiosis to T. urticae, including Oh43 and TAM-MITE1 (see section “Materials and Methods”), were generally representative of the majority of the 38 lines tested. In contrast to the findings for T. urticae, we did not observe marked variation in resistance to O. pratensis (an ANOVA was only significant for batch 2, p < 0.0004), and median values for O. pratensis progeny per female only ranged from ∼30 to 50, including for the inbred lines that exhibited the highest levels of resistance to T. urticae (i.e., B49, B75, and B96, compare Figures 1A,B).
For follow-up studies, we focused on the three most T. urticae resistant lines, B49, B75, and B96, as defined by lowest median values of progeny per female (Figure 1A). To validate the resistance profiles for these lines, along with susceptible B73 (Figure 1A, batches 1 and 2), we assessed resistance to both T. urticae and O. pratensis at a second greenhouse location. Compared to the initial screen of 38 lines, we used larger leaf enclosures with more mites, which were added to both the 8th and 9th leaves of individual plants in a completely randomized design. Overall, progeny per female for each line was lower than observed in the initial screen of the 38 lines, potentially reflecting environmental variation between locations, or differences in methods (compare Figure 2 to Figure 1, and see section “Materials and Methods”). Nevertheless, relative resistance among the four maize lines was identical to that observed in the initial screening. Briefly, for T. urticae, significant variation in resistance was observed (ANOVA, p < 10–4); while B49, B75, and B96 were not significantly different from each other, all were significantly more resistant than B73 (t-tests, p < 0.05, Hochberg correction for multiple testing; Figure 2A). In contrast, no significant variation in resistance to O. pratensis was detected (ANOVA, p > 0.05; Figure 2B).
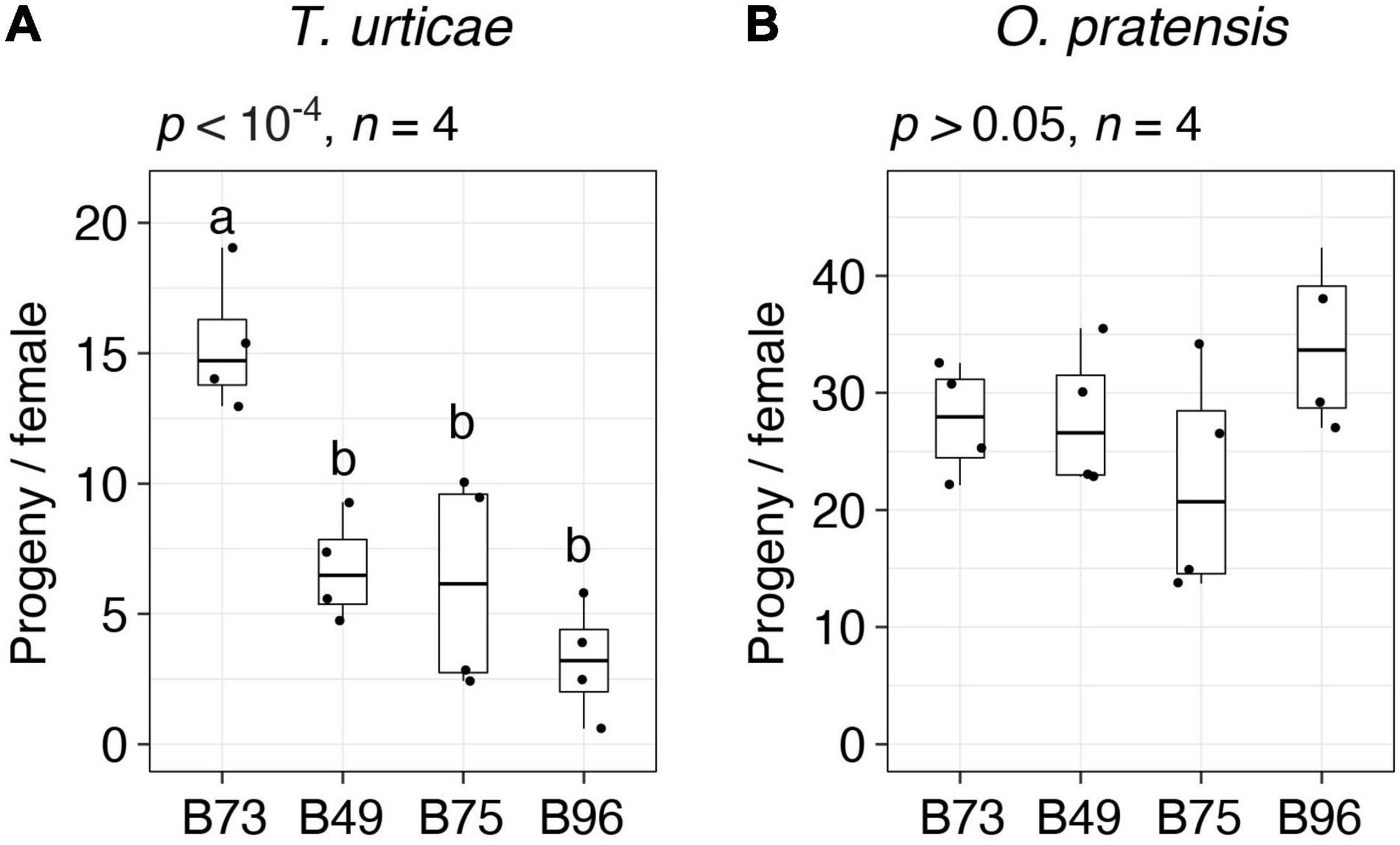
Figure 2. Spider mite resistance profiles for B73, B49, B75, and B96. Indicated is the number of progeny per adult T. urticae female (A) and O. pratensis female (B) for each of the four maize lines after 6 days of feeding in enclosures on the 8th and 9th leaves (boxplots with overlay of data points are shown; sample size, n, refers to the number of plants used). Where significant variation was observed by ANOVA (p < 0.05), pairwise significance was assessed with correction for multiple testing using the Hochberg method (different letters denote significant differences at p < 0.05).
B49, B75, and B96 Are Resistant to Multiple T. urticae Strains
Prior to the current study, the outbred W-GR strain of T. urticae had been maintained on B73 maize plants for at least 15 generations. Host adaptation over a modest number of generations has been documented for T. urticae populations (Sousa et al., 2019), and it is possible that adaptation of W-GR to B73 might have impacted its relative performance on other maize lines, including B49, B75, and B96, in comparison to non-adapted T. urticae strains. To assess the generality of our findings with the T. urticae W-GR strain, we therefore determined resistance for B49, B75, and B96, along with susceptible B73, to five unrelated inbred T. urticae strains with no known history of long-term propagation on maize. In a completely randomized design that included the four maize lines, the five inbred T. urticae strains, and the outbred T. urticae W-GR strain for reference, we observed high resistance for B49, B75, and B96 to every T. urticae strain tested (Figure 3). On a mite strain basis, all analyses of maize resistance were significant (ANOVA, each p < 0.01), with B49, B75, and B96 always significantly more resistant to T. urticae than B73 (t-tests, p < 0.05, Hochberg correction for multiple testing). For each of the six mite strains, no significant differences in resistance were observed between B49, B75, and B96.
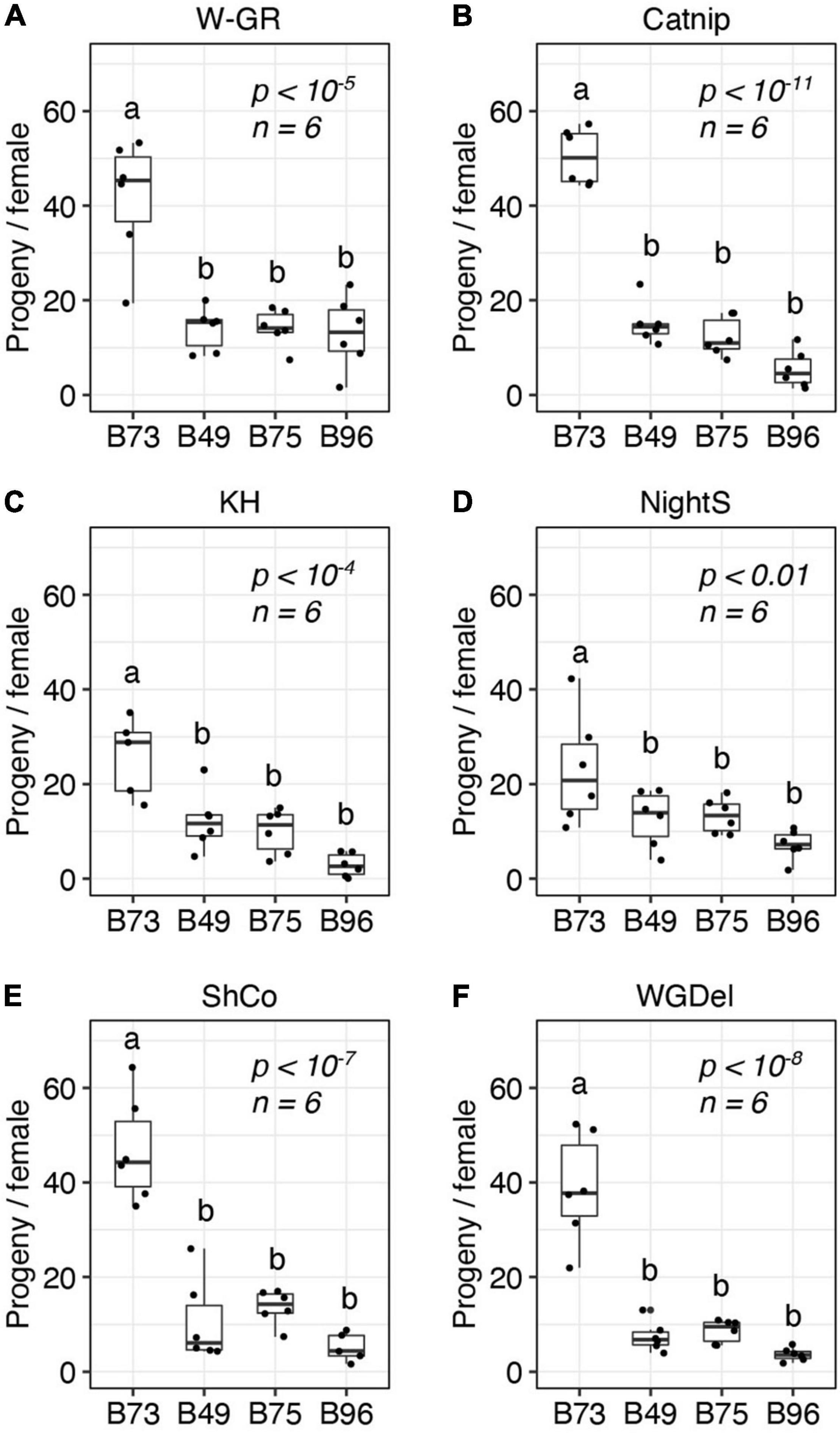
Figure 3. B49, B75, and B96 are resistant to multiple strains of T. urticae. The number of progeny per adult T. urticae female after 6 days of feeding is given for each of six unrelated T. urticae strains for each of four maize lines (A–F) (boxplots with overlay of data points are shown; each plot is titled by the name of the T. urticae strain tested). Assays were performed on the 8th leaves of maize plants. Where significant variation was observed by ANOVA by mite strain (p < 0.05), pairwise significance was assessed with correction for multiple testing using the Hochberg method (different letters denote significant differences at p < 0.05). Sample size (n) is as indicated, except for the B73/KH (C) and B96/ShCo (E) comparisons for which one less enclosure was successfully established (n = 5).
Genetic Basis of T. urticae Resistance in Lines B49, B75, and B96
To identify genomic regions in maize associated with T. urticae resistance, we performed BSA genetic mapping with F2 populations derived from crosses of resistant B49, B75, and B96 to susceptible B73. For each F2 population, screening with T. urticae strain W-GR was performed with two replicates of 200 plants each, with pools of extremes of resistance (most and least resistant plants) consisting of 50 individuals each. For phenotyping, we applied a visual damage scale to tissue within enclosures (1–7, most resistant to least resistant, respectively; see Supplementary Figure 1A, and section “Materials and Methods”). Phenotyping of plant damage, in contrast to scoring mite progeny, was employed because counting mite progeny from 1200 enclosures was not practical. Nevertheless, when assessed with a subset of plants from a B49 × B73 cross for which we also counted progeny, the visual scale based on plant damage reflected mite productivity (R2 = 0.83, p < 10–15, Supplementary Figure 1B), the measure of resistance by antibiosis employed to identify the T. urticae resistance lines (Figures 1–3).
With combined data from both replicates by cross, and using the QTLseqr package, at an FDR of 0.01 we detected a QTL for T. urticae resistance on chromosome 6 centered at ∼60 Mb in all three crosses (Figure 4). For the B49 × B73 and B75 × B73 crosses, this QTL was the only major QTL detected. In addition, for B96 a second QTL with similar G’ peak values (see section “Materials and Methods”) was apparent that extended across a larger genomic region than for the chromosome 6 QTL, from ∼29 to 167 Mb on chromosome 1 (Figure 4C). In all of these cases, alleles contributed to the mapping populations by the three T. urticae resistant maize lines were enriched in the resistant BSA pools in the respective QTL intervals. When we performed the same analyses using each replicate for each cross individually, we obtained the same or similar results. For instance, for each independent replicate in each cross, the chromosome 6 region was identified as a significant QTL, and in the B96 × B73 cross, so was the region on proximal chromosome 1 (Supplementary Figures 2–4). In several cases, in either the analyses with combined replicates, or in the analyses with individual replicates, G’ values reached or nearly reached the QTL detection threshold elsewhere in the genome. One genomic region in which the G’ values narrowly exceeded the QTL detection threshold was on chromosome 5 in both the B75 × B73 and B96 × B73 crosses when assessed with both replicates (Figures 4B,C), or for one replicate of the B96 × B73 cross but not the other (Supplementary Figure 4). Regardless, in the analyses with both replicates (Figure 4), the magnitude of all G’ peaks, except those on chromosomes 1 and 6, was minor (see also Discussion).
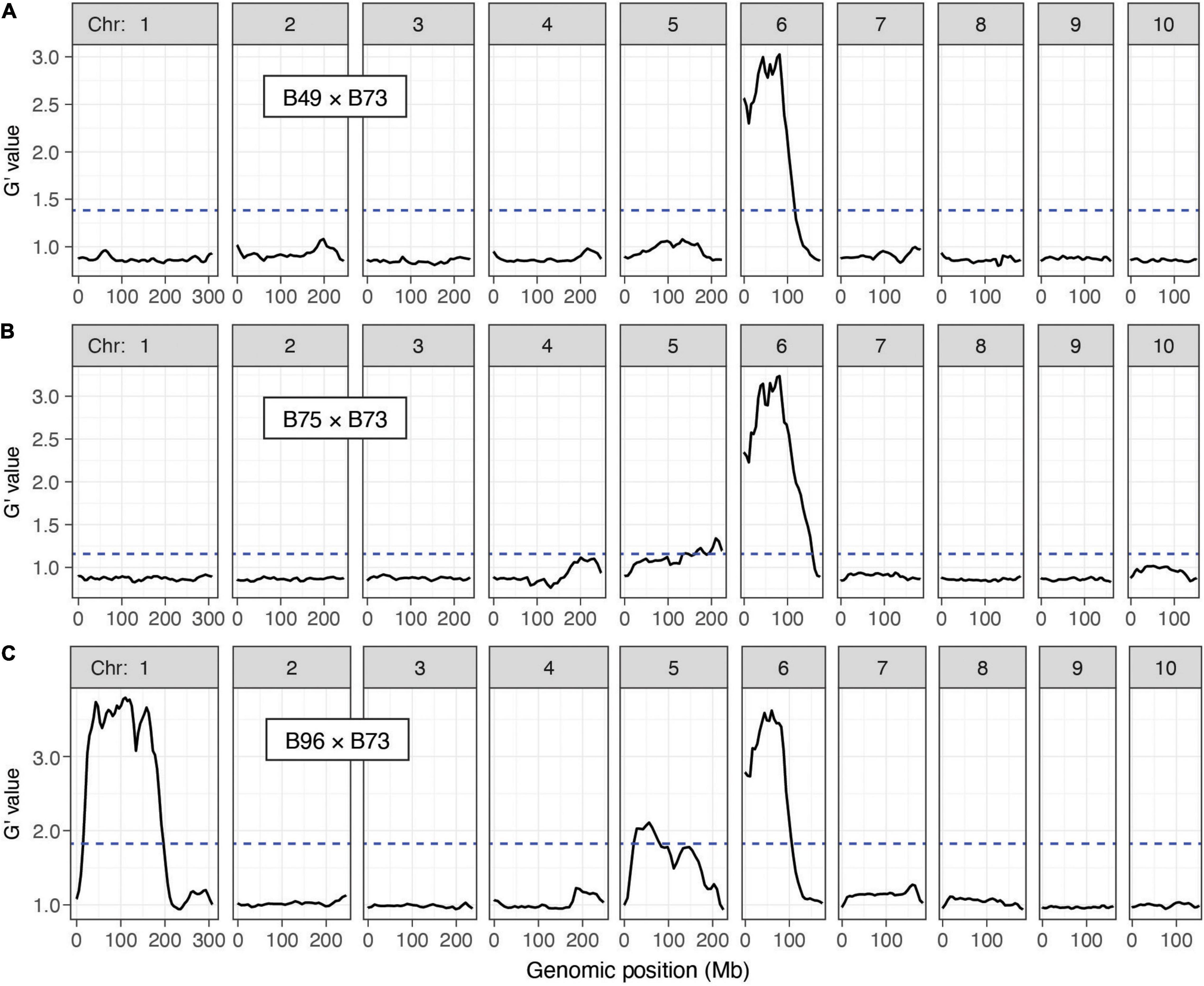
Figure 4. Resistance QTL to T. urticae as identified by BSA genetic mapping in F2 maize populations. Shown are plots of G’ obtained from contrasting pools of T. urticae strain W-GR resistant and sensitive plants from crosses of resistant maize lines B49, B75 and B96 to susceptible line B73 (A–C, respectively; chromosomes 1–10 are as indicated, left to right). Deflections in G’ values exceeding a genome-wide false discovery rate of 0.01 (dashed lines) identify QTL. Extent of resistance was based on leaf damage scores on the 4th leaves, and for each cross the panels represent the combined analysis of two experimental replicates (combined, 100 plants in each of the resistant and susceptible pools of phenotypic extremes; see section “Materials and Methods”). Respective individual analyses for each of the two replicates by cross, B49 × B73, B75 × B73, and B96 × B73, are given in Supplementary Figures 2–4, respectively. The plots shown were modified from the output of QTLseqr (see section “Materials and Methods”).
To provide additional confirmation of QTL detection by the BSA method, for the B49 × B73 and B75 × B73 crosses we performed single-locus genotyping with a PCR marker centered on the chromosome 6 QTL interval. Using genotypic data for all F2 individuals in a replicate for cross B49 × B73 and a replicate for cross B75 × B73, resistance scores were significantly different among the genotypic classes on chromosome 6 (ANOVA, each p < 10–11; Figure 5). Further, F2 plants homozygous for the resistant genotype coincident with the chromosome 6 QTL peaks were significantly more resistant than plants either homozygous or heterozygous for the respective B73 genotype (t-tests, p < 0.05, Hochberg correction for multiple testing). The latter two genotypic classes were not significantly different from each other, revealing that the resistance locus on chromosome 6 acted recessively. When assessed with the homozygous classes in an R2 analysis, 70.6 and 61.2% of the variances in resistance were explained by the marker genotype in the chromosome 6 QTL region in the B49 × B73 and B75 × B73 crosses, respectively (p < 10–15 in each case). In particular, the distributions of damage scores for the homozygous classes for the B49 × B73 cross were almost non-overlapping, excepting for a prominent outlier that plausibly reflects recombination between the marker and the causal resistance locus (Figure 5A, homozygous B49 bin, upper-most data point). Excluding this outlier, 80.1% of the variance in resistance was explained by the chromosome 6 marker genotype at the QTL peak in the B49 × B73 cross.
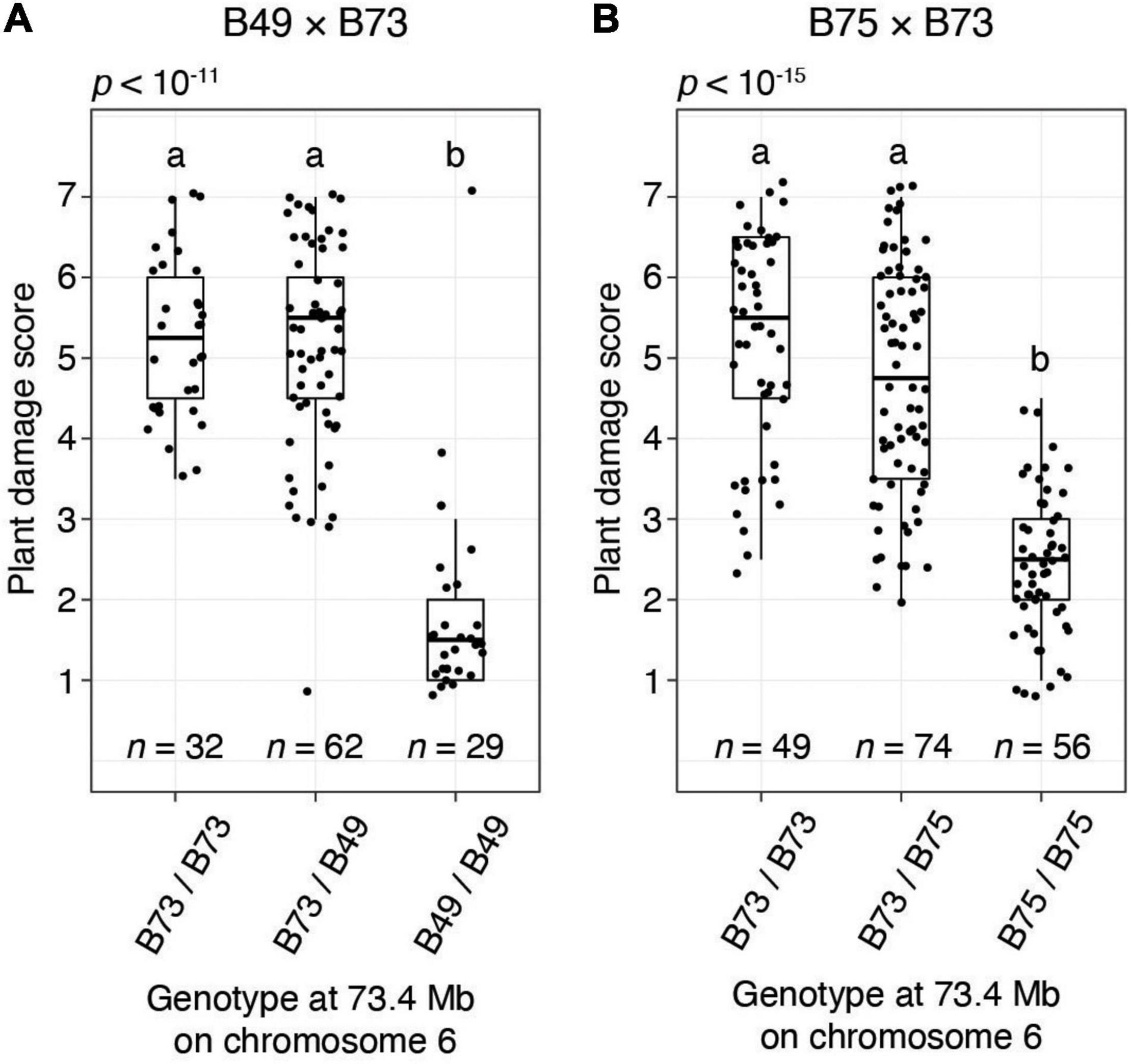
Figure 5. The chromosome 6 T. urticae resistance QTL has a recessive mode of action in B49 and B75 as assessed in crosses to susceptible B73. Shown are boxplots with an overlay of data points for resistance to T. urticae strain W-GR as assessed with a plant damage score and classified by genotype at a locus at 73.4 Mb on chromosome 6 that is within the peak chromosome 6 QTL interval (Figure 4). The data shown are from BSA replicate two of cross B49 × B73 (A) and BSA replicate one of cross B75 × B73 (B). Data for all plants for which genotyping was successful in each replicate population are shown (i.e., not just the plants comprising the pools of phenotypic extremes used in BSA scans, Figure 4 and Supplementary Figures 2B, 3A). Where significant variation was observed by ANOVA by cross (p < 0.05), pairwise significance was assessed with correction for multiple testing using the Hochberg method (different letters denote significant differences at p < 0.05).
A Common Haplotype for the Chromosome 6 QTL for B49, B75, and B96
B49 was derived from a cross with B96 as one parent, while B75 was derived in a breeding program involving 16 parents, including B49 (Portwood et al., 2019). The uncommon occurrence of high-level maize resistance to T. urticae (Figure 1A), the shared ancestry of the three maize lines that are highly resistant to T. urticae, the coincident location of a large-effect resistance QTL on chromosome 6, and the similar mode of action in crosses of B73 to both B49 and B75 (recessive resistance), raised the possibility of a shared genetic basis of maize resistance to T. urtiace for B49, B75, and B96. To assess this possibility, we sequenced the genomes of B49, B75, and B96, and used resulting SNP predictions to identify extended genomic intervals of high similarity (an expectation of recent ancestry) in pairwise comparisons as well as across all three lines (Figures 6A,B, respectively). In a sliding window analysis, and as expected from the B49 pedigree, we found many large regions of the B49 genome that were identical to B96 (Figure 6A). In contrast, only several extended chromosome regions from B75, which is more distantly related to B96, showed a similar pattern. On chromosome 1, no extended regions of haplotype identity were observed between B96 and either B49 or B75 in the interval for the resistance QTL that is unique to B96 (Figure 6A). In contrast, on chromosome 6 a region extending from ∼7.9 to 109.7 Mb was identical (or nearly so) between B49, B75, and B96. This region includes the peaks for the chromosome 6 QTLs identified in each of the individual crosses of B49, B75, and B96 to B73, suggesting that the genetic basis of resistance is the same (compare Figure 6A,B to Figure 4 and Supplementary Figures 2–4). Using high-quality SNPs from the haplotype region (see section “Materials and Methods”), and in a meta-analysis combining resistant and sensitive BSA pool data from all crosses and replicates (Supplementary Figures 2–4), the chromosome 6 peak in allele frequency difference spans a region from ∼31 to 86 Mb (Supplementary Figure 5), consistent with the approximate peak maxima identified in the G’ analyses (Figure 4 and Supplementary Figures 2–4). Within this region, the shift in allele frequencies that define the peak QTL interval forms a plateau (Supplementary Figure 5), a possible consequence of reduced recombination nearby the chromosome 6 centromere located at ∼50 Mb (Schnable et al., 2009).
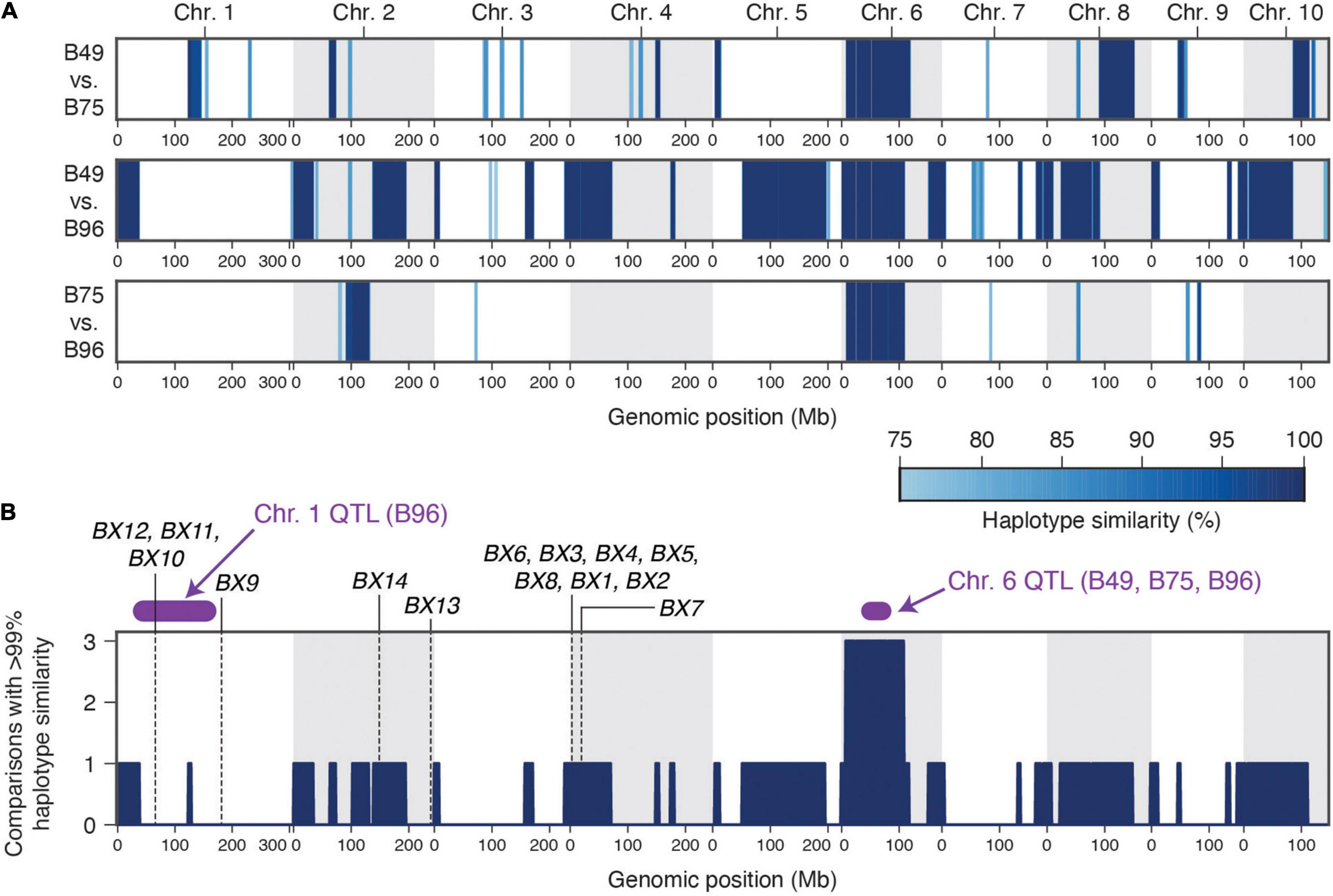
Figure 6. A single haplotype on chromosome 6 associates with high-level resistance to T. urticae in maize lines B49, B75, and B96. (A) Haplotype similarities as assessed with SNP data in sliding window analyses are shown for all pairwise comparisons between B49, B75, and B96. The depth of blue shading indicates the % haplotype similarity (see scale, lower right). (B) Genomic regions for which the identical (or nearly identical) haplotypes are observed in multiple comparisons among the three resistance maize lines (comparisons with > 99% similarity, dark blue). The location of peak genomic intervals for major QTL identified on chromosome 1 (B96) and chromosome 6 (B49, B75, and B96) (Figure 4 and section “Results”) are in purple as indicated, and the location of known benzoxazinoid biosynthetic genes (or gene clusters) are indicated by vertical dashed lines. In both panels, chromosomes are demarcated by alternating white and gray backgrounds.
Candidate Genes in Resistance QTL Intervals
Consistent with the expectation for genetic mapping using F2 populations, the approximate QTL peak regions for T. urticae resistance on chromosomes 1 and 6 include large genomic intervals as defined by the peak regions of the BSA scans. The QTL intervals on chromosome 1 and chromosome 6 extend from ∼29–167 Mb (138 Mb) and ∼31–86 Mb (55 Mb) (Figures 4, 6B and Supplementary Figure 5) and include 2,645 and 567 annotated protein coding genes, respectively. To date, the only endogenous maize plant defenses implicated in antibiosis to T. urticae are benzoxazinoids (Bui et al., 2018). While no genes encoding benzoxazinoid biosynthetic enzymes are located on chromosome 6, the BX10, BX11, and BX12 biosynthetic gene cluster is located at ∼67 Mb on chromosome 1, and is therefore within the peak interval for the B96 chromosome 1 QTL (Figure 6B). Another benzoxazinoid biosynthetic gene, BX9, which is located at 182.3 Mb on chromosome 1, is distal to the peak chromosome 1 QTL region.
Discussion
Previous work has suggested that while most maize lines are comparatively resistant to T. urticae at the seedling stage, they subsequently become susceptible (Mansour et al., 1993; Tadmor et al., 1999). This inversely correlates with constitutive benzoxazinoid levels, which impact T. urticae (Bui et al., 2018), are high in seedlings of many lines, and begin to decrease rapidly by the ∼2–3 leaf stage (Bing et al., 1990b), reflecting changes in defensive programs when maize plants transition from seedlings to mature plants. Nevertheless, moderate- to high-level resistance in some maize lines to both T. urticae and O. pratensis herbivory has been reported in studies that included plants at older stages (Kamali et al., 1989; Mansour et al., 1993; Tadmor et al., 1999; Bynum et al., 2004a), an important consideration because spider mites invade fields throughout the growing season to cause damage of economic importance on more mature plants (Peairs, 2010). In our study, we assessed reproduction of both T. urticae and O. pratensis on 38 maize lines at an agriculturally relevant post-seedling stage (the 8th leaf). Our findings were broadly consistent with some, but not all, earlier studies.
Consistent with prior screens of maize lines for resistance by antibiosis to T. urtiace, we found that most maize lines were comparatively susceptible to T. urticae, but we nonetheless identified a small number of lines, including B49, B75, and B96, that were highly resistant to the W-GR T. urticae strain. Our results confirm that B96 exhibits high antibiosis to T. urticae, a finding reported previously from both laboratory and field studies that used post-seedling stages, and unrelated mite strains (Kamali et al., 1989; Tadmor et al., 1999). Further, B49 was reported previously to be resistant to T. urticae at the seedling stage (Tadmor et al., 1999); to our knowledge, B75 has not been screened previously for spider mite resistance. While Oh43, a NAM line founder, was reported to be highly resistant to T. urticae at the 4th-leaf stage when assayed with detached leaf segments (Mansour and Bar-Zur, 1992), it was as susceptible as the majority of the other 37 lines screened in the current study. Our observation that Oh43 was not highly resistant may be explained by our use of older plants for screening, consistent with the finding of Kamali et al. (1989) who observed only modest resistance for Oh43 in a field trial using older plants. Alternatively, it might reflect the use of different T. urticae strains, and intra-specific variation in fitness on different plant hosts has been reported for field-derived or laboratory-evolved T. urticae strains (Sousa et al., 2019; Wybouw et al., 2019). The three lines that we found were highly resistant to the T. urticae W-GR strain were, however, also resistant to five unrelated T. urticae strains not known to have been recently propagated on maize (Wybouw et al., 2019; and section “Materials and Methods”). Therefore, resistance loci and alleles from B49, B75 and B96 are likely to be generally applicable to efforts to develop T. urticae resistant maize varieties.
Despite their resistance to T. urticae, neither B49, B75, B96, nor any of the other 35 maize lines we studied, exhibited marked antibiosis to the O. pratensis strain used in our study. This contrasts with the finding for T. urticae, but is consistent with Bui et al.’s (2018) work suggesting that the generalist T. urticae and the grass-specialist O. pratensis respond differently to maize defenses, as well as with the plausible expectation that the grass-specialist O. pratensis may have evolved more robust mechanisms to overcome the defenses of its host than the generalist herbivore. Additionally, it should be noted that although resistance by antibiosis to O. pratensis has been reported for some maize lines, in a number of cases resistance arising from tolerance was demonstrated or is plausible (Owens et al., 1976; Bynum et al., 2004a).
We included the founders of the maize NAM population in our study because this genetic resource has facilitated the identification of QTL for resistance to insect herbivores (Meihls et al., 2013; Tzin et al., 2015). However, our most T. urticae resistant maize lines were not NAM founders, so instead we exploited BSA genetic mapping to identify resistance QTL in B49, B75, and B96. It should be noted that given the scope of the BSA screens, of necessity we used younger plants, and we used a plant damage metric to assess resistance. However, in one cross (B49 × B73) we showed that plant damage was highly correlated with mite reproduction, suggesting that our BSA scans assessed resistance arising from antibiosis (Supplementary Figure 1B). Each of the three lines harbored a large-effect QTL on chromosome 6, which when assessed by single-locus genotyping in B49 and B75 crosses conferred recessive resistance, and explained ∼70% of the trait variance (Figure 5). Further, a second resistance QTL on chromosome 1 was unique to B96. In B75 and B96, a possible minor-effect locus or loci, or ones that are environmentally sensitive, may be located on chromosome 5 (i.e., chromosome 5 was identified as a QTL in only one of the two BSA replicates for the B96 × B73 F2 mapping population, which were performed at different times albeit in the same greenhouse bays, Supplementary Figure 4, and section “Materials and Methods”). Nonetheless, a role for chromosome 5 in resistance requires further study.
Our haplotype analyses that used genomic sequence data from each of the three highly T. urtiace resistant maize lines demonstrated that the chromosome 6 QTL was coincident with extended regions of haplotype identity among B49, B75, and B96. Because B96 was a parent of both B49 and B75 (Portwood et al., 2019), B96 may therefore have been the source of the chromosome 6 resistance variant (or variants) in the derived lines, although we cannot rule out a more complex origin during the B49 and B75 breeding programs (something that can be tested when additional and higher quality genomic data become available). The identification of a second unique chromosome 1 resistance QTL in B96 would seem to be at odds with our findings of no statistically significant increases in resistance for B96 as compared to either B49 or B75. Nevertheless, for nearly all cases where we compared resistance between the three lines, B96 had the lowest median number of T. urticae progeny (Figures 1A, 2A, 3), consistent with an additional resistance locus or loci. While few studies have mapped QTL for resistance to spider mites in any plant species, and to our knowledge none previously in grasses, the genetic architecture we observed for maize resistance to T. urticae is not unprecedented (i.e., one or several loci of modest or major effect). In tomato, a QTL for resistance to T. urticae was identified in a study using a recombinant inbred line population between susceptible Solanum lycopersicum L. and a resistant variety of the wild tomato species S. pimpinellifolium L. that explained more than 30% of the trait variance (Salinas et al., 2013).
B96 has been reported to be “nearly immune” to first-generation feeding by the European corn borer, O. nubilalis (Kamali et al., 1989), and was also observed to be highly resistant to a complex of three thrips species (Bing et al., 1990a). While it has poor agronomic properties, B96 was used to produce O. nubilalis resistant B49, B64, and B68, which were used in additional breeding programs, including for the generation of B75 that is also resistant to O. nubilalis (Portwood et al., 2019). B96 has also been reported to have high levels of DIMBOA at late developmental stages (Bing et al., 1990b), as has B75 (Barry et al., 1994), and Mo17, the fourth most T. urticae resistant line we identified (Figure 1A), is also delayed in the transition to the lower benzoxazinoid content of mature maize as compared to B73 (Zheng et al., 2015). The observation that T. urticae reproduction is inhibited by benzoxazinoids (Bui et al., 2018), and that the T. urticae resistant lines in the current study are resistant to O. nubilalis, for which benzoxazinoids are also detrimental (Wouters et al., 2016), raises the possibility of a shared molecular-genetic basis of resistance. However, such conjecture must be interpreted with caution. For example, B64 and B68 were included in our study and were comparatively susceptible to T. urticae, as was line CI31A, which is both resistant to O. nubilalis and is a high DIMBOA content line (Barry et al., 1994; Portwood et al., 2019). One interpretation of these findings is that molecular-genetic resistance mechanism(s), if shared between T. urticae and insects, may only partially overlap. An additional confounding factor is that while DIMBOA content has been reported for some lines in our study, the levels of other benzoxazinoids have not. For instance, the production of the DIMBOA derivative 2-hydroxy-4,7-dimethoxy-1,4-benzoxazin-3-one (HDMBOA) is thought to be especially important for defense against lepidopteran pests (Glauser et al., 2011), and absolute and relative levels of different benzoxazinoid metabolites can vary substantially among maize lines (Meihls et al., 2013). Support for a possible role for benzoxazinoids in explaining intraspecific maize variation for T. urticae resistance comes from the observation that the chromosome 1 QTL region in B96 harbors benzoxazinoid biosynthetic genes in the BX10-12 gene cluster involved in the synthesis of HDMBOA (Meihls et al., 2013). Moreover, although no known benzoxazinoid biosynthetic genes are located on chromosome 6, the peak region for the chromosome 6 QTL common to B49, B75, and B96 harbors the putative transcriptional regulator NACTF21 (at 69.0 Mb on chromosome 6). Recently, NACTF21 was implicated in a large-scale transcriptome network analysis as a potential regulator of benzoxazinoid biosynthetic genes, including BX1 and BX2 (Zhou et al., 2020), whose products perform the first two enzymatic steps required for the synthesis of all benzoxazinoids (Wouters et al., 2016). However, while benzoxazinoid biosynthetic genes and putative trans regulators should be investigated as potentially causal for the chromosome 1 and 6 QTLs, the peak regions identified in the BSA scans contain many genes. This is especially true for the broad QTL region identified on chromosome 1 in B96, potentially indicative of a complex QTL with multiple, linked resistance loci. Ultimately, fine mapping will be required to establish the identity of the causal gene (or genes) in each resistance QTL interval.
Concluding Remarks and Future Directions
Spider mites are threats to field-grown maize, and also other crops, under hot and dry environmental conditions. They are therefore likely to become more damaging in many agricultural regions as climate change intensifies. In this study, we investigated maize resistance to two spider mite species known to be economically damaging to maize in hot and dry growing regions (Peairs, 2010), or more generally when plants are drought stressed (Varenhorst et al., 2012; Al-Kaisi et al., 2013). We found that the genetic basis of maize resistance to the generalist T. urticae and the specialist O. pratensis differ, and we identified QTL regions underlying high-level resistance to T. urticae, the most cosmopolitan spider mite pest of maize that is found on all continents except Antarctica (Migeon and Dorkeld, 2021). The loci we identified should be of use in marker-assisted breeding programs to develop maize lines resistant to this generalist spider mite. Further, the large-effect nature of the QTLs – at least for the chromosome 6 resistance locus – suggests that fine-mapping to identify a specific causal variant (or variants) should be possible, although the proximity to the centromere may increase the challenge. More generally, the QTLs serve as entry points for future genetic studies to assess if the basis of spider mite resistance in maize is shared with that of major insect pests. This is of particular interest because B96, the potential sole origin of high-level resistance haplotypes for T. urticae in the diverse collection of 38 lines we examined, is also among the most resistant known maize lines to the European corn borer, Ostrinia nubilalis, as well as thrips species (Kamali et al., 1989; Bing et al., 1990b).
Code Availability
Custom Python code developed for the project, and used for the analyses presented in Figure 6 and Supplementary Figure 5, is publicly available on GitHub (https://github.com/rmclarklab/B49_B75_B96_haplotypes).
Data Availability Statement
The datasets presented in this study can be found in online repositories. The names of the repository/repositories and accession number(s) can be found below: https://www.ncbi.nlm.nih.gov/genbank/, PRJNA481365, PRJNA556665; https://figshare.com/, https://doi.org/10.6084/m9.figshare.13708375.v2.
Author Contributions
HB, RC, GG, and RR originated the study and experimental design. HB, RC, GG, CR, and SL performed the plant–mite interaction experiments. AK, HB, and RC created mite inbred lines. HB, RG, AK, and MJ performed the bioinformatic and statistical analyses. HB and RC assumed the primary role in writing the manuscript, which was reviewed and approved by all authors. All authors contributed to the article and approved the submitted version.
Funding
This work was supported by USA National Science Foundation Plant Genome Research Program award 1444449 to RC and RR. Additionally, the generation of the inbred mite lines was supported by USA National Science Foundation award 1457346 to RC.
Conflict of Interest
The authors declare that the research was conducted in the absence of any commercial or financial relationships that could be construed as a potential conflict of interest.
Acknowledgments
We thank Christopher Morrow for assistance with greenhouse work, and Gary Drews for helpful suggestions on the study. A preprint of this article was previously made available on bioRxiv (www.biorxiv.org/content/10.1101/2021.02.04.429847v1).
Supplementary Material
The Supplementary Material for this article can be found online at: https://www.frontiersin.org/articles/10.3389/fpls.2021.693088/full#supplementary-material
Footnotes
References
Al-Kaisi, M. M., Elmore, R. W., Guzman, J. G., Hanna, H. M., Hart, C. E., Helmers, M. J., et al. (2013). Drought impact on crop production and the soil environment: 2012 experiences from Iowa. J. Soil Water Conserv. 68, 19A–24A. doi: 10.2489/jswc.68.1.19A
Archer, T. L. (1987). “Techniques for Screening Maize for Resistance to Mites,” in Toward Insect Resistant Maize for the Third World: Proceedings of International Symposium on Methods of Development in Host Plant Resistance to Maize Insects, eds J. A. Mihm, B. R. Wiseman, and F. M. Davis (DF, Mexico: CIMMYT), 178–183.
Archer, T. L., and Bynum, E. D. Jr. (1993). Yield loss to corn from feeding by the Banks grass mite and two-spotted spider mite (Acari: Tetranychidae). Exp. Appl. Acarol. 17, 895–903. doi: 10.1007/BF02328066
Auger, P., Migeon, A., Ueckermann, E. A., Tiedt, L., and Navarro, M. N. (2013). Evidence for synonymy between Tetranychus urticae and Tetranychus cinnabarinus (Acari, Prostigmata, Tetranychidae): review and new data. Acarologia 53, 383–415.
Barry, D., Alfaro, D., and Darrah, L. L. (1994). Relation of European Corn Borer (Lepidoptera: Pyralidae) Leaf-Feeding Resistance and Dimboa Content in Maize. Environ. Entomol. 23, 177–182. doi: 10.1093/ee/23.1.177
Bensoussan, N., Santamaria, M. E., Zhurov, V., Diaz, I., Grbić, M., and Grbić, V. (2016). Plant-Herbivore Interaction: Dissection of the Cellular Pattern of Tetranychus urticae Feeding on the Host Plant. Front. Plant Sci. 7:105. doi: 10.3389/fpls.2016.01105
Bing, J. W., Dicke, F. F., and Guthrie, W. D. (1990a). Genetics of Resistance in Maize to a Complex of Three Species of Thrips (Thysanoptera: Thripidae). J. Econ. Entomol. 83, 621–624. doi: 10.1093/jee/83.2.621
Bing, J. W., Guthrie, W. D., Dicke, F. F., and Obryckp, J. J. (1990b). Relation of Corn Leaf Aphid (Homoptera: Aphididae) Colonization to DIMBOA Content in Maize Inbred Lines. J. Econ. Entomol. 83, 1626–1632. doi: 10.1093/jee/83.4.1626
Bryon, A., Kurlovs, A. H., Dermauw, W., Greenhalgh, R., Riga, M., Grbić, M., et al. (2017). Disruption of a horizontally transferred phytoene desaturase abolishes carotenoid accumulation and diapause in Tetranychus urticae. Proc. Natl. Acad. Sci. U. S. A. 114, E5871–E5880. doi: 10.1073/pnas.1706865114
Bui, H., Greenhalgh, R., Ruckert, A., Gill, G. S., Lee, S., Ramirez, R. A., et al. (2018). Generalist and Specialist Mite Herbivores Induce Similar Defense Responses in Maize and Barley but Differ in Susceptibility to Benzoxazinoids. Front. Plant Sci. 9:1222. doi: 10.3389/fpls.2018.01222
Bynum, E. D., Xu, W., and Archer, T. L. (2004a). Diallel Analysis of Spider Mite Resistant Maize Inbred Lines and F 1 Crosses. Crop Sci. 44, 1535–1541. doi: 10.2135/cropsci2004.1535
Bynum, E. D., Xu, W., and Archer, T. L. (2004b). Potential efficacy of spider mite-resistant genes in maize testcrosses. Crop Protect. 23, 625–634. doi: 10.1016/j.cropro.2003.11.013
Deutsch, C. A., Tewksbury, J. J., Tigchelaar, M., Battisti, D. S., Merrill, S. C., Huey, R. B., et al. (2018). Increase in crop losses to insect pests in a warming climate. Science 361, 916–919. doi: 10.1126/science.aat3466
Gill, G. S., Bui, H., Clark, R. M., and Ramirez, R. A. (2020). Varying responses to combined water-stress and herbivory in maize for spider mite species that differ in host specialization. Environ. Exp. Bot. 177:104131. doi: 10.1016/j.envexpbot.2020.104131
Glauser, G., Marti, G., Villard, N., Doyen, G. A., Wolfender, J.-L., Turlings, T. C. J., et al. (2011). Induction and detoxification of maize 1,4-benzoxazin-3-ones by insect herbivores: Defense induction and detoxification in maize. Plant J. 68, 901–911. doi: 10.1111/j.1365-313X.2011.04740.x
Grbic, M., Khila, A., Lee, K.-Z., Bjelica, A., Grbic, V., Whistlecraft, J., et al. (2007). Mity model: Tetranychus urticae, a candidate for chelicerate model organism. BioEssays 29, 489–496. doi: 10.1002/bies.20564
Howe, G. A., and Jander, G. (2008). Plant immunity to insect herbivores. Annu. Rev. Plant Biol. 59, 41–66. doi: 10.1146/annurev.arplant.59.032607.092825
Jiao, Y., Peluso, P., Shi, J., Liang, T., Stitzer, M. C., Wang, B., et al. (2017). Improved maize reference genome with single-molecule technologies. Nature 546, 524–527. doi: 10.1038/nature22971
Kamali, K., Dicke, F. F., and Guthrie, W. D. (1989). Resistance-Susceptibility of Maize Genotypes to Artificial Infestations by Twospotted Spider Mites. Crop Sci. 29:936. doi: 10.2135/cropsci1989.0011183X002900040020x
Kurlovs, A. H., Snoeck, S., Kosterlitz, O., Van Leeuwen, T., and Clark, R. M. (2019). Trait mapping in diverse arthropods by bulked segregant analysis. Curr. Opin. Insect Sci. 36, 57–65. doi: 10.1016/j.cois.2019.08.004
Li, H. (2013). Aligning sequence reads, clone sequences and assembly contigs with BWA-MEM. arXiv:1303.3997 [q-bio]. Available online at: http://arxiv.org/abs/1303.3997 (accessed August 6, 2018).
Li, H., Handsaker, B., Wysoker, A., Fennell, T., Ruan, J., Homer, N., et al. (2009). The Sequence Alignment/Map format and SAMtools. Bioinformatics 25, 2078–2079. doi: 10.1093/bioinformatics/btp352
Magwene, P. M., Willis, J. H., and Kelly, J. K. (2011). The statistics of bulk segregant analysis using next generation sequencing. PLoS Comput. Biol. 7:e1002255. doi: 10.1371/journal.pcbi.1002255
Mansfeld, B. N., and Grumet, R. (2018). QTLseqr: An R package for bulk segregant analysis with next-generation sequencing. Plant Genome 11:2. doi: 10.3835/plantgenome2018.01.0006
Mansour, F., and Bar-Zur, A. (1992). Resistance of maize inbred lines to the carmine spider mite. Tetranychus cinnabarinus (Acari: Tetranychidae). Maydica 37, 343–345.
Mansour, F., Bar-Zur, A., and Abo-Moch, F. (1993). Resistance of maize inbred lines to the carmine spider mite, Tetranychus cinnabarinus (Acari: Tetranychidae): Evaluation of antibiosis of selected lines at different growth stages. Maydica 38, 309–311.
McKenna, A., Hanna, M., Banks, E., Sivachenko, A., Cibulskis, K., Kernytsky, A., et al. (2010). The Genome Analysis Toolkit: a MapReduce framework for analyzing next-generation DNA sequencing data. Genome Res. 20, 1297–1303. doi: 10.1101/gr.107524.110
McMullen, M. D., Kresovich, S., Villeda, H. S., Bradbury, P., Li, H., Sun, Q., et al. (2009). Genetic properties of the maize nested association mapping population. Science 325, 737–740. doi: 10.1126/science.1174320
Meihls, L. N., Handrick, V., Glauser, G., Barbier, H., Kaur, H., Haribal, M. M., et al. (2013). Natural variation in maize aphid resistance is associated with 2,4-dihydroxy-7-methoxy-1,4-benzoxazin-3-one glucoside methyltransferase activity. Plant Cell 25, 2341–2355. doi: 10.1105/tpc.113.112409
Meihls, L. N., Kaur, H., and Jander, G. (2012). Natural Variation in Maize Defense against Insect Herbivores. Cold Spring Harb. Symp. Q. Biol. 77, 269–283. doi: 10.1101/sqb.2012.77.014662
Migeon, A., and Dorkeld, F. (2021). Spider Mites Web: a comprehensive database for the Tetranychidae. Available online at: http://www1.montpellier.inra.fr/CBGP/spmweb (accessed April 9, 2021).
Migeon, A., Nouguier, E., and Dorkeld, F. (2011). “Spider Mites Web: a comprehensive database for the Tetranychidae,” in Trends in Acarology, eds M. W. Sabelis and J. Bruin (Dordrecht: Springer Netherlands), 557–560. doi: 10.1007/978-90-481-9837-5_96
Owens, J. C., Ward, C. R., and Teetes, G. L. (1976). “Current status of spider mites in corn and sorghum,” in Proceeding. 31st Annual Corn and Sorghum Conference (Dordrecht: Springer Netherlands), 38–64.
Peairs, F. B. (2010). Spider Mites in Corn. Available online at: https://extension.colostate.edu/docs/pubs/insect/05555.pdf (accessed April 9, 2021).
Portwood, J. L., Woodhouse, M. R., Cannon, E. K., Gardiner, J. M., Harper, L. C., Schaeffer, M. L., et al. (2019). MaizeGDB 2018: the maize multi-genome genetics and genomics database. Nucleic Acids Res. 47, D1146–D1154. doi: 10.1093/nar/gky1046
R Core Team (2016). R: A Language and Environment for Statistical Computing. Vienna: R Foundation for Statistical Computing.
Salinas, M., Capel, C., Alba, J. M., Mora, B., Cuartero, J., Fernández-Muñoz, R., et al. (2013). Genetic mapping of two QTL from the wild tomato Solanum pimpinellifolium L. controlling resistance against two-spotted spider mite (Tetranychus urticae Koch). Theoret. Appl. Genet. 126, 83–92. doi: 10.1007/s00122-012-1961-0
Schnable, P. S., Ware, D., Fulton, R. S., Stein, J. C., Wei, F., Pasternak, S., et al. (2009). The B73 maize genome: complexity, diversity, and dynamics. Science 326, 1112–1115. doi: 10.1126/science.1178534
Snowdon, R. J., Wittkop, B., Chen, T.-W., and Stahl, A. (2020). Crop adaptation to climate change as a consequence of long-term breeding. Theor. Appl. Genet. doi: 10.1007/s00122-020-03729-3
Sousa, V. C., Zélé, F., Rodrigues, L. R., Godinho, D. P., Charlery de la Masselière, M., and Magalhães, S. (2019). Rapid host-plant adaptation in the herbivorous spider mite Tetranychus urticae occurs at low cost. Curr. Opin. Insect Sci. 36, 82–89. doi: 10.1016/j.cois.2019.08.006
Tadmor, Y., Lewinsohn, E., Abo-Moch, F., Bar-Zur, A., and Mansour, F. (1999). Antibiosis of maize inbred lines to the carmine spider mite, Tetranychus cinnabarinus. Phytoparasitica 27, 35–41. doi: 10.1007/BF02980725
Tzin, V., Hojo, Y., Strickler, S. R., Bartsch, L. J., Archer, C. M., Ahern, K. R., et al. (2017). Rapid defense responses in maize leaves induced by Spodoptera exigua caterpillar feeding. J. Exp. Bot. 68, 4709–4723. doi: 10.1093/jxb/erx274
Tzin, V., Lindsay, P. L., Christensen, S. A., Meihls, L. N., Blue, L. B., and Jander, G. (2015). Genetic mapping shows intraspecific variation and transgressive segregation for caterpillar-induced aphid resistance in maize. Mol. Ecol. 24, 5739–5750. doi: 10.1111/mec.13418
Van der Auwera, G. A., Carneiro, M. O., Hartl, C., Poplin, R., Del Angel, G., Levy-Moonshine, A., et al. (2013). From FastQ data to high confidence variant calls: the Genome Analysis Toolkit best practices pipeline. Curr. Protoc. Bioinform. 43, 11.10.1–11.10.33. doi: 10.1002/0471250953.bi1110s43
Van Leeuwen, T., Vontas, J., Tsagkarakou, A., Dermauw, W., and Tirry, L. (2010). Acaricide resistance mechanisms in the two-spotted spider mite Tetranychus urticae and other important Acari: A review. Insect Biochem. Mol. Biol. 40, 563–572. doi: 10.1016/j.ibmb.2010.05.008
Varenhorst, A., O’Neal, M., and Hodgson, E. (2012). Early confirmation of twospotted spider mite. Integr. Crop Manag. News. 8:2012.
Wickham, H. (2016). ggplot2: Elegant Graphics for Data Analysis. New York: Springer-Verlag New York.
Wouters, F. C., Blanchette, B., Gershenzon, J., and Vassão, D. G. (2016). Plant defense and herbivore counter-defense: benzoxazinoids and insect herbivores. Phytochem. Rev. 15, 1127–1151. doi: 10.1007/s11101-016-9481-1
Wybouw, N., Kosterlitz, O., Kurlovs, A. H., Bajda, S., Greenhalgh, R., Snoeck, S., et al. (2019). Long-Term Population Studies Uncover the Genome Structure and Genetic Basis of Xenobiotic and Host Plant Adaptation in the Herbivore Tetranychus urticae. Genetics 211, 1409–1427. doi: 10.1534/genetics.118.301803
Zheng, L., McMullen, M. D., Bauer, E., Schon, C.-C., Gierl, A., and Frey, M. (2015). Prolonged expression of the BX1 signature enzyme is associated with a recombination hotspot in the benzoxazinoid gene cluster in Zea mays. J. Exp. Bot. 66, 3917–3930. doi: 10.1093/jxb/erv192
Zhou, P., Li, Z., Magnusson, E., Gomez Cano, F., Crisp, P. A., Noshay, J. M., et al. (2020). Meta Gene Regulatory Networks in Maize Highlight Functionally Relevant Regulatory Interactions. Plant Cell 32, 1377–1396. doi: 10.1105/tpc.20.00080
Keywords: two-spotted spider mite (Tetranychus urticae), Tetranychus cinnabarinus, Banks grass mite, bulked segregant analysis (BSA), antibiosis, Oligonychus pratensis
Citation: Bui H, Greenhalgh R, Gill GS, Ji M, Kurlovs AH, Ronnow C, Lee S, Ramirez RA and Clark RM (2021) Maize Inbred Line B96 Is the Source of Large-Effect Loci for Resistance to Generalist but Not Specialist Spider Mites. Front. Plant Sci. 12:693088. doi: 10.3389/fpls.2021.693088
Received: 09 April 2021; Accepted: 25 May 2021;
Published: 21 June 2021.
Edited by:
Marcelino Perez De La Vega, Universidad de León, SpainCopyright © 2021 Bui, Greenhalgh, Gill, Ji, Kurlovs, Ronnow, Lee, Ramirez and Clark. This is an open-access article distributed under the terms of the Creative Commons Attribution License (CC BY). The use, distribution or reproduction in other forums is permitted, provided the original author(s) and the copyright owner(s) are credited and that the original publication in this journal is cited, in accordance with accepted academic practice. No use, distribution or reproduction is permitted which does not comply with these terms.
*Correspondence: Richard M. Clark, richard.m.clark@utah.edu
†Present address: Huyen Bui, Center for Biofilm Engineering, Montana State University, Bozeman, MT, United States; Gunbharpur S. Gill, Pee Dee Research and Education Center, Clemson University, Florence, SC, United States