- 1Council for Agricultural Research and Economics, Research Centre for Animal Production and Aquaculture, Lodi, Italy
- 2Department of Agricultural, Forest and Food Sciences, Plant Genetics and Breeding, University of Torino, Grugliasco, Italy
- 3Instituto de Biología Molecular y Celular de Plantas, Consejo Superior de Investigaciones Científicas, Universidad Politécnica de Valencia, Valencia, Spain
In the Medicago genus, triterpene saponins are a group of bioactive compounds extensively studied for their different biological and pharmaceutical properties. In this work, the CRISPR/Cas9-based approach with two single-site guide RNAs was used in Medicago truncatula (barrel medic) to knock-out the CYP93E2 and CYP72A61 genes, which are responsible for the biosynthesis of soyasapogenol B, the most abundant soyasapogenol in Medicago spp. No transgenic plants carrying mutations in the target CYP72A61 gene were recovered while fifty-two putative CYP93E2 mutant plant lines were obtained following Agrobacterium tumefaciens-mediated transformation. Among these, the fifty-one sequenced plant lines give an editing efficiency of 84%. Sequencing revealed that these lines had various mutation patterns at the target sites. Four T0 mutant plant lines were further selected and examined for their sapogenin content and plant growth performance under greenhouse conditions. The results showed that all tested CYP93E2 knock-out mutants did not produce soyasapogenols in the leaves, stems and roots, and diverted the metabolic flux toward the production of valuable hemolytic sapogenins. No adverse influence was observed on the plant morphological features of CYP93E2 mutants under greenhouse conditions. In addition, differential expression of saponin pathway genes was observed in CYP93E2 mutants in comparison to the control. Our results provide new and interesting insights into the application of CRISPR/Cas9 for metabolic engineering of high-value compounds of plant origin and will be useful to investigate the physiological functions of saponins in planta.
Introduction
Saponins are a large group of triterpene or steroid glycosides common to many plant species (Haralampidisis et al., 2002) exhibiting a wide spectrum of biological and pharmacological activities (Sparg et al., 2004; Tava and Avato, 2006; Augustin et al., 2011; Moses et al., 2014a). These properties have, in the last decades, stimulated research on triterpene saponin biosynthetic pathways using Medicago truncatula (barrel medic) as model plant species (Carelli et al., 2020). In the Medicago genus, saponins are a complex mixture of olean-type glycosides with medicagenic acid, zanhic acid, hederagenin, bayogenin and soyasapogenols A and B as aglycones (Tava and Avato, 2006). All the saponins from Medicago spp. possess the triterpenic pentacyclic nucleus belonging to the class of β-amyrin (Tava et al., 2011). Subsequent modifications in specific positions of the basic β-amyrin backbone by oxidative reactions mediated by different cytochrome P450 (CYP450s) oxygenases lead to distinct sapogenins (Tava et al., 2011) (Figure 1). The sapogenins are then subjected to glycosylation reactions mediated by glycosyltransferases to give the different saponins (Augustin et al., 2011). Based on the position and the oxidation degree of their functional groups, it is possible to distinguish two different classes of sapogenins in Medicago spp.: soyasapogenols are characterized by a hydroxyl group at the C-24 position and are defined non-hemolytic sapogenins while hemolytic sapogenins have a hydroxyl or carboxyl group at C-23 and a carboxyl group at C-28 (Tava et al., 2011) (Figure 1). The hemolytic and non-hemolytic sapogenins are synthesized through independent biosynthetic pathways, and have different chemical structures, biological activity and distribution in plant organs (Tava et al., 2011; Carelli et al., 2020). In particular, hemolytic sapogenins such as bayogenin, hederagenin, medicagenic acid, zanhic acid and their glycosides, which represent the most abundant saponins in the Medicago genus, showed significant biological and pharmacological properties, including fungistatic, insecticidal, antimicrobial, antifeeding, apoptotic, nematocidal, anthelmintic, cytotoxic, hypocholesterolemic, antiatherosclerotic, neuroprotective and anticarcinogenic activities (Avato et al., 2006, 2017; Tava and Avato, 2006; Argentieri et al., 2008; Balestrazzi et al., 2011; Rafińska et al., 2017; Maestrini et al., 2019, 2020; D'Addabbo et al., 2020). On the other hand, the soyasapogenol saponins are lacking of hemolytic characteristics and for this reason they have a low biological and pharmacological activity (Tava and Avato, 2006; Argentieri et al., 2008; Rafińska et al., 2017; Carelli et al., 2020). Due to their properties, hemolytic saponins from Medicago spp. are valuable specialized metabolites to be employed in the agro-industry and for pharmaceutical applications (Ellen et al., 2007; Bora and Sharma, 2011; Rafińska et al., 2017).
In M. truncatula, almost all of the P450s that are involved in the hemolytic sapogenin biosynthesis have been identified and their functional role has been characterized (Carelli et al., 2011; Fukushima et al., 2011, 2013; Biazzi et al., 2015; Ribeiro et al., 2020). However, the in planta function of a limited number of these P450s was determined. To date, two CYP450s key genes have been identified in the non-hemolytic sapogenin pathway in M. truncatula, and their role has been characterized only in vitro using a yeast heterologous expression system: CYP93E2 catalyzes the C-24 hydroxylation of β-amyrin (Fukushima et al., 2011), after which CYP72A61 catalyzes the hydroxylation of 24-hydroxy-β-amyrin at C-22-β position (Fukushima et al., 2013) resulting in the biosynthesis of soyasapogenol B, the most abundant soyasapogenol in the genus Medicago.
Mutants are pivotal for understanding gene function and to elucidate metabolic pathways and can be a valuable resource for crop improvement as well (Liu et al., 2018). Mutant legume plants with modified sapogenin composition have been described. By combining an activation tagging method and a reverse genetic TILLING approach, Carelli et al. (2011) identified M. truncatula plant lines mutated in CYP716A12, a key gene in hemolytic sapogenin biosynthesis. These mutants were unable to produce hemolytic sapogenins, synthesized only soyasaponins and showed severe phenotypic effects such as reduced plant growth and seed germination delay. In addition, another M. truncatula mutant line carrying a loss-of-function allele in CYP72A67 was discovered in the same TILLING collection. CYP72A67 mutant plants showed altered hemolytic sapogenin production without significant effects on plant growth and reproduction (Biazzi et al., 2015). Krishnamurthy et al. (2019), using a high-density soybean mutant library, isolated GmBas1, CYP93E1, and CYP72A61 mutants. In particular, they showed that CYP93E1 mutants were deficient in total saponins and accumulated a novel saponin having sophoradiol as aglycone. Suzuki et al. (2019) assessed in Lotus japonicus the in planta roles of two P450s (LjCYP93E1 and CYP716A51) in triterpenoid biosynthesis by performing gene loss-of-function studies, and showed that LjCYP93E1 homozygous mutant plants did not accumulate soyasapogenols. However, at present no mutant plant lines lacking non-hemolytic sapogenins have been reported and characterized in Medicago spp. Moreover, their specific physiological role in plant growth and development is still unexplored.
Innovative genetic engineering methods have been recently developed to modify specific sites in targeted genes and generate knock-out mutants (Sedeek et al., 2019; Confalonieri and Sparvoli, 2020). Of these, the CRISPR (Clustered Regularly Interspaced Short Palindromic Repeats)/Cas9 system due to its high efficiency, simplicity and specificity has become a powerful and effective tool for targeted genome editing and research into gene function in plants (Doudna and Charpentier, 2014; Manghwar et al., 2019). This technology makes it possible to induce point mutations in one or some target sequences simultaneously, as well as to introduce new genetic variants by homology directed recombination (HDR) or to target and modify transcription. At present, few studies have been published related to the CRISPR/Cas9-mediated genome editing on Medicago spp. (Michno et al., 2015; Curtin et al., 2017, 2018; Meng et al., 2017; Gao et al., 2018; Wang et al., 2018; Yu et al., 2018; Wolabu et al., 2020), but none of them involved specific genes associated with the saponin metabolism.
In this study, we used the CRISPR/Cas9 technology in the model legume Medicago truncatula to knock-out CYP93E2 and CYP72A61, the two key genes of the non-hemolytic sapogenin pathway, in order to elucidate their specific roles in saponin biosynthesis in planta and explore their possible involvement in plant growth and development. A number of new allelic CYP93E2 variants were obtained while no CYP72A61 mutants were produced. Selected CYP93E2 mutant lines were analyzed for sapogenin content and plant growth performances. The present work illustrates the feasibility of using CRISPR/Cas9-targeted mutagenesis to knock-out CYP93E2 in M. truncatula. We demonstrated for the first time the success of this strategy to block the metabolic flux to non-hemolytic saponins, highlighting the potential for converting β-amyrin to hemolytic saponins.
Materials and Methods
Plant Material and Growth Conditions
The Medicago truncatula cultivar Jemalong (M9-10a genotype) was used for tissue culture and transformation. This genotype was selected by Neves (2000) for its high regeneration potential via somatic embryogenesis. M9-10a plantlets were grown and micropropagated in vitro as described by Faè et al. (2014). In vitro cultures were kept in a growth chamber at 22–24°C with 16 h photoperiod of 65–75 μmol m−2 s−1 under cool white fluorescent lamp.
SgRNA Design and CRISPR/Cas9 Vector Construction
gRNA sequences were selected using the CRISPR-P webtool (http://crispr.hzau.edu.cn/CRISPR2/), taking into account gRNA position and their on-target scores, using the Medicago truncatula Mt40v2 genome as reference. Putative off-target loci were further evaluated with Cas-OFFinder (http://www.rgenome.net/cas-offinder/) using the same reference genome, looking for up to five mismatches. Vector assembly was carried out according to the GoldenBraid cloning standard (https://gbcloning.upv.es/). The GB parts used in the assembly are listed in Supplementary Table 1. The final pCambia pDGB3 omega1 vectors, pDGB3 omega1 nptII-gRNA1-gRNA2-Cas9 (CYP93E2) and pDGB3 omega1 nptII-gRNA1-gRNA2-Cas9 (CYP72A61), were transformed in EHA105 A. tumefaciens electrocompetent cells. As a negative control pDGB3 omega1 nptII-Cas9 vector was used.
Plant Transformation and Generation of Transgenic Barrel Medic Lines
The Agrobacterium tumefaciens-mediated transformation procedure of M9-10a leaf explants was based on the protocol described by Confalonieri et al. (2009). Briefly, wounded leaflets were dipped into the EHA105 Agrobacterium suspension (OD600 = 1.6) and then co-cultivated for 5 days on solid embryo induction medium (EIM) in the dark. Subsequently, the inoculated explants were transferred on the same medium supplemented with carbenicillin (500 mg/L) and kanamycin (100 mg/L). After 3 weeks, leaf explants with embryogenic calli were transferred and subcultured on embryo proliferation medium (EPM) with the same antibiotic concentrations, until somatic embryos could be isolated. The kanamycin-resistant embryos were then grown on selective Embryo Conversion Medium (ECM) to develop into plantlets. Before acclimation and transplanting into pots to the greenhouse, putative mutant and control transgenic plants were subjected to antibiotic screening and surviving shoots were transferred to root-inducing medium for regeneration of complete plants.
Screening for Mutations Induced by CRISPR/Cas9 in CYP93E2 Gene
Genomic DNA was extracted from leaves of analyzed plants using a genomic DNA extraction kit (Sigma-Aldrich) following the manufacturer's protocols. The genomic DNA was used as template in a PCR amplification reaction using primers that were designed to amplify the CAS9 gene and 600 and 650 bp amplicons containing the specific sg1RNA and sg2RNA target sequences, respectively (Supplementary Table 2). PCR products were treated with Exonuclease I and FastAp thermo-sensitive alkaline phosphatase, purified by precipitation with ethanol:EDTA and sequenced using the Mycrosynth Barcodes Easy run service (Microsynth SeqLabDE - 37081 Göttingen). Sequences were analyzed using the TIDE web tool (https://tide.nki.nl/ Brinkman et al., 2014) in order to evaluate editing efficiency at the target sites as well as pattern of mutations.
Greenhouse Evaluation of CYP93E2 Mutant Lines
To evaluate the effects of CRISPR/Cas9 editing of CYP93E2 on plant growth features and sapogenin content, the well-rooted in vitro CYP93E2 mutant and control transgenic plants (T0 generation) were transplanted into pots (12 cm diameter) containing a mixture of peat and soil (1:2) and acclimatized for a week under growth chamber conditions (16 h of light at 24°C and 8 h of dark at 22°C), until shoot growth started. Subsequently, they were transferred in greenhouse at the beginning of the growing season at a temperature of 20–24°C, with a natural photoperiod. All the agronomic techniques were performed following the standard trial procedures. A total of 20 transgenic plants were analyzed, corresponding to 4 biological replicates of four independent CYP93E2 mutants (T81 8, T83 1, T83 8, and T83 14) and a control transgenic line. The experimental design of the greenhouse experiment was a randomized complete block design with four replications of single-plant experimental units. Leaf, stem and root samples were collected from start flowering plants. The following parameters were evaluated: number of days from transplanting to flowering, fresh and dry weight of leaf, stem and root biomass. For dry weight measure, fresh samples were frozen at −80°C and then lyophilized.
Analysis of the Sapogenin Content in the Plant Material
Leaves, stems and roots were separately harvested from each plant grown in a greenhouse and independently analyzed. Four replicated plants from all CYP93E2 mutant lines and a control line were used for biochemical determination. Immediately after sampling, fresh plant tissues were frozen at −80°C and then lyophilized. All the aglycone moieties were considered as representative of the corresponding glycosides and evaluated by chemical methods. Sapogenins were obtained after acid hydrolyses of the corresponding saponins, as reported by Tava et al. (2020), identified by GC/MS and quantitated by GC/FID as their methyl/silyl derivatives considering all the obtained artifacts (Tava et al., 2017) (Supplementary Figure 1). Hundred milligram of plant material were treated with 50 ml of 2 M HCl in 50% aqueous methanol under reflux for 8 h. Uvaol (0.2 mg) was added as internal standard, methanol was removed in vacuo and the aglycones were extracted with ethyl acetate (3 × 20 ml). The organic solution was dried under anhydrous Na2SO4 and evaporated to dryness. Aglycones were dissolved in 0.5 mL of MeOH, treated with CH2N2 for 15 min and then the solvent eliminated under a stream of N2. Silylation was performed on the methylated sapogenins using 0.2 mL of a mixture of pyridine-hexamethyldisilazane-chlorotrimethylsilane (Merck) 2:1:1 at 70°C for 10 min. Samples were diluted with isooctane and used for GC/FID and GC/MS analyses. GC/FID analyses were performed with a 30 m × 0.32 mm, 0.25 μm i.d., DB-5 capillary column. Injector and detector temperatures were set at 350°C; the oven temperature program was: 90°C for 5 min, increased at 20°C/min to 250°C for 1 min and then increased at 4°C/min to 350°C for 15 min. Samples (1 μL) were injected in the “splitless” mode. He was the carrier gas with a head pressure of 12.2 psi. GC/MS analyses were carried out using a 30 m × 0.25 mm, 0.25 μm i.d., Elite-5MS capillary column using the same chromatographic conditions as for GC/FID. Mass spectra were acquired over 50–850 amu range at 1 scan/s with ionizing electron energy 70 eV. Transfer line 300°C, carrier gas He at 1.2 mL/min. Retention times and MS spectra were compared to those of previously identified sapogenins, considering all the formed artifact compounds as reported in Tava et al. (2017). All samples were analyzed in triplicate.
Expression Analysis of Saponin Metabolic Pathway-Related Genes in CYP93E2 Mutant Lines
Expression analysis of saponin pathway-related genes was performed by quantitative real-time PCR. CYP93E2, CYP72A61, CYP716A12, CYP72A67, CYP72A68, CYP88A13, and BAS1 genes were analyzed. Total RNA was extracted from fully developed trifoliate leaves and roots of mutant and control plants harvested at the beginning of flowering, using the Nucleospin RNA plant kit (Macherey-Nagel, Düren, Germany) according to the manufacturer's protocol. For each transgenic line, four plants (biological replicates) were analyzed. cDNA was synthesized using the iScript cDNA Synthesis Kit (Bio-Rad) starting from 1 μg of RNA. cDNA was diluted 1:5 and 3 μl was used as a template in a 10 μl reaction containing 5 μl of SSoFast EvaGreen supermix (Bio-Rad) and specific primers for the selected genes (Supplementary Table 2). Thermal cycling conditions were: 3 min of initial denaturation at 95°C, 50 cycles of denaturation (95°C; 25 s), annealing and extension (59.5°C; 3 s) and a final melting analysis from 55 to 95°C with a 1°C increase at each step. The Medicago sativa translationally controlled tumor protein Msc27 (Pay et al., 1992) and Actin control genes were amplified in the same conditions. qRT-PCR reactions and analyses were carried out on three replicates each in a Rotor Gene 6000 (Qiagen) as previously described (Carelli et al., 2015). The gene expression levels were calculated by the comparative Ct method using the equation E = 2−ΔΔCt. ΔΔCt values were calculated as differences in ΔCt between edited lines (leaves or roots) and the roots of control line.
Statistical Analysis
All data were subjected to the Analysis of Variance (ANOVA). Mean comparison between control and CYP93E2 mutant lines was done by Dunnett's Test (p < 0.05) (SAS Proc GLM, SAS Institute Inc., Cary, NC).
Results
CRISPR/Cas9 Design to Knock-Out CYP93E2 and CYP72A61 Genes in Barrel Medic
For each of the target genes, two specific gRNAs were designed, directed at their coding sequences. For each gene, one gRNA was directed at the C-terminal Heme Cys domain, while the other was chosen to be as close as possible to the 5' end of the coding sequence, in order to ensure the early disruption of translation. The selected gRNAs also did not pose any significant risk of off-target activity, as inferred by the in silico prediction of putative off-target loci in the M. truncatula genome (Supplementary Data 1). Each gRNA was put under the control of the Arabidopsis U6-26 promoter. For each target gene, the two gRNAs were assembled in a pCambia vector together with the Cas9 endonuclease and the nptII selection marker for kanamycin resistance.
Production of CYP93E2 Mutant Barrel Medic Lines
Medicago truncatula was transformed using the EHA105 A. tumefaciens strain harboring the CRISPR/Cas9 binary vectors targeting the CYP93E2 or the CYP72A61 genes. After several weeks on selective embryo inducing and proliferating media no somatic embryos were isolated from leaf explants co-cultivated with EHA105 carrying the CYP72A61 targeting construct. Differently, somatic embryos were produced from embryogenic calli obtained from explants transformed with the CYP93E2 targeting construct (Supplementary Figure 2; Supplementary Table 3). Fifty-two putative CYP93E2 mutant plant lines were recovered with a transformation efficiency of 6.4%. Fourteen kanamycin-resistant plant lines transformed with the Cas9 and nptII genes without the gRNAs (control) were also regenerated, with a comparable transformation efficiency (4.4%). The genome-edited and control plant lines were found to be phenotypically similar with no visible growth abnormalities during in vitro micropropagation and acclimation to soil.
CRISPR/Cas9 Induces Highly Efficient Mutagenesis of the CYP93E2 Gene
To determine whether the target CYP93E2 gene was mutated, we genotyped 51 independent putative CYP93E2 mutants and three control T0 plant lines using specific primers for the Cas9 gene and for regions flanking the CYP93E2 target sequences (Supplementary Table 2; Figure 2A). All the 51 mutants and the three control plant lines showed the genomic integration of the transgene. Sequencing and chromatogram decomposition analysis showed that, compared with the wild type sequence, 43 out of the 51 mutant plant lines (84.3%) carried mutations in at least one of the two targeted sites of the CYP93E2 gene (Supplementary Table 4). Considering gRNA1, all the 43 edited lines showed target sequence mutations. Among these, 21 lines were completely edited showing a homozygous mutation or biallelic profiles. The other 22 lines had a partially edited genotype (heterozygous) and retained a proportion of the wild type allele (Supplementary Table 4). At the gRNA2 target locus, a lower editing efficiency was observed as 25 of the 43 edited plant lines retained the wild type sequence. In 11 lines a partially edited genotype (heterozygous) was found and only seven lines were completely edited showing a homozygous mutation or biallelic profiles (Supplementary Table 4). The most frequent types of mutation at the gRNA1 target site were a −4 bp deletion and +T insertion (Figure 2B), while at the gRNA2 target site 32% of the observed mutations were long bp deletions. After identifying the targeted mutations, we randomly selected four (T81 8, T83 1, T83 8, and T83 14) T0 mutant lines based on the different type mutations at the target sites of the CYP93E2 gene. Focusing on gRNA1, two were biallelic mutants and two homozygous. Focusing on gRNA2, two were biallelic mutants, one heterozygous and one wild type. The edited sequences of the selected plant lines are shown in Figure 2C. The deduced amino acid sequences of the CYP93E2 protein for the selected lines show frame-shifting with the introduction of amino acid mutations in the catalytic site and stop codons responsible for the premature termination of protein translation (T81 8, T83 8) or the loss of the ATG starting codon (T83 1, T83 14).
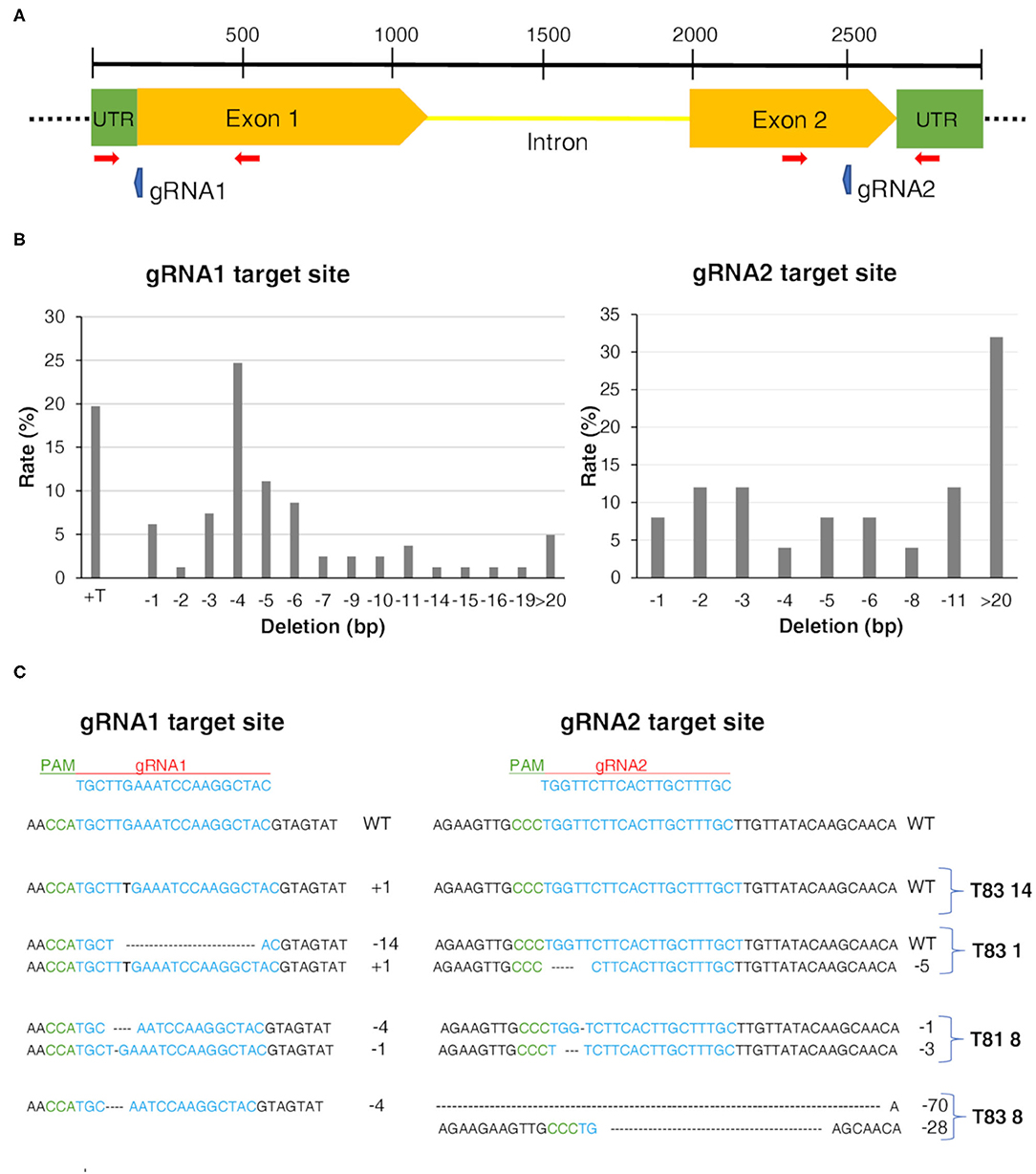
Figure 2. CRISPR/Cas9-editing in Medicago truncatula. (A) Gene structure of CYP93E2 with target sites of CRISPR/Cas9 gRNAs (blue arrows) designed in the first and second exon. The red arrows represent primers used to screen mutations. (B) Rate of mutation sizes. Percentages were calculated by dividing the number of events of each mutation size by the sum of total mutation events. (C) Representation of mutation events generated by the CRISPR/Cas9 system in CYP93E2 gene of the four selected plant lines. Mutations were detected using as reference the control plant lines. The CYP93E2 target sequences are colored in blue. Within the sequence alignment, deletions are represented by traits; insertions are shown with black bold letters. Mutation size and plant line ID are shown on the right. Guide RNA (gRNA) and protospacer adjacent motif (PAM) are indicated in red and green, respectively.
Phenotypic and Morphological Observations of CYP93E2 Mutant Barrel Medic Lines
CYP93E2 mutant and control plant lines showed similar morphological features, flowered and produced seeds under controlled environmental conditions. All tested plants showed root nodulation with no visible differences between the control and the CYP93E2 mutants. To investigate whether mutations in the CYP93E2 gene affect the plant growth and yield, we characterized the four selected T0 mutant lines by measuring the number of days from transplanting to flowering and leaf, stem and root biomass at the start of the flowering period. All the tested mutants showed a similar flowering time than the control (Figure 3A). Three mutant lines (T81 8, T83 1, and T83 8) showed plant growth performances similar to the control while the fourth mutant (T83 14) exhibited a significant increase in plant biomass production, according to Dunnett's test (Figures 3B–D; Supplementary Figure 3). The results obtained for the dry weight of shoot and root biomass confirmed the data of the fresh weight measurements (Supplementary Figure 4).
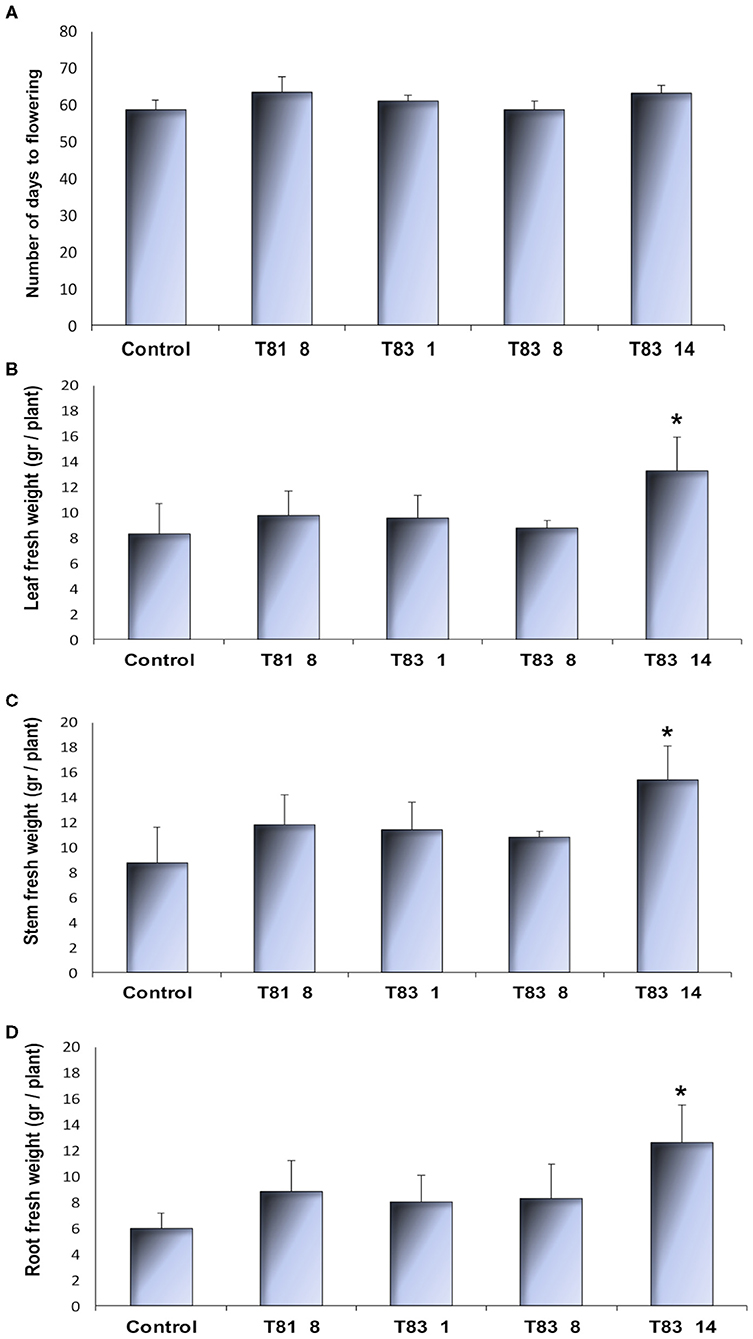
Figure 3. Morphological observations of control and selected CYP93E2 mutant barrel medic plant lines under greenhouse conditions. (A) Number of days from transplanting to flowering. (B–D) Leaf, stem and root fresh weight per plant at the start of plant flowering, respectively. The greenhouse experiment was performed with four biological replications of single-plant experimental units. Data are shown as the mean values with error bars representing standard deviation. An asterisk indicates means significantly different from control according to Dunnett's Test (p < 0.05).
Knock-Out of the CYP93E2 Gene by CRISPR/Cas9 Affect Both Non-hemolytic and Hemolytic Sapogenin Contents
To investigate the effects of knock-out mutations in the CYP93E2 gene on the triterpene saponin content of leaves, stems and roots, all selected mutant lines were biochemically analyzed. All sapogenins were identified by using authentic reference standards and quantitated considering all the obtained sapogenins (Tava et al., 2017) (Supplementary Figure 1). The results of this investigation are shown in Tables 1, 2 where the amount (mg/g DM) of each identified sapogenin is reported for aerial parts (leaves and stems) and roots, respectively. The hemolytic sapogenin composition of all CYP93E2 mutant lines is similar to the control, while no soyasapogenols have been detected in any of the analyzed organs. The ANOVA revealed significant differences (p < 0.0001) among the barrel medic lines for the soyasapogenol B and A contents in the leaves, stems and roots (Tables 1, 2). According to Dunnett's test, all mutant lines showed significant differences in the content of both soyasapogenols compared to the control. Considering the total content of hemolytic sapogenins in leaves, the ANOVA revealed significant differences (p = 0.005) among the barrel medic lines. Two (T81 8 and T83 8) mutant lines showed a significantly higher total hemolytic sapogenin content, according to Dunnett's Test (Table 1; Figure 4). All mutant lines displayed significant higher amounts of medicagenic acid when compared to the control. Furthermore, three (T81 8, T83 1, and T83 8) mutant lines showed significantly higher bayogenin level, and two (T831 and T83 8) of them also displayed a significant increase of zanhic acid content than the control (Table 1). The amount of medicagenic acid and zanhic acid, the major hemolytic sapogenins in M. truncatula aerial parts, was more than two times higher in T81 8 and T83 8 mutants compared to the control. The total leaf sapogenin content varied in the range 2.47–3.51 mg/g DM in CYP93E2 mutants and was not significantly different from the control line (3.13 mg/g DM). According to Dunnett's Test, all mutant lines displayed significant higher total amounts of hemolytic sapogenins in the stems when compared to the control (Table 1; Figure 4). As seen in the leaves, all tested lines showed significantly higher medicagenic acid level, and the same two lines (T81 8 and T83 8) displayed a significant increase of zanhic acid content than the control. With regard to total sapogenin content in the stems, T83 8 displayed a similar level to the control, while the other three mutant lines showed a significant reduction (Table 1). Differently from the aerial part, in the roots the hemolytic sapogenins oleanolic acid and 2β-hydroxy oleanolic acid were also detected in both the control and edited lines (Table 2). The content of all hemolytic sapogenins in roots, including oleanolic acid, 2β-hydroxy oleanolic acid, hederagenin, bayogenin and medicagenic acid, was higher in all mutants than in the control. Specifically, two (T81 8 and T83 8) mutant lines showed significantly higher hederagenin content, and one (T83 8) of them also displayed a significant increase of medicagenic acid content than the control, according to Dunnett's Test. No significant differences were observed between CYP93E2 mutants and the control for the total sapogenin content in roots. Interestingly, the biochemical characterization revealed the presence of β-amyrin (0.08 mg/g DM) in the roots of all mutant lines but not in the control, and these differences were significant, according to Dunnett's test (Table 2).
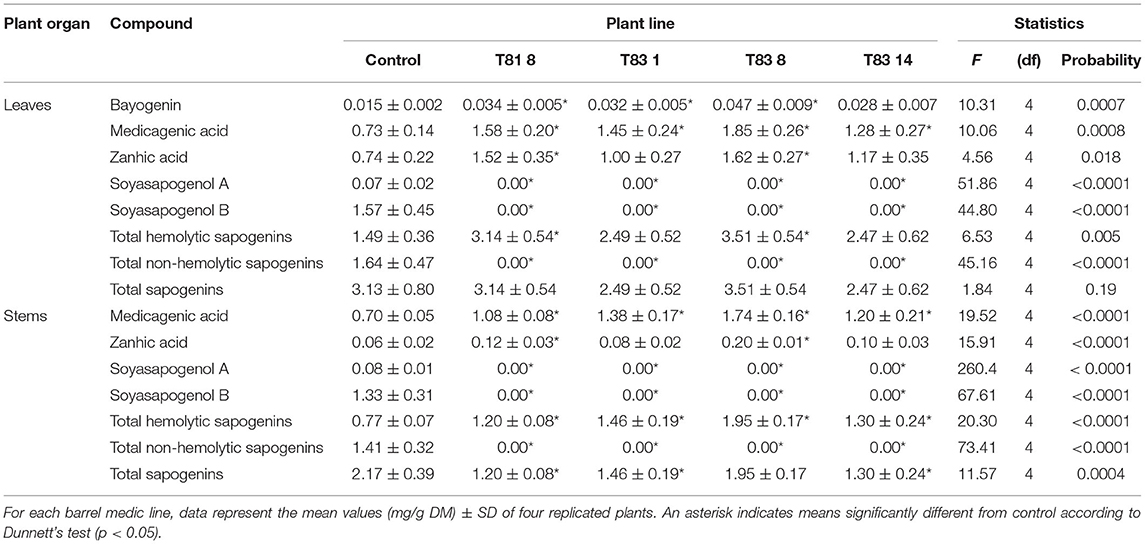
Table 1. Sapogenin content in aerial parts (leaves and stems) of control and CYP93E2 mutant barrel medic plant lines under greenhouse experimental conditions.
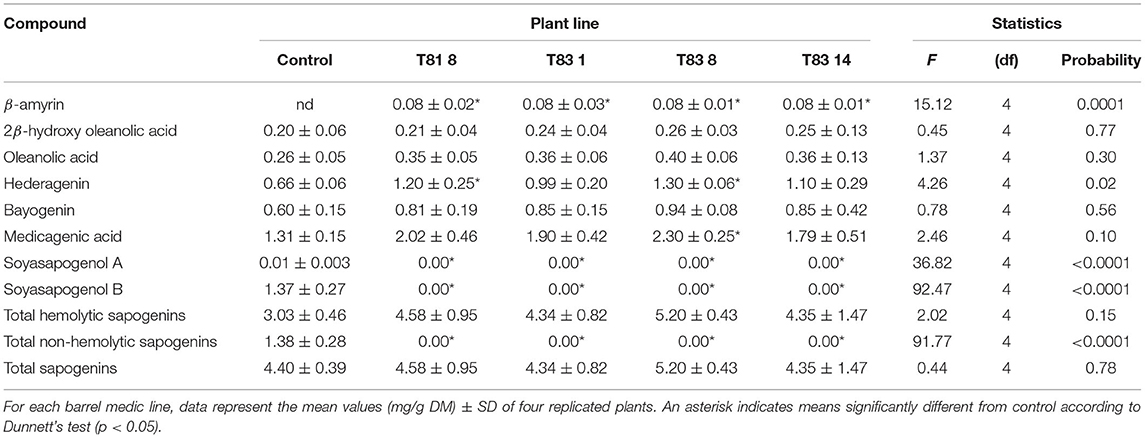
Table 2. Sapogenin content in roots of control and CYP93E2 mutant barrel medic plant lines under greenhouse experimental conditions.
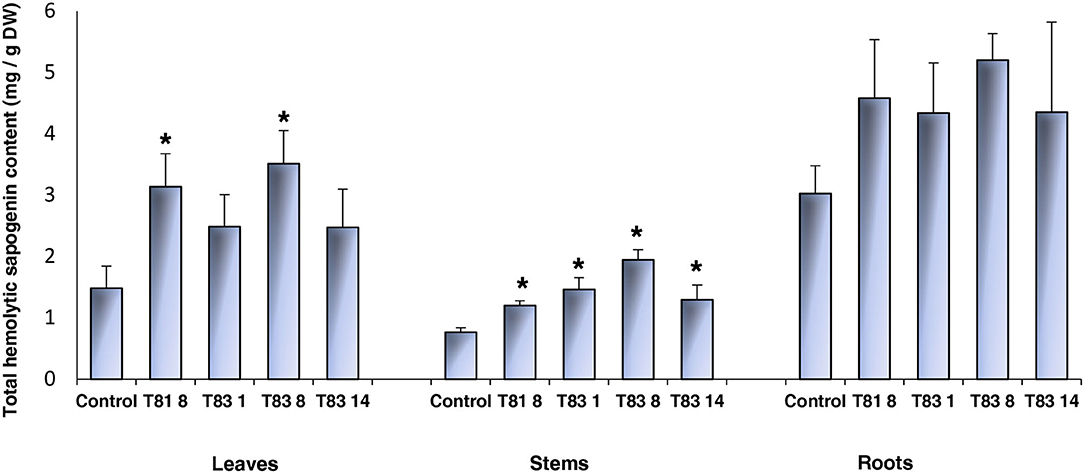
Figure 4. Total hemolytic sapogenin content in leaves, stem and roots of control and CYP93E2 mutant barrel medic plants under greenhouse experimental conditions. Data are shown as the mean values of four biological replications of single-plant experimental units with error bars representing standard deviation. An asterisk indicates means significantly different from control according to Dunnett's Test (p < 0.05).
Editing of CYP93E2 Affected Expression of Genes Involved in the Saponin Pathway
Quantitative real-time PCR analysis was performed on leaves and roots of the control and all the selected mutants for the β-amyrin synthase BAS1 gene (Suzuki et al., 2002), for the genes of the hemolytic sapogenin branch - CYP716A12 (Carelli et al., 2011), CYP72A68 (Fukushima et al., 2013), CYP72A67 (Biazzi et al., 2015), CYP88A13 (Ribeiro et al., 2020) and for the genes of the non-hemolytic sapogenin branch - CYP93E2 (Fukushima et al., 2011) and CYP72A61 (Fukushima et al., 2013). The results are expressed as relative expression values using the roots of the control line as reference. As regards the genes of the hemolytic pathway, Dunnett's Test revealed a significantly enhanced accumulation of CYP72A67 and CYP72A68 transcripts in the leaves of T83 1 mutant (Figure 5). Although ANOVA revealed no significant differences (p = 0.08) in CYP716A12 expression in the leaves of barrel medic lines, higher expression levels were detected for T83 1 and T83 14 mutants compared to the control. Interestingly, CYP88A13, the gene responsible for the synthesis of zanhic acid, was highly induced in leaves of three (T81 8, T83 1, and T83 14) mutant lines. Zanhic acid represent the last step of hemolytic saponin branch (Figure 1) and together with medicagenic acid were the most abundant sapogenins in leaves of CYP93E2 mutant lines (Table 1). The up-regulation of genes involved in the hemolytic sapogenin biosynthesis in some of the edited barrel medic lines was in accordance with the increased levels of hemolytic sapogenins found. As regards the genes of the non-hemolytic branch, two (T831 and T83 14) mutant lines showed a higher amount of CYP93E2 transcript in leaves, and one (T83 1) of them also displayed a significant CYP72A61 up-regulation when compared to the control (Figure 5). Concerning the BAS1 expression level in leaves, the ANOVA revealed significant differences (p = 0.04) among the tested lines and T83 1 showed a significant BAS1 up-regulation when compared to the control. No significant differences in the expression profile of both hemolytic and non-hemolytic genes in roots were observed among barrel medic lines (Figure 5).
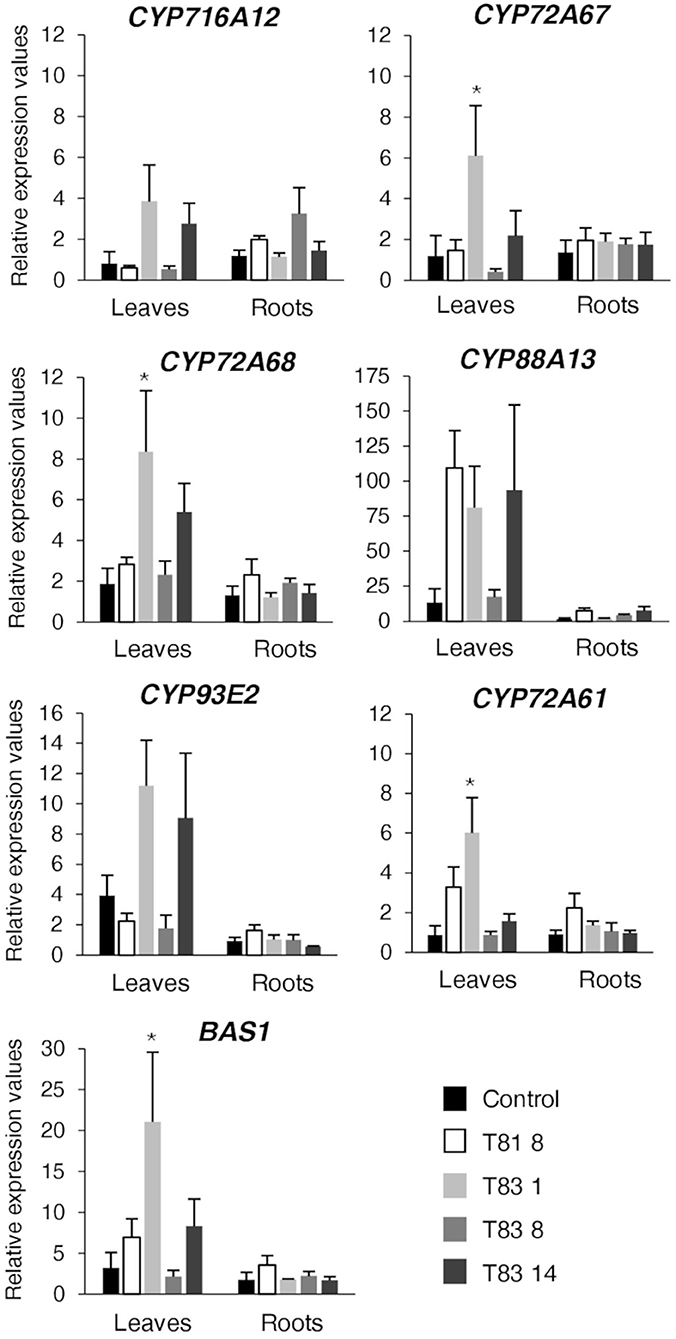
Figure 5. Expression analysis using quantitative RT-PCR on sapogenin pathway genes. Values were calculated by the comparative Ct (cycle threshold) method using roots of control line as reference. Values are means ± SE of four biological replicates. An asterisk indicates means significantly different from control according to Dunnett's Test (p < 0.05).
Discussion
Metabolic engineering of triterpene saponin biosynthesis in plants is a fascinating research topic from two main perspectives. The wide potential use of saponins as biologically and pharmacologically active compounds, and their possible effects on fundamental plant developmental processes, make their modulation an interesting tool for breeding and biotechnology. Also, the development of plants with modified saponin profiles could yield further understanding of their biosynthetic route and regulation mechanisms. The metabolic engineering of both the early and late stages of the saponin biosynthetic pathway has been the topic of several reviews (Yendo et al., 2010; Lambert et al., 2011; da Silva Magedans et al., 2020). The major requirement for the successful engineering of specific saponins is the knowledge of the genes/enzymes involved in their biosynthesis. Over recent years, the economic relevance of these valuable natural compounds led to a substantial understanding of the biosynthetic routes of triterpene saponins, in particular in M. truncatula, a rich source of different saponins and a model plant species for legumes (Pateraki et al., 2015; Carelli et al., 2020). Several members of P450 families have been characterized to be involved in saponin biosynthesis of M. truncatula (Carelli et al., 2011; Fukushima et al., 2011, 2013; Biazzi et al., 2015; Ribeiro et al., 2020) but the in planta function of a limited number of these P450s was determined. Furthermore, results from studies with heterologous microbial systems do not always correspond with in planta function. By using a yeast expression system, Biazzi et al. (2015) supported the hypothesis that CYP72A68 is mainly involved in the biosynthesis of medicagenic acid, while Tzin et al. (2019) showed that CYP72A68 is a multi-functional oxidase responsible for hederagenin, gypsogenin and gypsogenic acid based on in vivo and in planta evidence. To date, the functional roles in planta of CYP93E2 and CYP72A61, which participate in the biosynthesis of non-hemolytic sapogenins in M. truncatula, have not been demonstrated yet. The biochemical functions of these P450 key enzymes and the physiological roles of saponins in barrel medic could be elucidated by characterization of knock-out mutants or gene over-expression. Few studies reported the CRISPR/Cas9-mediated genome editing in Medicago spp. (Michno et al., 2015; Curtin et al., 2017, 2018; Meng et al., 2017; Gao et al., 2018; Wang et al., 2018; Yu et al., 2018; Wolabu et al., 2020) and none of them involved specific genes associated with the metabolism of saponins.
In this work, we used the CRISPR/Cas9 technology in the model legume Medicago truncatula to knock-out two key CYP450 genes of the non-hemolytic saponin pathway (CYP93E2 and CYP72A61), in order to demonstrate their specific role in planta and explore their possible involvement in growth and development. We adopted a dual gRNA approach for targeting CYP93E2 and CYP72A61 in order to enhance the genome editing efficiency by either increasing the probability of successful mutagenesis, or even deleting large gene fragments (Nian et al., 2017; Pauwels et al., 2018; Do et al., 2019), and we made use of assembly of the GoldenBraid GB cloning system suited for gene editing experiments (Vazquez-Vilar et al., 2016; Aliaga-Franco et al., 2019; Maioli et al., 2020). In this study, we demonstrate for the first time that CYP93E2 can be efficiently edited in M. truncatula, resulting in the manipulation of the saponin metabolic pathway. Heterozygous, biallelic and homozygous mutants were detected in T0 plants, suggesting that editing occurred at an early stage of the transformation/regeneration processes (Li et al., 2018). The overall gene editing efficiency was 84%, higher than what observed in other published works in M. truncatula (Meng et al., 2017; Curtin et al., 2018; Wolabu et al., 2020). All the 43 edited lines showed mutations at the gRNA1 locus. The gRNA2 locus showed a lower mutation rate, with 25 of the 43 (58%) edited plant lines not showing any editing effect (Supplementary Table 4). Although the editing efficiency was different between the two gRNAs, it was not possible to attribute this discrepancy to the different GC contents of the target sequences or sgRNA secondary structure or promoters that direct Cas9 and gRNAs expression (Ma et al., 2015). Interestingly, the most frequent types of mutation at the CYP93E2 gRNA1 target site were a −4 bp deletion and the insertion of a single nucleotide, while at the gRNA2 target site 32% of the observed mutations were long bp deletions (Figure 2B). In M. truncatula deletion of 1–10 nucleotides were the most common mutations (Michno et al., 2015; Meng et al., 2017; Curtin et al., 2018). The mutation pattern is consistent with that predicted with CRISPOR (http://crispor.tefor.net/) based on microhomology. Off-target mutations have not proven to be a cause for concern in plants (Hahn and Nekrasov, 2019) contrary to what was observed in human cells (Fu et al., 2013). The presence of mismatches in the seed region between the selected sgRNA and the off-targets supports the mutation efficiency (Hahn and Nekrasov, 2019), since this 3' terminal region of the target sequence strongly affects recognition by Cas9. The prospective off-target activity with the gRNAs used in this work was predicted using CasOFFinder, and no loci were found in the M. truncatula genome which could be considered as a likely source of off-target gene editing (Supplementary Data 1). In the only 2 prospective off-target loci with fewer than 3 mismatches with respect to the gRNA sequence, these were located in the seed region, and would therefore highly impair recognition.
Among all CYP93E2 T0 mutant plant lines, four representative lines were chosen for further phenotype observation and biochemical analyses. The results of greenhouse trial showed no significant differences between most of the tested mutant lines (T81 8, T83 1 and T83 8) and the control in shoot and root biomass (Figure 3). These plant lines exhibited normal flowering time and produced seeds. These results were similar to a previous report which showed that homozygous mutant lines of Lotus japonicus harboring Lotus retrotrasposon1 (LORE1) in LjCYP93E1, an orthologous of the M. truncatula CYP93E2, did not accumulate soyasapogenols without significant effects on plant growth and reproduction (Suzuki et al., 2019). The same authors suggest that the soyasaponins are not necessary for plant growth in Lotus japonicus. Biazzi et al. (2015) reported that TILLING barrel medic mutants of CYP72A67, a key enzyme in the biosynthesis of hemolytic sapogenins in M. truncatula, accumulated gypsogenin, gypsogenic acid, and 16α-hydroxy gypsogenic acid instead of medicagenic and zanhic acids in the leaves, without affecting plant growth and reproduction. Krishnamurthy et al. (2019) reported the modification of saponin composition in soybean mutants isolated from a high-density soybean mutant library targeted to β-amyrin synthase, and showed that mutant plants grow normally. Furthermore, Confalonieri et al. (2009) showed that the ectopic expression of a β-amyrin synthase-encoding sequence (AsOXA1) in transgenic M. truncatula led to higher accumulation of triterpene sapogenins and enhanced root nodulation without significant effects on plant growth and biomass. Unexpectedly, a fourth CYP93E2 mutant line (T83 14) showed a significant enhancement of biomass production, and exhibited flowering time and seed recovery similar to the control and the other CYP93E2 mutants (Figure 3). These specific results differ from previous reports which revealed detrimental effects on plant growth of M. truncatula associated to a mutation of genes encoding P450s or UDP- glycosyltransferases involved in hemolytic saponin biosynthesis (Naoumkina et al., 2010; Carelli et al., 2011). The differences in plant growth performances observed for T83 14 mutant line could result from positional effects or somaclonal variation which are not unusual in the production of transgenic plants and therefore cannot be excluded. In conclusion, no adverse influence was observed on the plant morphological features of CYP93E2 mutants under greenhouse conditions.
To examine in detail the metabolic changes between the control and CYP93E2 mutant barrel medic lines, we compared the triterpenoid sapogenin composition of the leaves, stems and roots. Our objective was to generate CYP93E2 M. truncatula knock-out mutants in which the metabolic flux to non-hemolytic saponins is totally blocked, with a potential impact on valuable hemolytic saponin levels. Gene editing tools allowed us to obtain mutants with a homogeneous genetic background, without stacking other mutations. As expected, all tested CYP93E2 mutants did not accumulate soyasapogenols in any aerial and subterranean organs. These results provide the first in planta demonstration of the key role of CYP93E2 in the control of triterpenoid saponin accumulation, confirming the results of yeast heterologous expression studies by Fukushima et al. (2011) and Moses et al. (2014b), and are consistent with previous findings reported for other legume species (Suzuki et al., 2019). So, in Medicago truncatula CYP93E2 is directly involved in soyasaponin biosynthesis by catalyzing the C-24 hydroxylation of the β-amyrin backbone and its loss of function is sufficient to completely suppress non-hemolytic sapogenin biosynthesis. Sophoradiol, a β-amyrin derivative oxidized at the C-22 position by the CYP72A61, was not detected in aerial and subterranean organs of all CYP93E2 mutant and control lines. Differently, Suzuki et al. (2019) showed accumulation of sophoradiol in LyCYP93E1 Lotus japonicus mutants, and Krishnamurthy et al. (2019) detected this compound in CYP93E1 soybean mutants. In particular, Suzuki et al. (2019) proposed for L. japonicus a triterpenoid biosynthetic pathway with two possible paths starting from β-amyrin toward soyasapogenol B, one of which is catalyzed by LjCYP93E1. Our results provide the evidence that CYP93E2 is the first and only enzyme responsible for the first key oxidative step of non-hemolytic saponin pathway in M. truncatula. The differences between results from L. japonicus and soybean and the ones observed in our study might be related to the physiological differences between the legume species and to the different plant organs (leaves, stems and roots vs. hypocotyls and cotyledons).
Knocking-out CYP93E2 influenced the biosynthetic pathway of hemolytic saponins and resulted in the redirection of metabolic fluxes in all the tested mutant lines. In fact, the leaves of all CYP93E2 mutant lines accumulated higher amounts of total hemolytic sapogenins than those of the control plants (Figure 4). In T81 8 and T83 8 mutants, the plant lines with the significantly higher total hemolytic sapogenin content, the amount of medicagenic and zanhic acid, known as the major hemolytic sapogenins in M. truncatula aerial parts (Kapusta et al., 2005; Tava and Avato, 2006; Lei et al., 2019), was more than doubled with respect to the control. Furthermore, the total leaf sapogenin content of all CYP93E2 mutants was not significantly different from the control line. In stems, despite the significant increase in hemolytic sapogenins in all the tested mutants, most of them did not totally compensate for the absence of soyasapogenols. In roots, the content of hemolytic sapogenins was less variable with only two mutants (T81 8 and T83 8) showing significantly higher hederagenin content than the control, and T83 8 having a significantly higher medicagenic acid content (Table 2). Overall, these results indicate that the absence of soyasapogenols induced a compensation by the increased accumulation of hemolytic sapogenins (Figure 4). Suzuki et al. (2019) showed an increase of oleanolic acid only in the roots of LyCYP93E1 mutant plants, while our quantitative biochemical data clearly indicate that the absence of soyasapogenols in the MtCYP93E2 mutants induced an increase in the accumulation of hemolytic sapogenins in all tested organs. The plant response to the absence of hemolytic sapogenins was not the same; in fact, Carelli et al. (2011) demonstrated that knock-out mutants of CYP716A12, a key enzyme catalyzing the first step of hemolytic sapogenin pathway, blocked the synthesis of all hemolytic sapogenins without significant and consistent effects on soyasapogenol levels. Interestingly, the biochemical characterization revealed the presence of β-amyrin in the roots of all mutant plant lines, but not in the control. The significant accumulation of β-amyrin, a common precursor of both hemolytic and non-hemolytic sapogenins, could be the direct consequence of the metabolic block toward soyasapogenol production imposed on CYP93E2 mutants, and this result is consistent with previous findings (Suzuki et al., 2019). Differently, β-amyrin was not detected in the leaves and stems of CYP93E2 mutants, suggesting that this precursor is entirely converted to hemolytic sapogenins.
To verify whether CYP93E2 mutations had caused changes in gene expression, qRT-PCR was used to detect the expression levels of genes related to hemolytic and non-hemolytic sapogenin pathway. All the tested mutant lines partially or totally compensate the absence of soyasapogenols by increasing the content of hemolytic sapogenins in order to maintain a total sapogenin “threshold” content similar to the control (Tables 1, 2; Figure 4). Overall, the increased content of hemolytic sapogenins was not directly correlated with the expression of the corresponding genes, except for the T 83 1 and T83 14 mutants. The reason of this discrepancy could be that at the sampling time of analysis (start of flowering) the edited lines had already reached the total sapogenin “threshold,” so the corresponding genes did not appear induced with respect to the control line. The hypothesis of a “threshold” value for sapogenin content is reinforced considering the role of CYP88A13, the gene responsible for the conversion of medicagenic to zanhic acid, highly induced in leaves of the CYP93E2 mutant lines. The conversion of medicagenic to zanhic acid could act as a leaf-specific way to maintain medicagenic acid within a genetically determined range. In fact, zanhic acid saponins showed lower hemolytic activities compared with medicagenic acid saponins (Oleszek et al., 1992), resulting in a reduced ability to cause membrane perturbation. The mutant lines showed higher levels of hemolytic sapogenins and in particular of medicagenic acid compared to the control line so the induction of CYP88A13 can be part of this balance mechanism. Evidence of this mechanism was found in leaves of Medicago inter-specific hybrid derivatives (Carelli et al., 2015), where no correlation between zanhic acid and the other hemolytic sapogenin content was found, but a strict control of medicagenic/zanhic ratio was evidenced. Differently from leaves and stems, roots were characterized by a larger number of aglycones; among these, the precursors of medicagenic acid (Figure 1; Tables 1, 2) in the control line represented 57% of the total hemolytic sapogenins with respect to 1% in leaves. The edited lines compensated the absence of root soyasapogenols by increasing, though not significantly, all the classes of hemolytic sapogenins by the intervention of CYP716A12 (oleanolic acid), CYP716A12 and CYP72A67 (2β-hydroxy oleanolic acid), CYP716A12 and CYP72A68-mediated hydroxylation (hederagenin), CYP716A12, CYP72A67 and CYP72A68-mediated carboxylation (medicagenic acid) (Figure 1). Then, the absence of a prevailing hemolytic sapogenin biosynthetic target in root, as it is the case for medicagenic acid in leaves, lead to a more diversified expression pattern of the sapogenin biosynthetic genes in the edited lines that could account for the lack of significant differences from the control.
With regard to CRISPR/Cas9 mediated gene editing of CYP72A61, it was not possible to recover somatic embryos and regenerate transformed plantlets carrying mutations in the target sequences. In fact, when leaf explants with calli were transferred to a selective hormone-free medium, somatic embryos started to develop, but only in the control, pointing to an effect dependent on the role of the targeted gene. P450s are involved in the synthesis of secondary metabolites and are known for their roles in many important cell processes. In particular, they are involved in the regulation of plant hormone metabolism and they function directly in plant growth and development (Xu et al., 2015). The manipulation of the biosynthesis of saponins and their intermediates which accumulate during normal growth and development can determine morphological and physiological effects at various developmental stages, including seed germination, vegetative growth, flowering and embryo development, fruiting and nodulation, suggesting additional roles for these molecules in primary plant processes (Moses et al., 2014a). As far as we know, the only manuscript describing the effects of alteration of endogenous levels of triterpenes on embryogenesis was published by Go et al. (2012). In particular, knock-out Arabidopsis mutants for marneral synthase 1 (MRN1), which converts 2,3-oxidosqualene to the triterpene marneral, displayed altered levels of triterpenoids and sterols, delayed zygotic embryogenesis, reduced growth and late flowering (Go et al., 2012). It is possible that the mutation in CYP72A61 is responsible for the absence of somatic embryo differentiation under selective in vitro conditions, presumably resulting from the altered flux balances among hormone and/or saponin biosynthesis, suggesting that CYP72A61 might be involved in inhibition of embryo and shoot development and therefore important for plant growth and survival. Also, mutations of genes encoding P450s involved in saponin biosynthesis have revealed negative effects on plant growth processes that may be related to the accumulation of toxic intermediated (Moses et al., 2014a). CYP72A61 in M. truncatula catalyzes the hydroxylation of C-22 in 24-hydroxy-β-amyrin (the product of CYP93E2) to yield soyasapogenol B (Fukushima et al., 2013). A loss of function mutation in CYP72A61 could presumably determine accumulation of 24-hydroxy-β-amyrin. This metabolic intermediate of soyasapogenols does not normally accumulate in the plant, and could result in toxic effects on somatic embryogenesis. To address both hypotheses, comprehensive molecular and metabolomic studies will be required to confirm the CYP72A61 mutations by CRISPR/Cas9 system and their effects at the cellular level, and the presence of 24-hydroxy-β-amyrin in undifferentiated calli.
In conclusion, we have assessed the in planta role of CYP93E2 in triterpene saponin biosynthesis in M. truncatula. The present study reports generation of CYP93E2 barrel medic mutants with high efficiency by CRISPR/Cas9-mediated mutagenesis, and demonstrates for the first time the success of this strategy to block the metabolic flux toward non-hemolytic saponins, highlighting the potential for converting β-amyrin to valuable hemolytic saponins. These mutant plants with high content of hemolytic saponins are consistent with the potential industrial extraction of saponins for a wide range of applications. Our findings provide new and interesting insights into the application of CRISPR/Cas9 for metabolic engineering of high-value compounds of plant origin, offer the opportunity to develop new biological sources and additional useful informations to further investigate the physiological functions of saponins in planta.
Data Availability Statement
The original contributions presented in the study are included in the article/Supplementary Material, further inquiries can be directed to the corresponding author/s.
Author Contributions
MCo: conceived and planned the study, designed and performed genetic transformation, tissue culture and growth chamber/greenhouse experiments, analyzed the data, and wrote the manuscript. SG and AM: designed and cloned gene editing constructs and contributed to the revision of the manuscript. MCa: conducted PCR and QRT-PCR experiments, analyzed the data, and contributed to the revision of the manuscript. EB and AT: performed biochemical analyses. All authors contributed to the article and approved the submitted version.
Funding
Research was financially supported by ROP ERDF 2014-2020 Lombardy – Innovation e Competitiveness.
Conflict of Interest
The authors declare that the research was conducted in the absence of any commercial or financial relationships that could be construed as a potential conflict of interest.
Publisher's Note
All claims expressed in this article are solely those of the authors and do not necessarily represent those of their affiliated organizations, or those of the publisher, the editors and the reviewers. Any product that may be evaluated in this article, or claim that may be made by its manufacturer, is not guaranteed or endorsed by the publisher.
Acknowledgments
We thank Dr. Carla Scotti from CREA-ZA, Lodi for her valuable revision of the manuscript. We are also grateful to Barbara Pintus for technical assistance and Prof. Manuel Pedro Salema Fevereiro from ITQB, Oeiras (Portugal) for providing the M9-10a Medicago truncatula genotype.
Supplementary Material
The Supplementary Material for this article can be found online at: https://www.frontiersin.org/articles/10.3389/fpls.2021.690231/full#supplementary-material
References
Aliaga-Franco, N., Zhang, C., Presa, S., Srivastava, A. K., Granell, A., Alabadí, D., et al. (2019). Identification of transgene-free CRISPR-edited plants of rice, tomato, and Arabidopsis by monitoring DsRED fluorescence in dry seeds. Front Plant Sci. 18:1150. doi: 10.3389/fpls.2019.01150
Argentieri, M. P., DAddabbo, T., Tava, A., Agostinelli, A., Jurzysta, M., and Avato, P. (2008). Evaluation of nematicidal properties of saponins from Medicago spp. Eur. J. Plant Pathol. 120, 189–197. doi: 10.1007/s10658-007-9207-8
Augustin, J. M., Kuzina, V., Andersen, S. B., and Bak, S. (2011). Molecular activities, biosynthesis and evolution of triterpenoid saponins. Phytochem. 72, 435–457. doi: 10.1016/j.phytochem.2011.01.015
Avato, P., Bucci, R., Tava, A., Vitali, C., Rosato, A., Bialy, Z., et al. (2006). Antimicrobial activity of saponins from Medicago spp.: structure–activity relationship. Phytother. Res. 20, 454–457. doi: 10.1002/ptr.1876
Avato, P., Migoni, D., Argentieri, M., Fanizzi, F. P., and Tava, A. (2017). Activity of saponins from Medicago species against HeLa and MCF-7 cell lines and their capacity to potentiate cisplatin effect. Anticancer Agents Med. Chem. 17, 1508–1518. doi: 10.2174/1871520617666170727152805
Balestrazzi, A., Agoni, V., Tava, A., Avato, P., Biazzi, E., Raimondi, E., et al. (2011). Cell death induction and nitric oxide biosynthesis in white poplar (Populus alba) suspension cultures exposed to alfalfa saponins. Physiol. Plant. 141, 227–238. doi: 10.1111/j.1399-3054.2010.01436.x
Biazzi, E., Carelli, M., Tava, A., Abbruscato, P., Losini, I., Avato, P., et al. (2015). CYP72A67 catalyzes a key oxidative step in Medicago truncatula hemolytic saponin biosynthesis. Mol. Plant 8, 1493–1506. doi: 10.1016/j.molp.2015.06.003
Bora, K. S., and Sharma, A. (2011). Phytochemical and pharmacological potential of Medicago sativa: a review. Pharm. Biol. 49, 211–220. doi: 10.3109/13880209.2010.504732
Brinkman, E. K., Chen, T., Amendola, M., and van Steensel, B. (2014). Easy quantitative assessment of genome editing by sequence trace decomposition. Nucleic Acids Res. 42:e168. doi: 10.1093/nar/gku936
Carelli, M., Biazzi, E., Panara, F., Tava, A., Scaramelli, L., Porceddu, A., et al. (2011). Medicago truncatula CYP716A12 is a multifunctional oxidase involved in the biosynthesis of hemolytic saponins. Plant Cell 23, 3070–3081. doi: 10.1105/tpc.111.087312
Carelli, M., Biazzi, E., Tava, A., Losini, I., Abbruscato, P., Depedro, C., et al. (2015). Sapogenin content variation in Medicago inter-specific hybrid derivatives highlights some aspects of saponin synthesis and control. New Phytol. 206, 303–314. doi: 10.1111/nph.13162
Carelli, M., Confalonieri, M., Tava, A., Biazzi, E., Calderini, O., Abbruscato, P., et al. (2020). “Saponin synthesis in Medicago truncatula plants: CYP450-mediated formation of sapogenins in the different plant organs,” in The Model Legume Medicago truncatula, eds F. J. de Bruijn (Bridgewater, NJ: John Wiley & Sons, Inc.), 225–236.
Confalonieri, M., Cammareri, M., Biazzi, E., Pecchia, P., Fevereiro, M. P., Balestrazzi, A., et al. (2009). Enhanced triterpene saponin biosynthesis and root nodulation in transgenic barrel medic (Medicago truncatula Gaertn.) expressing a novel beta-amyrin synthase (AsOXA1) gene. Plant Biotechnol J. 7, 172–182. doi: 10.1111/j.1467-7652.2008.00385.x
Confalonieri, M., and Sparvoli, F. (2020). “Recent advances in Medicago spp. genetic engineering strategies,” in The Model Legume Medicago truncatula, ed. F. J. de Bruijn (Bridgewater, NJ: John Wiley & Sons, Inc.), 1149–1161.
Curtin, S. J., Tiffin, P., Guhlin, J., Trujillo, D. I., Burghardt, L. T., Atkins, P., et al. (2017). Validating genome-wide association candidates controlling quantitative variation in nodulation. Plant Physiol. 173, 921–931. doi: 10.1104/pp.16.01923
Curtin, S. J., Xiong, Y., Michno, J. M., Campbell, B. W., Stec, A. O., Cermák, T., et al. (2018). CRISPR/Cas9 and TALENs generate heritable mutations for genes involved in small RNA processing of Glycine max and Medicago truncatula. Plant Biotechnol. J. 16, 1125–1137. doi: 10.1111/pbi.12857
da Silva Magedans, Y. Y., Phillips, M. A., and Fett-Neto, A. G. (2020). Production of plant bioactive triterpenoid saponins: from metabolites to gene and back. Phytochem. Rev. 20, 461–482. doi: 10.1007/s11101-020-09722-4
D'Addabbo, T., Argentieri, M. P., Zuchowski, J., Biazzi, E., Tava, A., Oleszek, W., et al. (2020). Activity of saponins from Medicago species against phytoparasitic nematodes. Plants 9:443. doi: 10.3390/plants9040443
Do, P. T., Nguyen, C. X., Bui, H. T., Tran, L. T. N., Stacey, G., Gillman, J. D., et al. (2019). Demonstration of highly efficient dual gRNA CRISPR/Cas9 editing of the homeologous GmFAD2–1A and GmFAD2–1B genes to yield a high oleic, low linoleic and α-linolenic acid phenotype in soybean. BMC Plant Biol. 19:311. doi: 10.1186/s12870-019-1906-8
Doudna, J. A., and Charpentier, E. (2014). The new frontier of genome engineering with CRISPR-Cas9. Science 346:1258096. doi: 10.1126/science.1258096
Ellen, D., Ellen, L., Danny, G., and Guy, S. (2007). Novel advances with plant saponins as natural insecticides to control pest insects. Pest Tech. 1, 96–105.
Faè, M., Balestrazzi, A., Confalonieri, M., Donà, M., Macovei, A., Valassi, A., et al. (2014). Copper-mediated genotoxic stress is attenuated by the overexpression of the DNA repair gene MtTdp2α (tyrosyl-DNA phosphodiesterase 2) in Medicago truncatula plants. Plant Cell Rep. 33, 1071–1080. doi: 10.1007/s00299-014-1595-6
Fu, Y., Foden, J. A., Khayter, C., Maeder, M. L., Reyon, D., Joung, J. K., et al. (2013). High-frequency off-target mutagenesis induced by CRISPR-Cas nucleases in human cells. Nat. Biotechnol. 31, 822–826. doi: 10.1038/nbt.2623
Fukushima, E. O., Seki, H., Ohyama, K., Ono, E., Umemoto, N., Mizutani, M., et al. (2011). CYP716A subfamily members are multifunctional oxidases in triterpenoid biosynthesis. Plant Cell Physiol. 52, 2050–2061. doi: 10.1093/pcp/pcr146
Fukushima, E. O., Seki, H., Sawai, S., Suzuki, M., Ohyama, K., Saito, K., et al. (2013). Combinatorial biosynthesis of legume natural and rare triterpenoids in engineered yeast. Plant Cell Physiol. 54, 740–749. doi: 10.1093/pcp/pct015
Gao, R., Feyissa, B. A., Croft, M., and Hannoufa, A. (2018). Gene editing by CRISPR/Cas9 in the obligatory outcrossing Medicago sativa. Planta 247, 1043–1050. doi: 10.1007/s00425-018-2866-1
Go, Y. S., Lee, S. B., Kim, H. J., Kim, J., Park, H. Y., Kim, J. K., et al. (2012). Identification of marneral synthase, which is critical for growth and development in Arabidopsis. Plant J. 72, 791–804. doi: 10.1111/j.1365-313X.2012.05120.x
Hahn, F., and Nekrasov, V. (2019). CRISPR/Cas precision: do we need to worry about off-targeting in plants? Plant Cell Rep. 38, 437–441. doi: 10.1007/s00299-018-2355-9
Haralampidisis, K., Trojanowska, M., and Osbourn, A. (2002). “Biosynthesis of triterpenoid saponins in plants,” in Advances in Biochemical Engineering/Biotechnology, ed T. Scheper (Berlin Heidelberg: Springer), 31–49.
Kapusta, I., Bogdan, J., Stochmal, A., and Oleszek, W. (2005). Determination of saponins in aerial parts of barrel medic (Medicago truncatula) by liquid chromatography-electrospray ionization/mass spectrometry. J. Agric. Food Chem. 53, 7654–7660. doi: 10.1021/jf051256x
Krishnamurthy, P., Fujisawa, Y., Takahashi, Y., Abe, H., Yamane, K., Mukaiyama, K., et al. (2019). High throughput screening and characterization of a high-density soybean mutant library elucidate the biosynthesis pathway of triterpenoid saponins. Plant Cell Physiol. 60, 1082–1097. doi: 10.1093/pcp/pcz025
Lambert, E., Faizal, A., and Geelen, D. (2011). Modulation of triterpene saponin production: in vitro cultures, elicitation, and metabolic engineering. Appl. Biochem. Biotechnol. 164, 220–237. doi: 10.1007/s12010-010-9129-3
Lei, Z., Watson, B. S., Huhman, D., Yang, D. S., and Sumner, L. W. (2019). Large-scale profiling of saponins in different ecotypes of Medicago truncatula. Front. Plant Sci. 10:850. doi: 10.3389/fpls.2019.00850
Li, X., Wang, Y., Chen, S., Tian, H., Fu, D., Zhu, B., et al. (2018). Lycopene is enriched in tomato fruit by CRISPR/Cas9-mediated multiplex genome editing. Front Plant Sci. 9:559. doi: 10.3389/fpls.2018.00559
Liu, Y., Merrick, P., Zhang, Z., Ji, C., Yang, B., and Fei, S. Z. (2018). Targeted mutagenesis in tetraploid switchgrass (Panicum virgatum L.) using CRISPR/Cas9. Plant Biotechnol. J. 16, 381–393. doi: 10.1111/pbi.12778
Ma, X., Zhang, Q., Zhu, Q., Liu, W., Chen, Y., Qiu, R., et al. (2015). A robust CRISPR/Cas9 system for convenient, high-efficiency multiplex genome editing in monocot and dicot plants. Mol. Plant 8, 1274–1284. doi: 10.1016/j.molp.2015.04.007
Maestrini, M., Tava, A., Mancini, S., Salari, F., and Perrucci, S. (2019). In vitro anthelmintic activity of saponins derived from Medicago spp. plants against donkey gastrointestinal nematodes. Vet. Sci. 6:35. doi: 10.3390/vetsci6020035
Maestrini, M., Tava, A., Mancini, S., Tedesco, D., and Perrucci, S. (2020). In vitro anthelmintic activity of saponins from Medicago spp. against sheep gastrointestinal nematodes. Molecules 25:242. doi: 10.3390/molecules25020242
Maioli, A., Gianoglio, S., Moglia, A., Acquadro, A., Valentino, D., Milani, A. M., et al. (2020). Simultaneous CRISPR/Cas9 editing of three PPO genes reduces fruit flesh browning in Solanum melongena L. Front. Plant Sci. 11:607161. doi: 10.3389/fpls.2020.607161
Manghwar, H., Lindsey, K., Zhang, X., and Jin, S. (2019). CRISPR/Cas system: recent advances and future prospects for genome editing. Trend Plant Sci. 24, 1102–1125. doi: 10.1016/j.tplants.2019.09.006
Meng, Y., Hou, Y., Wang, H., Ji, R., Liu, B., Wen, J., et al. (2017). Targeted mutagenesis by CRISPR/Cas9 system in the model legume Medicago truncatula. Plant Cell Rep. 36, 371–374. doi: 10.1007/s00299-016-2069-9
Michno, J. M., Wang, X., Liu, J., Curtin, S. J., Kono, T. J. Y., and Stupar, R. M. (2015). CRISPR/Cas mutagenesis of soybean and Medicago truncatula using a new web-tool and a modified Cas9 enzyme. GM Crops Food 6, 243–252. doi: 10.1080/21645698.2015.1106063
Moses, T., Papadopoulou, K. K., and Osbourn, A. (2014a). Metabolic and functional diversity of saponins, biosynthetic intermediates and semi-synthetic derivatives. Crit. Rev. Biochem. Mol. Biol. 49, 439–462 doi: 10.3109/10409238.2014.953628
Moses, T., Thevelein, J. M., Goossens, A., and Pollier, J. (2014b). Comparative analysis of CYP93E proteins for improved microbial synthesis of plant triterpenoids. Phytochem. 108, 47–56. doi: 10.1016/j.phytochem.2014.10.002
Naoumkina, M. A., Modolo, L. V., Huhman, D. V., UrbanczykWochniak, E., Tang, Y., Sumner, L. W., et al. (2010). Genomic and coexpression analyses predict multiple genes involved in triterpene saponin biosynthesis in Medicago truncatula. Plant Cell 22, 850–866. doi: 10.1105/tpc.109.073270
Neves, L. O. (2000). Regeneration and Transformation of Barrel Medic (Medicago truncatula Gaertn. cv. Jemalong) (PhD dissertation thesis). Faculdade de Ciências da Universidade de Lisboa, Lisboa.
Nian, C. S., Neelakandan Anjanasree, K., Nahampun, H., Bronwyn, F., Main, M., Spalding Martin, H., et al. (2017). An Agrobacterium-delivered CRISPR/Cas9 system for high-frequency targeted mutagenesis in maize. Plant Biotechnol J. 15, 257–268. doi: 10.1111/pbi.12611
Oleszek, W., Jurzysta, M., Ploszynski, M., Colquhoun, I. J., Price, K. R., and Fenwick, G. R. (1992). Zahnic acid tridesmoside and other dominant saponins from alfalfa (Medicago sativa) aerial parts. J. Agric. Food Chem. 40, 191–196. doi: 10.1021/jf00014a005
Pateraki, I., Heskes, A. M., and Hamberger, B. (2015). Cytochromes P450 for terpene functionalisation and metabolic engineering. Adv. Biochem. Eng. Biotechnol. 148, 107–139. doi: 10.1007/10_2014_301
Pauwels, L., De Clercq, R., Goossens, J., Iñigo, S., Williams, C., Ron, M., et al. (2018). A dual sgRNA approach for functional genomics in Arabidopsis thaliana. G3 Genes Genomes Genet. 8, 2603–2615. doi: 10.1534/g3.118.200046
Pay, A., Heberle-Bors, E., and Hirt, H. (1992). An alfalfa cDNA encodes a protein with homology to translationally controlled human tumor protein. Plant Mol. Biol. 19, 501–503. doi: 10.1007/BF00023399
Rafińska, K., Pomastowski, P., Wrona, O., Górecki, R., and Buszewski, B. (2017). Medicago sativa as a source of secondary metabolites for agriculture and pharmaceutical industry. Phytochem. Lett. 20, 520–539. doi: 10.1016/j.phytol.2016.12.006
Ribeiro, B., Lacchini, E., Bicalho, K., Mertens, J., Arendt, P., Vanden Bossche, R., et al. (2020). A seed-specific regulator of triterpene saponin biosynthesis in Medicago truncatula. Plant Cell 32, 2020–2042. doi: 10.1105/tpc.19.00609
Sedeek, K. E. M., Mahas, A., and Mahfouz, M. (2019). Plant genome engineering for targeted improvement of crop traits. Front. Plant Sci. 10:114. doi: 10.3389/fpls.2019.00114
Sparg, S. G., Light, M. E., and van Staden, J. (2004). Biological activities and distribution of plant saponins. J. Ethnopharmacol. 94, 219–243. doi: 10.1016/j.jep.2004.05.016
Suzuki, H., Achnine, L., Xu, R., Matsuda, S. P., and Dixon, R. A. (2002). A genomicsapproach to the early stages of triterpene saponin biosynthesis in Medicago truncatula. Plant J. 32, 1033–1048. doi: 10.1046/j.1365-313X.2002.01497.x
Suzuki, H., Fukushima, E. O., Shimizu, Y., Seki, H., Fujisawa, Y., Ishimoto, M., et al. (2019). Lotus japonicus triterpenoid profile and characterization of the CYP716A51 and LjCYP93E1 genes involved in their biosynthesis in planta. Plant Cell Physiol. 60, 2496–2509. doi: 10.1093/pcp/pcz145
Tava, A., and Avato, P. (2006). Chemical and biological activity of triterpene saponins from Medicago Species. Nat. Prod. Comm. 12, 1159–1180. doi: 10.1177/1934578X0600101217
Tava, A., Biazzi, E., Mella, M., Quadrelli, P., and Avato, P. (2017). Artefact formation during acid hydrolysis of saponins from Medicago spp. Phytochem. 238, 116–127. doi: 10.1016/j.phytochem.2017.02.018
Tava, A., Biazzi, E., Ronga, D., Mella, M., Doria, F., Accogli, R., et al. (2020). Triterpenic saponins from Medicago marina L. Phytochem. 174:112333. doi: 10.1016/j.phytochem.2020.112333
Tava, A., Scotti, C., and Avato, P. (2011). Biosynthesis of saponins in the genus Medicago. Phytochem Rev. 10, 459–469. doi: 10.1007/s11101-010-9169-x
Tzin, V., Snyder, J. H., Yang, D. S., Huhman, D. V., Watson, B. S., Allen, S. N., et al. (2019). Integrated metabolomics identifies CYP72A67 and CYP72A68 oxidases in the biosynthesis of Medicago truncatula oleanate sapogenins. Metabolomics 15:85. doi: 10.1007/s11306-019-1542-1
Vazquez-Vilar, M., Bernabe-Orts, J. M., Fernandez-Del-Carmen, A., Ziarsolo, P., Blanca, J., and Granell, A. (2016). A modular toolbox for gRNA-Cas9 genome engineering in plants based on the golden braid standard. Plant Methods 12, 10–21. doi: 10.1186/s13007-016-0101-2
Wang, Q., Liu, J., Li, H., Yang, S., Körmöczi, P., Kereszt, A., et al. (2018). Nodule-specific cysteine-rich peptides negatively regulate nitrogen-fixing symbiosis in a strain-specific manner in Medicago truncatula. Mol. Plant Microbe Interact. 31, 240–248. doi: 10.1094/MPMI-08-17-0207-R
Wolabu, T. W., Park, J. J., Chen, M., Cong, L., Ge, Y., Jiang, Q., et al. (2020). Improving the genome editing efficiency of CRISPR/Cas9 in Arabidopsis and Medicago truncatula. Planta 252:15. doi: 10.1007/s00425-020-03415-0
Xu, J., Wang, X., and Guo, W. (2015). The cytochrome P450 superfamily: key players in plant development and defense. J. Integr. Agric. 14, 1673–1686. doi: 10.1016/S2095-3119(14)60980-1
Yendo, A. C. A., de Costa, F., Gosmann, G., and Fett-Neto, A. G. (2010). Production of plant bioactive triterpenoid saponins: elicitation strategies and target genes to improve yields. Mol. Biotechnol. 46, 94–104. doi: 10.1007/s12033-010-9257-6
Keywords: Medicago truncatula, triterpene saponin, CRISPR/Cas9, secondary metabolism, genome editing, cytochrome P450
Citation: Confalonieri M, Carelli M, Gianoglio S, Moglia A, Biazzi E and Tava A (2021) CRISPR/Cas9-Mediated Targeted Mutagenesis of CYP93E2 Modulates the Triterpene Saponin Biosynthesis in Medicago truncatula. Front. Plant Sci. 12:690231. doi: 10.3389/fpls.2021.690231
Received: 02 April 2021; Accepted: 29 June 2021;
Published: 26 July 2021.
Edited by:
Toshiya Muranaka, Osaka University, JapanReviewed by:
Marco Betti, Sevilla University, SpainAmit Srivastava, Purdue University, United States
Copyright © 2021 Confalonieri, Carelli, Gianoglio, Moglia, Biazzi and Tava. This is an open-access article distributed under the terms of the Creative Commons Attribution License (CC BY). The use, distribution or reproduction in other forums is permitted, provided the original author(s) and the copyright owner(s) are credited and that the original publication in this journal is cited, in accordance with accepted academic practice. No use, distribution or reproduction is permitted which does not comply with these terms.
*Correspondence: Massimo Confalonieri, bWFzc2ltby5jb25mYWxvbmllcmkmI3gwMDA0MDtjcmVhLmdvdi5pdA==