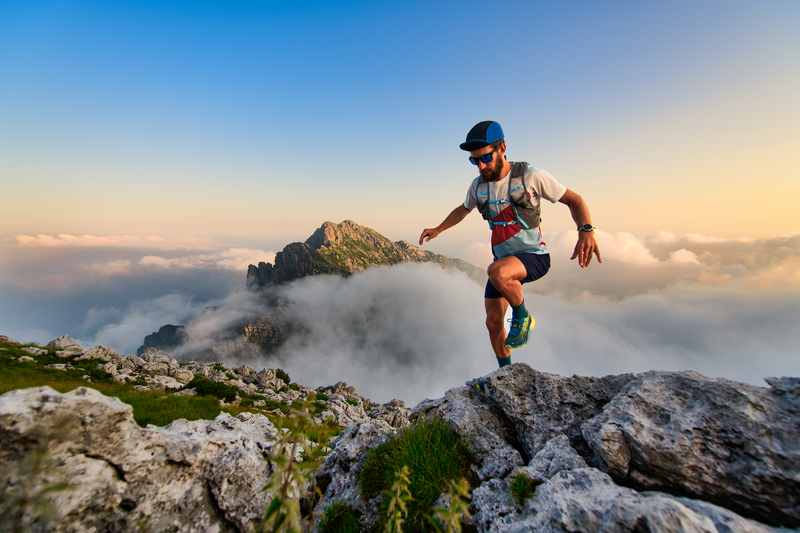
95% of researchers rate our articles as excellent or good
Learn more about the work of our research integrity team to safeguard the quality of each article we publish.
Find out more
ORIGINAL RESEARCH article
Front. Plant Sci. , 07 June 2021
Sec. Plant Biotechnology
Volume 12 - 2021 | https://doi.org/10.3389/fpls.2021.683762
This article is part of the Research Topic Engineering the Plant Biofactory for the Production of Biologics and Small-Molecule Medicines, Volume 2 View all 13 articles
Gaucher disease is an inherited lysosomal storage disorder caused by a deficiency of functional enzyme β-glucocerebrosidase (GCase). Recombinant GCase has been used in enzyme replacement therapy to treat Gaucher disease. Importantly, the terminal mannose N-glycan structure is essential for the uptake of recombinant GCase into macrophages via the mannose receptor. In this research, recombinant GCase was produced using Agrobacterium-mediated transient expression in both wild-type (WT) and N-acetylglucosaminyltransferase I (GnTI) downregulated Nicotiana benthamiana (ΔgntI) plants, the latter of which accumulates mannosidic-type N-glycan structures. The successfully produced functional GCase exhibited GCase enzyme activity. The enzyme activity was the same as that of the conventional mammalian-derived GCase. Notably, N-glycan analysis revealed that a mannosidic-type N-glycan structure lacking plant-specific N-glycans (β1,2-xylose and α1,3-fucose residues) was predominant in all glycosylation sites of purified GCase produced from ΔgntI plants. Our research provides a promising alternative plant line as a host for the production of recombinant GCase with a mannosidic-type N-glycan structure. This glycoengineered plant might be applicable to the production of other pharmaceutical proteins, especially mannose receptor targeted protein, for therapeutic uses.
Gaucher disease, one of the most common lysosomal storage disorders, is caused by the mutation of the GBA1 gene, resulting in the defective activity of a lysosomal enzyme called glucocerebrosidase (GCase, β-glucosidase; EC: 3.2.1.45). Enzyme replacement therapy (ERT) is the most effective treatment for Gaucher disease type 1; it involves intravenous infusions of exogenous recombinant GCase to patients (Siebert et al., 2014; Stirnemann et al., 2017; Boer et al., 2020). Three drugs are commercially available: Imiglucerase (Cerezyme®, Genzyme Corporation), Velaglucerase alfa (VPRIV®, Shire Plc), and Taliglucerase alfa (Elelyso®, Pfizer). These drugs are recombinant GCases produced in Chinese hamster ovary (CHO) cells, human fibroblasts, and carrot suspension cells, respectively. Moreover, all are glycoproteins containing different N-glycan structures (Bennett and Fellner, 2018).
Glycosylation is the enzymatic transfer and attachment of glycans to specific sites on proteins. It is important for certain protein characteristics, including folding, solubility, stability, functionality, half-life, and quality control (Castilho and Steinkellner, 2012; Loos and Steinkellner, 2014; Schoberer and Strasser, 2018; Zhou and Qiu, 2019). Since recombinant GCase is taken up by macrophage via the mannose receptor, the terminal mannose N-glycan structure is essential for the translocation of commercial drugs into macrophages (Friedman et al., 1999). For this reason, the N-glycan structures of three commercially available GCases have been modified using different approaches in order to possess terminal mannose residues. First, Imiglucerase has been modified by exoglycosidase digestion during the post-production process, whereas mannosidic-type N-glycan of Velaglucerase alfa has been achieved by the addition of kifunensine, a mannosidase I inhibitor, into medium. On the other hand, Taliglucerase alfa has been modified by targeting GCase to the vacuole in order to produce the pauci-mannosidic structure (Van Patten et al., 2007; Tekoah et al., 2013; Kallemeijn et al., 2017). Although Imiglucerase is the leading brand, its manufacturing plants were shut down due to viral contamination in 2009, which resulted in a shortage of drugs for ERT (Hollak et al., 2010). Compared to those two drugs, Taliglucerase alfa is free from contamination by human pathogens and does not require post-production modification (Grabowski et al., 2014; Gupta and Pastores, 2017; Zimran et al., 2018). Even though drugs are commercially available, a large amount of recombinant GCase is required for ERT. Recently, administration of an ERT drug at a dosage of 60 Units/kg body weight every 2 weeks costs ~$128,000 to $544,000 per patient annually. Thus, Gaucher disease drugs have been reported to be among the most expensive drugs available (Joerg et al., 2007; Burnett and Burnett, 2020).
A plant-based expression platform has many advantages for the production of pharmaceutical recombinant proteins, including low cultivation costs, scale-up possibility, complex protein production ability with post-translational modifications, and a low risk of contamination by human pathogens (Paul and Ma, 2011; Moon et al., 2019; Burnett and Burnett, 2020; Shanmugaraj et al., 2020). After Taliglucerase alfa became the first plant-made pharmaceutical approved by the U.S. Food and Drug Administration in 2012, studies on the use of plants for pharmaceutical and non-pharmaceutical recombinant protein production have been increasing (Van Dussen et al., 2013; Tschofen et al., 2016). Many studies have focused on the production of recombinant GCase in plants including Arabidopsis thaliana (He et al., 2012), rice suspension culture (Nam et al., 2017; Jung et al., 2019), and Nicotiana benthamiana (Limkul et al., 2015, 2016; Naphatsamon et al., 2018). However, some studies did not report the N-glycan structure of recombinant GCase (Limkul et al., 2015; Nam et al., 2017), whereas one study revealed the majority of N-glycan structure containing residues of plant-specific glycan (β1,2-xylose and α1,3-fucose) (Naphatsamon et al., 2018). Those nonhuman glycosylations have the potential to induce immunogenic responses in human (Gomord et al., 2005, 2010; Montero-Morales and Steinkellner, 2018). N-glycan modification is one of the approaches to overcome this problem. Many plant-based glycoengineering studies have focused on the knockdown or knockout of the N-acetyltglucosaminyltransferase I (GnTI) gene, leading to mannosidic-type structures and to the absence of plant-specific glycan residues (Strasser, 2014; Limkul et al., 2016; Montero-Morales and Steinkellner, 2018).
Agrobacterium-mediated transient expression (agroinfiltration) is widely used to produce large amounts of protein due to its high expression level. It also has other advantages, including effectiveness, low-cost production, time saving and scalability (Moon et al., 2019). Notably, this system is useful for pandemic responses due to rapid gene introduction (Burnett and Burnett, 2020). For instance, the end product of an influenza vaccine was produced within 3 weeks by the Medicago company using plant transient expression (D'Aoust et al., 2010). Compared to other plants, N. benthamiana has been widely used for recombinant protein production because of its high biomass and susceptibility to a wide range of plant pathogens, the latter of which is useful for introducing foreign genes (Goodin et al., 2008).
In this study, we developed an alternative system to produce recombinant GCase with mannosidic-type N-glycan structure in WT and glycoengineered N. benthamiana plants (NbGNTI-RNAi7; ΔgntI) (Limkul et al., 2016) using Agrobacterium-mediated transient expression. After purification using two steps of column chromatography, purified recombinant GCase was analyzed by an enzymatic activity assay. The glycan structure of purified recombinant GCase was analyzed at three glycosylated positions. Our work demonstrated that recombinant GCase was successfully produced as an active glycoprotein with a mannosidic-type glycan structure.
Stable glycoengineered gntI-knockdown N. benthamiana plants [NbGNTI-RNAi7; ΔgntI) T7 were generated as described previously (Limkul et al., 2016). Seeds of ΔgntI plants were sterilized using PPM solution (Plant Preservative Mixture; Plant Cell Technology, USA) and selected on a Murashige and Skoog (MS) medium agar plate supplemented with hygromycin (30 mg/L). Germinated seedlings were transferred to soil pots and grown at 25°C under a 16 h light and 8 h dark condition for 4 weeks. Seeds of WT N. benthamiana were geminated on soil and grown under same condition as described above.
Agrobacterium tumefaciens strain LBA4404 harboring GCase expression plasmid (pAt-GC-HSP) was constructed previously (Limkul et al., 2015) (Figure 1A). A vector for the expression of RNA silencing suppressor 19 (p19) was kindly provided by Prof. Atsushi Takeda (Ritsumeikan University). The p19 vector was transformed into A. tumefaciens by electroporation. An A. tumefaciens transformant was selected using kanamycin (50 mg/L), rifampicin (50 mg/L), and streptomycin (50 mg/L).
Figure 1. Transient production of recombinant GCase in wild-type (WT) and ΔgntI N. benthamiana plants. (A) The pAt-GC-HSP expression vector. 35S promoter: Cauliflower mosaic virus 35S RNA promoter; AtADH 5'UTR: 5'-UTR of Arabidopsis alcohol dehydrogenase (AtADH) gene; β-Glucocerebrosidase: β-glucocerebrosidase gene; HSP terminator: Transcription terminator from A. thaliana heat shock protein; Bar: bialaphos resistance gene; Km: Kanamycin resistance gene. (B) Infiltrated leaves and (C) GCase activity of crude protein from infiltrated WT and ΔgntI leaves at 7 days post-infiltration. NC: non-infiltrated leaves (negative control). The experiment was performed in five replications. Error bars represent the standard deviation (SD). (D) Total soluble protein: 10, 5, and 1 μg were loaded and analyzed by Coomassie Brilliant Blue (CBB) staining, anti-GCase blotting, and anti-HRP blotting, respectively. M, Precision Plus Protein standards marker; WT, wild-type N. benthamiana; ΔgntI, gntI-knockdown N. benthamiana; (-), non-infiltrated leaves (negative control); (+), Cerezyme®, a commercial GCase produced in Chinese Hamster Ovary (CHO) cells. Arrows represent the GCase band.
A single colony of Agrobacterium was inoculated into 2xYT liquid medium supplemented with the appropriate antibiotics and cultivated at 28°C overnight. Two milliliters of culture was inoculated into 2xYT liquid medium (200 ml) supplemented with the same antibiotics and cultivated at 28°C overnight. Cells were collected by centrifugation at 4,000 × g. Agrobacterium containing different vectors (GCase or p19) was resuspended and mixed at a 1:1 ratio in infiltration buffer (10 mM MES, 10 mM MgSO4, 100 μM acetosyringone, pH 5.5) with OD600 of 0.5. The Agrobacterium mixture was incubated at room temperature for 2–4 h. Both WT and ΔgntI N. benthamiana plants were infiltrated using vacuum infiltration (Chen et al., 2013). Infiltrated plants were grown at 25°C under 16 h light and 8 h dark condition for 1 week.
Agrobacterium culture and infiltration buffer were prepared as described above. For OD600 optimization, 4-week-old N. benthamiana plants were infiltrated in the Agrobacterium mixture with different OD600 values: 0, 0.25, 0.50, 0.75, and 1.0. Infiltrated plants were grown at 25°C under 16 h light and 8 h dark condition. Agroinfiltrated leaves were collected at 7 days post-infiltration (dpi). For the optimization of harvest time, N. benthamiana plants were infiltrated with the Agrobacterium mixture as described above (OD600 = 0.5). Agroinfiltrated leaves were collected at 5, 6, 7, and 8 days post-infiltration (dpi).
The protein extraction method was modified from a previous study (Limkul et al., 2015). Briefly, leaves were homogenized by grinding with liquid nitrogen. Leaf powders were suspended in GCase extraction buffer (20 mM Tris-HCl pH 7.0, 150 mM NaCl, 0.5% taurocholic acid, and ethylenediaminetetraacetic acid (EDTA)-free Protease Inhibitor Cocktail (Roche Diagnostics GmbH, Germany) and centrifuged at 12,000 × g for 15 min. The supernatant was collected and analyzed by a GCase activity assay, a Bradford assay, SDS-PAGE, and Western blotting, as described below.
The enzyme activity assay was performed as described previously (Limkul et al., 2015). Briefly, crude extract was co-incubated with GCase assay buffer (60 mM phosphate-citrate buffer pH 6.0, 4 mM β-mercaptoethanol, 1.3 mM EDTA, 0.15% Triton X-100, 0.125% taurocholic acid) and 0.2 mM of 4-methylumbelliferyl β-D-glucopyranoside (4-MUG; Wako, Japan) at 37°C for 1 h. The enzymatic reaction was terminated using cold glycine stop buffer (0.2 M glycine, 0.125 M sodium carbonate, pH 10.7). The fluorescence of the product, 4-methylumbelliferone (4-MU; Wako), was detected using an F-25000 fluorescence spectrophotometer (Hitachi High-Technologies, Japan) at λex = 365 nm and λem = 460 nm. The amount of reaction product from the GCase activity assay was calculated using the 4-MU standard curve. An enzyme unit was defined as the amount of enzyme used to release 1 nmol of 4-MU/min. GCase enzyme activity was calculated by Units/mg of total soluble protein (TSP).
Crude extracted protein of agroinfiltrated leaves was separated by 10% sodium dodecyl sulfate-polyacrylamide gel electrophoresis (SDS-PAGE). Proteins were stained using Coomassie Brilliant Blue (CBB) following the manufacturer's instructions (Ready to Use; Nacalai Tesque, Japan). For Western blot analysis, proteins in polyacrylamide gel were transferred to a polyvinylidene difluoride (PVDF) membrane (Millipore, USA). The membrane was incubated with skim milk (5%) in PBS-T (1.47 mM KH2PO4, 10 mM Na2HPO4, 2.7 mM KCl, 137 mM NaCl, 0.05% Tween-20, pH 7.4) at room temperature for 1 h. Then it was further incubated with 1:5,000 diluted polyclonal anti-glucocerebrosidase (GCase) antibody from rabbit (Sigma-Aldrich, USA) or polyclonal anti-horseradish peroxidase from rabbit (Sigma-Aldrich) with 1:12,500 dilution at 25°C for 1 h. The membrane was washed with PBS-T and further incubated with the 1:5,000 diluted anti-rabbit IgG, HRP-linked whole antibody (GE Healthcare, Japan) at 25°C for 1 h. After washing with PBS-T, proteins were detected by incubation with LuminataTM (Millipore) at room temperature for 5 min using the iBright Imaging System (Invitrogen, USA).
Purification was performed as described previously (Limkul et al., 2016). Agroinfiltrated leaves were homogenized by grinding with liquid nitrogen. Leaf powders were suspended in GCase extraction buffer and incubated on ice for 20 min. Crude extracts were collected using centrifugation (10,000 x g for 20 min) and loaded into a Concanavalin (Con A)- Agarose column (Vector Laboratories, USA) using a peristaltic pump (Perista Pump SJ-1211; ATTO, Japan) with a recycling system at 4°C for 12 h. The column was washed with Con A buffer (500 mM NaCl, 1 mM MgCl2, 1 mM MnCl2, 1 mM CaCl2 in 20 mM Tris-HCl pH 7.0). Proteins were eluted with GCase assay buffer containing 300 mM α-methyl-D-mannoside (Nacalai Tesque). Each eluted fraction was analyzed by a GCase enzymatic assay. All eluted fractions with GCase activity were collected and resuspended in 2 M NaCl. Protein solution was loaded onto a Phenyl 650M column (Tosoh, Japan). Proteins were eluted by decreasing the NaCl concentration. Each elution fraction was analyzed using the GCase enzyme assay. Active fractions were dialyzed and concentrated using an Amicon® Ultra centrifugal filter (Millipore). Concentrated protein was analyzed by the GCase activity assay, Bradford assay, SDS-PAGE, and Western blotting.
Purified GCase from agroinfiltrated leaves was used for N-glycan analysis as described previously (Limkul et al., 2016). The purified GCase was separated using SDS-PAGE and stained by PageBlue protein stain solution (Thermo Fisher Scientific, USA). In-gel digestion was performed as follows: GCase bands were de-stained using 1:1 v/v of acetonitrile and 50 mM NH4HCO3 at room temperature overnight. The proteins were then digested with Trypsin Gold (Promega, USA) in ProteaseMAXTM surfactant solution (Promega) at 50°C for 1 h. The reaction was terminated by 1% trifluoroacetic acid in 60% acetonitrile. Peptides were extracted from gel and evaporated at 30°C for 3 h. Peptides were dissolved in 0.1% formic acid and applied to an ESI-Qq-TOF mass spectrometer (microTOF-Q II, Bruker Daltonics, Germany). The conditions used for N-glycan analysis were as described previously (Limkul et al., 2016). Briefly, a nano-LC system (120 series; Agilent Technologies) was used with a trap column (5 μ, 0.3 × 5 mm) and an analytical column (3.5 μ, 0.075 x 150 mm). Both 0.1% formic acid in water and 0.1 % formic acid in acetonitrile were used as the mobile phase. Peptides were trapped in the trapped column and eluted at a flow rate of 0.6 μL/min. The system was completely controlled by microTOF control software (Bruker Daltonics). N-Glycan structures were analyzed using DataAnalysis version 4.0 (Bruker Daltonics) with both MS and MS/MS modes.
To produce recombinant GCase in N. benthamiana plants, 4-week-old plants were infiltrated with Agrobacterium mixture harboring GCase and p19 vectors at an OD600 of 0.5. At 7 days post-infiltration, leaf samples were collected for protein extraction and crude proteins were further analyzed using an enzymatic assay and protein blotting.
After infiltration, no necrosis was observed from the infiltrated WT or ΔgntI leaves (Figure 1B). The enzymatic assay detected GCase activity from crude protein of both plants (Figure 1C). The GCase produced in ΔgntI plants showed higher GCase activity (134.3 ± 17.3 U/mg TSP) than that produced in WT plants (81.5 ± 6.9 U/mg TSP). On the other hand, non-infiltrated WT and ΔgntI leaves (negative control) showed low GCase activity (4.7 ± 1.3 and 6.5 ± 1.4 U/mg TSP in WT and ΔgntI plants, respectively). The GCase activity detected in the negative control was considered as an effect of plant-produced β-glucosidase (Seshadri et al., 2009; Pankoke et al., 2013). Crude proteins were further analyzed by CBB staining and Western blotting compared to Cerezyme®, a commercial GCase produced in CHO cells as a positive control (Figure 1D). A GCase band was detected using anti-GCase antibody from crude protein extracted from agroinfiltrated WT and ΔgntI leaves with a molecular weight of ~58 kDa. On the other hand, no GCase band was detected from the negative control. Additionally, plant-specific glycans were determined using anti-HRP antibody blotting. Anti-HRP antibody binds to plant specific N-glycan residues, including β1,2-xylose and α1,3-fucose residues (Jin et al., 2008; Iskratsch et al., 2009; Paschinger et al., 2009). In this work, higher-intensity bands were detected from crude protein produced in WT plants than that of ΔgntI plants using anti-HRP antibody. This implies that the GCase produced from ΔgntI plants contains less plant specific glycan residues compared to GCase produced from WT plants. These results indicated that the functional recombinant GCase was successfully produced in both WT and ΔgntI plants. Furthermore, ΔgntI-infiltrated leaves had fewer plant-specific N-glycan structures than WT leaves.
To obtain a large amount of recombinant GCase, infiltration conditions, including the optical density (OD600) of Agrobacterium mixture and the harvest time, were optimized in this study. WT and ΔgntI plants were infiltrated using the Agrobacterium mixture with different OD600 values: 0.25, 0.50, 0.75, and 1.0. Leaf samples were collected at 7 days post-infiltration.
Crude extracted at an OD600 of 0.50 showed the highest GCase activity in both WT and ΔgntI plants (108.5 ± 27.9 and 139.5 ± 19.7 U/mg TSP, respectively), followed by that extracted at an OD600 of 0.75 (Figure 2A). However, the difference was not significant. Regarding the Western blotting result, OD600 values of 0.50 and 0.75 revealed higher intensities of GCase bands compared to OD600 values of 0.25 and 1.0 (Figure 2B). Because it had the highest GCase activity, OD600 of 0.5 was chosen as the optical density for GCase production. At harvest time, infiltrated leaf samples were collected at 5, 6, 7, and 8 days post-infiltration (dpi). The highest GCase activity was observed at 7 dpi in both WT (149.7 ± 14.5 U/mg TSP) and ΔgntI (153.6 ± 17.2 U/mg TSP) plants (Figure 2C). Additionally, GCase bands detected in anti-GCase blotting at 5 and 8 dpi showed lower intensities compared to those at 6 and 7 dpi (Figure 2D). Therefore, 7 dpi was chosen as the collection day for GCase purification.
Figure 2. Optimization of GCase transient production. GCase activity of crude protein from wild-type (WT) and ΔgntI leaves infiltrated with (A) different OD600 values and (C) different days post-infiltration. Total soluble proteins: 5 and 10 μg were loaded and analyzed using anti-GCase antibody and CBB staining, respectively (B,D). Error bars represent the standard deviation (SD). The experiment was performed in three replications. (-), negative control from non-infiltrated leaves; (+), Cerezyme®; WT, wild-type N. benthamiana; ΔgntI, glycoengineered N. benthamiana.
Two types of column chromatography were used for GCase purification: Concanavalin (Con A) affinity chromatography and phenyl hydrophobic (P650M) chromatography. The binding between Con A and mannose residues of N-glycan structure have been reported (Maupin et al., 2012). After purification, GCases from infiltrated WT and ΔgntI leaves were analyzed using the enzymatic assay and protein blotting.
With the infiltrated WT leaves, purified GCase bands were detected from P650M elution fractions 6 and 7 on CBB staining and Western blotting (Figure 3A). The GCase band was ~58 kDa, which corresponded to the size of the positive control, Cerezyme®. Additionally, no band was detected from the negative control, crude protein extracted from non-infiltrated leaves. The specific enzyme activity was measured using GCase activity assay. Purified GCase hydrolyzed the substrate, 4-MUG to glucose and 4-MU which was detected using a fluorescence spectrophotometer. The 4-MU standard curve was used to calculate the amount of purified GCase. The GCase-specific activities of purified GCase from eluted fractions 6 and 7 were ~3301.1 ± 48.4 and 1360.7 ± 8.9 U/mg TSP, respectively (Figure 3B). As expected, the GCase-specific activities of both eluted fractions were higher than that of crude protein (149.4 ± 18.3 U/mg TSP). The amounts of purified GCase from infiltrated WT leaves were ~5.4 μg and 2.7 μg in eluted fractions 6 and 7, respectively. The productivity of purified GCase was about 0.45 μg/g of fresh leaves.
Figure 3. GCase purification from infiltrated wild-type (WT) and ΔgntI N. benthamiana leaves. (A) Total proteins from infiltrated WT leaves (E6 and E7); 2.5 μg and 100 ng were loaded and analyzed by CBB staining and Western blotting, respectively. (B) GCase activity of crude protein and purified GCase from infiltrated WT leaves: elution fractions 6 and 7. Error bars represent the standard deviation (SD) (N = 3). (C) GCase activity levels of crude protein and purified GCase from infiltrated ΔgntI leaves: elution fractions 6 and 7 (E6 and E7). Error bars represent the standard deviation (SD) (N = 3). (D) CBB staining and Western blot analysis of crude protein and purified GCase from infiltrated ΔgntI leaves elution fraction 6. M, PageRuler™ protein ladder; Crude, crude protein; Purified GCase, purified GCase from Phenyl 650M elution fractions 6 (E6); +, Cerezyme®.
On the other hand, purified GCase from infiltrated ΔgntI leaves was detected from P650M eluted fractions 6 and 7. The specific activities of purified GCase from eluted fractions 6 and 7 were 2001.7 ± 7.7 and 1,000.0 ± 13.8 U/mg TSP, respectively (Figure 3C). These numbers are much higher than that from the crude protein (84.7 ± 3.2 U/mg TSP). Additionally, a purified GCase band from eluted fraction 6 was detected on CBB staining and Western blotting compared to the positive control (Figure 3D). Different amounts of purified GCase from infiltrated ΔgntI leaves were determined from eluted fractions 6 and 7 (9.8 and 17.4 μg, respectively) with an approximate productivity of 2.6 μg/g of fresh leaves.
To analyze the N-glycan structures of purified GCase from both WT and ΔgntI samples, trypsin-digested glycopeptides were analyzed using nanoLC-MS/MS.
Three N-glycosylation sites (N270, N146, and N59) were detected in purified GCase from both WT and ΔgntI plants (Figure 4). All possible N-glycan structures of each position were analyzed from deconvoluted MS spectra (Supplementary Figures 1–4). The composition percentage at each N-glycosylation site was also summarized (Table 1). In the case of WT GCase, GN2M3XF was the predominant structure at positions 59, 146 and 270 with percentages of 29.7%, 63.0%, and 38.2%, respectively. Additionally, GCase at positions N270 (100%), N146 (100%), and N59 (100%) was a N-glycosylated with at least one plant-specific N-glycans (β1,2-xylose and/or α1,3-fucose residues). On the other hand, Man5 was detected as the predominant structure at the N59 (89.2%), N146 (85%), and N270 (73.0%) positions in ΔgntI GCase. Additionally, the mannosidic-type structure without plant-specific glycan predominated in all positions, including N59 (100%), N146 (100%), and N270 (100%). These results demonstrated that recombinant GCase was successfully produced in ΔgntI plants with mannosidic-type N-glycan structures.
Figure 4. Nano LC–MS spectra of glycopeptides derived from purified GCase produced in wild-type (WT) and ΔgntI-infiltrated leaves. Glycan structures of glycopeptides are shown at different N-glycosylation sites (N59, N146, and N270).
Table 1. Composition of N-glycan structures on purified GCases from wild type and ΔgntI agroinfiltrated plants at three N-glycosylation sites (N59, N146, and N270).
A large amount of recombinant GCase is required for the ERT treatment of Gaucher disease type 1 (Burnett and Burnett, 2020). Since exogenous GCase is translocated into macrophages via the mannose receptor, the mannose terminal N-glycan structure is essential for recombinant GCase (Friedman et al., 1999). In this work, we investigated the impact of gntI-downregulated N. benthamiana plants (ΔgntI) as a host for the transient expression of recombinant GCase with a mannosidic-type N-glycan structure.
Our results demonstrated that recombinant GCase was successfully produced in WT and ΔgntI N. benthamiana plants (Figure 1). Since Agrobacterium-infiltration conditions usually affect the transformation efficiency, resulting in different expression levels of recombinant protein (Hanittinan et al., 2020), two main factors, Agrobacterium cell density and harvest time, were optimized to produce a large amount of GCase with biological activity. Previous studies demonstrated that too low cell density might lead to insufficient Agrobacterium infection, whereas too high density may cause necrosis (Kagale et al., 2012; Leuzinger et al., 2013; Yan et al., 2019). Additionally, the harvesting time also affects the necrosis and amount of protein production (Kim et al., 2021). Our work revealed that the Agrobacterium cell density (OD600) of 0.5 and the harvest time of 7 dpi were the optimal conditions for GCase transient production, as they resulted in the highest GCase enzyme activity in both WT and ΔgntI-infiltrated leaves (Figure 2). Notably, the crude GCase activity in our work was higher than in previous studies: 65.5 U/mg TSP (Limkul et al., 2016), 24 U/mg TSP (He et al., 2012), 44.5 U/mg TSP (Limkul et al., 2015), and 81.4 U/mg TSP (Naphatsamon et al., 2018). On the other hand, the low GCase activity observed in the negative control was attributed to internal β-glucosidases produced in plants, which plays important roles in plant defense systems. This enzyme is also related to plant physiology, including cell wall degradation and to the activation of both phytohormones and chemical compounds (Morant et al., 2008; Pankoke et al., 2013). Internal β-glucosidases were also reported previously (Naphatsamon et al., 2018). Our results indicated that this system has the potential to be competitive for recombinant GCase production with high levels of biological activity.
Two different GCase purification strategies have been reported: hexa histidine-tag (His-tag) and a three-step purification method (Nam et al., 2017; Jung et al., 2019). However, the removal of his-tag and the multiple steps required for purification may increase the production cost. In this study, recombinant GCase was successfully purified as a single band using two types of column chromatography (Con A and P650M columns) adapted from our current works (Limkul et al., 2015, 2016; Naphatsamon et al., 2018) (Figure 3). The specific activity levels of purified GCase from infiltrated WT and ΔgntI leaves are competitive with those of the recombinant GCase produced in WT transgenic N. benthamiana plants (2641 U/mg TSP) and N. benthamiana root culture (1618 U/mg TSP) from previous studies (Limkul et al., 2015; Naphatsamon et al., 2018). Although recombinant GCase produced in stable glycoengineered N. benthamiana revealed higher specific activity (3063 U/ mg TSP) (Limkul et al., 2016), our recombinant GCase had a higher composition of the mannose terminal N-glycan structure, which we discuss below.
Three commercially available recombinant GCases have been approved for the ERT treatment of Gaucher disease type I. The drugs are administered to patients by IV infusions and taken up into macrophages via the mannose receptor (Stirnemann et al., 2017). Moreover, GCase is translocated into lysosome using a lysosomal membrane protein called LIMP-2 (Zhao et al., 2014). It has been demonstrated that the mannose terminal N-glycan structure is essential for the macrophage uptake of ERT drugs (Van Patten et al., 2007). Therefore, the present study aimed to produce recombinant GCase with a mannosidic terminal structure and few plant-specific N-glycans. Our method relies on the knockdown of the gntI gene encoding the key enzyme for the mannose-5 terminal N-glycan structure (Strasser, 2014; Montero-Morales and Steinkellner, 2018; Amann et al., 2019). Analysis of the N-glycan composition of purified GCase from ΔgntI plants revealed that the mannose-5 terminal (Man5) structure predominated in all glycosylation sites: N59, N146, and N270 (Figure 4 and Table 1). Notably, these percentages of mannosidic-type N-glycan structure without plant-specific glycans were higher than those of the purified GCase produced from stable glycoengineered N. benthamiana (ranging from 73.3 to 85.5%) and A. thaliana complex-glycan-deficient (cgl) seeds (85%) in previous studies (He et al., 2012; Limkul et al., 2016). Additionally, the diverse structure and different degree of N-glycosylation at N59, N146, and N270 sites were considered to be a microheterogeneity caused by the inefficient enzymatic pathways of the glycoprotein biosynthesis (Zacchi and Schulz, 2016). These results indicated that our ΔgntI N. benthamiana plants have the potential to produce recombinant GCase with a mannosidic-type N-glycan structure without plant-specific N-glycans.
In our previous study, recombinant GCase was produced in the stable transgenic N. benthamiana plant (Limkul et al., 2016). Compared to the stable expression, our transient expression system provided ~2.3-fold higher crude GCase activity. The increase of crude GCase activity resulted from Agrobacterium-mediated transient expression which is more efficient than that of stable expression by the gene integration (Burnett and Burnett, 2020). Additionally, the N-glycan structures of purified GCase produced from both stable and transient expression systems were different.
In the case of WT plants, the predominant N-glycan structure of purified GCase from stable and transient plants were M3XF and GN2M3XF, respectively. The high amount of GN2M3XF structure may be caused by the rapid production in transient system. In general, two terminal GlcNAc residues of the GN2M3XF structure are cleaved by plant β-hexosaminidases (HEXOs), resulting in the M3XF structure (Altmann et al., 1995; Bosch et al., 2013). In this work, the recombinant GCase was transiently produced in a short time. Therefore, cellular stress may have been induced and HEXOs activity may not have been sufficient to completely convert the GN2M3XF into the M3XF structure. Additionally, the GA2F2GN2M3XF structure was found only in purified GCase produced in transient system, corresponding with previous report on the structures of secreted glycoprotein mostly found in the apoplast (Bosch et al., 2013).
In the case of ΔgntI plants, GCase produced through transient expression had mannosidic structures only (100%). On the other hand, some plant-type structures were remained in the purified GCase from the stable expression (ranging from 14.5 to 26.7%). Therefore, the transient expression in ΔgntI plants has a higher potential to produce recombinant GCase with mannosidic N-glycan structures.
Furthermore, this plant can be applied as a host for the production of other pharmaceutical proteins, especially mannose-targeted proteins, for therapeutic uses. The interaction between glycosylated proteins and carbohydrate-binding proteins (lectins) is important for cellular recognition processes, including cellular migration, enzyme trafficking, and immunogenic function (Irache et al., 2008). Several mannosylated structures have been considered as promising devices for drug delivery or vaccination due to their ability to bind the mannose receptor, which is highly expressed on the surfaces of immune cells such as macrophages and dendritic cells (Keler et al., 2004).
Our study demonstrated that the ΔgntI N. benthamiana plant can be used for the transient production of recombinant GCase with a mannosidic-type N-glycan structure. Furthermore, this glycoengineered plant-based transient expression system has potential as an alternative platform for the production of other recombinant therapeutic proteins. This plant is also potentially useful for therapeutic uses during pandemics and outbreaks by virtue of to their rapid response.
The raw data supporting the conclusions of this article will be made available by the authors, without undue reservation.
KF supervised and conceived the original idea of this work. NU designed and performed the experiments with technical support from HK and wrote the manuscript with support from KF, HK, and RM. All authors approved the final manuscript.
The research was supported by the Ministry of Education, Culture, Sports, science, and technology (MEXT).
The authors declare that the research was conducted in the absence of any commercial or financial relationships that could be construed as a potential conflict of interest.
We gratefully thank Dr. Juthamard Limkul for the generation of stable glycoengineered gntI-knockdown N. benthamiana plants (NbGNTI-RNAi7) used in this study. We are grateful to Prof. Atsushi Takeda from Ritsumeikan University for providing the RNA silencing suppressor 19 (p19) vector.
The Supplementary Material for this article can be found online at: https://www.frontiersin.org/articles/10.3389/fpls.2021.683762/full#supplementary-material
Altmann, F., Schwihla, H., Staudacher, E., Glössl, J., and März, L. (1995). Insect cells contain an unusual, membrane-bound beta-N-acetylglucosaminidase probably involved in the processing of protein N-glycans. J. Biol. Chem. 270, 17344–17349. doi: 10.1074/jbc.270.29.17344
Amann, T., Schmieder, V., Faustrup Kildegaard, H., Borth, N., and Andersen, M. R. (2019). Genetic engineering approaches to improve posttranslational modification of biopharmaceuticals in different production platforms. Biotechnol. Bioeng. 116, 2778–2796. doi: 10.1002/bit.27101
Bennett, L. L., and Fellner, C. (2018). Pharmacotherapy of Gaucher disease: current and future options. Pharmacol. Ther. 43, 274–309.
Boer, D. E. C., van Smeden, J., Bouwstra, J. A., and Aerts, J. M. F. G. (2020). Glucocerebrosidase: functions in and beyond the lysosome. J. Clin. Med. 9:736. doi: 10.3390/jcm9030736
Bosch, D., Castilho, A., Loos, A., Schots, A., and Steinkellner, H. (2013). N-glycosylation of plant-produced recombinant proteins. Curr. Pharm. Des. 19, 5503–5512. doi: 10.2174/1381612811319310006
Burnett, M. J. B., and Burnett, A. C. (2020). Therapeutic recombinant protein production in plants: challenges and opportunities. Plants People Planet. 2, 121–132. doi: 10.1002/ppp3.10073
Castilho, A., and Steinkellner, H. (2012). Glyco-engineering in plants to produce human-like N-glycan structures. Biotechnol. J. 7, 1088–1098. doi: 10.1002/biot.201200032
Chen, Q., Lai, H., Hurtado, J., Stahnke, J., Leuzinger, K., and Dent, M. (2013). Agroinfiltration as an effective and scalable strategy of gene delivery for production of pharmaceutical proteins. Adv. Tech. Biol. Med. 1:103. doi: 10.4172/atbm.1000103
D'Aoust, M. A., Couture, M. M., Charland, N., Trépanier, S., Landry, N., Ors, F., et al. (2010). The production of hemagglutinin-based virus-like particles in plants: a rapid, efficient and safe response to pandemic influenza. Plant Biotechnol. J. 8, 607–619. doi: 10.1111/j.1467-7652.2009.00496.x
Friedman, B., Vaddi, K., Preston, C., Mahon, E., Cataldo, J. R., and McPherson, J. M. (1999). A comparison of the pharmacological properties of carbohydrate remodeled recombinant and placental-derived beta-glucocerebrosidase: implications for clinical efficacy in treatment of Gaucher disease. Blood. 93, 2807–2816. doi: 10.1182/blood.V93.9.2807.409k08_2807_2816
Gomord, V., Chamberlain, P., Jefferis, R., and Faye, L. (2005). Biopharmaceutical production in plants: problems, solutions and opportunities. Trends Biotechnol. 23, 559–565. doi: 10.1016/j.tibtech.2005.09.003
Gomord, V., Fitchette, A. C., Menu-Bouaouiche, L., Saint-Jore-Dupas, C., Plasson, C., Michaud, D., et al. (2010). Plant-specific glycosylation patterns in the context of therapeutic protein production. Plant Biotechnol. J. 8, 564–587. doi: 10.1111/j.1467-7652.2009.00497.x
Goodin, M. M., Zaitlin, D., Naidu, R. A., and Lommel, S. A. (2008). Nicotiana benthamiana: its history and future as a model for plant-pathogen interactions. Mol. Plant Microb. Interact. 21, 1015–1026. doi: 10.1094/MPMI-21-8-1015
Grabowski, G. A., Golembo, M., and Shaaltiel, Y. (2014). Taliglucerase alfa: an enzyme replacement therapy using plant cell expression technology. Mol. Genet. Metab. 112, 1–8. doi: 10.1016/j.ymgme.2014.02.011
Gupta, P., and Pastores, G. M. (2017). Spotlight on taliglucerase alfa in the treatment of pediatric patients with type 1 gaucher disease. Pediatr. Health Med. Ther. 8, 73–81. doi: 10.2147/PHMT.S93634
Hanittinan, O., Oo, Y., Chaotham, C., Rattanapisit, K., Shanmugaraj, B., and Phoolcharoen, W. (2020). Expression optimization, purification and in vitro characterization of human epidermal growth factor produced in Nicotiana benthamiana. Biotechnol. Rep. 28:e00524. doi: 10.1016/j.btre.2020.e00524
He, X., Galpin, J. D., Tropak, M. B., Mahuran, D., Haselhorst, T., von Itzstein, M., et al. (2012). Production of active human glucocerebrosidase in seeds of Arabidopsis thaliana complex-glycan-deficient (cgl) plants. Glycobiology 22, 492–503. doi: 10.1093/glycob/cwr157
Hollak, C. E., vom Dahl, S., Aerts, J. M., Belmatoug, N., Bembi, B., Cohen, Y., et al. (2010). Force majeure: therapeutic measures in response to restricted supply of imiglucerase (Cerezyme) for patients with gaucher disease. Blood Cells Mol. Dis. 44, 41–47. doi: 10.1016/j.bcmd.2009.09.006
Irache, J. M., Salman, H. H., Gamazo, C., and Espuelas, S. (2008). Mannose-targeted systems for the delivery of therapeutics. Expert Opin. Drug Deliv. 5, 703–724. doi: 10.1517/17425247.5.6.703
Iskratsch, T., Braun, A., Paschinger, K., and Wilson, I. B. (2009). Specificity analysis of lectins and antibodies using remodeled glycoproteins. Anal. Biochem. 386, 133–146. doi: 10.1016/j.ab.2008.12.005
Jin, C., Altmann, F., Strasser, R., Mach, L., Schähs, M., Kunert, R., et al. (2008). A plant-derived human monoclonal antibody induces an anti-carbohydrate immune response in rabbits. Glycobiology. 18, 235–241. doi: 10.1093/glycob/cwm137
Joerg, S., Ludger Wilhelm, P., and Stephan vom, D. (2007). Therapy of adult gaucher disease. Haematologica 92, 148–152. doi: 10.3324/haematol.11193
Jung, J. W., Choi, H. Y., Huy, N. X., Park, H., Kim, H. H., Yang, M. S., et al. (2019). Production of recombinant human acid β-glucosidase with high mannose-type N-glycans in rice gnt1 mutant for potential treatment of gaucher disease. Protein Exp. Purif. 158, 81–88. doi: 10.1016/j.pep.2019.02.014
Kagale, S., Uzuhashi, S., Wigness, M., Bender, T., Yang, W., Borhan, M. H., et al. (2012). TMV-Gate vectors: gateway compatible tobacco mosaic virus based expression vectors for functional analysis of proteins. Sci. Rep. 2:874. doi: 10.1038/srep00874
Kallemeijn, W. W., Scheij, S., Hoogendoorn, S., Witte, M. D., Herrera Moro Chao, D., van Roomen, C. P., et al. (2017). Investigations on therapeutic glucocerebrosidases through paired detection with fluorescent activity-based probes. PLoS ONE 12:e0170268. doi: 10.1371/journal.pone.0170268
Keler, T., Ramakrishna, V., and Fanger, M. W. (2004). Mannose receptor-targeted vaccines. Expert Opin. Biol. Ther. 4, 1953–1962. doi: 10.1517/14712598.4.12.1953
Kim, K., Kang, Y. J., Park, S. R., Kim, D. S., Lee, S. W., Ko, K., et al. (2021). Effect of leaf position and days post-infiltration on transient expression of colorectal cancer vaccine candidate proteins GA733-Fc and GA733-FcK in Nicotiana benthamiana plant. PeerJ. 9:e10851. doi: 10.7717/peerj.10851
Leuzinger, K., Dent, M., Hurtado, J., Stahnke, J., Lai, H., Zhou, X., et al. (2013). Efficient agroinfiltration of plants for high-level transient expression of recombinant proteins. J. Vis. Exp. 77:50521. doi: 10.3791/50521
Limkul, J., Iizuka, S., Sato, Y., Misaki, R., Ohashi, T., Ohashi, T., et al. (2016). The production of human glucocerebrosidase in glyco-engineered Nicotiana benthamiana plants. Plant Biotechnol. J. 14, 1682–1694. doi: 10.1111/pbi.12529
Limkul, J., Misaki, R., Kato, K., and Fujiyama, K. (2015). The combination of plant translational enhancers and terminator increase the expression of human glucocerebrosidase in Nicotiana benthamiana plants. Plant Sci. 240, 41–49. doi: 10.1016/j.plantsci.2015.08.018
Loos, A., and Steinkellner, H. (2014). Plant glyco-biotechnology on the way to synthetic biology. Front. Plant Sci. 5:523. doi: 10.3389/fpls.2014.00523
Maupin, K. A., Liden, D., and Haab, B. B. (2012). The fine specificity of mannose-binding and galactose-binding lectins revealed using outlier motif analysis of glycan array data. Glycobiology 22, 160–169. doi: 10.1093/glycob/cwr128
Montero-Morales, L., and Steinkellner, H. (2018). Advanced plant-based glycan engineering. Front. Bioeng. Biotechnol. 6:81. doi: 10.3389/fbioe.2018.00081
Moon, K. B., Park, J. S., Park, Y. I., Song, I. J., Lee, H. J., Cho, H. S., et al. (2019). Development of systems for the production of plant-derived biopharmaceuticals. Plants 9:30. doi: 10.3390/plants9010030
Morant, A. V., Jørgensen, K., Jørgensen, C., Paquette, S. M., Sánchez-Pérez, R., Møller, B. L., et al. (2008). Beta-glucosidases as detonators of plant chemical defense. Phytochemistry 69, 1795–1813. doi: 10.1016/j.phytochem.2008.03.006
Nam, H. J., Kwon, J. Y., Choi, H. Y., Kang, S. H., Jung, H. S., and Kim, D. I. (2017). Production and purification of recombinant glucocerebrosidase in transgenic rice cell suspension cultures. Appl. Biochem. Biotechnol. 181, 1401–1415. doi: 10.1007/s12010-016-2292-4
Naphatsamon, U., Ohashi, T., Misaki, R., and Fujiyama, K. (2018). The production of human β-glucocerebrosidase in Nicotiana benthamiana root culture. Int. J. Mol. Sci. 19:1972. doi: 10.3390/ijms19071972
Pankoke, H., Buschmann, T., and Müller, C. (2013). Role of plant β-glucosidases in the dual defense system of iridoid glycosides and their hydrolyzing enzymes in Plantago lanceolata and Plantago major. Phytochemistry 94, 99–107. doi: 10.1016/j.phytochem.2013.04.016
Paschinger, K., Rendić, D., and Wilson, I. B. (2009). Revealing the anti-HRP epitope in Drosophila and Caenorhabditis. Glycoconj. J. 26, 385–395. doi: 10.1007/s10719-008-9155-3
Paul, M., and Ma, J. K. (2011). Plant-made pharmaceuticals: leading products and production platforms. Biotechnol. Appl. Biochem. 58, 58–67. doi: 10.1002/bab.6
Schoberer, J., and Strasser, R. (2018). Plant glyco-biotechnology. Semin. Cell Dev. Biol. 80, 133–141. doi: 10.1016/j.semcdb.2017.07.005
Seshadri, S., Akiyama, T., Opassiri, R., Kuaprasert, B., and Cairns, J. K. (2009). Structural and enzymatic characterization of Os3BGlu6, a rice beta-glucosidase hydrolyzing hydrophobic glycosides and (1->3)- and (1->2)-linked disaccharides. Plant Physiol. 151, 47–58. doi: 10.1104/pp.109.139436
Shanmugaraj, B. I., Bulaon, C. J., and Phoolcharoen, W. (2020). Plant molecular farming: a viable platform for recombinant biopharmaceutical production. Plants 9:842. doi: 10.3390/plants9070842
Siebert, M., Sidransky, E., and Westbroek, W. (2014). Glucocerebrosidase is shaking up the synucleinopathies. Brain 137, 1304–1322. doi: 10.1093/brain/awu002
Stirnemann, J., Belmatoug, N., Camou, F., Serratrice, C., Froissart, R., Caillaud, C., et al. (2017). A review of gaucher disease pathophysiology, clinical presentation and treatments. Int. J. Mol. Sci. 18:441. doi: 10.3390/ijms18020441
Strasser, R. (2014). Biological significance of complex N-glycans in plants and their impact on plant physiology. Front. Plant Sci. 5:363. doi: 10.3389/fpls.2014.00363
Tekoah, Y., Tzaban, S., Kizhner, T., Hainrichson, M., Gantman, A., Golembo, M., et al. (2013). Glycosylation and functionality of recombinant β-glucocerebrosidase from various production systems. Biosci. Rep. 33:e00071. doi: 10.1042/BSR20130081
Tschofen, M., Knopp, D., Hood, E., and Stöger, E. (2016). Plant molecular farming: much more than medicines. Annu. Rev. Anal. Chem. 9, 271–294. doi: 10.1146/annurev-anchem-071015-041706
Van Dussen, L., Zimran, A., Akkerman, E. M., Aerts, J. M., Petakov, M., Elstein, D., et al. (2013). Taliglucerase alfa leads to favorable bone marrow responses in patients with type I gaucher disease. Blood Cells Mol. Dis. 50, 206–211. doi: 10.1016/j.bcmd.2012.11.001
Van Patten, S. M., Hughes, H., Huff, M. R., Piepenhagen, P. A., Waire, J., Qiu, H., et al. (2007). Effect of mannose chain length on targeting of glucocerebrosidase for enzyme replacement therapy of gaucher disease. Glycobiology 17, 467–478. doi: 10.1093/glycob/cwm008
Yan, R., Wang, Z., Ren, Y., Li, H., Liu, N., and Sun, H. (2019). Establishment of efficient genetic transformation systems and application of CRISPR/Cas9 genome editing technology in Lilium pumilum DC. Fisch. and Lilium longiflorum white heaven. Int. J. Mol. Sci. 20:2920. doi: 10.3390/ijms20122920
Zacchi, L. F., and Schulz, B. L. (2016). N-glycoprotein macroheterogeneity: biological implications and proteomic characterization. Glycoconj. J. 33, 359–376. doi: 10.1007/s10719-015-9641-3
Zhao, Y., Ren, J., Padilla-Parra, S., Fry, E. E., and Stuart, D. I. (2014). Lysosome sorting of β-glucocerebrosidase by LIMP-2 is targeted by the mannose 6-phosphate receptor. Nat. Commun. 5:4321. doi: 10.1038/ncomms5321
Zhou, Q., and Qiu, H. (2019). The mechanistic impact of N-glycosylation on stability, pharmacokinetics, and immunogenicity of therapeutic proteins. J. Pharm. Sci. 108, 1366–1377. doi: 10.1016/j.xphs.2018.11.029
Keywords: β-glucocerebrosidase, transient expression, Nicotiana benthamiana, N-glycosylation, plant-made pharmaceuticals, N-acetylglucosaminyltransferase I
Citation: Uthailak N, Kajiura H, Misaki R and Fujiyama K (2021) Transient Production of Human β-Glucocerebrosidase With Mannosidic-Type N-Glycan Structure in Glycoengineered Nicotiana benthamiana Plants. Front. Plant Sci. 12:683762. doi: 10.3389/fpls.2021.683762
Received: 22 March 2021; Accepted: 07 May 2021;
Published: 07 June 2021.
Edited by:
Ryo Matsuda, The University of Tokyo, JapanReviewed by:
Richard Strasser, University of Natural Resources and Life Sciences Vienna, AustriaCopyright © 2021 Uthailak, Kajiura, Misaki and Fujiyama. This is an open-access article distributed under the terms of the Creative Commons Attribution License (CC BY). The use, distribution or reproduction in other forums is permitted, provided the original author(s) and the copyright owner(s) are credited and that the original publication in this journal is cited, in accordance with accepted academic practice. No use, distribution or reproduction is permitted which does not comply with these terms.
*Correspondence: Kazuhito Fujiyama, ZnVqaXlhbWFAaWNiLm9zYWthLXUuYWMuanA=
Disclaimer: All claims expressed in this article are solely those of the authors and do not necessarily represent those of their affiliated organizations, or those of the publisher, the editors and the reviewers. Any product that may be evaluated in this article or claim that may be made by its manufacturer is not guaranteed or endorsed by the publisher.
Research integrity at Frontiers
Learn more about the work of our research integrity team to safeguard the quality of each article we publish.