- Key Laboratory of Crop Physiology, Ecology and Genetic Breeding, Ministry of Education, College of Agronomy, Jiangxi Agricultural University, Nanchang, China
The panicle apical abortion (PAA) causes severe yield losses in rice production, but details about its development and molecular basis remain elusive. Here, we detected PAA quantitative trait loci (QTLs) in three environments using a set of chromosome segment substitution lines (CSSLs) that was constructed with indica Changhui121 as the recurrent parent and japonica Koshihikari as the donor parent. First, we identified a novel major effector quantitative trait locus, qPAA7, and selected a severe PAA line, CSSL176, which had the highest PAA rate among CSSLs having Koshihikari segments at this locus. Next, an F2 population was constructed from a cross between CSS176 and CH121. Using F2 to make recombinantion analysis, qPAA7 was mapped to an 73.8-kb interval in chromosome 7. Among nine candidate genes within this interval, there isn’t any known genes affecting PAA. According to the gene annotation, gene expression profile and alignment of genomic DNA, LOC_Os07g41220 and LOC_Os07g41280 were predicted as putative candidate genes of qPAA7. Our study provides a foundation for cloning and functional characterization of the target gene from this locus.
Introduction
Rice is the staple food of half of the world’s population. A high, stable yield of rice has always been one of the most important goals pursued by breeders. The rice yield is mainly determined by the number of panicles per unit area, grain number per panicle and 1,000-grain weight (Xing and Zhang, 2010). Increasing the grain number per panicle is a prior goal of many rice breeders in high-yield breeding. The panicle architecture, which is characterized by its size and branching pattern, determines the number of spikelets, and then number of grains per panicle (Sakamoto and Matsuoka, 2004). Large panicle with more branches and spikelets have been preferred in breeding programs for new rice types with higher yield. Thus, understanding the molecular genetic mechanisms of panicle development and identification of superior alleles for large panicles are of great interest to both plant biologists and plant breeders.
Rice panicles are composed of main axis, primary branches, secondary branches and spikelets (Itoh et al., 2005). In rice, panicle development can be roughly divided into two main stages. First, when vegetative meristems changed to the reproductive meristems, the shoot apical meristem (SAM) transformed into an inflorescence meristem (IM), which further initiates the primary branch meristems (BMs) and forms the main axis of the inflorescence. Subsequently, the primary BMs produce secondary BMs and spikelet meristems (SMs). SMs are also initiated from the secondary BMs and finally form spikelets (Komatsu K. et al., 2003; Ikeda et al., 2004). In most of the studies, spikelet degeneration has been reported during the panicle elongation stage (Ali et al., 2019). For example, invasion of any stress during meiosis, could block the development of floral organs that results in spikelet degeneration. In a practical sense, early stage degeneration of spikelets is a detrimental factor for the final yield, which could result in 3–50% yield loss in a single panicle (Yamagishi et al., 2004; Cheng et al., 2011; Li et al., 2018).
Over the past two decades, a number of genes regulating panicle development have been identified and functionally characterized (Vollbrecht et al., 2005; Teo et al., 2014; Zuo and Li, 2014). For example, LAX PANICLE1 (Lax1) (Komatsu K. et al., 2003; Tetsuo and Junko, 2009); Lax2 (Tabuchi et al., 2011) and MONOCULM1 (MOC1) (Li et al., 2003) are important regulatory factors in axillary meristem formation in rice. LAX PANICLE (LAX) gene controls early developmental switches involved in the initiation of axillary meristems and branch formation in rice (Komatsu K. et al., 2003). ABERRANT PANICLE ORGANIZATION 1 (APO1) (Ikeda et al., 2005, 2007), LEAFY (RFL)/APO2 (Rao et al., 2008) and FRIZZY PANICLE (FZP) (Komatsu M. et al., 2003; Zhu et al., 2003) are involved in maintaining the identity of primary branch meristems by preventing precocious conversion of primary branch meristems to spikelet meristems. In addition, GRAIN-NUMBER1 (Gn1a) is a gene for cytokinin oxidase/dehydrogenase (OsCKX2), an enzyme that degrades the phytohormone cytokinin. Reduced expression of OsCKX2 causes cytokinin accumulation in inflorescence meristems and increases the number of reproductive organs, resulting in enhanced grain yield (Ashikari et al., 2005). These genes mainly play a role in the initiation and transformation stages of panicle development. Elucidating the functions of these genes and their regulatory relationships will contribute to breeding new elite rice cultivars with “ideal” plant architecture and higher grain yield.
During the growth and development of rice, panicle abortion frequently occurs at either the top or basal parts of the panicle, especially under adverse climatic conditions (Yao et al., 2000; Tan et al., 2011). Panicle apical abortion (PAA) is very unfavorable for the formation of large panicles. PAA quantitative trait loci (QTLs) have been detected on chromosomes 1–11 (Xu et al., 2007; Cheng et al., 2011; Tan et al., 2011; Wang et al., 2011; Zhang et al., 2017). However, only a few of QTLs have been fine mapped. For example, qPAA8 was fine mapped between RM22476-8-IN112 markers on chromosome 8 in an interval of approximately 37-kb (Cheng et al., 2011), and qPAA3 was fine mapped between M6929-RM1319 markers on chromosome 3 in an interval of approximately 97.3-kb (Zhang X.Y. et al., 2015). So far, QTL of PAA has not been successfully cloned. In recent years, several PAA genes have been cloned using the PAA mutants of rice (Bai et al., 2015; Heng et al., 2018; Peng et al., 2018; Wang et al., 2018; Zafar et al., 2020). For example, Mutation in TUTOU1, which encodes a SCAR-like protein modulating actin organization, causes a pleiotropic phenotype including panicle apical abortion (Bai et al., 2015). OsALMT7 is reported as a malate transporter that plays its role in the development of panicle apical portions (Heng et al., 2018). The function of OsCIPK31 is disrupted due to excessive accumulation of reactive oxygen species (ROS), leading to cell death in rice panicle (Peng et al., 2018). SQUAMOSA PROMOTERBINDING PROTEIN-LIKE 6 (SPL6) represses signaling outputs of endoplasmic reticulum (ER) stress in control of panicle cell death in rice (Wang et al., 2018). DEGENERATED PANICLE AND PARTIAL STERILITY 1 (DPS1) encodes a cystathionine β-synthase domain containing protein that plays a vital role in regulating ROS homeostasis, anther cuticle formation, and panicle development in rice (Zafar et al., 2020). Despite this progress, the molecular and genetic mechanisms underlying PAA are still poorly understood.
In this study, a set of 132 CSSLs was used to detect the QTLs of PAA under three environments, aiming to identify a novel major QTLs/genes of PAA and clarify the mechanism of PAA genetic regulation, which provides a theoretical basis for breeding high-yielding rice varieties.
Materials and Methods
Plant Materials and Planting Conditions
A set of CSSLs was constructed by using Changhui 121 (CH121) and Koshihikari as parental lines that included 132 lines at BC3F8, BC4F7 or BC5F6. The recurrent parent CH121 is an elite rice restorer line breeding developed by our laboratory (He et al., 2008). The donor parent Koshihikari is a Japanese japonica variety. To fine map the major locus qPAA7, a severe PAA line, CSSL176, which harbors the qPAA7 allele, was selected for backcrossing with the recurrent CH121 parent. Derived F2 CSSL176/CH121 populations were subsequently constructed by self-pollination.
The test environments for the two parents and 132 CSSLs are shown in Table 1. Each family was represented by three rows of ten plants that were planted in a randomized block design. CH121, CSSL176 and F2 were planted as middle-season rice and late-season rice in Nanchang, Jiangxi (N 28.45°, E 115.50°) in 2017 and 2018, respectively, and CH121, CSSL176 and F2 were planted in Sanya, Hainan (N 18.14°, E 109.31°) in 2019, with a spacing of 17 × 22 cm. Crop management and the control of diseases and insect pests were performed as locally recommended.
Phenotypic Evaluation
At the rice heading stage, the aborted spikelets rate was investigated to CSSL176/CH121 F2 individuals, and five plants that were randomly selected from the parents and 132 CSSLs, respectively. The aborted spikelets rate was calculated according to the following equation: aborted spikelets rate (%) = (PAA spikelet number/total spikelet number)∗100.
To investigate the occurring time of PAA during panicle development, we divided the developmental course of panicles into eight stages according to the panicle length (∼1, 4, 7, 10, 13, 16, 20, and 24 cm).
Marker Analysis
Genomic DNA was extracted from fresh leaves by using CTAB method (Rogers and Bendich, 1985). The PCR reaction mixture (total volume of 10 μL) (Panaud et al., 1996), contained 1 μL 10 × buffer (Mg2+ Plus), 0.2 μL dNTPs, 0.5 μL forward primer (10 μmol/L), 0.5 μL reverse primer (10 μmol/L), 2 μL DNA, 5.7 μL ddH2O2 and 0.1 μL Taq polymerase (5 U/μL). The reaction cycles were as follows: One initial step (94°C, 5 min), 34 cycles (94°C, 30 s; 55–58°C, 30 s; 72°C, 30 s), a final extension step (72°C, 10 min) and followed by storage at 4°C. The PCR products were subjected to electrophoresis on 8% polyacrylamide gel and silver staining for visualization.
The simple sequence repeat (SSR) primers and sequence-tagged site (STS) were designed according to sequences from the Gramene database1. The insertion/deletion (InDel) markers were designed using Primer 5.0 according to the CH121 and CSSL176 resequencing data.
Construction of Genetic Linkage Map
According to the genotype of 132 CSSLs using 125 SSR and 17 STS markers, the original data set was constructed. “1” indicates Koshihikari genotype; “2” represents CH121 genotype; “H” indicates heterozygote; “0” denotes an unknown genotype (Supplementary Figure 1). The linkage map was constructed by MapMaker/EXP 3.0 (Lander et al., 1987) software with the default parameter. The main procedures were as follows: First, the linkage groups were calculated through two-point method, and “GROUP” was used to infer the optimal linkage groups. Second, the framework structure of genetic linkage map was constructed using multi-point analysis, and “COMPARE” was used to sorted SSR/STS markers. The recombination frequencies were converted into map distances (cM) using the function of Kosambi (1944). Finally, according to the map distances among markers, Mapchart 2.1 (Voorrips, 2002) was used to draw the genetic linkage map.
qRT-PCR Analysis
Total RNA was extracted using the TaKaRa MiniBEST Universal RNA Extraction Kit (TaKaRa, China). qRT-PCR was carried out using an ABI7500 fast real-time PCR system with the SYBR Premix Ex Taq (TaKaRa; RR041A), following the manufacturer’s instructions. The OsActin gene was used as an internal control. The gene expression differences were estimated using the 2–Δ Δ Ct method (Livak and Schmittgen, 2001). Three biological and three technical repeats were performed in the experiments. The information of primers used in the qRT-PCR analysis is listed in Supplementary Table 1.
Data Analysis and QTL Mapping
QTL analysis was performed using the IciMapping 4.1 software (Wang et al., 2006; Meng et al., 2015), and a logarithm-of-odds (LOD) score of 2.5 was chosen as a threshold for determining QTLs of the traits. Data analysis was performed using the one-way analysis of variance (ANOVA) module within Statistical Package for Social Sciences 17.0 (SPSS 17.0). Statistical significance was set at an alpha level of P < 0.05.
Results
Aborted Spikelets Rate of Parents and CSSL
The aborted spikelets rate of 132 CSSLs were investigated in three environments. Relative to the recurrent parent CH121, 8 CSSLs show the aborted spikelets at the apical portion of panicle under three environments, but the other 124 CSSLs did not show aborted spikelets. The aborted spikelets rates of 8 CSSLs were significantly higher than CH121 (Table 2). Comparing with the others lines, CSSL176 had the highest aborted spikelets rates, i.e., 9.05, 14.65 and 18.85% in three environments, with a mean aborted spikelets rate of 14.18% (Figure 1).
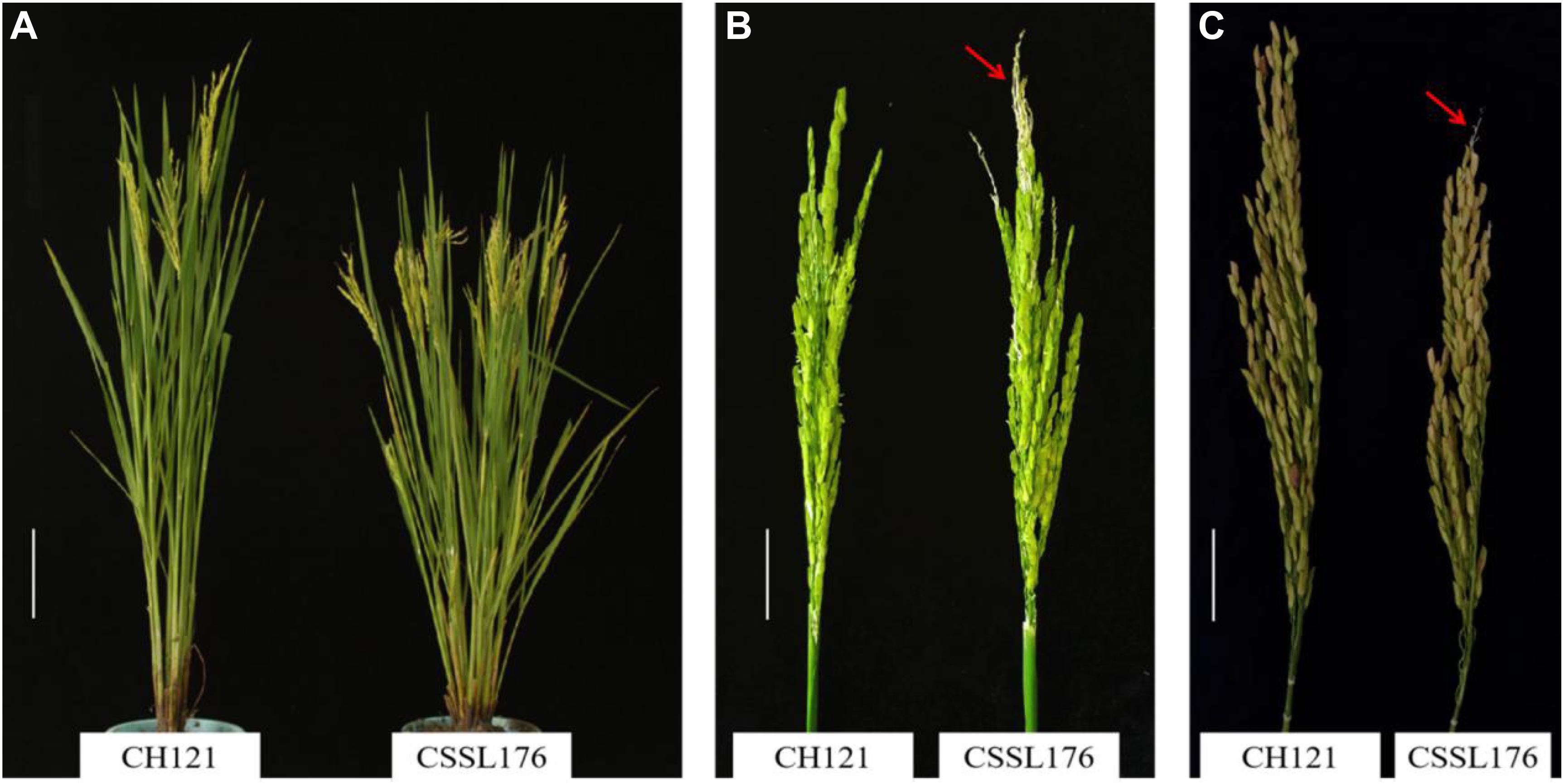
Figure 1. Phenotypes of the CSSL176 and CH121. (A) Plants at the heading stage, Bar = 16 cm. (B) Panicles at the heading stage. Bar = 4 cm. (C) Panicles at maturity period, Bar = 3 cm. The arrows show the PAA in CSSL176.
PAA QTL Detection
In previously study, a set of CSSLs was constructed in our laboratory (Wang et al., 2020b). To identify genes controlling PAA, we conducted a QTL analysis for PAA using 132 CSSLs population. Genotyping was performed using 142 molecular markers, including 125 SSR markers and 17 STS markers. The linkage map of SSR/STS markers was shown in Figure 2.
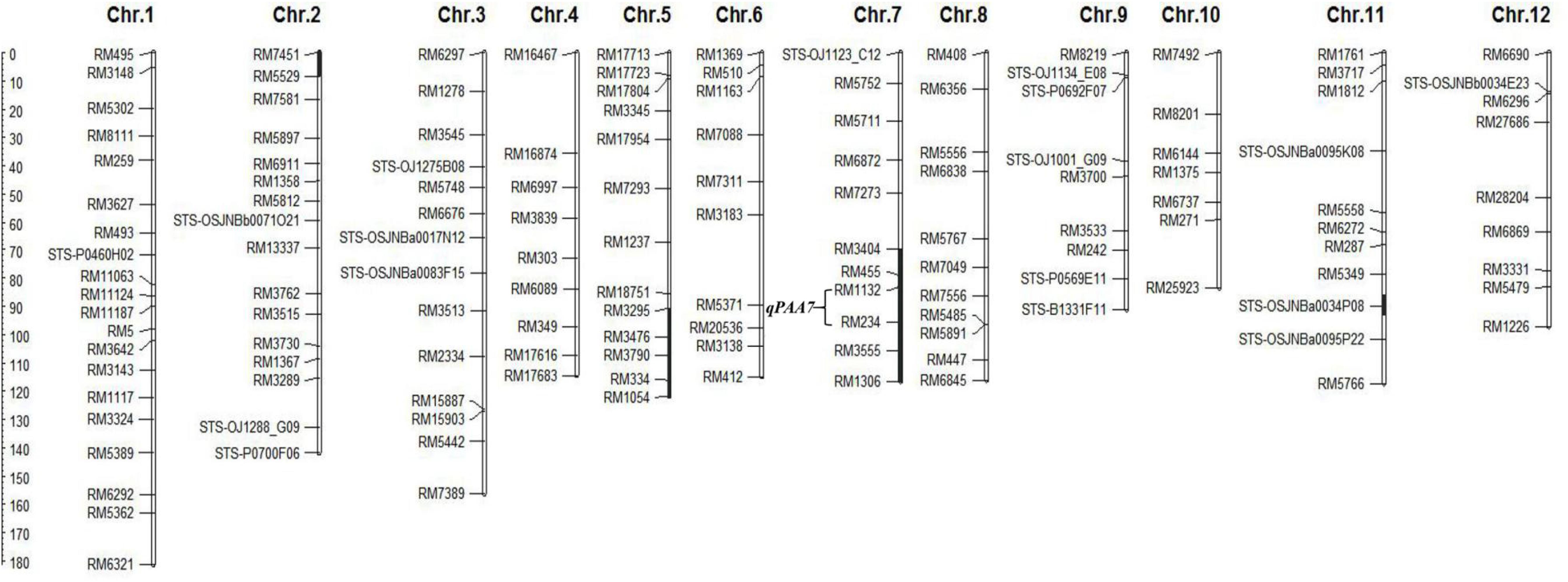
Figure 2. Linkage map of CSSL176. The black bars represent the Koshihikari chromosomal segments in CSSL176. The scale of the ruler indicates the genetic distance, the unit “cM”.
In total, ten QTLs of PAA were identified using 132 CSSLs under three environments, and mapped to seven chromosomes (Table 3). Among them, qPAA3, qPAA4.1 and qPAA7 were detected in all three environments, with an average phenotypic variation explained (PVE) of 4.26, 6.85 and 26.96%, respectively. The others QTL, qPAA1.1, qPAA1.2, qPAA4.2, qPAA5.1, qPAA5.2, qPAA9, and qPAA11 were detected in one or two environments, with PVE of 1.38–12.52%. PVE of qPAA7 is relatively large, with the positive allele from Koshihikari.
Further Localization of qPAA7
As mentioned above, qPAA7 was detected between markers RM1132-RM234 in three environments. Among 132 CSSLs, CSSL15, CSSL176, CSSL205, CSSL207 and CSSL208 lines harbors qPAA7 and showed aborted spikelets at the apical portion of panicle at the heading stage (Table 4). CSSL176 was selected according to its graphical genotype and phenotypic performance, then backcrossed with CH121. A segregating F2 population was constructed to identify the existence of qPAA7. Four substituted fragments from the donor parent Koshihikari, distributed on chromosomes 2, 5, 7, and 11, covered by 16 SSR markers (Figure 2).
We surveyed the aborted spikelets rates of different F1 and F2 populations as middle-season rice in Nanchang, Jiangxi, in 2017 and as late-season rice in Nanchang, Jiangxi, in 2018. The results indicated that F1 showed the phenotype of PAA, and F2 populations presented a skewed distribution (Figure 3). However, the individual-plant aborted spikelets rate of F2 was between those of CSSL176 and CH121. We speculated that the trait of PAA was controlled by a semidominant gene. Next, thirty plants were selected from F2 in 2017 middle-season rice and 2018 late-season rice, respectively, to generate high-PAA and non-PAA bulks, each consisting of fifteen plants. The high-PAA and non-PAA bulks were amplificated with the 16 SSR molecular markers that came from the donor parent Koshihikari and distributed on chromosomes 2, 5, 7 and 11. It was found that 6 SSR molecular markers were polymorphic at the end of chromosome 7 (RM3404, RM455, RM1132, RM234, RM3555, and RM1306).
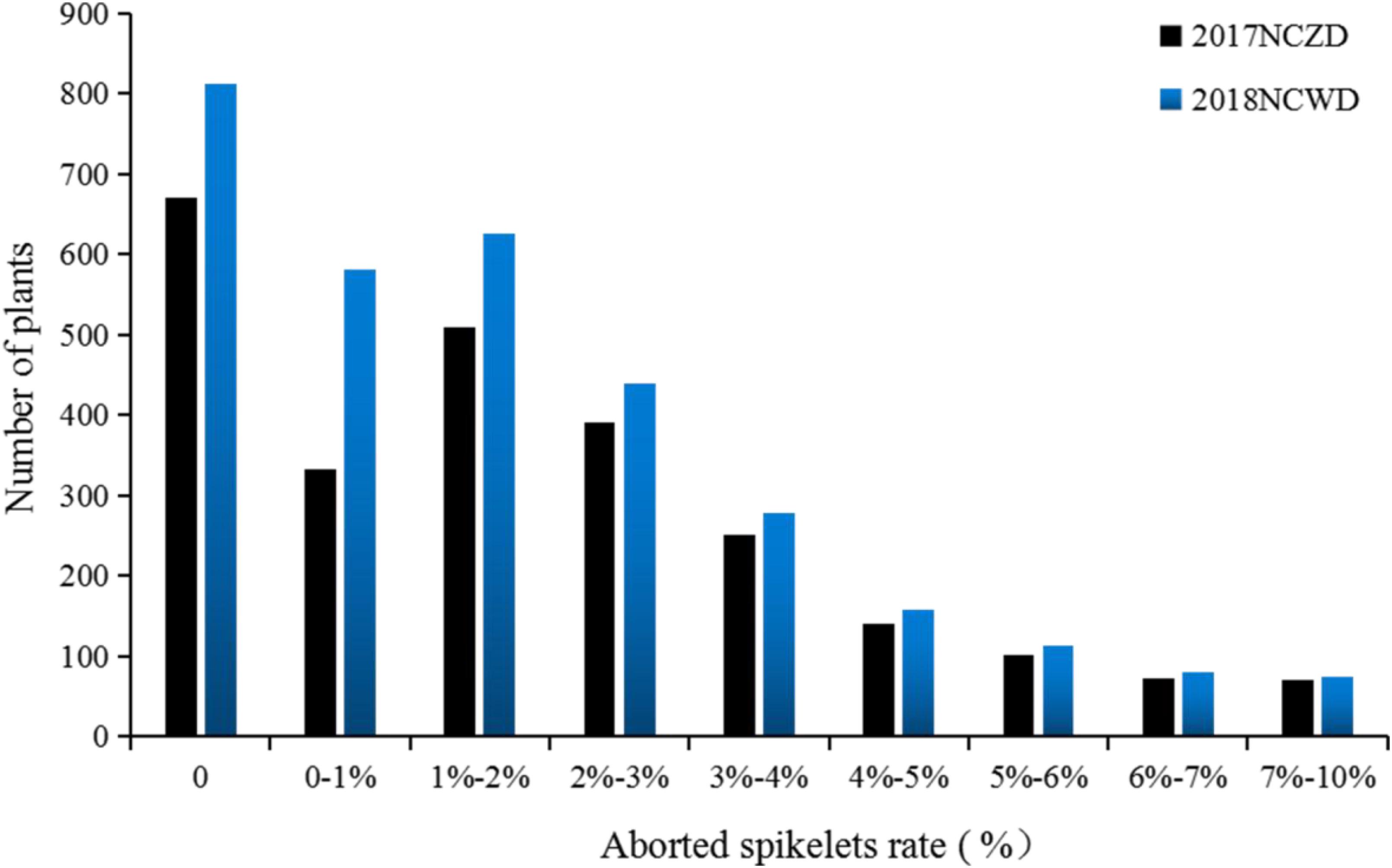
Figure 3. The severity of panicle apical abortion (PAA) distribution of F2 plants from the cross of CSSL176 × CH121. 2017NCZD and 2018 NCWD represent the middle-season rice in Nanchang, Jiangxi in 2017 and the late-season rice in Nanchang, Jiangxi in 2018, respectively.
Fine Mapping of qPAA7
The CSSL176/CH121 backcrossed generations were applied to finely map qPAA7. For the first round of fine mapping. Nine recombinants were identified from 300 F2 individuals using six markers (RM3404, RM455, RM1132, RM234, RM3555 and RM1306), which were located in the target region and were polymorphic between “CH121” and “CSSL176.” Nine recombinants were divided into six genotypes (I–VI), and among them, recombinants I, II, and V confirmed the right border of the region of interest, whereas recombinants III, IV, and VI confirmed the left border (Figure 4A). The genotypic and phenotypic analysis of the recombinants narrowed the qPAA7 region to the segment between markers RM1132 and RM234, which corresponded to a physical distance of 1.48 Mb.
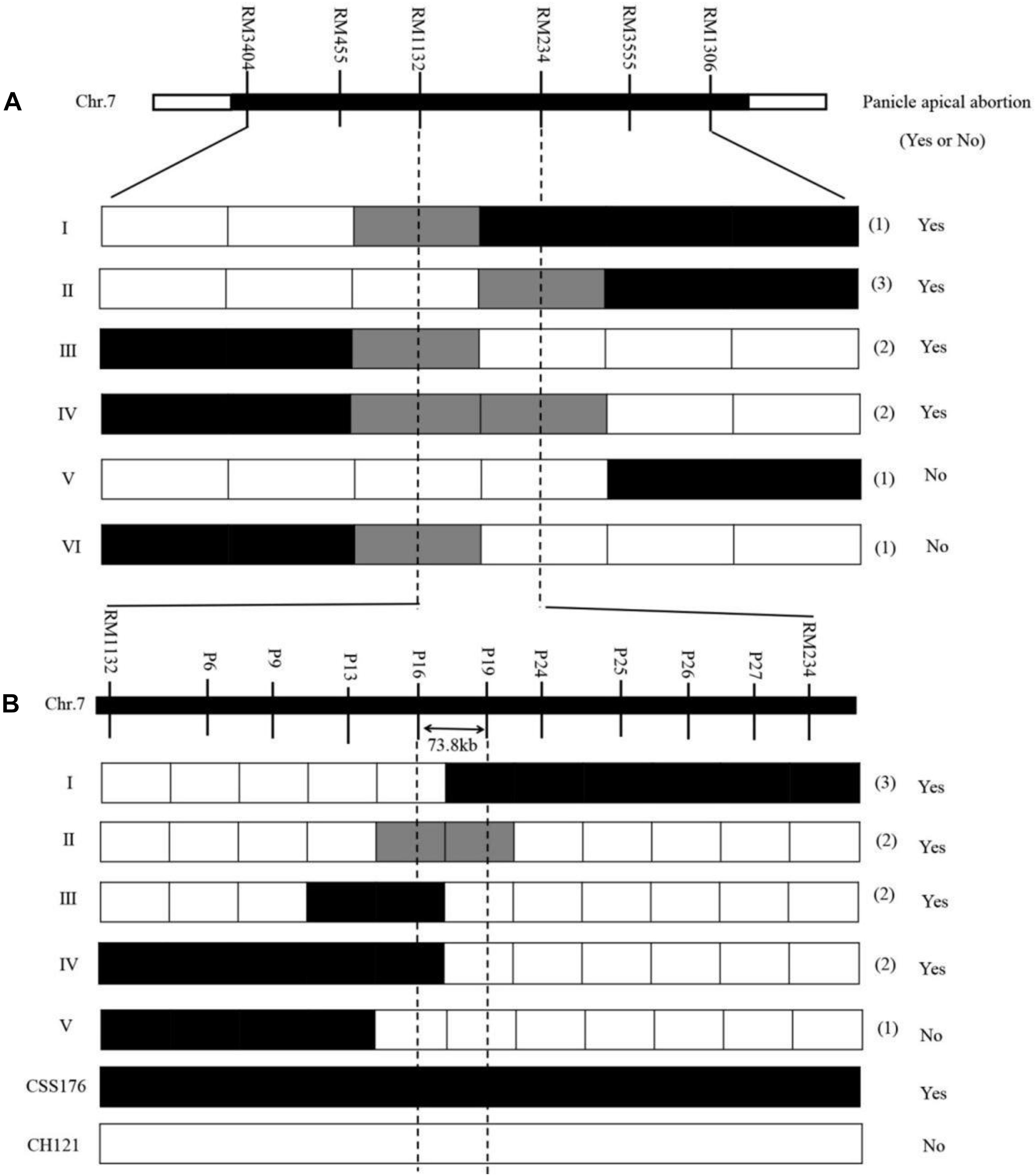
Figure 4. Fine mapping of qPAA7. (A) Linkage analysis of phenotypes and marker genotypes between RM1132 and RM234. (B) Linkage analysis of phenotypes and marker genotypes between P16 and P19. Panicle apical abortion (Yes or No), represents the panicle apical abortion, and no panicle apical abortion, respectively.
For the second round of fine mapping. The genotypes of the 3152 F2 individuals were analyzed by using the polymorphism markers RM1132 and RM234, and 24 residual heterozygous lines (RHLs) were found. The selfing of these RHLs generated a new segregating F2 population (from the heterozygote F1 for the target chromosomal segment) that contained 2100 individuals, which were evaluated for qPAA7 and rescreened using nine polymorphisms InDel markers. The InDel markers were located within the target region (Supplementary Table 2). Based on the genotypic and phenotypic analysis, the qPAA7 region was narrowed to the segment flanked by markers P11 and P19 (Figure 4B). This new region corresponded to a 73.8-kb segment in the “Nipponbare” reference genome.
Candidate Gene Analysis
According to the Nipponbare genome annotation2. Nine genes were annotated within the 73.8-kb region of qPAA7 (Table 5), including five cloned genes (LOC_Os07g41200, LOC_Os07g41210, LOC_Os07g41230, LOC_Os07g41240 and LOC_Os07g41250). Among them, LOC_Os07g41200 encodes a TONNEAU1-recruiting motif protein, which regulates longitudinal cell elongation (Wang S.K. et al., 2015). LOC_Os07g41210 is a negative regulator of GL7 (Wang Y.X. et al., 2015). LOC_Os07g41230, which is annotated as methyl esterase-like, affects reactive oxygen species (ROS) accumulation by interacting with thioredoxin OsTrxm in rice (Hu et al., 2021). LOC_Os07g41240 encodes CYP78A13, which regulates cell size in the rice grain embryo (Nagasawa et al., 2013; Yang et al., 2013; Xu et al., 2015). LOC_Os07g41250 annotated as nitrate and di/tripeptide transporter (NPF) gene family. Studies have shown that the nitrate and di/tripeptide transporter (NPF) have diverse substrates and underlie many biological processes in plants (Ouyang et al., 2010; Léran et al., 2014).
We observed the spikelets of rice at eight stages according to the panicle length (∼1, 4, 7, 10, 13, 16, 20, and 24 cm) and found that the period of 4–10 cm panicle length is critical for PAA. Therefore, we analyzed the expression levels of the nine candidate genes between CH121 and CSSL176 in samples of 4, 7, and 10 cm panicles. qRT-PCR data analysis indicated that LOC_Os07g41200, LOC_Os07g41210, LOC_Os07g41230, LOC_Os07g41240, LOC_Os07g41250, LOC_Os07g41260, and LOC_Os07g41270 showed no significant difference between CH121 and CSSL176. LOC_Os07g41220 showed a significant difference between CH121 and CSSL176 in 4 and 7 cm panicles, but no significant difference between CH121 and CSSL176 in 10 cm panicles. LOC_Os07g41280 showed significant differences between CH121 and CSSL176 in 4, 7, and 10 cm panicles (Figure 5). To further obtain strong evidence to determine the most promising candidate gene, the genomic DNA sequences of the LOC_Os07g41220 and LOC_Os07g41280 were amplified and compared between CH121 and CSSL176. We found that LOC_Os07g41220 and LOC_Os07g41280 have different sequences in the CDS region and promoter region, respectively. Among them, LOC_Os07g41220 had one SNP (C deletion) in the CDS region in CSSL176 compared with CH121 (421 bp downstream from the start codon ATG). LOC_Os07g41280 had two deletions (9–10 and 70–81 bp upstream from the start codon ATG), together two two-base substitutions at 64–65 and 67–68 bp upstream from the start codon ATG) in the promoter region (Figure 6).
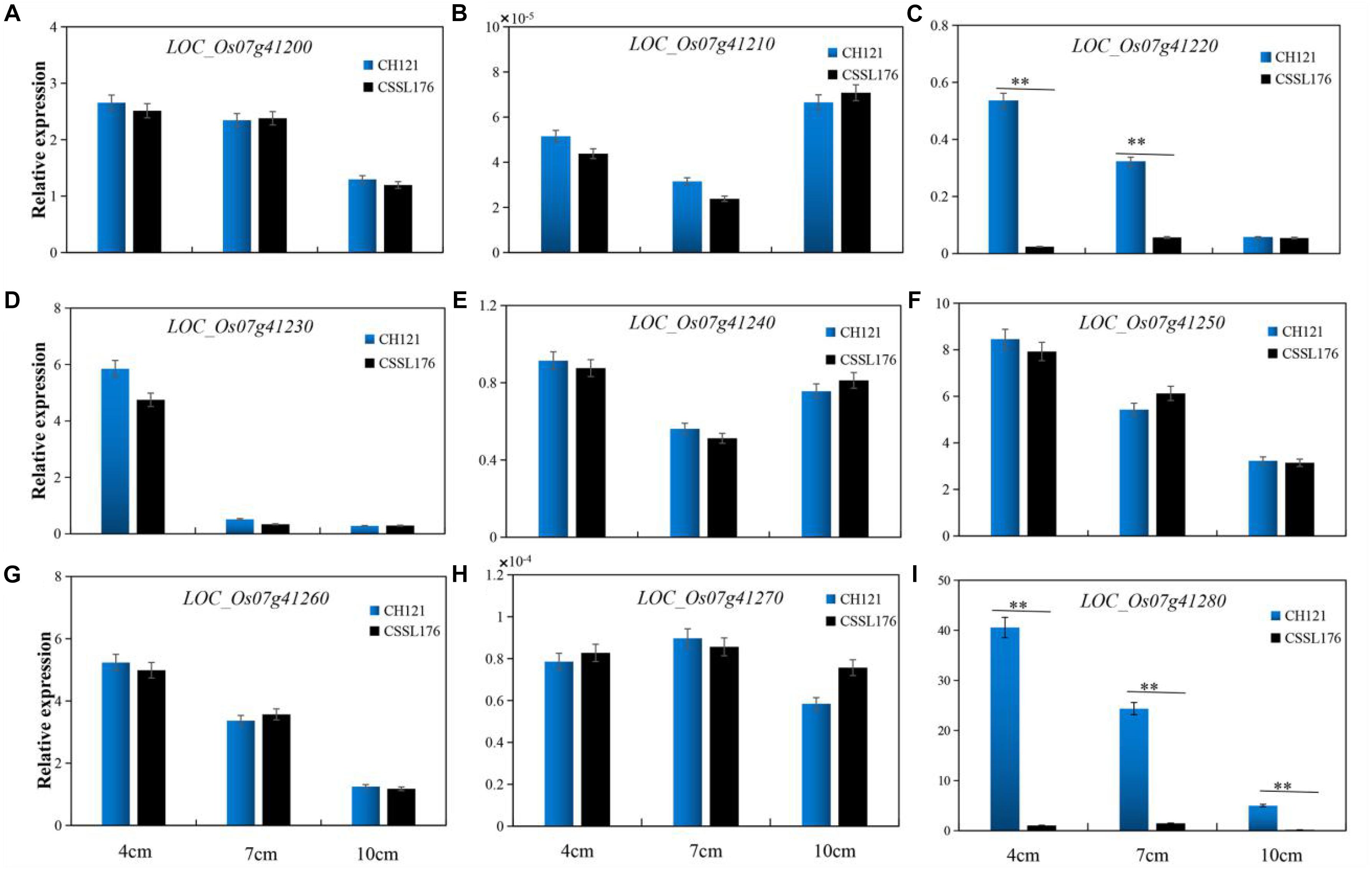
Figure 5. The expression levels of (A) LOC_Os07g41200, (B) LOC_Os07g41210, (C) LOC_Os07g41220, (D) LOC_Os07g41230, (E) LOC_Os07g41240, (F) LOC_Os07g41250, (G) LOC_Os07g41260, (H) LOC_Os07g41270, (I) LOC_Os07g41280 between CH121 and CSSL176 in 4 cm, 7 cm, and 10 cm panicles, respectively.
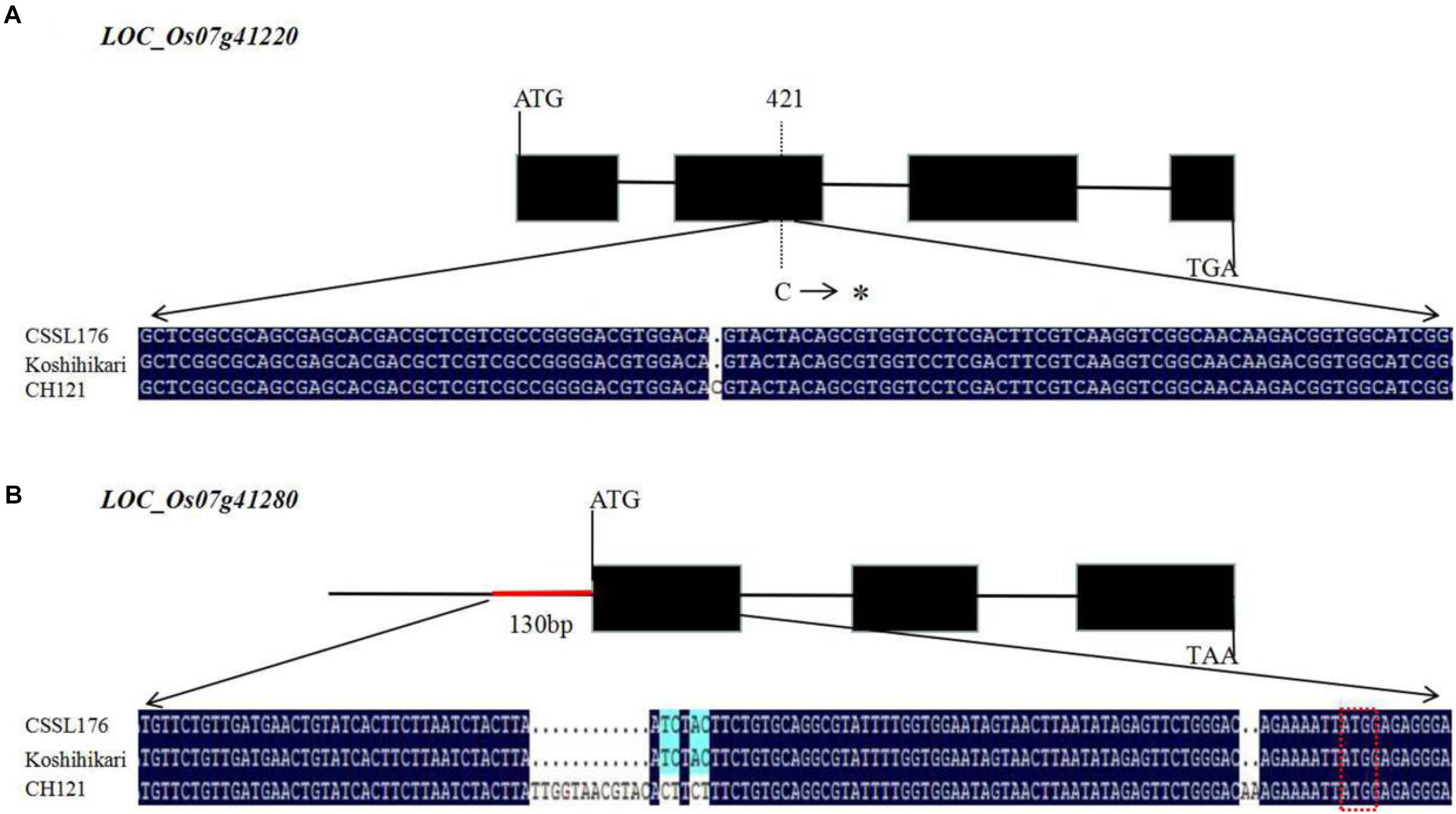
Figure 6. Structure and sequence alignment of candidate genes. (A) Gene structure of LOC_Os07g41220 and sequence differences in LOC_Os07g41220 between CH121 and CSSL176 (B) Gene structure of LOC_Os07g41280 and sequence differences in LOC_Os07g41280 between CH121 and CSSL176. The three base sequences in the red box are the start codon. The symbol * means the C deletion.
Discussion
Increasing the number of grains per panicle and cultivating large-panicle-type rice are important ways to further increase rice yield. Normal panicle development is the basis of the large panicle formation. PAA often occurs in agricultural production, reducing the total spikelet number per panicle, and finally causing great yield loss. The aborted spikelets rates of some rice varieties can reach 50–60% under extreme weather conditions (Wang et al., 2017; Ali et al., 2019). Elucidating the genetic mechanism of PAA is helpful for preventing yield loss due to PAA in rice and for cultivating new rice varieties with “ideal” plant architectures and high yields. However, the genetic and molecular mechanisms of PAA are still unclear. Indica and Japonica are two subspecies of cultivated rice in Asia. Because hybrids between indica and japonica show strong heterosis, they were widely used in super-high yield rice breeding. Indica/japonica hybrids have shown prominent heterosis in rice panicle characteristics, such as many rachis branches, relatively stout stems and a large number of grains per panicle. Due to large genetic differentiation between Indica and Japonica subspecies, PAA often occurs in indica/japonica hybrid progeny (Yamagishi et al., 2004; Tan et al., 2011). In this study, a set of CSSLs were constructed from Changhui 121 (non-PAA) and Koshihikari (non-PAA), including BC3F8, BC4F7, and BC5F6. Among 132 CSSLs, eight CSSLs showed obvious PAA under three environments. The same phenomenon has been observed in another set of inter-subspecific CSSLs constructed by our laboratory, we speculated that the gene-gene interaction between subspecies lead to the phenomenon of PAA, which has been reported in other traits of rice (Zeng et al., 2009; Sun et al., 2010).
Genetic factors are the direct cause of PAA in rice, while environmental conditions such as temperature, humidity and N nutrition levels in panicle primordium differentiation stage have a great influence on PAA. In this study, we found that the aborted spikelets rate of the 8 CSSLs in winter in Sanya, Hainan, was obviously higher than that in middle-season and late-season in Nanchang, Jiangxi (Table 2), being consistent with Tan et al. (2011). Considering the differences in localities and seasons during panicle primordium differentiation stage, especially the differences in temperature. The results of variance analysis showed that line and experimental environment accounted for 83.86 and 5.76% of the total variation, respectively, and line-environment interaction accounted for 10.61% of the total variation, all of them reached an extremely significant level (Supplementary Table 3). It is shown that the phenotypic differences between parents and CSSLs under the three environments are related to genotype-environment interactions. Low temperature during panicle development stage should be noticed as one of the important unfavorable factors. When the CSSL176 was planted in the winter of Sanya, Hainan, the panicle primordium differentiation stage is from late February to early March. We found that the daily average temperature of February 22nd–23th and March 7th–10th were below 23°C (Supplementary Figure 2), this possibly influences the panicle development of CSSL176 and leads to an increase in the aborted spikelets rate.
PAA have a complex genetic background, and greatly affected by the environment. Therefore, it is difficult to fine map QTLs using the common population types such as F2, recombinant inbred lines (RILs) and backcross populations (Qiao et al., 2016). However, CSSL or near-isogenic lines (NILs) from interspecific hybridization are useful for QTL mapping (Wang et al., 2020a) and marker-assisted breeding (Yang et al., 2010). In addition, secondary F2 and F3 groups can be derived from further backcrossing of selected CSSLs/NILs with the recurrent parent and can be used for the fine mapping and cloning of QTLs (Hu et al., 2018; Zhang et al., 2020). In this study, 132 CSSLs were used to identify the QTL of PAA. Seven QTLs were detected in one or two environments, and the other three QTLs, qPAA3, qPAA4.1 and qPAA7, were observed in all three environments. It was found that the QTL for PAA (qPAA5) were identified and located in the vicinity of QTL detected in previous reports (Cheng et al., 2011). However, the QTLs/genes of PAA had not been publicly reported in the qPAA7 interval. Therefore, qPAA7 is a novel locus that controls PAA in rice.
The higher accumulation of ROS in cells is one of the main reasons for the PAA in rice (Peng et al., 2018; Zafar et al., 2020). Recent studies have shown that ROS is an important signal for gene activation and plays an important role in plant growth and development, biotic and abiotic environmental stimuli responses, and programmed cell death (PCD) (Mittler, 2017). We analyzed the nine gene annotations in the qPAA7 interval. According to the results of the previous studies, LOC_Os07g41220,LOC_Os07g41230, and LOC_Os07g41280 showed the PCD or accumulation of ROS functions, and the other six candidate genes did not show similar functions (Zhang L. et al., 2015; Xiao et al., 2018; Lv et al., 2020). LOC_Os07g41220 was annotated as a peptidase aspartic family protein, and studies have found that aspartic proteases are related to plant development and cell death (Niu et al., 2013). Therefore, it is speculated that LOC_Os07g41220 has a similar functions. LOC_Os07g41230, which is annotated as methyl esterase-like, affects ROS accumulation by interacting with thioredoxin OsTrxm in rice (Hu et al., 2021). LOC_Os07g41280 is annotated as 6-phosphogluconolactase, and abnormal plant development is observed in Arabidopsis T-DNA insertion mutants (Xiong et al., 2009). In Arabidopsis, 6-phosphogluconolactonase 3 (PGL3) can interact with thioredoxin Trxm2 in the cytosol, further affecting the redox balance in the cell (Holscher et al., 2014). According to the previous alignment of genomic DNA and qRT-PCR analyze between CH121 and CSSL176, we found that LOC_Os07g41220 and LOC_Os07g41280 were most likely the candidate genes of qPAA7.
In this study, we identified the QTLs for panicle apical abortion using a total of 132 CSSLs in three environments. A novel major quantitative trait locus, qPAA7, was identified, then was fine mapped into an approximate 73.8Kb interval between the P11 and P19 markers on chromosome 7. There are nine candidate genes in qPAA7 region, among which LOC_Os07g41220 and LOC_Os07g41280 are most likely candidate genes of qPAA7. Transgenic studies are the gold standard for the validation of candidate gene function. In the future, such studies should be carried out for LOC_Os07g41220 and LOC_Os07g41280 to further elucidate their roles.
Data Availability Statement
The original contributions presented in the study are included in the article/Supplementary Material, further inquiries can be directed to the corresponding author/s.
Author Contributions
XW and CZ performed the experiments and wrote the manuscript. XS and LO analyzed the phenotypic data. JX, JF, XC, and XP contributed to PCR genotyping. WL, XH, and LL contributed to fled experiments. JB, LH, YC, HF, and DZ performed QTL analysis. HH and CZ designed the experiments. All the authors read and approved the final manuscript and approved the submitted version.
Funding
This work was supported by the National Natural Science Foundation of China (Grant No. 31860373), the Key R&D projects in Jiangxi Province, China (Grant No. 20192ACB60009), and the“5511”superior science and Technology Innovation team project of Jiangxi Province, China (Grant No. 2016-5BCB19005).
Conflict of Interest
The authors declare that the research was conducted in the absence of any commercial or financial relationships that could be construed as a potential conflict of interest.
Supplementary Material
The Supplementary Material for this article can be found online at: https://www.frontiersin.org/articles/10.3389/fpls.2021.683329/full#supplementary-material
Supplementary Figure 1 | The genotype of 132 CSSLs.
Supplementary Figure 2 | The daily average temperature of the 30-day period from rice booting to heading stage.
Supplementary Table 1 | Primers for qRT-PCR in this study.
Supplementary Table 2 | Primers for SSR and InDel markers in this study.
Supplementary Table 3 | Analysis of variance for aborted spikelets rate of 8 CSSLs in three environments.
Footnotes
References
Ali, A., Xu, P. Z., Riaz, A., and Wu, X. J. (2019). Molecular sciences review current advances in molecular mechanisms and physiological basis of panicle degeneration in rice. Int. J. Mol. Sci. 20:1613. doi: 10.3390/ijms20071613
Ashikari, M., Sakakibara, H., Lin, S. Y., Yamamoto, T., Takashi, T., Nishimura, A., et al. (2005). Cytokinin oxidase regulates rice grain production. Science 309, 741–745. doi: 10.1126/science.1113373
Bai, J. T., Zhu, X. D., Wang, Q., Zhang, J., Chen, H. Q., Dong, G. J., et al. (2015). Rice TUTOU1 encodes a suppressor of cAMP receptor-like protein that is important for actin organization and panicle development. Plant Physiol. 169, 1179–1191. doi: 10.1104/pp.15.00229
Cheng, Z. J., Mao, B. G., Gao, S. W., Zhang, L., Wang, J. L., Lei, C. L., et al. (2011). Fine mapping of qPAA8, a gene controlling panicle apical development in rice. J. Integr. Plant Biol. 53, 710–718. doi: 10.1111/j.1744-7909.2011.01055.x
He, H. H., Fu, J. R., Zhu, C. L., He, X. P., Peng, X. S., Chen, X. R., et al. (2008). Gan xin 688, a new combination of fragrant super hybrid rice. Hybrid Rice 23, 80–82.
Heng, Y. Q., Wu, C. Y., Long, Y., Luo, S., Ma, J., Chen, J., et al. (2018). OsALMT7 maintains panicle size and grain yield in rice by mediating malate transport. Plant Cell 30, 889–906. doi: 10.1105/tpc.17.00998
Holscher, C., Meyer, T., and Schaewen, A. V. (2014). Dual-Targeting of Arabidopsis 6-Phosphoglu conolactonase 3 (PGL3) to chloroplasts and peroxisomes involves interaction with Trxm2 in the cytosol. Mol. Plant 7, 252–255. doi: 10.1093/mp/sst126
Hu, B., Zhou, Y., Zhou, Z. H., Sun, B., Zhou, F., Yin, C. X., et al. (2021). Repressed OsMESL expression triggers reactive oxygen species mediated broad-spectrum disease resistance in rice. Plant Biotechnol. J. doi: 10.1111/pbi.13566 [Epub ahead of print].
Hu, Z. J., Lu, S. J., Wang, M. J., He, H. H., Sun, L., Wang, H. R., et al. (2018). A novel QTL qTGW3 encodes the GSK3/SHAGGY-like kinase OsGSK5/OsSK41 that interacts with OsARF4 to negatively regulate grain size and weight in rice. Mol. Plant 11, 736–749. doi: 10.1016/j.molp.2018.03.005
Ikeda, K., Ito, M., Nagasawa, N., Kyozuka, J., and Nagato, Y. (2007). Rice ABERRANT PANICLE ORGANIZATION 1, encoding an F-box protein, regulates meristem fate. Plant J. 51, 1030–1040. doi: 10.1111/j.1365-313X.2007.03200.x
Ikeda, K., Nagasawa, N., and Nagato, Y. (2005). ABERRANTPANICLE ORGANIZATION 1 temporally regulates meristem identity in rice. Dev. Biol. 282, 349–360. doi: 10.1016/j.ydbio.2005.03.016
Ikeda, K., Sunohara, H., and Nagato, Y. (2004). Developmental course of inflorescence and spikelet in rice. Breed Sci. 54, 147–156. doi: 10.1270/jsbbs.54.147
Itoh, J. I., Nonomura, K. I., Ikeda, K., Yamakl, S., Inukal, Y., Yamagishi, H., et al. (2005). Rice plant development: from zygote to spikelet. Plant Cell Physiol. 46, 23–47. doi: 10.1093/pcp/pci501
Komatsu, K., Maekawa, M., Ujiie, S., Satake, Y., Furutani, I., Okamoto, H., et al. (2003). LAX and SPA: major regulators of shoot branching in rice. Proc. Natl. Acad. Sci. U.S.A. 100, 11765–11770. doi: 10.1073/pnas.1932414100
Komatsu, M., Chujo, A., Nagato, Y., Shimamoto, K., and Kyozuka, J. (2003). FRIZZY PANICLE is required to prevent the formation of axillary meristems and to establish floral meristem identity in rice spikelets. Development 130, 3841–3850. doi: 10.1242/dev.00564
Kosambi, D. D. (1944). The estimation of map distances from recombination values. Ann. Eugen. 12, 172–175. doi: 10.1111/j.1469-1809.1943.tb02321.x
Lander, E. S., Green, O., Abrahamson, J., Barlow, A., Daly, M. J., Lincoln, S. E., et al. (1987). Mapmaker: an interactive computer package for constructing primary genetic linkage maps of experimental and natural populations. Genomics 1, 174–181. doi: 10.1016/0888-7543(87)90010-3
Léran, S., Varala, K., Boyer, J. C., Chiurazzi, M., Crawford, N., Françoise, D. V., et al. (2014). A unified nomenclature of NITRATE TRANSPORTER 1/PEPTIDE TRANSPORTER family members in plants. Trends Plant Sci. 19, 5–9. doi: 10.1016/j.tplants.2013.08.008
Li, L. F., Sun, X. T., Ouyang, L. J., Zeng, B. H., Liu, Y., Luo, L. Y., et al. (2018). Influencing factors and genetic research progress of rice spikelet degeneration. Acta Agric. Nucl. Sin. 32, 291–296.
Li, X. Y., Qian, Q., Fu, Z. M., Wang, Y. H., Xiong, G. S., Zeng, D. L., et al. (2003). Control of tillering in rice. Nature 422, 618–621. doi: 10.1038/nature01518
Livak, K. J., and Schmittgen, T. D. (2001). Analysis of relative gene expression data using real-time quantitative PCR and the 2-δδCT method. Methods 25, 402–408. doi: 10.1006/meth.2001
Lv, J., Shang, L. G., Chen, Y., Han, Y., Yang, X. Y., Xie, S. Z., et al. (2020). OsSLC1 encodes a pentatricopeptide repeat protein essential for early chloroplast development and seedling survival. Rice 13:25. doi: 10.1186/s12284-020-00385-5
Meng, L., Li, H. H., Zhang, L. Y., and Wang, J. K. (2015). QTL IciMapping: integrated software for genetic linkage map construction and quantitative trait locus mapping in biparental populations. Crop J. 3, 269–283. doi: 10.1016/j.cj.2015.01.001
Nagasawa, N., Hibara, K. I., Heppard, E. P., Vander Velden, K. A., Luck, S., Beatty, M., et al. (2013). GIANT EMBRYO encodes CYP78A13, required for proper size balance between embryo and endosperm in rice. Plant J. 75, 592–605. doi: 10.1111/tpj.12223
Niu, N. N., Liang, W. Q., Yang, X. J., Jin, W. L., Wilson, A. Z., Hu, J. P., et al. (2013). EAT1 promotes tapetal cell death by regulating aspartic proteases during male reproductive development in rice. Nat. Commun. 4:1445. doi: 10.1038/ncomms2396
Ouyang, J., Cai, Z. Y., Xia, K. F., Wang, Y. Q., Duan, J., and Zhang, M. Y. (2010). Identification and analysis of eight peptide transporter homologs in rice. Plant Sci. 179, 374–382. doi: 10.1016/j.plantsci.2010.06.013
Panaud, O., Chen, X., and McCouch, S. R. (1996). Development microsatel lite markers characterization of simple sequence length polymorphism (SSLP) in rice (Oryza sativa L.). Mol. Gen. Genet. 252, 597–607. doi: 10.1007/BF02172406
Peng, Y. B., Hou, F. X., Bai, Q., Xu, P. Z., Liao, Y. X., Zhang, H. Y., et al. (2018). Rice calcineurin B-like protein-interacting protein kinase 31 (OsCIPK31) is involved in the development of panicle apical spikelets. Front. Plant Sci. 9:1661. doi: 10.3389/fpls.2018.01661
Qiao, W. H., Qi, L., Cheng, Z. J., Su, L., Li, J., Sun, Y., et al. (2016). Development and characterization of chromosome segment substitution lines derived from Oryza rufipogon in the genetic background of O. sativa spp. indica cultivar 9311. BMC Genomics 17:580. doi: 10.1186/s12864-016-2987-5
Rao, N. N., Prasad, K., Kumar, P. R., and Vijayraghavan, U. (2008). Distinct regulatory role for RFL, the rice LFY homolog, in determining flowering time and plant architecture. Proc. Natl. Acad. Sci. U.S.A. 105, 3646–3651. doi: 10.1073/pnas.0709059105
Rogers, S. O., and Bendich, A. J. (1985). Extraction of DNA from milligram amounts of fresh, herbarium and mummified plant tissues. Plant Mol. Biol. 5, 69–76. doi: 10.1007/BF00020088
Sakamoto, T., and Matsuoka, M. (2004). Generating high-yielding varieties by genetic manipulation of plant architecture. Curr. Opin. Biotechnol. 15, 144–147. doi: 10.1016/j.copbio.2004.02.003
Sun, Y. J., Zhou, J., Xu, H. S., and Yu, S. B. (2010). QTL and their interactions for plant height in rice chromosomal substitution segment lines. Mol. Plant Breed. 08, 1068–1073.
Tabuchi, H., Zhang, Y., Hattori, S., Omae, M., Sato, S. S., Oikawa, T., et al. (2011). LAX PANICLE2 of rice encodes a novel nuclear protein and regulates the formation of axillary meristems. Plant Cell 23, 3276–3287. doi: 10.1105/tpc.111.088765
Tan, C. J., Sun, Y. J., Xu, H. S., and Yu, S. B. (2011). Identification of quantitative trait locus and epistatic interaction for degenerated spikelets on the top of panicle in rice. Plant Breed. 130, 117–184. doi: 10.1111/j.1439-0523.2010.01770.x
Teo, Z. W. N., Song, S. Y., Wang, Y. Q., Liu, J., and Yu, H. (2014). New insights into the regulation of inflorescence architecture. Trends Plant Sci. 19, 158–165. doi: 10.1016/j.tplants.2013.11.001
Tetsuo, O., and Junko, K. (2009). Two-Step regulation of LAX PANICLE1 protein accumulation in axillary meristem formation in rice. Plant Cell 21, 1095–1108. doi: 10.1105/tpc.108.065425
Vollbrecht, E., Springer, P. S., Goh, L., Buckler, E. S., and Martienssen, R. (2005). Architecture of floral branch systems in maize and related grasses. Nature 436, 1119–1126. doi: 10.1038/nature03892
Voorrips, R. E. (2002). MapChart: software for the graphical presentation of linkage maps and QTLs. J. Hered. 93, 77–78. doi: 10.1093/jhered/93.1.77
Wang, B., Liu, H. M., Mao, B. G., Gao, S. W., Xu, H. B., and Ge, J. G. (2011). QTL analysis on apical spikelet abortion in rice. Chin. J. Rice Sci. 25, 561–564.
Wang, J. K., Wang, X. Y., Crossa, J., Crouch, J. T., Weng, J. F., Zhai, H. Q., et al. (2006). QTL mapping of grain length in rice (Oryza sativa L.) using chromosome segment substitution lines. Genet. Res. 88, 93–104. doi: 10.1017/S0016672306008408
Wang, Q. L., Sun, A. Z., Chen, S. T., Chen, L. S., and Guo, F. Q. (2018). SPL6 represses signalling outputs of ER stress in control of panicle cell death in rice. Nat. Plants 4, 280–288. doi: 10.1038/s41477-018-0131-z
Wang, S. K., Li, S., Liu, Q., Wu, K., Zhang, J. Q., Wang, S. S., et al. (2015). The OsSPL16-GW7 regulatory module determines grain shape and simultaneously improves rice yield and grain quality. Nat. Genet. 47, 949–954. doi: 10.1038/ng.3352
Wang, X. L., Li, W. X., Zeng, B. H., Sun, X. T., Ouyang, L. J., Chen, X. R., et al. (2020a). QTL detection and stability analysis of rice grain shape and thousand-grain weight based on chromosome segment substitution lines. Acta Agron. Sin. 46, 1517–1525.
Wang, X. L., Liu, Y., Sun, X. T., Ouyang, L. J., Pan, J. L., Peng, X. S., et al. (2020b). Identification and stability analysis of QTL for grain quality traits under multiple environments in rice. Chin. J. Rice Sci. 34, 17–27.
Wang, Y. L., Zhang, Y. P., Xiang, J., Wang, L., Chen, H. Z., Zhang, Y. K., et al. (2017). Response of indica rice spikalet differentiation and degeneration to air temperature and solar radiation of different sowing dates. Chin. J. Appl. Ecol. 28, 3571–3580.
Wang, Y. X., Xiong, G. S., Hu, J., Jiang, L., Yu, H., Xu, J., et al. (2015). Copy number variation at the GL7 locus contributes to grain size diversity in rice. Nat. Genet. 47, 944–948. doi: 10.1038/ng.3346
Xiao, H. J., Zhang, Q. N., Qin, X. J., Xu, Y. H., Ni, C. Z., Huang, J. S., et al. (2018). Rice PPS1 encodes a DYW motif-containing pentatricopeptide repeat protein required for five consecutive RNA-editing sites of nad3 in mitochondria. New Phytol. 220, 878–892. doi: 10.1111/nph.15347
Xing, Y. Z., and Zhang, Q. F. (2010). Genetic and molecular bases of rice yield. Annu. Rev. Plant Biol. 61, 421–422. doi: 10.1146/annurev-arplant-042809-112209
Xiong, Y. Q., DeFraia, C., Williams, D., Zhang, X. D., and Mou, Z. L. (2009). Characterization of arabidopsis 6-Phosphogluconolactonase T-DNA insertion mutants reveals an essential role for the oxidative section of the plastidic pentose phosphate pathway in plant growth and development. Plant Cell Physiol. 50, 1277–1291. doi: 10.1093/pcp/pcp070
Xu, F., Fang, J., Ou, S. J., Gao, S. P., Zhang, F. X., Du, L., et al. (2015). Variations in CYP78A13 coding region influence grain size and yield in rice. Plant Cell Environ. 38, 800–811. doi: 10.1111/pce.12452
Xu, H. S., Sun, Y. J., Zhou, H. J., and Yu, S. B. (2007). Development and characterization of contiguous segment substitution lines with background of an elite restorer line. Acta Agron. Sin. 33, 979–986.
Yamagishi, J., Miyamoto, N., Hirotsu, S., Laza, R. C., and Nemoto, K. (2004). QTLs for branching, floret formation, and pre-flowering floret abortion of rice panicle in a temperate japonica × tropical japonica cross. Theor. Appl. Genet. 109, 1555–1561. doi: 10.1007/s00122-004-1795-5
Yang, T. F., Zeng, R. Z., Zhu, H. T., Chen, L., Zhang, Z. M., Ding, X. H., et al. (2010). Effect of grain length gene GS3 in pyramiding breeding of rice. Mol. Plant Breed. 8, 59–66.
Yang, W. B., Gao, M. J., Yin, X., Liu, J. Y., Xu, Y. H., Zeng, L. J., et al. (2013). Control of rice embryo development, shoot apical meristem maintenance, and grain yield by a novel cytochrome P450. Mol. Plant 6, 1945–1960. doi: 10.1093/mp/sst107
Yao, Y. L., Yamamoto, Y., Yoshida, T., Nitta, Y., and Miyazaki, A. (2000). Response of differentiated and degenerated spikelets to top-dressing, shading and day/night temperature treatments in rice cultivars with large panicles. Soil Sci. Plant Nutr. 46, 631–641. doi: 10.1080/00380768.2000.10409128
Zafar, S. A., Patil, S. B., Uzair, M., Fang, J. J., Zhao, J. F., Guo, T. T., et al. (2020). DEGENERATED PANICLE AND PARTIAL STERILITY 1 (DPS1) encodes a cystathionine β-synthase domain containing protein required for anther cuticle and panicle development in rice. New Phytol. 225, 356–375. doi: 10.1111/nph.16133
Zeng, B., Li, M., Yang, Z. R., Tan, C. J., Dong, H. L., and Yu, S. B. (2009). Mapping of a novel semi-sterile pollen QTL in rice. Acta Agron. Sin. 35, 1584–1589. doi: 10.3724/sp.j.1006.2009.01584
Zhang, D., Fang, Y. X., Pan, J. J., Ju, P. N., Cui, J., Ge, C. W., et al. (2017). Genetic analysis and fine mapping of the gene for a no spikelet mutant nsp1 in rice. Acta Agric. Nucl. Sin. 31, 1–7.
Zhang, L., Mao, D. H., Xing, F., Bai, X. F., Zhao, H., Yao, W., et al. (2015). Loss of function of OsMADS3 via the insertion of a novel retrotransposon leads to recessive male sterility in rice (Oryza sativa). Plant Sci. 238, 188–197. doi: 10.1016/j.plantsci.2015.06.007
Zhang, T., Wang, S. M., Sun, S. F., Zhang, Y., Li, J., You, J., et al. (2020). Analysis of QTL for grain size in a rice chromosome segment substitution line Z1392 with long grains and fine mapping of qGL-6. Rice 13:40. doi: 10.1186/s12284-020-00399-z
Zhang, X. Y., Luo, S., Wang, M., Cong, N., Zhao, Z. C., and Cheng, Z. J. (2015). Fine mapping of rice panicle apical abortion gene qPAA3 interacting with SP1. Sci. Agric. Sin. 48, 2287–2295.
Zhu, Q. H., Hoque, M. S., Dennis, E. S., and Upadhyaya, N. M. (2003). Dstagging of BRANCHED FLORETLESS 1 (BFL1) that mediates the transition from spikelet to floret meristem in rice. BMC Plant Biol. 3:6. doi: 10.1186/1471-2229-3-6
Keywords: rice, panicle apical abortion, fine mapping, quantitative trait locus, CSSL
Citation: Wang X, Li L, Sun X, Xu J, Ouyang L, Bian J, Chen X, Li W, Peng X, Hu L, Cai Y, Zhou D, He X, Fu J, Fu H, He H and Zhu C (2021) Fine Mapping of a Novel Major Quantitative Trait Locus, qPAA7, That Controls Panicle Apical Abortion in Rice. Front. Plant Sci. 12:683329. doi: 10.3389/fpls.2021.683329
Received: 20 March 2021; Accepted: 09 June 2021;
Published: 07 July 2021.
Edited by:
Hanwei Mei, Shanghai Agrobiological Gene Center, ChinaReviewed by:
Deyong Ren, China National Rice Research Institute, Chinese Academy of Agricultural Sciences, ChinaTao Guo, South China Agricultural University, China
Copyright © 2021 Wang, Li, Sun, Xu, Ouyang, Bian, Chen, Li, Peng, Hu, Cai, Zhou, He, Fu, Fu, He and Zhu. This is an open-access article distributed under the terms of the Creative Commons Attribution License (CC BY). The use, distribution or reproduction in other forums is permitted, provided the original author(s) and the copyright owner(s) are credited and that the original publication in this journal is cited, in accordance with accepted academic practice. No use, distribution or reproduction is permitted which does not comply with these terms.
*Correspondence: Haohua He, hhhua64@163.com; Changlan Zhu, zhuchanglan@163.com