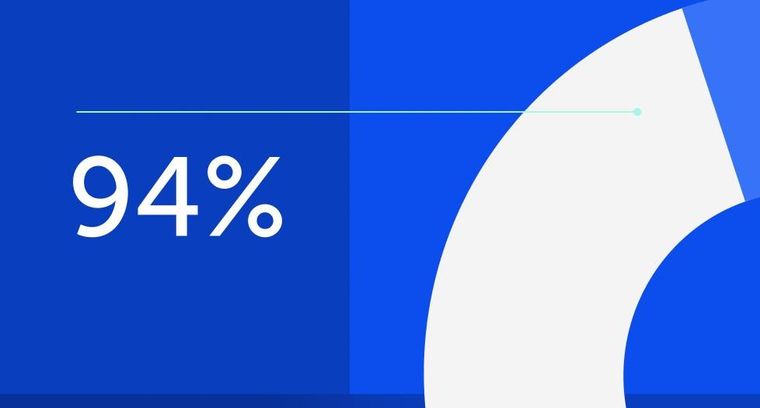
94% of researchers rate our articles as excellent or good
Learn more about the work of our research integrity team to safeguard the quality of each article we publish.
Find out more
ORIGINAL RESEARCH article
Front. Plant Sci., 02 June 2021
Sec. Plant Breeding
Volume 12 - 2021 | https://doi.org/10.3389/fpls.2021.674327
This article is part of the Research TopicAccelerating Genetic Gains in PulsesView all 15 articles
The contemporary lentil (Lens culinaris ssp. culinaris) industry in Australia started in the late 1980s. Yield in farmers’ fields averages 1.2 t ha–1 nationally and has not increased over three decades. Lack of yield progress can be related to a number of non-mutually exclusive reasons: expansion of lentil to low-yielding environments, lack of genetic gain in yield, lack of progress in agronomic practices, and lack of adoption of superior technologies. The aims of this study were to (i) quantify the genetic gain in lentil yield since 1988, (ii) explore the variation in the expression of genetic gain with the environment, and (iii) identify shifts in crop phenotype associated with selection for yield and agronomic adaptation. We grew a historic collection of 19 varieties released between 1988 and 2019 in eight environments resulting from the factorial combination of two sowing dates, two water regimes, and two seasons. Across environments, yield varied 11-fold from 0.2 to 2.2 t ha–1. The rate of genetic gain averaged 20 kg ha–1 year–1 or 1.23% year–1 across environments and was higher in low-yield environments. The yield increase was associated with substantial shifts in phenology. Newer varieties had a shorter time to flowering and pod emergence, and the rate of change in these traits was more pronounced in slow-developing environments (e.g., earlier sowing). Thermal time from sowing to end of flowering and maturity were shorter in newer varieties, and thermal time from pod emergence to maturity was longer in newer varieties; the rate of change in these traits was unrelated to developmental drivers and correlated with environmental mean yield. Genetic gain in yield was associated with increased grain number and increased harvest index. Despite their shorter time to maturity, newer varieties had similar or higher biomass than their older counterparts because crop growth rate during the critical period increased with the year of release. Genotype-dependent yield increased over three decades in low-yield environments, whereas actual farm yield has been stagnant; this suggests an increasing yield gap requiring agronomic solutions. Genetic improvement in high-yield environments requires improved coupling of growth and reproduction.
Australia currently produces over 300,000 t of lentils annually and contributes to approximately 10% of global trade, whereas Canada produces over 3 Mt and accounts for 50% of trade. The contemporary lentil industry in Australia started in the late 1980s with the introduction of late flowering, low-yielding forage types, and after a lag phase, acreage increased linearly since the mid-1990s (Figure 1A). Production increased in parallel to acreage (Figure 1B), whereas national average yield remained stagnant at 1.2 t ha–1, with large variation from failed crops to ∼2 t ha–1 (Figure 1C). In comparison, the acreage of the Canadian lentil industry grew exponentially since its inception, and increases in both acreage and yield contributed to an increase in production (Figures 1D–F).
Figure 1. Area, production, and yield of lentils in (A–C) Australia and (D–F) Canada. In (A,B,E,F), slopes and standard errors are shown for the fitted least-square regressions. In (A,B,E), inflection points were identified fitting piece-wise models. Note difference in scales between Australia and Canada for area and production. Source: FAOSTAT, July 2020.
Lack of progress in lentil average national yield in Australia can be related to several non-mutually exclusive reasons: expansion of the crop to drier, lower-yielding environments; lack of genetic improvement in yield; lack of progress in agronomic practices; and lack of adoption of superior technologies. Most of the Australian lentil is grown in the medium rainfall areas (350–450 mm year–1) of southern Australia, in particular, the sandy loam soils in South Australia and the alkaline gray cracking clays of Victoria. These regions feature winter-dominant rainfall, with a combination of drought, frost, and heat restricting the yield of pulses (Sadras et al., 2012; Lake et al., 2016, 2021). Supported by better agronomy (Llewellyn et al., 2012), pulses in the Mallee have increased from 7% in 2006 to 24% in 2017; this increase was at the expense of fallow, which declined from 18 to 2%, and pasture, which declined from 18 to 12% (Moodie and Brand, 2019). In comparison with the more productive Wimmera (440 mm year–1)1, where lentil yield can reach more than 4.5 t ha–1, yields in the Mallee (300 mm year–1)2 are up to ∼3.5 t ha–1. Hence, expansion of the crop into drier areas has likely contributed to stagnant national average yield. A strong focus on lentil herbicide tolerance to improve weed management may have also had indirect consequences for yield (Mao et al., 2015; McMurray et al., 2019).
Here, we focus on genetic improvement. Despite recognized limitations, retrospective studies comparing historic collections of varieties are routinely used with two objectives—to quantify the rate of genetic gain of a given breeding program and to uncover phenotypic changes associated with selection for yield (Austin et al., 1980; Slafer, 1994; Fischer et al., 2014; Tamagno et al., 2020). The assumption underlying the second objective is that making explicit the realized phenotypic change can guide further improvement. The absolute rate of genetic gain (kilograms per hectare per year) is often higher in environments with higher yield potential (Austin et al., 1980; Sadras et al., 2016), whereas the relative rate of genetic gain (percentage per year) is mostly independent of the environment (Fischer et al., 2014); quantifying the environmental influence on the expression of genetic gain in yield is thus important. The aims of this study were to (i) quantify the genetic gain in lentil yield since 1988, (ii) explore variation in the expression of genetic gain with the environment, and (iii) identify shifts in the crop phenotype associated with selection for yield and agronomic adaptation.
We reanalyze the results of experiments reported by Lake and Sadras (2021), including 19 varieties released and used in the Australian lentil breeding program between 1988 and 2019 (Table 1). Crops were grown in eight environments with an 11-fold variation in yield from 0.2 to 2.2 t ha–1. Lake and Sadras (2021) emphasized yield components from a physiological perspective; here, we focus on yield and phenotypic shifts with the year of release.
Trials were established on a calcic luvisol soil at Roseworthy (−34.5, 138.69). Briefly, environments resulted from the combination of two seasons (2018, 2019), two sowing dates, and two water regimes. Early sowings were on April 24, 2018, and April 29, 2019, and the late sowings on June 6, 2018, and June 24, 2019. Early-sown crops were irrigated or rainfed until June 26, 2018, and August 1, 2019, when rainout shelters were deployed to exclude rainfall until harvest, whereas late-sown crops were irrigated or rainfed. Hereafter, we refer to irrigated treatment as “wet” and rainfed and rainout shelter treatments as “dry.” Sowing date was assigned to the main plot, water regime to subplot, and varieties randomized within subplots with three replicates per treatment. Each experimental plot comprised six rows, 0.23 m apart, 5 m long, with a target plant density of 120 plants m–2.
Crops were phenotyped for phenology, crop growth rate, yield, and its components: biomass, harvest index, grain number, and grain size.
We scored phenology twice weekly to determine the time from sowing (S) to 50% of the plants within the plot at flowering (F), pod emergence (PE), end of flowering (EoF), and maturity (M). Phenological stages are expressed on a thermal time scale with a base temperature of 0°C (Summerfield et al., 1985). The ratio PE-M:S-M was taken as a measure of the grain filling period in relation to the total cycle.
We measured biomass and crop growth rate non-destructively using the Canopeo app (Patrignani and Ochsner, 2015), which provides a two-dimensional measure of canopy coverage, combined with canopy height to return a three-dimensional trait. We used a calibration derived from a separate trial, in which we regressed actual biomass vs. Canopeo × height. Canopeo photographs were taken looking down from 140 cm every 7–10 days.
At maturity, we harvested shoots in 1-m2 sections from the four central rows of the plot to determine grain yield and its components. Harvest index was derived from shoot biomass and grain yield. Further details of methods are in Lake and Sadras (2021).
We tested trait response to variety, environment, and the interaction using analysis of variance with Genstat (20th edition). Best linear unbiased predictions were calculated with Multi Environment Trial Analysis with R for Windows version 6.0. We calculated the genetic rate of change as the slope of the least-square regression between trait and year of release. We calculated actual rates, e.g., kilograms per hectare per year for yield, and rates relative to the newest variety (Fischer et al., 2014). Rates were calculated for data pooled across all environments and for each environment separately. Environmental dependence in the expression of genetic shifts in yield and other traits was explored by plotting the rate of genetic change against the environmental mean of yield and the environmental mean of the trait. We report p-value as a continuous quantity and Shannon information transform [s = -log2(p)] as a measure of the information against the tested hypothesis (Greenland, 2019).
Table 2 summarizes growing conditions and yield in the eight environments. Growing-season rainfall + irrigation ranged from 117 mm for the early-sown, dry crop in 2018, to 332 mm for the early-sown, wet crop in 2019. Across varieties, yield ranged from 21 g m–2 for early-sown, dry treatment in 2018, to 221 g m–2 for early-sown, wet treatment in 2018. Across varieties, average yield was positively associated with growing season rainfall (y = −18.1 + 0.59 x, R2 = 0.50; p = 0.052, s = 4.3) and with minimum temperature (y = −90.8 + 38.2 x, R2 = 0.69; p = 0.010, s = 6.6).
Table 2. Mean yield across varieties and growing conditions in eight environments resulting from combinations of season, sowing date, and water regime.
All phenostages varied with variety, environment, and their interaction (Supplementary Table 1). Table 3 shows absolute and relative rates of change of phenological traits for the pooled data. Across environments, thermal time from sowing to flowering, pod emergence, end of flowering, and maturity were all shortened with the year of release. In contrast, the thermal time between pod emergence and maturity and the proportion of the season between pod emergence and maturity both increased with the year of release.
Table 3. Absolute and relative rate of genetic change (±SE) for lentil traits in varieties released between 1988 and 2019.
Figure 2 shows the rate of change of phenological traits with the year of release as a function of (a) the environmental mean for the trait and (b) the environmental mean for yield. The environmental mean of the trait captures temperature, photoperiod, and water influences on development, empirically defining slow- and fast-developing environments. For example, the environmental mean thermal time to flowering ranged from 1039°Cd in the late-sown wet treatment 2019 to 1451°Cd in the early-sown wet treatment in 2019 (Table 2). The rates of change in thermal time to flowering and to pod emergence were stronger, i.e., more negative, in environments favoring slower development (Figures 2A,C). For example, the rate of change in flowering changed from −1.20 to −0.46% year–1 with environmental means from 1411 to 1167°Cd. The rates of change in thermal time to flowering and maturity were proportional to environmental mean yield (Figures 2F,H,J) and unrelated to the environmental mean of the phenostage (Figures 2E,G,I). Thermal time from pod emergence to maturity relative to thermal time from sowing to maturity was related to the environmental mean for both duration of phenostage and yield (Figure 3).
Figure 2. Rate of change of thermal time from sowing to flowering, pod emergence, end of flowering and maturity, and the duration between pod emergence and maturity against the environmental mean phenostage (A,C,E,G,I) and the environmental mean yield (B,D,F,H,J). Lines are least-square regressions and are only presented where p < 0.05, s > 4.3. Rates are relative to the newest variety. Symbols are: blue (2018), red (2019), circles (early sowing), square (late sowing), open (rainfed), closed (irrigated).
Figure 3. Rate of change of the ratio: time between pod emergence and maturity/time to maturity against environmental mean of the ratio (A) and environmental mean yield (B). Lines are least-square regressions. Rates are relative to the newest variety. Symbols are: blue (2018), red (2019), circles (early sowing), square (late sowing), open (rainfed), closed (irrigated).
Yield varied ninefold with variety (Table 1) and 10-fold with environment (Table 2), with no interaction between environment and variety (Supplementary Table 1). Across environments, yield increased with the year of release at 20 kg ha–1 year–1 or 1.23% year–1 (Table 3). The rate of genetic gain in yield declined linearly with increasing environmental mean yield (Figure 4A).
Figure 4. Rate of change of yield (A), grain number (B), grain size (C), biomass (D), crop growth rate (E), and harvest index (F) against environmental mean yield. Lines are least-square regressions. Rates are relative to the newest variety. Symbols are: blue (2018), red (2019), circles (early sowing), square (late sowing), open (rainfed), closed (irrigated).
Grain number varied fourfold with variety and 10-fold with the environment, with a significant interaction between environment and variety (Supplementary Table 1). Across environments, grain number increased with the year of release at 34 seeds m–2 year–1 or 0.92% year–1 (Table 3). The rate of change in grain number with the year of release was higher in low-yielding environments (Figure 4B). Grain size varied with variety (twofold) and with the interaction between environment and variety (Supplementary Table 1). Across environments, grain size increased by 0.40 mg seed year–1 or 0.96% year–1 (Table 3). The rate of genetic change in grain size was unrelated to environmental mean yield (Figure 4C).
Shoot biomass at maturity varied little between varieties (<1.5-fold) and varied ∼5-fold with environment, with no interaction between environment and variety (Supplementary Table 1). Across environments, the absolute rate of change in biomass with the year of release was close to zero, and the relative rate was 0.38% year–1 (Table 3). The association between the relative rate of change in biomass and environmental mean yield was weak and negative (Figure 4D).
The crop growth rate in the critical period varied 2.5-fold with variety and fourfold with the environment, with no interaction between environment and variety (Supplementary Table 1). Across environments, the crop growth rate increased with the year of release at 0.07 kg ha–1°Cd–1 year–1 or 1.46% year–1. The rate of change in crop growth rate with the year of release was higher in more stressful environments (Figure 4E).
Harvest index varied sixfold with variety and 3.5-fold with the environment and also varied with the interaction between environment and variety (Supplementary Table 1). Across environments, the harvest index increased 0.0042 year–1 or 1.25% year–1 (Table 3). The rate of increase in harvest index with the year of release almost halved between the lowest and highest yielding environments (Figure 4F).
Our measured genetic gain for Australian lentils between 1988 and 2019 averaged 20 kg ha–1 year–1 or 1.23% year–1 across eight environments. It compares with the rate of 18–27 kg ha–1 year–1 for Ethiopian lentil in two environments (Bogale et al., 2015); 31–35 kg ha–1 year–1 for Moroccan lentil (Idrissi et al., 2019); 11–17 kg ha–1 year–1 for kabuli (Tadesse et al., 2018), and 32 kg ha–1 year–1 for desi chickpea in Ethiopia (Bekele et al., 2016).
Contrary to the observation that relative rates of genetic gain are independent of the environment in cereals (Fischer et al., 2014), here, we found that the expression of genetic gain in lentil yield was stronger under stress and often close to zero in high-yielding environments (Figure 4A). The rates of genetic change in the main drivers of yield, including grain number, crop growth rate, and harvest index, were also larger in low-yielding environments (Figure 4). Consistent with our finding, well-managed National Variety Trials in southern Australia, which benchmark current and new germplasm, show no improvement in either maximum or environmental mean yield between 2009 and 2018 (Supplementary Table 1). For lentils in Ethiopia, the rate of genetic gain in yield relative to the newest variety was 0.80% year–1 in an environment of 1.3 t ha–1 average yield and 0.92% year–1 in an environment of 4.8 t ha–1 (Bogale et al., 2015). For lentils in Morocco, the rate of genetic gain relative to the local check was 0.68% year–1 in a dry environment (200–350 mm year–1) compared with 1.0% year–1 in a wetter environment (300–500 mm year–1). We conclude that the proposition of environment-independent relative rates of genetic gain cannot be generalized.
The higher rate of genetic gain in low-yielding environments partially associates with the late phenology of early introductions. The breeding program has continually decreased time to flowering, podding, and maturity (Figure 2 and Table 3) as earliness is critical for yield in short, dry seasons (Silim et al., 1993; Kumar et al., 2012). Similarly, breeding has focused on taller and more upright crops to facilitate improved machine harvest in drier environments with actual gains of 0.12 cm year–1 (data not shown); lower crop growth rate and shorter plants of earlier varieties would impact yield under dry or short-season conditions (Erskine, 2009; Muehlbauer et al., 2009).
Genetic gain in yield in Mediterranean, East Asian, and Sub-Saharan African environments has been associated with earlier flowering in lentils, chickpea, and wheat (Siddique et al., 1989; Erskine et al., 1994; Berger et al., 2004, 2006; Sadras and Lawson, 2011; Bogale et al., 2015). This is an important adaptation, achieving yield before the concurrent water and thermal stress later in the season (Thomson et al., 1997; Erskine et al., 2011).
We found three traits that offset the reduction in yield associated with shorter time to flowering and maturity: a longer period from pod emergence to maturity relative to crop duration (sowing to maturity), an increased harvest index, and an increased growth rate during the critical period. Harvest index was partially related to the extended period from pod emergence to maturity.
In indeterminate lentil, early flowering, combined with a lengthening of the reproductive period, increases the probability of grain set and filling to occur in favorable conditions while maintaining vegetative growth. However, a lengthening of the reproductive period may have negative effects under extreme stress, with Syrian research showing reproductive duration was negatively associated with lentil yield (Silim et al., 1993). For our set of varieties and environments, there was a negative association between time to flowering and time between pod emergence and maturity in the longer duration environments, with no relationship in the stress environments (Supplementary Table 2). This is a reflection of the later flowering, earlier Australian releases being adapted from material originating in longer season environments where they can flower later and extend reproduction.
The average rate of genetic gain in yield, 1.23% year–1, compares with the rate of change of 1.46% year–1 for growth rate, 0.92% year–1 for grain number, and 1.25% year–1 for harvest index. In soybean, early gains in yield were driven by increased biomass and harvest index (Koester et al., 2014; Suhre et al., 2014), and allometric analysis further highlights the improvement in reproductive allocation (Tamagno et al., 2020). Lentil can grow large dense canopies and tend to suffer from a low harvest index, particularly in higher-yielding conditions (Kusmenoglu and Muehlbauer, 1998; Hanlan et al., 2006; Lake and Sadras, 2021). Phenotypes adapted to the main producing regions of Canada are assumed to combine moderate biomass and high harvest index (Hanlan et al., 2006). Averaged across environments, CIPAL 1701 had the highest harvest index at 0.33, and the average across varieties was 0.23 compared with reported maxima 0.44–0.59 (Whitehead et al., 2000; Malhi et al., 2007; Unkovich et al., 2010); the maximum for our dataset (0.54) indicates an opportunity for improvement.
Grain size in Canadian lentil (Muehlbauer, 1974) and kabuli chickpea in India (Gowda et al., 2011) was negatively correlated with yield. Australian breeding between 1988 and 2019 has achieved both increased grain size and yield (Table 3). In United States soybean improvement, grain size increased initially (Specht and Williams, 1984), but more recent work shows grain number has driven yield gain (Tamagno et al., 2020); this is also the case for Canadian soybean (Voldeng et al., 1997); and Ethiopian common bean (Bezaweletaw et al., 2006).
The indeterminate nature of lentils provides opportunities and challenges with large environmental variation in biomass. As biomass has low heritability, selection for crop growth rate in physiologically meaningful windows and harvest index are likely to be effective in increasing yield (Lake and Sadras, 2021). In short-season Mediterranean environments, combining early flowering and longer reproductive duration may improve harvest index and reduce problems associated with excessive vegetative growth. Successfully combining these traits may provide genetic gains in yield with less risk of a trade-off between yield in high- and low-yielding environments. Selection for early flowering is desirable in shorter Mediterranean environments, but there is a limit to how far flowering can be advanced against frost risk in the target population of environments (Lake et al., 2021). A longer flowering window can offset yield losses from limited frosts, but regular frosts may be more problematic, particularly in shorter seasons.
Over the three decades of Australian lentil breeding and for our sample of varieties and environments, genetic gain in yield was 20 kg ha–1 year–1 or 1.23% year–1. The estimated genetic gain in yield was larger in lower-yielding environments. This genetic gain combined with improved agronomy has allowed the spread of lentils into lower rainfall regions of Australia, increasing rotational options and allowing more diverse cropping systems (Llewellyn et al., 2012; Moodie and Brand, 2019). The lack of improvement in the national average yield over this period is partially related to the expansion of the crop to intrinsically lower-yielding environments. Further improvements in lentil production require the adoption of improved practices to close the gap between water-limited and actual yield and a stronger focus in breeding for superior combinations of crop growth rate, biomass, and harvest index for higher yield potential.
The raw data supporting the conclusions of this article will be made available by the authors, without undue reservation.
VS contributed to the planning and analysis of research and the writing of the manuscript. GR contributed to the writing of the manuscript. LL contributed to the planning, performing, analysis of research, and the writing of the manuscript. All authors contributed to the article and approved the submitted version.
The authors declare that the research was conducted in the absence of any commercial or financial relationships that could be construed as a potential conflict of interest.
We thank the Grains Research and Development Corporation of Australia for financial support, Jason Brand, Arun Shunmugan, and Agriculture Victoria for contributing seed and knowledge, Penny Roberts, Phil Rundle, John Nairne, and SARDI Clare for the maintenance of crops and Han Chow, Hadtamu Tura, Vy-Price Beck, Annabel O’Dea, Tanja Lenz, Jose Fernandez, Justyn Thompson, and Tan Dang for field and laboratory work.
The Supplementary Material for this article can be found online at: https://www.frontiersin.org/articles/10.3389/fpls.2021.674327/full#supplementary-material
Austin, R. B., Bingham, J., Blackwell, R. D., Evans, L. T., Ford, M. A., Morgan, C. L., et al. (1980). Genetic improvements in winter wheat yields since 1900 and associated changes. J. Agric. Sci. 94, 675–689. doi: 10.1017/S0021859600028720
Bekele, D., Fikre, A., Eshete, M., Korbu, L., and Girma, N. (2016). “Genetic progresses achieved in Ethiopian chickpea (Cicer arietinum L.) breeding program based on grain yield and seed size,” in Proceeding of the Harnessing Chickpea Value Chain for Nutrition Security and Commercialization of Smallholder Agriculture in Africa, (ReaserchGate), 119.
Berger, J. D., Ali, M., Basu, P. S., Chaudhary, B. D., Chaturvedi, S. K., Deshmukh, P. S., et al. (2006). Genotype by environment studies demonstrate the critical role of phenology in adaptation of chickpea (Cicer arietinum L.) to high and low yielding environments of India. Field Crops Res. 98, 230–244. doi: 10.1016/j.fcr.2006.02.007
Berger, J. D., Turner, N. C., Siddique, K. H. M., Knights, E. J., Brinsmead, R. B., Mock, I., et al. (2004). Genotype by environment studies across Australia reveal the importance of phenology for chickpea (Cicer arietinum L.) improvement. Australian J. Agric. Res. 55, 1071–1084. doi: 10.1071/ar04104
Bezaweletaw, K., Belete, K., and Sripichitt, P. (2006). Genetic gain in grain yield potential and associated agronomic traits in haricot bean (Phaseolus vulgaris L.). Agric. Nat. Resour. 40, 835–847.
Bogale, D. A., Mekbib, F., and Fikre, A. (2015). Genetic improvement of lentil (Lens culinaris Medikus) between 1980 and 2010 in Ethiopia. Malaysian J. Med. Biol. Res. 2, 284–292. doi: 10.18034/mjmbr.v4i2.437
Erskine, W., Sarker, A., and Kumar, S. (2011). Crops that feed the world 3. Investing in lentil improvement toward a food secure world. Food Sec. 3:127. doi: 10.1007/s12571-011-0124-5
Erskine, W., Tufail, M., Russell, A., Tyagi, M. C., Rahman, M. M., and Saxena, M. C. (1994). Current and future strategies in breeding lentil for resistance to biotic and abiotic stresses. Euphytica 73, 127–135. doi: 10.1007/bf00027189
Fischer, R. A., Byerlee, D., and Edmeades, G. O. (2014). Crop Yields and Global Food Security. Will Yield Increase Continue to Feed the World?. Canberra: ACIAR.
Gowda, C. L. L., Upadhyaya, H. D., Dronavalli, N., and Singh, S. (2011). Identification of large-seeded high-yielding stable kabuli chickpea germplasm lines for use in crop improvement. Crop Sci. 51, 198–209. doi: 10.2135/cropsci2010.01.0078
Greenland, S. (2019). Valid p-values behave exactly as they should: some misleading criticisms of p-values and their resolution with s-values. Am. Stat. 73, 106–114. doi: 10.1080/00031305.2018.1529625
Hanlan, T., Ball, R., and Vandenberg, A. (2006). Canopy growth and biomass partitioning to yield in short-season lentil. Canadian J. Plant Sci. 86, 109–119. doi: 10.4141/P05-029
Idrissi, O., Sahri, A., Houasli, C., and Nsarellah, N. (2019). Breeding progress, adaptation, and stability for grain yield in moroccan lentil improved varieties. Crop Sci. 59, 925–936. doi: 10.2135/cropsci2018.07.0431
Inder, P., Materne, M., Taylor, P. W. J., and Ford, R. (2008). Genotyping elite genotypes within the Australian lentil breeding program with lentil-specific sequenced tagged microsatellite site (STMS) markers. Australian J. Agric. Res. 59, 222–225. doi: 10.1071/AR07188
Koester, R. P., Skoneczka, J. A., Cary, T. R., Diers, B. W., and Ainsworth, E. A. (2014). Historical gains in soybean (Glycine max Merr.) seed yield are driven by linear increases in light interception, energy conversion, and partitioning efficiencies. J. Exp. Botany 65, 3311–3321. doi: 10.1093/jxb/eru187
Kumar, J., Basu, P., Srivastava, E., Chaturvedi, S., Nadarajan, N., and Kumar, S. (2012). Phenotyping of traits imparting drought tolerance in lentil. Crop Pasture Sci. 63, 547–554. doi: 10.1071/CP12168
Kusmenoglu, I., and Muehlbauer, F. J. (1998). Genetic variation for biomass and residue production in lentil: I. Relation to agronomic traits. Crop Sci. 38, 907–910. doi: 10.2135/cropsci1998.0011183X003800040002x
Lake, L., Chauhan, Y. S., Ojeda, J. J., Cossani, C. M., Thomas, D., Hayman, P. T., et al. (2021). Modelling phenology to probe for trade-offs between frost and heat risk in lentil and faba bean. Eur. J. Agronomy 122:126154. doi: 10.1016/j.eja.2020.126154
Lake, L., Chenu, K., and Sadras, V. O. (2016). Patterns of water stress and temperature for Australian chickpea production. Crop. Pasture Sci. 67, 204–215. doi: 10.1071/CP15253
Lake, L., and Sadras, V. O. (2021). Lentil yield and crop growth rate are coupled under stress but uncoupled under favourable conditions. Eur. J. of Agronomy 126:126266. doi: 10.1016/j.eja.2021.126266
Llewellyn, R. S., D’Emden, F. H., and Kuehne, G. (2012). Extensive use of no-tillage in grain growing regions of Australia. Field Crops Res. 132, 204–212. doi: 10.1016/j.fcr.2012.03.013
Malhi, S. S., Johnston, A. M., Schoenau, J. J., Wang, Z. H., and Vera, C. L. (2007). Seasonal biomass accumulation and nutrient uptake of pea and lentil on a black chernozem soil in Saskatchewan. J. Plant Nutr. 30, 721–737. doi: 10.1080/01904160701289578
Mao, D., Paull, J., Preston, C., Yang, S. Y., Sutton, T., Michelmore, S., et al. (2015). Improving herbicide tolerance in pulse crops. Eyre Peninsula Farming Syst. 2015, 74–77.
McMurray, L. S., Preston, C., Vandenberg, A., Mao, D., Oldach, K. H., Meier, K. S., et al. (2019). Development of high levels of metribuzin tolerance in lentil. Weed Sci. 67, 83–90. doi: 10.1017/wsc.2018.57
Moodie, M., and Brand, J. (2019). “The expanding pulse _Adapting pulses to Mallee environments,” in Proceedings from the Australian Pulse Conference, (Horsham: Vic).
Muehlbauer, F. J. (1974). Seed yield components in lentils 1. Crop Science 14, 403–406. doi: 10.2135/cropsci1974.0011183X001400030019x
Muehlbauer, F. J., Mihov, M., Vandenberg, A., Tullu, A., and Materne, M. (2009). “Improvement in Developed Countries,” in The Lentil: Botany, Production and Uses, eds W. Erskine, F. J. Muehlbauer, A. Sarker, and B. Sharma (Wallingford: CAB International 2009), 137–154.
Patrignani, A., and Ochsner, T. E. (2015). Canopeo: a powerful new tool for measuring fractional green canopy cover. Agronomy J. 107, 2312–2320. doi: 10.2134/agronj15.0150
Sadras, V. O., Hayman, P. T., Rodriguez, D., Monjardino, M., Bielich, M., Unkovich, M., et al. (2016). Interactions between water and nitrogen in Australian cropping systems: physiological, agronomic, economic, breeding and modelling perspectives. Crop Pasture Sci. 67, 1019–1053. doi: 10.1071/CP16027
Sadras, V. O., Lake, L., Chenu, K., McMurray, L. S., and Leonforte, A. (2012). Water and thermal regimes for field pea in Australia and their implications for breeding. Crop Pasture Sci. 63, 33–44. doi: 10.1071/CP11321
Sadras, V. O., and Lawson, C. (2011). Genetic gain in yield and associated changes in phenotype, trait plasticity and competitive ability of South Australian wheat varieties released between 1958 and 2007. Crop Pasture Sci. 62, 533–549. doi: 10.1071/cp11060
Siddique, K., Belford, R., Perry, M., and Tennant, D. (1989). Growth, development and light interception of old and modern wheat cultivars in a Mediterranean-type environment. Australian J. Agric. Res. 40, 473–487. doi: 10.1071/AR9890473
Silim, S., Saxena, M., and Erskine, W. (1993). Adaptation of lentil to the Mediterranean environment. I. Factors affecting yield under drought conditions. Exp. Agric. 29, 9–19. doi: 10.1017/S0014479700020366
Specht, J. E., and Williams, J. H. (1984). “Contribution of genetic technology to soybean productivity — retrospect and prospect,” in Genetic Contributions to Yield Gains of Five Major Crop Plants”, ed. W. R. Fehr (New York, NY: American Society of Agronomy), 49–74. doi: 10.2135/cssaspecpub7.c3
Suhre, J. J., Weidenbenner, N. H., Rowntree, S. C., Wilson, E. W., Naeve, S. L., Conley, S. P., et al. (2014). Soybean yield partitioning changes revealed by genetic gain and seeding rate interactions. Agronomy J. 106, 1631–1642. doi: 10.2134/agronj14.0003
Summerfield, R. J., Roberts, E. H., Erskine, W., and Ellis, R. H. (1985). Effects of temperature and photoperiod on flowering in lentils (Lens culinaris Medic.). Ann. Botany 56, 659–671. doi: 10.1093/oxfordjournals.aob.a087055
Tadesse, M., Funga, A., Fikre, A., Degefu, T., Eshete, M., Korbu, L., et al. (2018). Breeding progress for grain yield and yield related characters of Kabuli chickpea (Cicer arietinum L.) in Ethiopia using regression analysis. J. Agric. Sci. 10, 1–11. doi: 10.5539/jas.v10n2p195
Tamagno, S., Sadras, V. O., Ortez, O. A., and Ciampitti, I. A. (2020). Allometric analysis reveals enhanced reproductive allocation in historical set of soybean varieties. Field Crops Res. 248:107717. doi: 10.1016/j.fcr.2020.107717
Thomson, B., Siddique, K., Barr, M., and Wilson, J. (1997). Grain legume species in low rainfall Mediterranean-type environments I. Phenology and seed yield. Field Crops Res. 54, 173–187. doi: 10.1016/S0378-4290(97)00048-8
Unkovich, M., Baldock, J., and Forbes, M. (2010). “Variability in harvest index of grain crops and potential significance for carbon accounting: examples from Australian agriculture,” in Advances in Agronomy, Vol. 105, ed. D. L. Sparks (Amsterdam: Elsevier), 173–219. doi: 10.1016/S0065-2113(10)05005-4
Voldeng, H., Cober, E., Hume, D., Gillard, C., and Morrison, M. (1997). Fifty-eight years of genetic improvement of short-season soybean cultivars in Canada. Crop Sci. 37, 428–431. doi: 10.2135/cropsci1997.0011183X003700020020x
Keywords: crop growth rate, biomass, genetics, harvest index, phenology, phenotype
Citation: Sadras VO, Rosewarne GM and Lake L (2021) Australian Lentil Breeding Between 1988 and 2019 Has Delivered Greater Yield Gain Under Stress Than Under High-Yield Conditions. Front. Plant Sci. 12:674327. doi: 10.3389/fpls.2021.674327
Received: 01 March 2021; Accepted: 13 April 2021;
Published: 02 June 2021.
Edited by:
Aditya Pratap, Indian Institute of Pulses Research (ICAR), IndiaReviewed by:
Harsh Kumar Dikshit, Indian Agricultural Research Institute (ICAR), IndiaCopyright © 2021 Sadras, Rosewarne and Lake. This is an open-access article distributed under the terms of the Creative Commons Attribution License (CC BY). The use, distribution or reproduction in other forums is permitted, provided the original author(s) and the copyright owner(s) are credited and that the original publication in this journal is cited, in accordance with accepted academic practice. No use, distribution or reproduction is permitted which does not comply with these terms.
*Correspondence: Lachlan Lake, bGFjaGxhbi5sYWtlQHNhLmdvdi5hdQ==
Disclaimer: All claims expressed in this article are solely those of the authors and do not necessarily represent those of their affiliated organizations, or those of the publisher, the editors and the reviewers. Any product that may be evaluated in this article or claim that may be made by its manufacturer is not guaranteed or endorsed by the publisher.
Research integrity at Frontiers
Learn more about the work of our research integrity team to safeguard the quality of each article we publish.