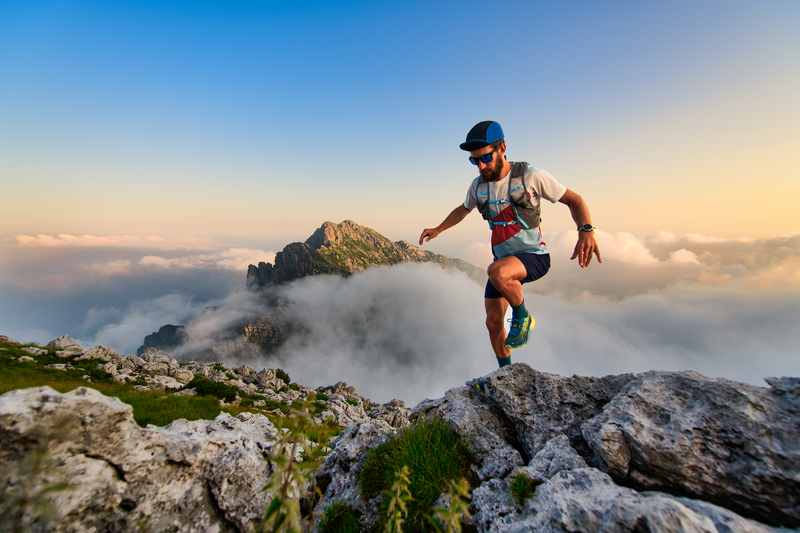
95% of researchers rate our articles as excellent or good
Learn more about the work of our research integrity team to safeguard the quality of each article we publish.
Find out more
ORIGINAL RESEARCH article
Front. Plant Sci. , 25 May 2021
Sec. Plant Cell Biology
Volume 12 - 2021 | https://doi.org/10.3389/fpls.2021.668792
This article is part of the Research Topic Stomatal Biology and Beyond View all 16 articles
Drought causes a major constraint on plant growth, development, and crop productivity. Drought stress enhances the synthesis and mobilization of the phytohormone abscisic acid (ABA). Enhanced cellular levels of ABA promote the production of reactive oxygen species (ROS), which in turn induce anion channel activity in guard cells that consequently leads to stomatal closure. Although Cyclophilins (CYPs) are known to participate in the biotic stress response, their involvement in guard cell ABA signaling and the drought response remains to be established. The Arabidopsis thaliana gene ROC3 encodes a CYP. Arabidopsis roc3 T-DNA mutants showed a reduced level of ABA-activated S-type anion currents, and stomatal closure than wild type (WT). Also, roc3 mutants exhibited rapid loss of water in leaf than wild type. Two complementation lines of roc3 mutants showed similar stomatal response to ABA as observed for WT. Both complementation lines also showed similar water loss as WT by leaf detached assay. Biochemical assay suggested that ROC3 positively regulates ROS accumulation by inhibiting catalase activity. In response to ABA treatment or drought stress, roc3 mutant show down regulation of a number of stress responsive genes. All findings indicate that ROC3 positively regulates ABA-induced stomatal closure and the drought response by regulating ROS homeostasis and the expression of various stress-activated genes.
Drought stress causes a major constraint on plant growth, development, and productivity (Langridge and Reynolds, 2015). Stomata are surrounded by pairs of specialized epidermal cells termed guard cells, and which are essential for controlling gas exchange (carbon dioxide and oxygen) and water loss. Plants have the ability to adapt to drought stress by regulating stomatal closure (Agurla et al., 2018). The turgor and volume of the pair of guard cells determine the extent of the stomata's aperture (Schroeder et al., 2001). Various environmental cues regulate stomatal movement, but at the cellular level the most critical factor is the phytohormone abscisic acid (ABA) (Raghavendra et al., 2010; Kollist et al., 2014; Osakabe et al., 2014; Murata et al., 2015). During moisture deficiency, ABA synthesis in the leaf vasculature is enhanced and the hormone is transported to guard cells (Seo and Koshiba, 2011; Munemasa et al., 2015). The accumulation of ABA activates S-type anion channels in guard cells, resulting in an efflux of anions and a consequent decreases in guard cells' turgor, which eventually induces stomatal closure (Li et al., 2000; Wang et al., 2001; Vahisalu et al., 2008).
The simultaneous functions of many signaling molecules in guard cells provide stomatal defense response against drought stress. Reactive oxygen species (ROS) are major secondary messengers which play a crucial role in ABA-triggered stomatal closure (Pei et al., 2000; Zhang et al., 2001; Kwak et al., 2003; Bright et al., 2006; Miao et al., 2006; Murata et al., 2015). Drought stress induces the accumulation of ABA in guard cells, subsequently that leads to the activation of NADPH oxidase and the promotion of ROS production. The elevated level of ROS can also enhance the level of NO and cytosolic calcium. As a result, plasma membrane localized anion channels in guard cells are activated, consequently that leads to the efflux of anions and stomatal closure (Pei et al., 2000; Schroeder et al., 2001; Kwak et al., 2003; Mori et al., 2006; Munemasa et al., 2015). However, oxidative stress is induced in response to high concentration of ROS that causes injuries in plant cells. Hence plant cells are equipped with the antioxidant systems composed of superoxide dismutase (SOD), glutathione peroxidase (GPX), ascorbate peroxidase (APX), catalase (CAT), and non-enzymatic antioxidants to control the homeostasis of ROS (Willekens et al., 1997; Corpas et al., 2001; Mittler, 2002; Apel and Hirt, 2004; Nyathi and Baker, 2006; Palma et al., 2009; Jannat et al., 2011). However, uncontrolled action of antioxidant systems could reduce appropriate levels of ROS that function as secondary messengers, which would negatively impact the ABA and/or drought signal transduction systems. It is unknown how antioxidant systems are regulated during ABA and drought signaling to maintain the suitable levels of ROS in plants.
The Cyclophilins (CYPs) belong to a large class of proteins, referred to as the immunophilins. This class of protein is widely distributed across both prokaryotes and eukaryotes (Handschumacher et al., 1984; Wang et al., 2005; Kim et al., 2012). Plant CYPs have been shown to participate in a diversity of physiological processes, including protein folding, transcriptional regulation, and stress response (Santos and Park, 2019). For example, the rice protein CYP18-2, regulates the transcription and post-transcriptional modification of a number of stress-related genes (Lee et al., 2015), and over-expression of rice CYP19-4 could enhance the plant cold tolerance as well (Yoon et al., 2016). There are 29 genes predicted to encode CYP or CYP-like proteins in the Arabidopsis thaliana genome (He et al., 2004). The ROC3 (also known as AtCYP19-1) has been shown to boost the plant's ability to withstand infection by the pathogen Pseudomonas syringae (Pogorelko et al., 2014). The present experiments were designed to explore whether ROC3 also plays any roles in drought stress tolerance and ABA signaling in A. thaliana.
The A. thaliana, Columbia-0 (Col-0) was used as the wild-type (WT) for all experiments in this study. Seeds of the two T-DNA insertion mutants, roc3-1 (SALK_063724c) and -2 (SALK_095698c), in Columbia-0 background, were obtained from Arabidopsis Biological Resource Center (http://abrc.osu.edu/). The homozygous status of both T-DNA mutants was verified using a PCR assay (primer sequences are given in Supplementary Table 1). Seeds were surface sterilized in 75% v/v ethanol for 3 min, followed by 1 min in 95% v/v ethanol and then dried in air. The sterilized seeds were plated on half strength Murashige and Skoog (1962) medium (1/2 MS) containing 0.7% w/v agar. The plates were kept in the dark for 3 days at 4°C for vernalization, then transferred to a controlled growth chamber (~70% relative humidity) for 7–10 days, with 16 h photoperiod (100 μmol m−2s−1 fluorescent lamp light) and day/night temperature regime of 22 ± 1°C/16 ± 4°C. Thereafter, the seedlings were transplanted to pots containing mixture of soil and vermiculite (2:1 (v/v), and transferred back to the growth chamber.
The ROC3 native promoter, 1854 bp fragment upstream of the initiation codon (from CTTCTCACAT to AAAAAAAGAA of WT genomic DNA), was PCR-amplified from WT genomic DNA using the primer pair ROC3-GUS-F/R (sequences are given in Supplementary Table 1) and the amplicon was inserted into the HindIII and SmaI cloning sites of the pCambia-ubiGUS vector to generate the construct pROC3::GUS. The recombinant plasmid was introduced into Agrobacterium tumefaciens strain GV3101, then transformed into Arabidopsis via Agrobacterium-mediated transformation using the floral dip technique (Clough and Bent, 1998). pROC3::GUS activity was detected in seedling (2-week-old), root (2-week-old), leaf (2-week-old), flower (6-week-old), silique (8-week-old), and guard cells (4-week-old) of transgenic plants. The described plant tissues were immersed for ~3 h at 37°C in 50 mM sodium phosphate buffer (pH 7.2) containing 2 mM X-Gluc, 2 mM K3Fe(CN)6, 2 mM K4Fe(CN)6, 0.1% v/v Triton X-100, and 10 mM EDTA. Subsequently, the samples were incubated in absolute ethanol to remove chlorophyll and then inspected under a stereomicroscope (SZX2-ILLT; OLYMPUS, Tokyo, Japan). For ABA treatment tests, the samples were treated with 50 μM ABA or absolute ethanol (solvent control) respectively for 2.5h before staining. Relative GUS activity was quantified from three independent biological replicates using ImageJ open source software (v. 1.37, https://imagej.nih.gov/ij/).
To generate the construct p35S::ROC3-GFP, the full length cDNA of ROC3 was PCR-amplified from WT cDNA using the primer pair ROC3-GFP-F/R (Supplementary Table 1) and the amplicon inserted into the BamHI and SalI sites of the pBI221-GFP vector, with the GFP in the carboxyl terminus (Lin et al., 2009). The plasmids p35S::GFP, p35S::ROC3-GFP and p35S::AHL22-RFP (used as a nuclear localization marker) (Xiao et al., 2009) were isolated with NucleoBond® Xtra Midi Kit (Macherey-Nagel, Germany) and transfected into Arabidopsis mesophyll protoplasts (Sheen, 2001). The protoplast cultures were incubated in the dark for 16 h at 23°C and then fluorescence was assessed using laser scanning confocal microscopy (LSM880; Carl Zeiss, Oberkochen, Germany). The GFP signal was detected at excitation wavelengths of 488 and emission between 495 and 540 nm. The excitation of RFP was conducted at 543 nm, with emission being captured between 580 and 620 nm.
The bioassay for stomatal aperture was performed as reported by Li et al. (2016) with a slight modification. In short, the leaves of 4-week-old plants were excised and incubated in closure buffer (20 mM KCl, 1 mM CaCl2, 5 mM MES-KOH, pH 6.15) at 23°C for 2.5 h in light (100 μmol m−2s−1 fluorescent lamp light), then added ABA (1, 10, 50 μM), 20 mM 3-amino-1,2,4-triazole (AT, an inhibitor of catalase) (Jannat et al., 2011), 100 μM H2O2 or absolute ethanol (solvent control) respectively for an additional 2.5 h in the same incubation place and condition. Subsequently, abaxial epidermal strips were peeled off and immediately photographed by a light inverted microscope. Stomatal aperture width and length were measured by the open access software ImageJ (v1.37, https://imagej.nih.gov/ij/). Each experiment included at least three biological replicates, with no fewer than 60 guard cells that were measured per each sample. The Student′s t-test was used to determine whether differences between mean values were statistically significant.
Seedlings were potted into the soil/vermiculite mixture and grown for about 4 weeks in well-watered condition, then water was withheld for 3 weeks. At the end of the treatment, the plants were re-watered over a 3 d period and photographed. Water loss assay from detached rosette leaves was sampled from 4-week-old well-watered plants. Detached rosette leaves were placed on filter paper in the light at room temperature and measured by weighing the leaves every 30 min over a 3 h period to measure the rate of water loss. The entire experiment was performed at least three biological replicates in the controlled growth chamber under the same light condition.
Two-week-old seedlings grown on a half-strength Murashige-Skoog (1/2 MS) medium (0.7% w/v agar) were incubated in liquid 1/2 MS for 24 h in a growth chamber (~70% relative humidity, 16 h photoperiod, 100 μmol m−2s−1 fluorescent lamp light, 22 ± 1°C/16 ± 4°C). Then the first set of seedlings was transferred to 1/2 MS liquid medium containing ABA (final concentration 50 μM); and a second set of seedlings was exposed on filter paper for drought treatment. Both ABA and drought treated seedlings, together with their control samples, were placed in the controlled growth chamber. Treated samples and their controls were collected at the same time after treatments, and snap-frozen in liquid nitrogen. RNA extracted from the frozen seedlings using the TRIzol reagent (Sigma–Aldrich, St. Louis, United States), was used to synthesize cDNA using a 5X All-In-One MasterMIX (with an AccuRT Genomic DNA Removal Kit (ABM, Canada). A quantitative real time (qRT)-PCR assay was performed using the FastStart Universal SYBR Green master mix (Roche, Basel, Switzerland) and the ROC3-qRT-F/R and ACTIN2-qRT-F/R primer pairs (Supplementary Table 1). qRT-PCR was also performed to quantify the transcript abundances of various genes encoding ROS signaling enzymes and stress-responsive proteins using specific primer pairs (Supplementary Table 1). All the quantitative analyses included three independent biological replicates, and each replicate contained three technical duplicates. ACTIN2 used as the internal reference. The reaction steps were firstly pre-incubated at 95°C for 300 s, then ran 40 cycles with 95°C for 15 s, 58°C for 15 s, and 72°C for 20 s. CFX ConnectTM Real-Time PCR Detection System (Bio-Rad, California, U.S.A.) was used and the data was quantified by the ΔΔCt method.
The ROC3 open reading frame was PCR-amplified from Col-0 cDNA using the primer pair ROC3-C-F/R (Supplementary Table 1). The amplicon was inserted into the SmaI and SacI cloning sites of pROC3::GUS vector to generate the transgene construct pROC3::ROC3. Agrobacterium tumefaciens (strain GV3101) was transformed using this construct. The roc3-1 and roc3-2 mutants were transformed via Agrobacterium mediated transformation using the floral dip method (Clough and Bent, 1998). Transformants were selected on 1/2 MS Agar medium containing 30 mg/L hygromycin. ROC3 complementation lines generated from roc3-1 and roc3-2 mutants were named as C-1 and C-2, respectively.
A. thaliana guard cell protoplasts were isolated as described previously (Zhang et al., 2008) with slight modifications. In brief, 10–12 rosette leaves from 4-week-old plants were cut off, and epidermal strips were peeled off. Then, the peeled epidermal strips were blended in a blender filled with 750 mL cold distilled water for 30 s, and filtered through a 100-μm nylon mesh and placed in a 10 mL beaker filled with 2 mL enzyme solution I [0.7% Cellulysin cellulase, 0.1% PVP-40, 0.25% BSA in 55% basic solution (5 mM MES, 0.5 mM CaCl2, 0.5 mM MgCl2, 0.5 mM ascorbic acid, 10 μM KH2PO4, 0.55 M sorbitol, pH 5.5)]. The beaker was placed in a water bath shaker and shook at 80 rpm for 30 min at 28°C, then 2 mL basic solution was added to enzyme solution I and shook for another 10 min. After that, the strips were filtered through a 100-μm nylon mesh and placed in beaker containing 2 mL enzyme solution II (1.5% Onuzuka cellulase RS, 0.01% cellulase Y-23, 0.25% BSA in 100% basic solution), and shaking was continued at 60 rpm for at least 15 min. Subsequently, the materials were mixed by pipetting up and down with a 1-mL pipette and filtered through a 30-μm nylon mesh. The protoplasts were centrifuged at 800 rpm for 5 min and washed twice with basic solution.
The whole-cell mode patch-clamp electrophysiology tests were carried out as described in the previous articles (Schroeder and Hagiwara, 1989; Pei et al., 1997; Vahisalu et al., 2008; Acharya et al., 2013). For anion current recordings, the bath solution contained 2 mM MgCl2, 30 mM CsCl, 1 mM CaCl2, 10 mM MES-Tris (pH 5.6) and the osmolarity of this solution was adjusted to 480 mOsm with sorbitol. The pipette solution contained 150 mM CsCl, 2 mM MgCl2, 6.7 mM EDTA, 3.35 mM CaCl2, and 10 mM HEPES (pH 7.5), the osmolarity of this solution was adjusted to 500 mOsm with sorbitol. ATP (10 mM Mg-ATP) and GTP (10 mM) were added to it before experiments. The whole-cell currents were recorded using the Axopath-200B amplifier (Molecular Devices, Downingtown, PA, USA) after the whole-cell configuration was achieved. The holding potential was +30 mV and voltage steps were applied from −145 to +35 mV with +30 mV increments, and each test voltage lasted 60 s. For ABA treatment tests, guard cell protoplasts were treated with 50 μM ABA for at least 1h before measurement. In order to obtain the currents and draw the current density voltage plots, pCLAMP software (version10.2; Axon Instruments, Sunnyvale, CA, USA) and SigmaPlot 12.0 (Systat Software, Richmond, CA, USA) were used respectively.
Quantification ROS content of both WT and roc3 guard cells was performed using the fluorescent dye CM-H2DCFDA (Thermo Fisher, Waltham, MA, USA) as described previously with slight modifications (Miao et al., 2006; Zhang et al., 2011). In order to open the stomata, abaxial epidermis strips were prepared from the leaves of 4-week-old plants and immersed in 1 mM CaCl2, 20 mM KCl, 5 mM MES-KOH (pH 6.15) for 2.5 h in the light; then added 50 μM ABA or absolute ethanol as solvent control and the incubation continued for an additional 2.5 h. At the end of this period, the epidermal peels were transferred to aqueous 50 μM CM-H2DCFDA for 10 min in the dark, then rinsed at least three times in distilled water to remove excess dye. The H2DCFDA fluorescence was captured using laser scanning confocal microscope and inverted fluorescence microscope (TI-1; NIKON, Tokyo, Japan), and the fluorescence intensity was quantified by using ImageJ software.
To measure the leaf H2O2 content, the leave samples (4-week-old) were first weighed and immersed in liquid buffer (20 mM KCl, 1 mM CaCl2, 5 mM MES-KOH, pH 6.15) for 2.5 h, then added 50 μM ABA or absolute ethanol (solvent control), and the incubation continued for a additional 2.5 h. Snap-frozen leaf material was ground to a powder and processed using a commercial H2O2 content determination kit (Comin, Suzhou, China) according to the supplier's protocol. Finally, 200 μL aliquots of these reaction mix were pipetted into plates, and their absorbance at 415 nm was measured; H2O2 content was calculated according to the formula: H2O2 (μmol/g) = 2.67 × (ΔA − 0.0006) ÷ W (ΔA = Asample − −Ablank, W:sample weight).
Catalase assay were performed using a CAT detection kit (Comin, Suzhou, China). The samples were treated as described above in the method of H2O2 content assay. Then catalase extraction was performed according to the manufacturers' instruction. To quantify catalase, 10 μL aliquot of the extract added to 190 μL of Comin catalase reaction solution in a 96-well UV plate. The reactions' absorbance at 240 nm was recorded immediately (A1) and subsequently after 1 min (A2). Catalase activity was calculated on the basis of the formula: CAT (nmol/min/g) = 918 × ΔA ÷ W (ΔA = A1 − −A2, W:sample weight).
Gene expression profiles of ROC3 showed that the gene was induced by both ABA treatment and drought stress (Figures 1A,B). To test spatial expression of ROC3 promoter, histochemical analysis was performed in transgenic plants expressing pROC3::GUS construct. GUS expression was observed in seedling, leaf, flower, and silique (Figures 1D–G). Besides, ROC3 promoter was mildly induced in the guard cells of plants treated with ABA (Figures 1C,H,I). Transient expression of GFP-tagged ROC3 (p35S::ROC3-GFP) in Arabidopsis mesophyll protoplasts showed that ROC3 located in both the cytoplasm and the nucleus (Figure 1J). To further confirm the nucleus localization of ROC3, the p35S::ROC3-GFP was co-transformed into protoplasts with the p35S::AHL22-RFP (AHL22, a known nucleus protein), and the GFP and RFP signals substantially overlapped in the nucleus (Figure 1J).
Figure 1. Transcriptional and expression profiling of ROC3. (A,B) Relative transcript abundances assessed using qRT-PCR in seedlings subjected to (A) ABA treatment, (B) drought stress. (C) Relative GUS activity of pROC3::GUS in guard cells. Error bars represent the SE (n = 30), **: means differed significantly from control (P < 0.01). The experiments were repeated three times with similar results. (D–I) GUS staining reveals the tissue expression of pROC3::GUS transgene in (D) the whole seedling (bar: 1.5 mm), (E) the leaf (bar: 0.3 mm), (F) the flower (bar: 0.3 mm), (G) the silique (bar: 1 mm) and (H,I) guard cells in plants (H) not exposed to ABA (bar: 10 μm), (I) exposed to 50 μM ABA (bar: 10 μm). (J) The topological expression of p35S::GFP, p35S::ROC3-GFP, and p35S::AHL22-RFP in protoplasts (bar: 10 μm).
Since the expression of ROC3 could be induced by ABA (Figure 1A) and pROC3::GUS was expressed in guard cells (Figures 1H,I), we hypothesized that ROC3 may play a role in ABA-regulated stomatal movement. Two independent T-DNA insertion roc3 mutants (Figures 2A,B; Supplementary Figure 1) were used to examine the role of ROC3 in stomatal closure. Under control conditions, stomatal apertures did not show significant difference between either of the roc3 mutants and WT, but when leaves were treated with a range of ABA concentrations (1–50 μM), the aperture of the mutants' stomata was clearly bigger than that of WT stomata (Figure 2C). Similarly, the rate of water loss from roc3 mutant excised rosette leaves was higher than WT plants (Figure 2D), while the survival rate of soil-grown roc3 mutant plants was lower than that of WT plants after rehydration (Figures 2E,F).
Figure 2. Stomatal closure in ROC3 knock-down mutants is induced by exposure to ABA and the plants exhibit a lesser level of drought tolerance. (A) The T-DNA insertion points in the two independent roc3 mutants. (B) Relative ROC3 transcript abundances assessed using qRT-PCR in WT and roc3 seedlings. Values shown in the form mean ± SE (n = 3). (C) ABA-induced stomatal closure, as quantified by the stomatal width/length ratio measured in at least 60 stomata per genotype per replicate. Error bars represent the SE (n = 60), **: means differed significantly from WT (P < 0.01). (D) The rate of water loss from detached rosette leaves of WT and roc3 mutants. Values shown in the form mean ± SE (n = 3), *: means differed significantly from WT (P < 0.05). (E) The appearance of WT and roc3 mutant plants grown under conditions of drought stress. (F) The survival rate of WT and roc3 mutant plants grown under conditions of moisture stress. Data are shown as means ± SE (n = 8).
To further confirm the function of ROC3 in stomatal regulation and drought response, we generated transgenic plants harboring pROC3::ROC3 in roc3 mutant backgrounds. The abundance of ROC3 transcript in the ROC3 complementation lines, C1 and C2, was similar as in WT plants (Figure 3A). When C-1 and C-2 line plants were assessed either for their water loss from detached rosette leaves or their growth response to drought stress, their performance was almost indistinguishable from that of WT plants (Figures 3B,D,E). Similarly, stomatal aperture was similar between ROC3 complementation lines and WT plants upon ABA treatment (Figure 3C).
Figure 3. The insertion of the pROC3::ROC3 transgene rescues the roc3 mutants' phenotype. (A) Relative ROC3 transcript abundances assessed using qRT-PCR in WT and the complementation lines C-1 and C-2. Error bars represent the SE (n = 3). (B) The rate of water loss from rosette leaves detached from WT, C-1, and C-2 plants. Values shown in the form mean ± SE (n = 3). (C) ABA-induced stomatal closure in the WT, C-1, and C-2 leaf epidermis, stomatal aperture width/length ratios measured in at least 60 stomata per genotype per replicate. Data are shown as means ± SE (n = 60). (D) The survival rate of WT, C-1, and C-2 plants growing under drought stress conditions. Values shown in the form mean ± SE (n = 3). (E) The appearance of drought-stressed WT, C-1, and C-2 plants.
Previous research findings described that the activation of S-type anion channels in guard cell plasma membrane can lead to anion outflow, change in guard cell turgor, and finally promote stomatal closure (Vahisalu et al., 2008; Kim et al., 2010; Zhang et al., 2018). To investigate whether ROC3 positively regulates ABA-induced stomatal closure through the activation of S-type anion channels, we examined the activity of S-type anion channel currents in guard cells. Under control conditions, no difference was observed for S-type anion channel currents in the guard cells of WT, roc3 mutants and the two ROC3 complementation lines. In contrast, in response to ABA, ABA-activated of anion currents was clearly smaller in the roc3 mutants than in either WT or the C-1 and C-2 lines (Figures 4A,B).
Figure 4. ROC3 is involved in the ABA-induced activation of anion channels in guard cells. (A) Patch-clamp whole cell recordings of S-type anion currents present in guard cell protoplasts isolated from WT, roc3 mutants and the C-1 and C-2 complementation lines, incubated in the presence/absence of 50 μM ABA. (B) Average current-voltage relationships of whole cell S-type anion currents. The number of guard cells measured were: WT (5), WT+ABA (6), roc3-1 (4), roc3-1+ABA (6), roc3-2 (5), roc3-2+ABA (6), C-1 (4), C-1+ABA (6), C-2 (4), C-2+ABA (5). Values shown in the form mean ± SE.
ROS are crucial messenger molecules that participate in ABA regulation of anion channels and stomatal movement (Pei et al., 2000; Zhang et al., 2001; Munemasa et al., 2007). We wanted to know whether ROC3 plays any regulatory roles in ABA-triggered ROS accumulation. Firstly, a fluorescence-based assay was used to measure the ROS content of WT and roc3 guard cells. In the control condition, the guard cells of roc3 showed reduced accumulation of ROS compared to WT. In response to ABA, the guard cells of WT showed higher accumulation of ROS compared to the guard cells of roc3 (Figures 5A,B; Supplementary Figure 2). Similarly, H2O2 levels were lower in ABA-treated roc3 than in ABA-treated WT plants (Figure 5C). After ABA treatment, the absence of ROC3 had no obvious effect on the transcripts of two NADPH oxidase genes, RbohD and RbohF, which are involved in the ABA induced ROS production (Supplementary Figure 3). However, there was a moderate effect on the expression of both CAT1 and CAT2, genes encode catalases which act as ROS scavengers: the expression of both genes was greater in roc3 mutants than in WT plants upon ABA treatment (Figures 5D,E). Similarly, leaf catalase activity was higher in the mutants' leaves than WT in response to ABA treatment (Figure 5F).
Figure 5. The accumulation of ROS is less efficient in roc3 mutants than in WT guard cells. (A) Laser confocal micrographs revealing the ROS content of guard cells sampled from WT or roc3 mutant plants either exposed or not exposed to ABA; the fluorescent signal is generated from CM-H2DCFDA. (B) Quantification of the fluorescence intensities shown in (A). At least 100 guard cells were sampled from each genotype. Error bars represent the SE (n = 100). The experiments were repeated three times with similar results. (C) The H2O2 content in the leaf of WT and roc3 plants, either exposed or not exposed to 50 μM ABA. Values shown in the form mean ± SE (n = 3). (D,E) Relative transcript abundances assessed using qRT-PCR in plants either exposed or not exposed to ABA: (D) CAT1, (E) CAT2. Data are shown as means ± SE (n = 3). (F) Quantification of catalase activity assay in WT and roc3 mutants, either exposed or not exposed to 50 μM ABA. Values shown in the form mean ± SE (n = 3). *,**: values differed significantly from WT (P < 0.05, 0.01).
To explore whether ROC3 was involved in ABA-induced stomatal closure by affecting ROS accumulation, we carried out stomatal closure experiments with H2O2 or AT (an inhibitor of catalase) treatment, in the presence or absence of ABA. The absence of functional ROC3 had no discernible effect on H2O2-induced stomatal closure in leaf epidermis strips, but the genotypic difference in stomatal aperture induced by ABA treatment was abolished by addition of exogenous H2O2 (Figure 6A). AT treatment had little influence on the stomatal movement, but the presence of AT promoted ABA-induced stomatal closure and abolished the difference in ABA-promoted stomatal closure between WT and roc3 (Figure 6B).
Figure 6. ROC3 participates in ROS signal-regulated stomatal closure. (A) Stomatal closure assays of WT and roc3 mutant leaf epidermis treated with either ABA and/or H2O2, and stomatal aperture width/length ratios measured in at least 60 stomata per genotype per replicate. Means shown in the form mean ± SE (n = 60), **: means differed significantly from WT (P < 0.01). (B) Stomatal closure assays of WT and roc3 mutant leaf epidermis treated with ABA and/or AT, and stomatal aperture width/length ratios measured in at least 60 stomata per genotype per replicate. Values shown in the form mean ± SE (n = 60), **: means differed significantly from WT (P < 0.01). Both of the experiments were repeated three times.
Previous findings have shown that, ABA can induce the expression of many downstream key genes involved in plant dehydration stress (Yamaguchi-shinozaki and Shinozaki, 2006; Ju et al., 2020). An examination of the effect of ROC3 on the transcription of a series of genes known to participate in the stress response (RD29A, RD29B, RAB18, ABI5, ABF2, ABF3, ERD10, and COR47) showed that in each gene, transcript abundances were lower in roc3 mutant than in WT plants in response to ABA treatment or drought stress (Figure 7).
Figure 7. Transcriptional profiling of the indicated genes associated with the stress response in roc3 mutant exposed to either ABA treatment or drought stress. Relative transcript abundances assessed using qRT-PCR in plants subjected to (A) 6 h exposure to ABA, (B) 6 h exposure to drought stress. Means shown in the form mean ± SE (n = 3), *, **: means differed significantly from control treatment (P < 0.05, 0.01).
Drought exerts significant negative effects on crop productivity (Boyer, 1982; Venuprasad et al., 2007). Attempts to increase the resilience of crop varieties to this stress via conventional breeding has achieved only a modest level of success, while the potential of transgenic technology to address this issue has also been demonstrated (Manavalan et al., 2009; Hu and Xiong, 2014). The key to the success of a transgenic-based improvement strategy for a specific stress like drought is very much dependent on the specific choice of transgene(s). Therefore, the identification of genes contributing to a plant's defense against drought remains a major research priority and it is very much necessary to understand how the candidate genes affect the plant's response to the stress. Most of the water lost by plants passes through the stomata, so control on stomatal movement will have a major impact on the plant's hydration status (Schroeder et al., 2001; Martin-StPaul et al., 2017; Agurla et al., 2018). It has been established that ABA-triggered activation of guard cell anion channels results in the efflux of anions, which in turn reduces the turgor of guard cells to close the stomata (Cutler et al., 2010; Hubbard et al., 2010; Lee and Luan, 2012) and other proteins also have been implicated in this process.
The outcome of our experiments support the notion that the CYP member ROC3 of A. thaliana acts as a positive regulator of ABA-induced stomatal closure. ROC3 was found to be induced by both ABA treatment and the drought stress (Figures 1A,B). pROC3::GUS activity was detected in seedling, leaf, flower, silique, and was mildly induced in guard cells upon ABA treatment (Figures 1C–I), implying that ROC3 may play a role in guard cell ABA signaling. The stomata of roc3 mutant plants were more open than those of WT plants in ABA treatment (Figure 2C), and the mutants were also less tolerant of drought (Figures 2D–F). Supportively, ROC3 complementation lines, C1 and C2, restored the WT phenotype (Figure 3), suggesting that ROC3 plays a positive regulatory role in ABA-induced stomatal closure and the drought stress response. Plasma membrane localized anion channels are activated by the accumulation of ABA in guard cells, leading to the depolarization of plasma membrane and anion outflow which causes the reduction of guard cell turgor, that ultimately leads to stomatal closure (MacRobbie, 1998; Hedrich, 2012; Roelfsema et al., 2012; Hedrich and Geiger, 2017). A patch clamp experiment demonstrated that in protoplasts exposed to ABA, S-type anion channel activity was lower in roc3 mutants compared to WT or the ROC3 complementation line plants (Figure 4), indicating that the absence of functional ROC3 causes reduced outflow of anions from the guard cells in response to ABA, hence stomatal aperture remains larger. It remains to be explored how ROC3 influences anion channel activity in response to drought stress or ABA treatment. Further research will be conducted to analyze whether ROC3 can regulate the expression of the known genes encoding anion channels (e.g., SLAC1) or these channels activity regulation (e.g., CPK3).
It is well-known that ABA signaling in guard cells requires the participation of multiple signaling elements. Among them, ROS (notably H2O2) work as crucial secondary messengers in regulating ABA-induced stomatal closure (Hua et al., 2012; Gayatri et al., 2013; Song et al., 2014; Dietz et al., 2016; Li et al., 2017). A rise in leaf ABA content, due to endogenous processes or an exogenous ABA treatment, stimulates the production of ROS (Bright et al., 2006; Kreslavski et al., 2012). The concentration of ROS in the leaves of roc3 mutant plants exposed to ABA was less than in WT leaves (Figures 5A–C; Supplementary Figure 2), consistent with the proposed positive regulatory roles of ROC3 in both stomatal closure and the drought stress response. Interestingly, the stomatal aperture of roc3 was similar to Col-0's when simultaneously exposed to ABA and H2O2, and the hyposensitive phenotype of the roc3 mutants with respect to ABA-induced stomatal closure was abolished when supplemented with exogenous H2O2 (Figure 6A). It is possible that ROS act as downstream components during ROC3-modulated stomatal closure. Previously it has been shown that the ABA-induced production of ROS is primarily catalyzed by plasma membrane localized NADPH oxidases, while ROS neutralization is carried out by various enzymatic (notably catalase) and non-enzymatic antioxidants (Willekens et al., 1995; Zhang et al., 2001; Dietz, 2003; Kwak et al., 2003; Mittler et al., 2004; Mhamdi et al., 2010). In response to ABA, roc3 mutants showed the upregulation of both CAT1 and CAT2 compared to WT plants (Figures 5D,E), although the roc3 plants did not show any differences in the gene expression profile of either RbohD or RbohF (Supplementary Figure 3). Furthermore, higher catalase activity was observed in roc3 mutants than in WT in response to ABA (Figure 5F). These findings suggested that reduced accumulation of ROS in roc3 mutants was due to a higher level of catalase activity. This hypothesis was tested by stomatal movement experiments with a known catalase inhibitor, AT. The difference of stomatal aperture between WT and roc3 mutants in ABA treatment was abolished when AT was combined with ABA (Figure 6B). Our findings propose that by unknown mechanism ROC3 suppresses catalase activity, thereby affecting the accumulation of ROS that in turn positively regulates ABA-induced stomatal closure.
Many genes involved in the drought stress response are known to be induced by ABA treatment (Kang et al., 2002; Yamaguchi-shinozaki and Shinozaki, 2006; Kovacs et al., 2008; Liu and Stone, 2010; Ma et al., 2019; Ju et al., 2020). Comparisons of the profiles of the expression of some of these genes between roc3 and WT leaves which were either treated with ABA or subjected to drought stress indicated that their expression in response to either stress was somewhat compromised in the mutant (Figure 7), providing an additional explanation for the reduced sensitivity of roc3 to exogenous ABA or drought stress. The mechanistic basis of this interaction is unknown.
A working model summarizing the participation of ROC3 in ABA-induced stomatal closure and the drought stress response of A. thaliana is depicted in Figure 8. Drought stress promotes the accumulation of ABA in guard cells. Then, ABA induces the production of ROS and subsequently the activation of anion channels that leads to stomatal closure, thereby cutting off the major route by which water is lost from the plant. Our current findings suggest that ROC3 acts as a positive regulator in ABA-induced stomatal closure. ROC3 inhibits CAT activity, thereby maintaining a sufficient level of ROS in guard cells to ensure stomatal closure. At the same time, ROC3 also contributes to both ABA signaling and tolerance to drought stress by maintaining the expression of a number of genes that play important roles in plant's stress response. The present experimental findings have revealed a novel function for ROC3 beyond its known involvement in protein folding, the catalysis of cis-trans isomerization of proline imidic peptide bonds in oligopeptides and the response to pathogen infection. The exact mechanism remains to be elucidated how ROC3 interacts with ABA signaling machineries, how it suppresses catalase activity, and how it influences the expression of stress response genes.
Figure 8. A proposed working model for the role of ROC3 in the regulation of stomatal movement and the drought stress response of A. thaliana.
The raw data supporting the conclusions of this article will be made available by the authors, without undue reservation.
WZ designed the experiments, interpreted the results, and edited the manuscript. HL performed the major experiments, analyzed the results, and wrote the manuscript. CY and DL performed a few experiments. JS, BA, MW, and DC designed a few experiments and analyzed some data. All authors contributed to the article and approved the submitted version.
This work was financially supported by Distinguished Young Scholar of Shandong University (61200088963137), Natural Science Foundation of Shandong Province (ZR201807100168 to WZ), and National Natural Science Foundation of China (31771353 and 31471158 to MW). Cooperation project on nutritional value and health care function of special vegetable for pregnant women and infants (61200012001902 to WZ).
The authors declare that the research was conducted in the absence of any commercial or financial relationships that could be construed as a potential conflict of interest.
We thank the Arabidopsis Biological Resource Center (ABRC) for providing T-DNA insertion seeds SALK_063724c, SALK_095698c. We are also thankful to Haiyan Yu, Xiaomin Zhao, and Sen Wang from SKLMT (State Key Laboratory of Microbial Technology, Shandong University) for the assistance in microimaging of LSCM analysis.
The Supplementary Material for this article can be found online at: https://www.frontiersin.org/articles/10.3389/fpls.2021.668792/full#supplementary-material
Supplementary Table 1. Primer sequences used in the experiments.
Supplementary Figure 1. PCR screening of homozygous T-DNA mutants of ROC3. 1: roc3-1-LP+LBb1.3; 2: roc3-1-RP+LBb1.3; 3: roc3-1-LP+ roc3-1-RP; 4: roc3-2-LP+LBb1.3; 5: roc3-2-RP+LBb1.3; 6: roc3-2-LP+ roc3-2-RP; M: Marker.
Supplementary Figure 2. Fluorescence micrographs revealing the ROS content of guard cells sampled from WT or roc3 mutant plants either exposed or not exposed to ABA; the fluorescent signal is generated from CM-H2DCFDA and captured by a conventional fluorescence microscope. There are 60 guard cells were sampled from each genotype. Error bars represent the SE (n = 60), **: means differed significantly from Col-0 (P <0.01).
Supplementary Figure 3. Transcriptional profiling of two genes encoding NADPH/respiratory burst oxidase proteins. Relative transcript abundances of (A) RBOHD, (B) RBOHF, assessed using qRT-PCR, in WT or roc3 mutants either exposed or not exposed to ABA. Values shown in the form mean ± SE (n = 3).
Acharya, B. R., Jeon, B. W., Zhang, W., and Assmann, S. M. (2013). Open Stomata 1 (OST1) is limiting in abscisic acid responses of Arabidopsis guard cells. New phytol. 200, 1049–1063. doi: 10.1111/nph.12469
Agurla, S., Gahir, S., Munemasa, S., Murata, Y., and Raqhavendra, A. S. (2018). Mechanism of stomatal closure in plants exposed to drought and cold stress. Adv. Exp. Med. Biol. 1081, 215–232. doi: 10.1007/978-981-13-1244-1_12
Apel, K., and Hirt, H. (2004). Reactive oxygen species: metabolism, oxidative stress, and signal transduction. Annu. Rev. Plant Biol. 55, 373–399. doi: 10.1146/annurev.arplant.55.031903.141701
Boyer, J. S. (1982). Plant productivity and environment. Science 218, 443–448. doi: 10.1126/science.218.4571.443
Bright, J., Desikan, R., Hancock, J. T., Weir, I. S., and Neill, S. J. (2006). ABA-induced NO generation and stomatal closure in Arabidopsis are dependent on H2O2 synthesis. Plant J. 45, 113–122. doi: 10.1111/j.1365-313X.2005.02615.x
Clough, S. J., and Bent, A. F. (1998). Floral dip: a simplified method for Agrobacterium-mediated transformation of Arabidopsis thaliana. Plant J. 16, 735–743. doi: 10.1046/j.1365-313x.1998.00343.x
Corpas, F. J., Barroso, J. B., and del Río, L. A. (2001). Peroxisomes as a source of reactive oxygen species and nitric oxide signal molecules in plant cells. Trends Plant Sci. 6, 145–150. doi: 10.1016/S1360-1385(01)01898-2
Cutler, S. R., Rodriguez, P. L., Finkelstein, R. R., and Abrams, S. R. (2010). Abscisic acid: emergence of a core signaling network. Annu. Rev. Plant Biol. 61, 651–679. doi: 10.1146/annurev-arplant-042809-112122
Dietz, K. J. (2003). Plant peroxiredoxins. Annu. Rev. Plant Biol. 54, 93–107. doi: 10.1146/annurev.arplant.54.031902.134934
Dietz, K. J., Mittler, R., and Noctor, G. (2016). Recent progress in understanding the role of reactive oxygen species in plant cell signaling. Plant Physiol. 171, 1535–1539. doi: 10.1104/pp.16.00938
Gayatri, G., Agurla, S., and Raghavendra, A. S. (2013). Nitric oxide in guard cells as an important secondary messenger during stomatal closure. Front. Plant Sci. 4:425. doi: 10.3389/fpls.2013.00425
Handschumacher, R. E., Harding, M. W., Rice, J., Drugge, R. J., and Speicher, D. W. (1984). Cyclophilin: a specific cytosolic binding protein for cyclosporin A. Science 226, 544–546. doi: 10.1126/science.6238408
He, Z., Li, L., and Luan, S. (2004). Immunophilins and parvulins. Superfamily of peptidyl prolyl isomerases in Arabidopsis. Plant Physiol. 134, 1248–1267. doi: 10.1104/pp.103.031005
Hedrich, R. (2012). Ion channels in plants. Physiol. Rev. 92, 1777–1811. doi: 10.1152/physrev.00038.2011
Hedrich, R., and Geiger, D. (2017). Biology of SLAC1-type anion channels-from nutrient uptake to stomatal closure. New Phytol. 216, 46–61. doi: 10.1111/nph.14685
Hu, H., and Xiong, L. (2014). Genetic engineering and breeding of drought-resistant crops. Annu. Rev. Plant Biol. 65, 715–741. doi: 10.1146/annurev-arplant-050213-040000
Hua, D. P., Wang, C., He, J. N., Liao, H., Duan, Y., Zhu, Z. Q., et al. (2012). A plasma membrane receptor kinase, GHR1, mediates abscisic acid, and hydrogen peroxide-regulated stomatal movement in Arabidopsis. Plant Cell. 24, 2546–2561. doi: 10.1105/tpc.112.100107
Hubbard, K. E., Nishimura, N., Hitomi, K., Getzoff, E. D., and Schroeder, J. I. (2010). Early abscisic acid signal transduction mechanisms: newly discovered components and newly emerging questions. Genes Dev. 24, 1695–1708. doi: 10.1101/gad.1953910
Jannat, R., Uraji, M., Morofuji, M., Islam, M. M., Bloom, R. E., Nakamura, Y., et al. (2011). Roles of intracellular hydrogen peroxide accumulation in abscisic acid signaling in Arabidopsis guard cells. J. Plant Physiol. 168, 1919–1926. doi: 10.1016/j.jplph.2011.05.006
Ju, Y. L., Min, Z., Yue, X. F., Zhang, Y. L., Zhang, J. X., Zhang, Z. Q., et al. (2020). Overexpression of grapevine VvNAC08 enhances drought tolerance in transgenic Arabidopsis. Plant Physiol. Biochem. 151, 214–222. doi: 10.1016/j.plaphy.2020.03.028
Kang, J. Y., Choi, H. I., Im, M. Y., and Kim, S. Y. (2002). Arabidopsis basic leucine zipper proteins that mediate stress-responsive abscisic acid signaling. Plant Cell 14, 343–357. doi: 10.1105/tpc.010362
Kim, S. K., You, Y. N., Park, J. C., Joung, Y., Kim, B. G., Ahn, J. C., et al. (2012). The rice thylakoid lumenal cyclophilin OsCYP20-2 confers enhanced environmental stress tolerance in tobacco and Arabidopsis. Plant Cell Rep. 31, 417–426. doi: 10.1007/s00299-011-1176-x
Kim, T. H., Böhmer, M., Hu, H., Nishimura, N., and Schroeder, J. I. (2010). Guard cell signal transduction network: advances in understanding abscisic acid, CO2, and Ca2+ signaling. Annu. Rev. Plant Biol. 61, 561–591. doi: 10.1146/annurev-arplant-042809-112226
Kollist, H., Nuhkat, M., and Roelfsema, M. R. (2014). Closing gaps: linking elements that control stomatal movement. New phytol. 203, 44–62. doi: 10.1111/nph.12832
Kovacs, D., Kalmar, E., Torok, Z., and Tompa, P. (2008). Chaperone activity of ERD10 and ERD14, two disordered stress-related plant proteins. Plant Physiol. 147, 381–390. doi: 10.1104/pp.108.118208
Kreslavski, V. D., Los, D. A., Allakhverdiev, S. I., and Kuznetsov, V. V. (2012). Signaling role of reactive oxygen species in plants under stress. Russ. J. Plant Physl. 59, 141–154. doi: 10.1134/S1021443712020057
Kwak, J. M., Mori, I. C., Pei, Z. M., Leonhardt, N., Torres, M. A., Dangl, J. L., et al. (2003). NADPH oxidase AtrbohD and AtrbohF genes function in ROS-dependent ABA signaling in Arabidopsis. EMBO J. 22, 2623–2633. doi: 10.1093/emboj/cdg277
Langridge, P., and Reynolds, M. P. (2015). Genomic tools to assist breeding for drought tolerance. Curr. Opin. Biotechnol. 32, 130–135. doi: 10.1016/j.copbio.2014.11.027
Lee, S. C., and Luan, S. (2012). ABA signal transduction at the crossroad of biotic and abiotic stress responses. Plant Cell Environ. 35, 53–60. doi: 10.1111/j.1365-3040.2011.02426.x
Lee, S. S., Park, H. J., Yoon, D. H., Kim, B. G., Ahn, J. C., Luan, S., et al. (2015). Rice cyclophilin OsCYP18-2 is translocated to the nucleus by an interaction with SKIP and enhances drought tolerance in rice and Arabidopsis. Plant Cell Environ. 38, 2071–2087. doi: 10.1111/pce.12531
Li, C. L., Wang, M., Wu, X. M., Chen, D. H., Lv, H. J., Shen, J. L., et al. (2016). THI1, a thiamine thiazole synthase, interacts with Ca2+-dependent protein kinase CPK33 and modulates the S-type anion channels and stomatal closure in Arabidopsis. Plant Physiol. 170, 1090–1104. doi: 10.1104/pp.15.01649
Li, J., Wang, X. Q., Watson, M. B., and Assmann, S. M. (2000). Regulation of abscisic acid-induced stomatal closure and anion channels by guard cell AAPK kinase. Science 287, 300–303. doi: 10.1126/science.287.5451.300
Li, J. J., Li, Y., Yin, Z. G., Jiang, J. H., Zhang, M. H., Guo, X., et al. (2017). OsASR5 enhances drought tolerance through a stomatal closure pathway associated with ABA and H2O2 signalling in rice. Plant Biotechnol. J. 15, 183–196. doi: 10.1111/pbi.12601
Lin, H., Wang, R., Qian, Q., Yan, M., Meng, X., Fu, Z., et al. (2009). DWARF27, an iron-containing protein required for the biosynthesis of strigolactones, regulates rice tiller bud outgrowth. Plant Cell 21, 1512–1525. doi: 10.1105/tpc.109.065987
Liu, H., and Stone, S. L. (2010). Abscisic acid increases Arabidopsis ABI5 transcription factor levels by promoting KEG E3 ligase self-ubiquitination and proteasomal degradation. Plant Cell 22, 2630–2641. doi: 10.1105/tpc.110.076075
Ma, Y., Cao, J., Chen, Q., He, J., Liu, Z., et al. (2019). The kinase CIPK11 functions as a negative regulator in drought stress response in Arabidopsis. Int. J. Mol. Sci. 20:2422. doi: 10.3390/ijms20102422
MacRobbie, E. A. (1998). Signal transduction and ion channels in guard cells. Philos. Trans. R. Soc. Lond. B Biol. Sci. 353, 1475–1488. doi: 10.1098/rstb.1998.0303
Manavalan, L. P., Guttikonda, S. K., Tran, L. S., and Nguyen, H. T. (2009). Physiological and molecular approaches to improve drought resistance in soybean. Plant Cell Physiol. 50, 1260–1276. doi: 10.1093/pcp/pcp082
Martin-StPaul, N., Delzon, S., and Cochard, H. (2017). Plant resistance to drought depends on timely stomatal closure. Ecol. Lett. 20, 1437–1447. doi: 10.1111/ele.12851
Mhamdi, A., Queval, G., Chaouch, S., Vanderauwera, S., Van Breusegem, F., and Noctor, G. (2010). Catalase function in plants: a focus on Arabidopsis mutants as stress-mimic models. J. Exp. Bot. 61, 4197–4220. doi: 10.1093/jxb/erq282
Miao, Y., Lv, D., Wang, P., Wang, X. C., Chen, J., Miao, C., et al. (2006). An Arabidopsis glutathione peroxidase functions as both a redox transducer and a scavenger in abscisic acid and drought stress responses. Plant Cell 18, 2749–2766. doi: 10.1105/tpc.106.044230
Mittler, R. (2002). Oxidative stress, antioxidants, and stress tolerance. Trends Plant Sci. 7, 405–410. doi: 10.1016/S1360-1385(02)02312-9
Mittler, R., Vanderauwera, S., Gollery, M., and Van Breusegem, F. (2004). Reactive oxygen gene network of plants. Trends Plant Sci. 9, 490–498. doi: 10.1016/j.tplants.2004.08.009
Mori, I. C., Murata, Y., Yang, Y., Munemasa, S., Wang, Y. F., Andreoli, S., et al. (2006). CDPKs CPK6 and CPK3 function in ABA regulation of guard cell S-type anion- and Ca2+- permeable channels and stomatal closure. PLoS Biol. 4:e327. doi: 10.1371/journal.pbio.0040327
Munemasa, S., Hauser, F., Park, J., Waadt, R., Brandt, B., and Schroeder, J. I. (2015). Mechanisms of abscisic acid-mediated control of stomatal aperture. Curr. Opin. Plant Biol. 28, 154–162. doi: 10.1016/j.pbi.2015.10.010
Munemasa, S., Oda, K., Watanabe-Sugimoto, M., Nakamura, Y., Shimoishi, Y., and Murata, Y. (2007). The coronatine-insensitive 1 mutation reveals the hormonal signaling interaction between abscisic acid and methyl jasmonate in Arabidopsis guard cells. Specific impairment of ion channel activation and second messenger production. Plant Physiol. 143, 1398–1407. doi: 10.1104/pp.106.091298
Murashige, T., and Skoog, F. (1962). A revised medium for rapid growth and bioassays with tobacco tissue cultures. Physiol. Plant. 15, 473–472. doi: 10.1111/j.1399-3054.1962.tb08052.x
Murata, Y., Mori, I. C., and Munemasa, S. (2015). Diverse stomatal signaling and the signal integration mechanism. Annu. Rev. Plant Biol. 66, 369–392. doi: 10.1146/annurev-arplant-043014-114707
Nyathi, Y., and Baker, A. (2006). Plant peroxisomes as a source of signalling molecules. Biochem. Biophys. Acta 1763, 1478–1495. doi: 10.1016/j.bbamcr.2006.08.031
Osakabe, Y., Osakabe, K., Shinozaki, K., and Tran, L. S. (2014). Response of plants to water stress. Front. Plant Sci. 5:86. doi: 10.3389/fpls.2014.00086
Palma, J. M., Corpas, F. J., and del Río, L. A. (2009). Proteome of plant peroxisomes: new perspectives on the role of these organelles in cell biology. Proteomics 9, 2301–2312. doi: 10.1002/pmic.200700732
Pei, Z. M., Kuchitsu, K., Ward, J. M., Schwarz, M., and Schroeder, J. I. (1997). Differential abscisic acid regulation of guard cell slow anion channels in Arabidopsis wild-type and abi1 and abi2 mutants. Plant Cell 9, 409–423. doi: 10.1105/tpc.9.3.409
Pei, Z. M., Murata, Y., Benning, G., Thomine, S., Klüsener, B., Allen, G. J., et al. (2000). Calcium channels activated by hydrogen peroxide mediate abscisic acid signalling in guard cells. Nature 406, 731–734. doi: 10.1038/35021067
Pogorelko, G. V., Mokryakova, M., Fursova, O. V., Abdeeva, I., Piruzian, E. S., and Bruskin, S. A. (2014). Characterization of three Arabidopsis thaliana immunophilin genes involved in the plant defense response against Pseudomonas syringae. Gene 538, 12–22. doi: 10.1016/j.gene.2014.01.029
Raghavendra, A. S., Gonugunta, V. K., Christmann, A., and Grill, E. (2010). ABA perception and signaling. Trends Plant Sci. 15, 395–401. doi: 10.1016/j.tplants.2010.04.006
Roelfsema, M. R., Hedrich, R., and Geiger, D. (2012). Anion channels: master switches of stress responses. Trends Plant Sci. 17, 221–229. doi: 10.1016/j.tplants.2012.01.009
Santos, I. B. D., and Park, S. W. (2019). Versatility of cyclophilins in plant growth and survival: a case study in Arabidopsis. Biomolecules 9:20. doi: 10.3390/biom9010020
Schroeder, J. I., Allen, G. J., Hugouvieux, V., Kwak, J. M., and Waner, D. (2001). Guard cell signal transdution. Annu. Rev. Plant Physiol. Plant Mol. Biol. 52, 627–658. doi: 10.1146/annurev.arplant.52.1.627
Schroeder, J. I., and Hagiwara, S. (1989). Cytosolic calcium regulates ion channels in the plasma membrane of Vicia faba guard cells. Nature 338, 427–430. doi: 10.1038/338427a0
Seo, M., and Koshiba, T. (2011). Transport of ABA from the site of biosynthesis to the site of action. J. Plant Res. 124, 501–507. doi: 10.1007/s10265-011-0411-4
Sheen, J. (2001). Signal transduction in maize and Arabidopsis mesophyll protoplasts. Plant Physiol. 127, 1466–1475. doi: 10.1104/pp.010820
Song, Y., Miao, Y., and Song, C. P. (2014). Behind the scenes: the roles of reactive oxygen species in guard cells. New Phytol. 202, 1121–1140. doi: 10.1111/nph.12565
Vahisalu, T., Kollist, H., Wang, Y. F., Nishimura, N., Chan, W. Y., Valerio, G., et al. (2008). SLAC1 is required for plant guard cell S-type anion channel function in stomatal signalling. Nature 452, 487–491. doi: 10.1038/nature06608
Venuprasad, R., Lafitte, H. R., and Atlin, G. N. (2007). Response to direct selection for grain yield under drought stress in rice. Crop Sci. 47, 285–293. doi: 10.2135/cropsci2006.03.0181
Wang, X., Shi, X., Hao, B., Ge, S., and Luo, J. (2005). Duplication and DNA segmental loss in the rice genome: implications for diploidization. New Phytol. 165, 937–946. doi: 10.1111/j.1469-8137.2004.01293.x
Wang, X. Q., Ullah, H., Jones, A. M., and Assmann, S. M. (2001). G protein regulation of ion channels and abscisic acid signaling in Arabidopsis guard cells. Science 292, 2070–2072. doi: 10.1126/science.1059046
Willekens, H., Chamnongpol, S., Davey, M., Schraudner, M., Langebartels, C., Montagu, M. V., et al. (1997). Catalase is a sink for H2O2 and is indispensable for stress defence in C3 plants. EMBO J. 16, 4806–4816. doi: 10.1093/emboj/16.16.4806
Willekens, H., Inzé, D., Van Montagu, M., and Van Camp, W. (1995). Catalases in plants. Mol. Breed. 1, 207–228. doi: 10.1007/BF02277422
Xiao, C., Chen, F., Yu, X., Lin, C., and Fu, Y. F. (2009). Over-expression of an AT-hook gene, AHL22, delays flowering, and inhibits the elongation of the hypocotyl in Arabidopsis thaliana. Plant Mol. Biol. 71, 39–50. doi: 10.1007/s11103-009-9507-9
Yamaguchi-shinozaki, K., and Shinozaki, K. (2006). Transcriptional regulatory networks in cellular responses and tolerance to dehydration and cold stresses. Annu. Rev. Plant Biol. 57, 781–803. doi: 10.1146/annurev.arplant.57.032905.105444
Yoon, D. H., Lee, S. S., Park, H. J., Lyu, J. I., Chong, W. S., Liu, J. R., et al. (2016). Overexpression of OsCYP19-4 increases tolerance to cold stress and enhances grain yield in rice (Oryza sativa). J. Exp. Bot. 67, 69–82. doi: 10.1093/jxb/erv421
Zhang, J., Wang, N., Miao, Y., Hauser, F., McCammon, J. A., Rappel, W. J., et al. (2018). Identification of SLAC1 anion channel residues required for CO2/bicarbonate sensing and regulation of stomatal movements. Proc. Natl. Acad. Sci. U.S.A. 115, 11129–11137. doi: 10.1073/pnas.1807624115
Zhang, W., Jeon, B. W., and Assmann, S. M. (2011). Heterotrimeric G-protein regulation of ROS signalling and calcium currents in Arabidopsis guard cells. J. Exp. Bot. 62, 2371–2379. doi: 10.1093/jxb/erq424
Zhang, W., Nilson, S. E., and Assmann, S. M. (2008). Isolation and whole-cell patch clamping of Arabidopsis guard cell protoplasts. CSH Protoc. 2008:pdb.prot5014. doi: 10.1101/pdb.prot5014
Keywords: ROC3, abscisic acid, stomatal closure, drought stress, anion channel, reactive oxygen species, catalase
Citation: Liu H, Shen J, Yuan C, Lu D, Acharya BR, Wang M, Chen D and Zhang W (2021) The Cyclophilin ROC3 Regulates ABA-Induced Stomatal Closure and the Drought Stress Response of Arabidopsis thaliana. Front. Plant Sci. 12:668792. doi: 10.3389/fpls.2021.668792
Received: 17 February 2021; Accepted: 28 April 2021;
Published: 25 May 2021.
Edited by:
Wenxiu Ye, Shanghai Jiao Tong University, ChinaReviewed by:
Izumi C. Mori, Okayama University, JapanCopyright © 2021 Liu, Shen, Yuan, Lu, Acharya, Wang, Chen and Zhang. This is an open-access article distributed under the terms of the Creative Commons Attribution License (CC BY). The use, distribution or reproduction in other forums is permitted, provided the original author(s) and the copyright owner(s) are credited and that the original publication in this journal is cited, in accordance with accepted academic practice. No use, distribution or reproduction is permitted which does not comply with these terms.
*Correspondence: Wei Zhang, d2VpemhhbmdAc2R1LmVkdS5jbg==
Disclaimer: All claims expressed in this article are solely those of the authors and do not necessarily represent those of their affiliated organizations, or those of the publisher, the editors and the reviewers. Any product that may be evaluated in this article or claim that may be made by its manufacturer is not guaranteed or endorsed by the publisher.
Research integrity at Frontiers
Learn more about the work of our research integrity team to safeguard the quality of each article we publish.