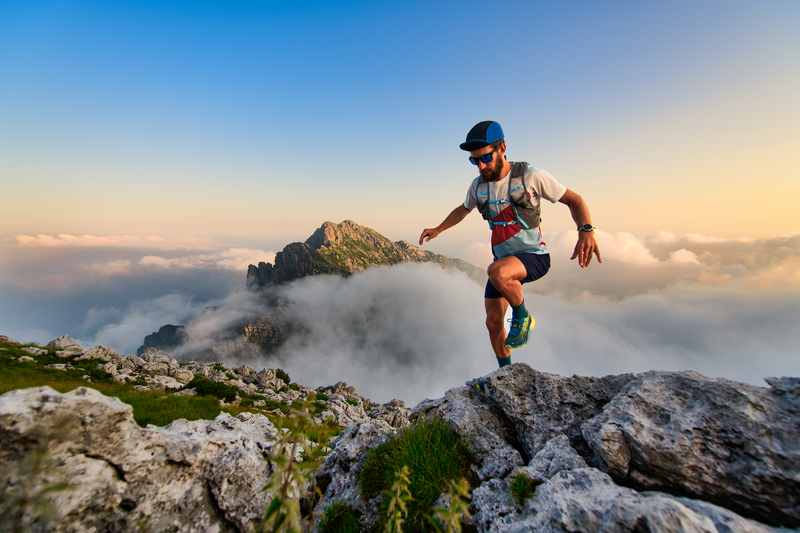
95% of researchers rate our articles as excellent or good
Learn more about the work of our research integrity team to safeguard the quality of each article we publish.
Find out more
REVIEW article
Front. Plant Sci. , 19 May 2021
Sec. Aquatic Photosynthetic Organisms
Volume 12 - 2021 | https://doi.org/10.3389/fpls.2021.665206
This article is part of the Research Topic Lipids in Cyanobacteria, Algae, and Plants - From Biology to Biotechnology View all 17 articles
The remarkable diversity of sterol biosynthetic capacities described in living organisms is enriched at a fast pace by a growing number of sequenced genomes. Whereas analytical chemistry has produced a wealth of sterol profiles of species in diverse taxonomic groups including seed and non-seed plants, algae, phytoplanktonic species and other unicellular eukaryotes, functional assays and validation of candidate genes unveils new enzymes and new pathways besides canonical biosynthetic schemes. An overview of the current landscape of sterol pathways in the tree of life is tentatively assembled in a series of sterolotypes that encompass major groups and provides also peculiar features of sterol profiles in bacteria, fungi, plants, and algae.
Sterols are mandatory components of eukaryotic life as building blocks of cellular membranes and as bioactive signals both functions having specific molecular structural requirements (Schaller, 2010; Darnet and Schaller, 2019). The sterol repertoire, which is built from the C30H50O triterpenic committed precursors cycloartenol and lanosterol is part of the ubiquitous terpenome that is inseparable from the origin of life (Ourisson and Nakatani, 1994), just as DNA, RNA, and proteins. Sterols are also found in some bacterial groups but not in archaea (Salvador-Castell et al., 2019). Hopanoids in bacteria are considered as functional triterpenic counterparts of sterols in eukaryotes, but their presence is not strictly restricted to prokaryotes since some lycophyte species synthesize hopanoids (Kushiro and Ebizuka, 2010). Such an overlapping distribution of structurally different triterpenes and terpenogenic enzymes at play in distantly related taxa illustrates the fact that those pathways are quite ancient acquisitions, which have evolved through multiple mechanisms (Keeling and Palmer, 2008). The diversity and complexity of sterol pathways and the evolution of enzymes is closely associated to the rise of oxygen on earth about two billion years ago (Holland, 2002; Summons et al., 2006). In fact, the enzymatic conversion of squalene to diploptene (the simplest hopanoid) in bacteria is a one-step dioxygen-independent biosynthetic process, whereas the nineteen-enzymatic step conversion of squalene into 24-ethylsterols in viridiplantae requires 12 molecules of dioxygen (Nes, 2011; Gold et al., 2017). The analysis and dating of hydrocarbon biomarkers particularly steranes, which are molecular fossils produced by the diagenetic transformation of sterols, have helped to apprehend the importance of certain eukaryotic groups in geological periods. For instance, isopropylcholestane is debated as the most probable marker of chlorophyta in neoproterozoic sediments (Bobrovskiy et al., 2021).
The elucidation of sterol pathways from biosynthetic to genetic studies has attracted immense research efforts over the last decades (Cornforth, 2002). Major findings from the initial cyclization of the oxidized squalene (3S)-2,3-oxidosqualene into steroidal triterpenols (cycloartenol, lanosterol) to their conversion into cholesterol, ergosterol and phytosterols and the biological significance of those have been assembled in a global picture proposed for mammal, invertebrate, fungi, and plant model species (Nes, 2011; Brown et al., 2018; Moreau et al., 2018). The understanding of accurate biosynthetic processes for example with chemical radiotracers (Cornforth, 2002) preceded the characterization of corresponding enzyme activities but was limited to a small number of laboratory-compatible organisms. The growing access to genome mining is now rapidly broadening the understanding of the origin and distribution of sterol biosynthetic capabilities in the tree of life. The best example when considering sterol pathways is the widespread occurrence of cycloartenol (and cycloartenol synthase, CAS) in prokaryotes (Bode et al., 2003) and eukaryotes like Dictyostelium discoidum (Godzina et al., 2000; Meyer et al., 2002) or Naegleria (Raederstorff and Rohmer, 1987). In fact, novel and massive genome and metagenome analysis coupled with ad hoc functional validation assays will facilitate the access to yet undisclosed sterol biosynthetic enzymes or more generally new pathways and therefore new aspects of cellular homeostasis.
Here, the purpose is to provide an overview of main aspects of sterol profiles and corresponding enzymes from various organisms including plants and algae but not exclusively, and to tentatively classify organisms into sterolotypes, considering a wealth of genetic, biochemical, and phylogenomic data.
Enzymes implied in the post-squalene pathways to sterols in model organisms (mammals: human, mouse; fungi: Saccharomyces cerevisiae; and chlorophyta: Arabidopsis thaliana) have been quite comprehensively described and orthologous or paralogous enzymes were found in related species or taxonomic groups based on functional genomics (Bloch, 1989; Benveniste, 2004; Schaller, 2010; Nes, 2011; Sonawane et al., 2016; Darnet and Schaller, 2019). Initial biosynthetic studies have led to the characterization of enzyme activities responsible for the successive substrate-product bioconversions identified in incorporation experiments or by the targeted action of chemical inhibitors (Guo et al., 1997). Organic analytical chemistry also preceded functional genomics to unveil a major pathway for the production in C5 isoprenic units (isopentenyl diphosphate, dimethylallyl diphosphate) in prokaryotes and some eukaryotes, condensed in linear terpenic precursors (Rohmer, 1999). The occurrence of this so-called methylerythritol 4-phosphate (MEP) pathway in prokaryotes and plastids of the chlorophyta and apicomplexa, along with the classical (first described) mevalonate pathway in vertebrates, invertebrates, fungi and plants is now mapped on the tree of life with an increasing precision and consequently with exceptions or alternatives (Lombard and Moreira, 2011). Forward and reverse genetic screens and genome mining provided scientists with a compendium of genes implied in the conversion of squalene into sterol end-products like cholesterol, ergosterol, stigmasterol, and poriferasterol in human, yeast, land plants and algae, respectively (Figure 1). In brief, human (or mammals and other groups of sterol autotroph animals) and fungi use lanosterol as a committed precursor to produce cholesterol, a C27 product with an 8-carbon atom side chain, and ergosterol, a C28 product with one additional exocyclic carbon atom at C-24 added by a sterol-C-methyltransferase [SMT, here a zymosterol-C24-methyltransferase (SMT1)], respectively. Differences between mammals and fungi includes also a sterol-C22(23)-desaturase (C22D) present in yeast only and a sterol-7-reductase (C7R) absent from yeast only, indicative of gene gain and loss as a powerful driver of sterol profile diversification. The A. thaliana sterol pathway as a model for higher plants displays very strong differences with human and yeast canonical pathways. Plants use cycloartenol as a committed precursor. A further sterol diversification by the action of two distinct SMT1 and SMT2 is responsible for the formation of 24-methylsterols and 24-ethylsterols (C29 products), respectively, (Bouvier-Nave et al., 1998; Schaeffer et al., 2001; Nakamoto et al., 2015). The Arabidopsis pathway also includes a C22D of the same cytochrome P450-dependent monooxygenases group (CYP710A) as the fungi enzyme (Morikawa et al., 2006). Arabidopsis has also evolved two distinct sterol methyl oxidases SMO1 and SMO2 acting non-consecutively during the course of the conversion of cycloartenol into the complex 24-alkyl-Δ5-sterol mixture typical of plants (Darnet and Rahier, 2004). Conversely, a single SMO in fungi and mammals contributes to the C4-demethylation complex of enzymes [including in addition to SMO, a 3β-hydroxysteroid dehydrogenase/C4-decarboxylase (βHSD), a sterone ketoreductase (SR), and a tethering ERG28 protein] that removes successively both methyl groups at C-4 (Figure 1; Bouvier et al., 2005). In addition, the mandatory biosynthesis of phytosterols from cycloartenol (Babiychuk et al., 2008) requires a cyclopropyl sterol isomerase (CPI; Men et al., 2008), which is also present in some protists like D. discoidum that use the cycloartenol pathway (Lovato et al., 2000). Mammalian, fungi and plant sterol pathways therefore define major sterolotypes based on sterol profiles and the associated enzymes like lanosterol synthase (LAS) in mammals, LAS and SMT1 in fungi, and CAS and SMT1 and SMT2 in higher plants (Figure 2). Gene gain or loss differentiate these pathways, otherwise sharing significant orthology and paralogy thus enabling functional cloning in yeast of human and plant enzymes for biochemical complementation or metabolic interference (Corey et al., 1993; Lecain et al., 1996; Husselstein et al., 1999). Those canonical pathways have been quite extensively reviewed (Benveniste, 2004; Schaller, 2010; Nes, 2011; Brown et al., 2018; Moreau et al., 2018). Very strikingly, further gene discovery in sterol metabolism still relies on direct and possibly challenging biochemical approaches. The recent finding of aspartyl ergosterol and the set of enzymes promoting conjugation and deconjugation of ergosterol with amino acids represent a prominent example in the field. This novel group of sterols restricted to fungi (except in S. cerevisiae where the gene is absent) is formed by the action of non-canonical tRNA acyltransferases (Yakobov et al., 2020).
Figure 1. Synthetic view of sterol pathways. Dashed lines discriminate mammalian (blue), fungal (red), and plants (green) canonical pathways. The so-called non-canonical pathways shown for algae is framed in mixed red and green colors to point out Δ5,7-sterols in both fungal and algal groups, and to recall common side chain modifying enzymes SSR2 and SMTs. Substrate-product enzymatic conversion is visualized with a single arrow. The dashed line between campesterol and castasterone (brassinosteroids) represents multiple steps. Sterol names are non-systematic names. The comprehensive IUPAC recommendations and conventional system for sterol nomenclature based on cholestane, ergostane, and stigmastane scaffolds, and the associated stereochemistry is given in Moss (1989) and also commented in Nes (2011). LAS, lanosterol synthase; CAS, cycloartenol synthase; C14DM, sterol-14-demethylase; C14R, sterol-14-reductase; SMO, sterol methyl oxidase; βHSD, 3β-hydroxysteroid dehydrogenase/C4-decarboxylase; SR, sterone ketoreductase; 7ISO, sterol-7(8)-isomerase; C5D, sterol-5(6)-desaturase; C7R, sterol-7-reductase; C24R, sterol-24-reductase; SMT1, sterol-C24-methyltransferase; SMT2, sterol-C28-methyltransferase; CPI, cyclopropyl isomerase; and C22D, sterol-22-desaturase.
Figure 2. Major sterolotypes defined in living organisms. Names in black boxes are chosen according to species or taxonomic groups for which a sterol pathway is well documented. Green boxes indicated whether a CAS (cycloartenol synthase) or LAS (lanosterol synthase) defines a sterol pathway; brackets are for minor contribution of a pathway as currently understood. Orange boxes are marking an AltSQE, alternative squalene epoxidase (canonical SQE otherwise not indicated in sterolotypes). Blue and magenta boxes are for sterol side chain modifications by SSR2 (sterol side chain reductase 2), SMT, sterol-C-methyltransferases. Major or representative sterols are represented for each sterolotype. Nomenclature as in Figure 1. Arabido-type and Solanum-type exhibit the epimeric pair of campesterol (C24α-methylcholesterol) and 22(23)-dihydrobrassicasterol (C24β-methylcholesterol) represented by 24-methylcholesterol.
Besides canonical pathways, sterol biosynthetic processes in non-model eukaryotes are often overlooked, since sterol profiles only are reported but genomic data are just emerging. This is particularly the case for the vast group of algae (chromalveolate microalgae, rhodophyta, and green algae, etc.), which had been described for their sterol content in authoritative reports years ago (Patterson, 1971, 1974) or more recently (Kumari et al., 2013; Volkman, 2016; Figure 1). Major catalysts that generate the above-mentioned sterol diversity are SMT and sterol-Δ24-reductases (C24R) or C22D implied in the modification of the sterol side chain (Nes, 2011). These enzymes are of pivotal importance for producing the epimeric 24-alkyl-sterols in different groups. The big picture obtained from decades of analytical work shows a taxonomy that recapitulates sterol stereochemistry at C24, with algae producing C24β-ethylsterols (clionasterol, poriferasterol) and higher plants producing C24α-ethylsterols (β-sitosterol, stigmasterol; Goad and Goodwin, 1972; Figure 1). Epimeric pairs of sterols like campesterol (C24α) and 22(23)-dihydrobrassicasterol (C24β), sitosterol (C24α), and clionasterol (C24β), stigmasterol (C24α), and poriferasterol (C24β; Figures 1, 3) can be distinguished by NMR spectroscopic methods (Rubinstein et al., 1976). Studies with biological membrane mimics have shown equivalent structural behavior of 24-ethyl epimers (Marsan et al., 1996). The unequal distribution in taxa of those epimers is raising the interesting question of stereochemistry possibly recapitulating biology, as suggested from the progress in brassinosteroid signaling and biosynthesis made at a fast pace since 1996 (Li et al., 1996). In Arabidopsis, campesterol (24α-methylcholesterol) is the committed precursor of brassinosteroids through a C22 hydroxylation pathway whereas the epimeric 22(23)-dihydrobrassicasterol has not been reported as an active brassinosteroid precursor (Fujita et al., 2006; Bajguz et al., 2020). The astonishing diversity of sterol side chain biosynthetic modifications will be further scrutinized thanks to the growing number of eukaryotic genome and metagenome sequencing initiatives. The current global picture of sterol profiles across phyla shows a widespread distribution of C27, C28, and C29 sterols whereas C30 products are restricted to a few species (Figure 4).
Figure 3. Diverse sterols found in algae and plants. Side chain C22, C23, and C24 positions are in red. Chiral substituents at C24 of the sterol side chain are C24α for a methyl or ethyl group in front: campesterol, sitosterol, crinosterol, stigmasterol, chondrillasterol; or C24β for a methyl or ethyl group in back: dinosterol, clionasterol, gorgosterol, poriferasterol. Nomenclature as in Figure 1.
Figure 4. Sterol profiles in algae and plants. Sterol compositions of twenty species are plotted on a schematic diagram, which indicates proportions in percent and molecular structures by color codes. C27, sterols bearing a C8-side chain (e.g., cholesterol); C28, C9-side chain (e.g., ergosterol); and C29, C10-side chain (e.g., fucosterol). C30a, C30H50O direct cyclization products of (3S)-2,3-oxidosqualene (e.g., tetrahymanol). C30b, sterol products with additional methyl groups on the tetracyclic moiety and on the side chain (e.g., dinosterol). C30c, sterol products with a C11-side chain (e.g., propylidene sterols). Sterol compositions as percent of the total were found in published references indicated by upper case numbering of species. Sterols contributing less than 2% of a total were not considered for clarity of this schematic representation. 1, Leblond et al. (2011); 2, Miller et al. (2012); 3, Akihisa et al. (1992); 4, Raederstorff and Rohmer (1987); 5, Chiu et al. (1985); 6, Morikawa et al. (2009); 7, Schaller et al. (1998); 8, Schaeffer et al. (2000); 9, Mitova et al. (1999); 10, Bhatt and Bhatt (1984); 11, Tasende (2000); 12, Lu et al. (2014); 13, Mercer et al. (1974); 14, Lu et al. (2020); 15, Conner et al. (1982); 16, Rampen et al. (2010); 17, Patterson and Nes (1991); 18, Mikami et al. (2018); 19, Giner et al. (2009); 20, Lamacka and Sajbidor (1997); and 21, Chakrabarti et al. (2017). Tetrahymena thermophila, Saccharomyces cerevisiae, and Homo sapiens are shown as single pathway end-product species. Sterols tagged with an asterisk * are C-24 epimeric mixtures.
Squalene is the committed C30 isoprenic precursor of tetrahymanol and hopanoids, and after its conversion into (3S)-2,3-oxidosqualene, of steroidal (cycloartenol, lanosterol, and parkeol) and non-steroidal triterpenoids (Figure 5). While squalene- and (3S)-2,3-oxidosqualene-derived non-steroidal triterpenoids exhibit a skeletal chemical structure distinct from that of steroidal compounds (Xu et al., 2004), tetrahymanol and hopanoids do exert membrane structural functions classically assigned to sterols, as described below. Tetrahymanol is produced in the ciliate Tetrahymena pyriformis by a squalene-tetrahymanol cyclase (THC; Saar et al., 1991; Wiersma et al., 2020). A S. cerevisiae loss-of-function mutant impaired in ergosterol biosynthesis was able to grow autotrophically when expressing the Tetrahymena THC, indicating therefore a functionality of tetrahymanol as a sterol surrogate (Wiersma et al., 2020). Some bacteria are also tetrahymanol producers, however, their biosynthetic process leading to tetrahymanol requires first a squalene-hopene cyclase (SHC) that generates a product on which the bacterial tetrahymanol synthase (THS) performs additional ring expansion of the triterpenic product (Banta et al., 2015). Consequently, THC and THS enzymes define distinct systematic groups, which possess identical triterpene products resulting from evolutionary convergent processes.
Figure 5. Cyclization products of squalene and (3S)-2,3-oxidosqualene in species and taxonomic groups. THC, tetrahymanol synthase; SHC, squalene hopene cyclase; SQE, squalene epoxidase; AltSQE, alternative squalene epoxidase; PAS, parkeol synthase; LAS, lanosterol cyclase; and CAS, cycloartenol cyclase.
Hopanoids are widely distributed in ecosystems. This is shown by their presence in sediments as stable hopane derivatives (or geohopanoids), which are markers of life of particular interest in petroleum geochemistry (Ourisson et al., 1982). These hopanoids are found in many prokaryotic groups (bacteria, cyanobacteria) and are quite often designated as “sterol surrogates” acting as phospholipidic bilayer stabilizers or reinforcers (Bird et al., 1971; De Rosa et al., 1971; Rohmer et al., 1979; Delgado-Baquerizo et al., 2018). This was established in a series of in vitro experiments showing that diplopterol and cholesterol achieved identical effects on phase behavior and ordering of sphingolipids in model membranes (Saenz et al., 2012; Mangiarotti et al., 2019). Hopanoids have attracted considerable research focus on plant-bacteria interactions particularly in legume-rhizobia symbiotic root nodulation since the initial characterization of hopanoid-lipid A conjugates (Silipo et al., 2014; Belin et al., 2018). Hopanoid producers are found within eukaryotes, in lichens and ferns producing both squalene-derived products, and (3S)-2,3-oxidosqualene-derived sterols just like canonical land plants. A cycloartenol to β-sitosterol pathway was described in Selaginella (Weng and Noel, 2013) and in Azolla filiculoides (Brouwer et al., 2015). Genome mining in these species provided new observations on fern-cyanobacteria symbiosis, with the identification of the cyanobacterial origin (from Nostoc azollae also called Anabaena azollae) of the lycophytic SHC (Pereira and Vasconcelos, 2014; Li et al., 2018). Functions of hopanoids as membrane lipids have been more specifically assigned in oxygenic photosynthetic cyanobacteria to the efficient protection through compartmentation, of the essential nitrogenase implied in nitrogen fixation, which is a dioxygen-sensitive process. At the most global scale of the oceans it is proposed that hopanoids are recruited to optimize the efficiency of cyanobacterial diazotrophs (Cornejo-Castillo and Zehr, 2019). This is of course reminiscent of the role of hopanoids as reinforcers of the vesicular structure that protects the site of nitrogenase activity in plant-rhizobia symbiotic relationships (Berry et al., 1993).
The mandatory biosynthetic route to sterols through cycloartenol and not lanosterol in plants was established in a series of comprehensive biosynthetic and genetic studies (Corey et al., 1993; Benveniste, 2004; Babiychuk et al., 2008; Gas-Pascual et al., 2015). However, extensive genome mining based on protein motifs and specific amino acid residue conservation or variation unveiled the presence of LASs in many plant species (Kolesnikova et al., 2006; Suzuki et al., 2006). The significance of a catalytically functional LAS from plants, when expressed in a heterologous yeast, is yet asking for more studies since a loss of function las allele from Arabidopsis displayed no morphogenetic inhibitions (Ohyama et al., 2009). In Euphorbiaceae, a LAS ortholog is producing loads of lanosterol in the cytoplasm of laticifers, in combination with other triterpene synthases like CAS and a butyrospermol synthase (Forestier et al., 2019). Solanaceae do have a LAS, but produce high amounts of cholesterol through the cycloartenol route like it is the case for other phytosterols. In fact, the lanosterol-unrelated cholesterol is a precursor of solanine and chaconine and of some other steroidal glycoalkaloids in tomato and potato, accounting for some 15–20% of total sterols. Besides a canonical plant sterol-Δ24(28)-reductase (Figure 1), a solanaceae-specific sterol-Δ24(25)-reductase also named sterol side chain reductase 2 (SSR2) reduces the Δ24(25) of the 8-carbon side chain of cycloartenol and desmosterol, yielding cholesterol (Sawai et al., 2014; Sonawane et al., 2016). Consequently, a sterol pathway based on a CAS, SSR2, and SMT1 and SMT2 defines a major sterolotype among eukaryotes (Figure 2). The solanaceae Withania somnifera (Indian ginseng or ashwagandha) has a sterol-Δ24-reductase coined 24ISO performing the conversion of 24-methylenecholesterol to 24-methyldesmosterol and consequently channeling the latter substrate into the specific biosynthetic segment for withanolides (Knoch et al., 2018).
Sterols are quite often classified as eukaryotic lipids but in fact some bacterial species synthesize sterols. A prominent example of a bacterial pathway is given by the methylotroph Methylococcus capsulatus, which produces unique Δ8(14) -sterols (Bouvier et al., 1976). M. capsulatus genes encoding a squalene epoxidase, a LAS and a lanosterol-14-demethylase have been identified (Lamb et al., 2007). This bacterial pathway converts lanosterol into a series of metabolites of which 4α-methylcholesta-8(14),24-dienol could be the end-product, which implies a set of enzymes for the demethylation at C-4, reduction at C-24, and isomerization of the lanosterol double bond 8(9) into 8(14). The discovery of C-4 demethylation proteins SdmA (a Rieske-type oxygenase) and SdmB [a NAD(P)-dependent reductase], which convert lanosterol into the corresponding 4-demethylated product shows that a sterol C4-demethylation process has evolved independently in prokaryotes and in eukaryotes (in which a C4-methyl group oxidation is carried out by enzymes from the fatty hydroxylase group; Lee et al., 2018). Other bacteria have evolved other minimal sterol pathways. Gemmata obscuriglobus is a planctomycetes that contains a (3S)-2,3-oxidosqualene cyclase (OSC) responsible for the formation of the isomers lanosterol and parkeol, without further metabolization of these steroidal triterpenes (Pearson et al., 2007). Stigmatella aurantiaca is a myxobacterium that produce sterols from cycloartenol (Bode et al., 2003). Genomic and metagenomic surveys pointed out the presence of OSCs in several bacterial phyla (bacteroidetes, planctomycetes, proteobacteria, and some others), broadening thus the evolutionary success of a bacterial type of sterol pathway (Wei et al., 2016). Although scarce some recent data indicate possible symbiotic interactions at the sterol metabolism interface: the combination of genomics and isotopic ratio mass spectrometry of sterols has been exploited in a probably unique study suggesting a metabolic cooperation between a methanotroph endosymbiont and its eukaryotic host, the mussel Bathymodiolus genus. As a matter of fact, the isotopic ratio δ13C measured for the host cholesterol suggest a direct biogenetic link with methane from deep sea environments. In addition, transcripts for steroidogenic enzymes from the endosymbiont -which would provide the upstream biosynthetic pathway- and the host -which would provide the downstream biosynthetic pathway- were colocalized at the gills of the bivalves in in situ hybridization experiments (Takishita et al., 2017).
Cyanobacteria and other oxygenic photosynthetic bacteria from the genera Anabaena, Nodularia, Nostoc, Spirulina, Chlorogloeopsis, Phormidium conceal some sterol producing species as it was debated in the sixties especially with the detection of 24-ethylcholesterol (De Souza and Nes, 1968) and reviewed more recently (Volkman, 2003, 2016). Biosynthetic studies showing the conversion of isoprenic precursors to sterol products were not recently reported in any of the common cyanobacteria. Phormidium autumnale was shown to produce sterols albeit in heterotrophic culture systems compatible with an industrial scale-up (Fagundes et al., 2018). Metagenomic analysis of prokaryotic sequences pointed out OSC genes in some other genera of cyanobacteria like Planktothrix or Prochloron (Wei et al., 2016), but not in the genome of one of the most popular cyanobacterium Synochocystis sp. (Kaneko et al., 1996).
Tetrahymena spp. and Paramecium tetraurelia are sterol auxotrophs that develop on dietary sterols unless degraded environmental conditions like starvation switches on a capacity to make tetrahymanol (Raederstorff and Rohmer, 1988; Najle et al., 2020). The enzymes recruited for metabolization of exogenous sterols are a canonical sterol-C5-desaturase (Poklepovich et al., 2012) belonging to the fatty acid hydroxylase group (Sperling et al., 2003), a C24-dealkylase (or sterol-24-de ethylase) acting on the sterol side chain (Tomazic et al., 2011, 2014), and a Rieske Oxygenase performing a sterol-C7-desaturation (Nusblat et al., 2005; Najle et al., 2013). Enzymes of the same type are found in distant sterol-auxotroph, namely, insects, and nematodes (Zhou et al., 2020). This observation adds on typical cases of convergent evolution, here for a functional remodeling of phytosterols, by enzymes most probably acquired by horizontal gene transfer (Darnet et al., 2020). Genome wide investigations in Tetrahymena led to the identification of a sterol-22-desaturase belonging to the super family of fatty acid hydroxylases, and not to the canonical P450 monooxygenases (Najle et al., 2020). The multiple desaturations of exogenous cholesterol by Tetrahymena thermophila (Figure 6) is reminiscent of the conversion of dietary cholesterol into dafachronic acids – a class of signaling steroids- by Caenorhabditis elegans, a distantly related sterol-auxotroph (Zhou et al., 2020). Enzyme evolution and innovations (Newton et al., 2018) particularly in the super group of fatty acid hydroxylases is not restricted to ciliates when it comes to the post-squalene pathway. An enzyme of the fatty acid hydroxylase type was shown to use squalene as a substrate to produce (3S)-2,3-oxidosqualene in a diatom, therefore named alternative squalene epoxidase (AltSQE; Pollier et al., 2019).
Figure 6. Sterol metabolization reactions in the ciliate Tetrahymena. The conversion of sitostanol is shown [adapted from Najle et al. (2013)]. C5-DES, sterol-5(6)-desaturase; C7-DES, sterol-7-desaturase; C22-DES, sterol-22-desaturase; and C24DET, sterol-24-de-ethylase enzyme abbreviations from Najle et al. (2013).
Dinoflagellates from the SAR group (comprising stramenopila, alveolate, and rhizaria) shown in the new tree of eukaryotes (Burki et al., 2020) exhibit very striking sterol biosynthetic peculiarities. This group is widespread in aquatic ecosystems and is most importantly hosting symbiotic species required for the remarkable biology of cnidaria and especially reef-building corals, which depend on their symbionts for nutrients and sterols (Lu et al., 2020). The sterol supply to an anemone from its symbiodinaceae symbionts was shown to be associated with a symbiosome enriched with a Niemann-Pick type C2 (NPC2) transporter protein (Hambleton et al., 2019). The implication in sterol transport of NPC proteins are otherwise well characterized in mammalian lysosomal cholesterol metabolism (Strauss et al., 2002; Kidambi and Patel, 2008).
Dinoflagellates produce a specific 4-methylsterol called dinosterol and some other particular sterols like gorgosterol (C30 sterol products). These organisms use lanosterol as a sterol precursor (Shimizu et al., 1976; Alam, 1979; Volkman, 2016; Lu et al., 2020), and have evolved a so-called alternative squalene epoxidase to produce (3S)-2,3-oxidosqualene (Pollier et al., 2019). Dinosterol amounts are highly variable between dinoflagellates species in the genera Symbiodinium, Gymnodinium, Fragilidium, and Gonyaulax (Piretti et al., 1997; Amo et al., 2010). Dinosterol (4α,22,23-trimethylcholesta-5,22-dienol; Figure 3) has 3 exocyclic carbon atoms when compared to cholesterol. Sterols with an exocyclic methyl group at C4 are found in roundworms like Caenorhabditis elegans (Hannich et al., 2009; Zhou et al., 2020). In these invertebrates, which are sterol auxotrophs, dietary cholesterol is metabolized by several enzymes into 4α-methyllophenol just like in a cholesterol retro-biosynthetic segment. Among these enzymes, STRM-1 is a sterol-C4-methyltransferase (4-SMT) from the orthologous group of SMT (Zhou et al., 2020). 4-SMT has distinct specificity and substrate recognition characteristics when compared to SMT1 and SMT2 that catalyze methyl transfers on olefinic sterol side chains (Darnet et al., 2020). An orthologous 4-SMT sequence from Symbiodinium is reported in databases suggesting that 4-methylsterols in dinoflagellates originate from the same biosynthetic process functionally described in the case of round worms (Lu et al., 2020). The occurrence of 4-methylsterols produced through C4-methylation in very distant taxa suggest a convergent evolutionary process at play to gain biological functions, yet unclear in the case of dinoflagellates. Fugacium kawagutii exhibits a sterol profile with all compounds methylated at C4, and dinosterol and 24-desmethyldinosterol accounting for sixty percent of the total (Lu et al., 2020). Dinosterol has also two exocyclic carbon atoms in its side chain, at C24 and at the unusual C23 position for a sterol side chain alkylation, and a double bond at C22. This favors the existence of an additional SMT to SMT1, yet to be identified. Dinoflagellates may probably have a sterol-C22-desaturase reminiscent of the type of reaction catalyzed by a cytochrome P450 dependent monooxygenase in canonical pathways but this particular point requires further genome mining and functional assays in heterologous systems to uncover orthologous or novel side chain modifying enzymes. Besides dinosterol other sterol molecules like gorgosterol are considered as taxonomic markers of the dinoflagellates (Mackenzie et al., 1982). Gorgosterol is a very particular sterol not seen in canonical models but it features the wealth of complex sterol structures describes in marine organisms (Giner and Djerassi, 1991). Gorgosterol harbors a cyclopropyl group at C22 indicative of a side chain rearrangement most probably through reactions well studied in the case of cyclopropyl and cyclopropenyl sterols found in sponges (Giner, 1993). The sterolotype defined by dinoflagellates with a LAS and the particular 4-SMT in addition to SMT1 (Figure 2) would certainly urge further research effort to clarify a possible role of specific 4-methylsterols in the success of symbiotic association with the sterol auxotrophic cnidarians and this in the context of strong abiotic environmental stress leading to coral bleaching (Lu et al., 2020).
Two major groups found in Alveolata and Excavata (Burki et al., 2020) are the apicomplexan parasites, which are sterol auxotrophs, and the kinetoplastida trypanosomatidae, which are sterol autotrophs. The search for therapeutic chemicals has driven significant aspects of sterol research in these organisms particularly on sterol uptake and biosynthesis that are targets of unbroken medical and economic interest (Roberts et al., 2003; Chaudhary and Roos, 2005).
Species from the trypanosomatidae are causing severe parasitic diseases in human and other mammals like T. cruzi causes Chagas disease, T. brucei causes sleeping sickness (African trypanosomiasis), and Leishmania causes leishmaniasis. Trypanosomes synthesize their 24-alkylsterols de novo from isoprenic precursors produced through the mevalonate pathway (El-Sayed et al., 2005; Cosentino and Aguero, 2014; Gold et al., 2016). The conversion of lanosterol to ergosterol has been demonstrated in these parasites, which display therefore a fungal type of sterol pathway (Fügi et al., 2014; Gold et al., 2016). Some enzymes implied in this pathway especially those that are major targets for biocides have been the focus of intensive research. These enzymes are 3-hydroxy-3-methylglutaryl-CoA-reductase (HMGR), squalene epoxidase, lanosterol-C14-demethylase and sterol-C24-methyltransferase (SMT), inhibited by statins, allylamines, azoles, and azasterols, respectively, Buckner et al. (2000), Urbina et al. (2002), Roberts et al. (2003), Lepesheva et al. (2004, 2018), Friggeri et al. (2018), Darnet and Schaller (2019). Remarkably, a distinct sterol composition of specific parasitic developmental stages was described in T. cruzi. Epimastigotes contain ergosterol and amastigotes produce 24-methyl-Δ7-sterols and 24-ethylidene-Δ7-sterols (Cosentino and Aguero, 2014). In T. brucei, a complex network of ergosterol and novel ergostatrienols coined ETO [ergosta-5,7,25(27)-trien-3β-ol] and 24-DTO [dimethyl ergosta-5,7,25(27)-trienol] were detected in procyclic and bloodstream forms of the parasite (Nes et al., 2012). Amoeba and other metamonada are classified with the excavates (Burki et al., 2020). Some species present a sophisticated sterol metabolism that includes an endogenous sterol biosynthetic pathway and an additional capacity to metabolize exogenous cholesterol. Naegleria lovaniensis, Naegleria gruberi, and Acanthamoeba polyphaga are producing ergosterol, cholesterol, and poriferasterol derivatives (Raederstorff and Rohmer, 1987; Zhou et al., 2018). In these unicellulars, ergosterol biosynthesis occurs via cycloartenol (and not lanosterol), which is converted into 31-norlanosterol and from that point a pathway closely identical to the algae pathway is leading to the above-mentioned end-products. More recent studies combining chemistry and genomics in Naegleria fowleri confirmed a cycloartenol to cholesterol pathway in the amoeba, and also a dual origin of cholesterol of both biosynthetic and dietary origin (Zhou et al., 2018). Naegleria converts cholesterol into Δ5,7-dienic sterols most probably using a sterol-C7-desaturase of the Rieske oxygenase type, which has been initially discovered in sterol auxotrophs like Drosophila and round worms or Tetrahymena. These organisms require specific sterols at specific developmental stages of their life span and for this recruit specific enzymes yet considered as unusual with respect to the canonical view on sterol pathways.
The sterol content of diatoms has been reported (Volkman, 2003, 2016). The search for biomarkers of these planktonic organisms is motivated by the tremendous role they play in carbon dioxide fixation through photosynthesis and this at a global scale (Gold et al., 2017). An attempt to link a 18S RNA-based molecular phylogeny to sterol profiles of hundreds of species including raphid pennates, araphid pennates, radial centrics, and others came to the conclusive evidence that diatoms may not be specifically marked with a sterol profile (Rampen et al., 2010). In rare cases 23-methylsterols and 23,24-dimethylsterols were found, eventually distinguishing those species from other microalgae. The unusual sterol side chains with a methyl group at C-23, a 22-cyclopropane or a 24-propylidene is also found in dinoflagellates or sponges (Giner et al., 1991). In diatoms, the isoprenic precursors are provided by the plastidial MEP pathway but some species produce the C5 building blocks from mevalonate (Cvejic et al., 2000). The quite well studied Phaeodactylum tricornutum performs squalene epoxidation to (3S)-2,3-oxidosqualene using the alternative squalene epoxidase AltSQE that belongs to the fatty acid hydroxylase type of oxygenases, is widely distributed in many eukaryotic groups, and is distinct from the flavoprotein-type of squalene epoxidase (Pollier et al., 2019). Diatoms produce 24-alkyl-Δ5-sterols through the cycloartenol route (Fabris et al., 2014; Gallo et al., 2020). The effect of light spectral quality and temperature were shown as major physiological parameters acting on the sterol composition and quantity in Phaeodactylum tricornutum (Veron et al., 1996). P. tricornutum, Thalassiosira sp. and Nannochloropsis oceanica have been studied with an objective to assess their biotechnological potential for hydrocarbon or fine chemical industrial supply (Lu et al., 2014). Proteomic and chemical lipid profiling in these organisms unveiled particular aspects of sterol compartmentation and lipid homeostasis (Lupette et al., 2019). Interference with synthetic estrogens observed in a chemical screening for strong triacylglycerol producers in P. tricornatum resulted in a reallocation of acetyl-CoA building blocks from the isoprenoid/sterol pool to the fatty acyl-CoA pool (Conte et al., 2018). Two diatoms Skeletonema marinoi and Cyclotella cryptica were subjected to transcriptome profiling and sterol biosynthetic studies implementing 13C-glucose feeding to cultures (Gallo et al., 2020). Clionasterol (C24β-ethylcholesterol) and fucosterol were identified in these species (Gallo et al., 2020). A considerable amount of analytical work carried out on algae around the seventies has firmly established the distribution of epimeric pairs of C24-alkylsterols in eukaryotes as already depicted above, with pairs such as β-sitosterol (24α) and clionasterol (24β) found in higher plants and in algae, respectively. Likewise, the stereoisomers isofucosterol (transC24) and fucosterol (cisC24) are found in higher plants and in algae, respectively, (Patterson, 1971). In silico analysis of the transcriptomes from S. marinoi and C. cryptica suggest that the formation of fucosterol is mediated by an algal SMT2 (in diatoms and red algae, therefore named red SMT), which may have diverged from an ancestor quite early in the evolution toward canonical SMT1 and SMT2 enzymes (Gallo et al., 2020). Alltogether the combination of AltSQE, CAS, and algal SMT2 defines an interesting diatom sterolotype (Figure 2). Strikingly, P. tricornatum is lacking a SMT2, which explains the absence of 24-ethylsterols in P. tricornutum (Rampen et al., 2010; Lu et al., 2014). The cycloartenol-to-cholesterol route was recently confirmed in the chaetocerid diatom Chaetoceros muelleri (Jaramillo-Madrid et al., 2020b).
Conjugation of sterols in diatoms is suggested to play a role in growth dynamics and particularly oceanic phytoplankton blooming, a process which is controlled by programmed cell death (Gallo et al., 2017). Sulfonation of sterols in diatoms may regulate blooming dynamics. In fact, organic extracts prepared from growth phase declining cultures of S. marinoi were used in bio-guided fractionation assays to isolate sterol sulfates. Cholesterol and dihydrobrassicasterol sulfates were found, but also sitosterol sulfate in the active fractions. In the latter case one may find intriguing that diatoms biosynthesize clionasterol (24β-ethylcholesterol) but use sitosterol and stigmasterol (24α-ethylsterols) as a substrate for sulfotransferases (Gallo et al., 2018; Nuzzo et al., 2018).
Emiliania huxleyi is a phytoplanktonic prymnesiophyte (haptophyte). This ubiquitous coccolithophore that is forming large blooms in the oceans has a tremendous impact on carbon and sulfur cycling, ecosystems, and ultimately on climate (Holligan et al., 1983; Rivero-Calle et al., 2015). E. huxleyi is certainly not the only unicellular phytoplanktonic alga with such an ecological importance (Rivero-Calle et al., 2015) but recent work showed that its growth and bloomings regulated by coccolithoviruses implied a considerable metabolic remodeling and reprogramming of the host lipid metabolism during the infection process (Rosenwasser et al., 2014; Frada et al., 2017). Major effects were seen on fatty acid and sphingolipid metabolism but also on the mevalonate/isoprenoid and sterol pathways apparently hijacked by the virus for its replication (Rosenwasser et al., 2014). The exact nature of all coccolithophore sterols was not reported in this study although previous chemotaxonomic studies established those sterol profiles in the context of geochemistry surveys (Taipale et al., 2016; Volkman, 2016). E. huxleyi produces a 24-ethylsterol (24-ethylcholesta-5,22E-dien-3β-ol) as a major component and small amount of cholesterol among other sterols (Volkman et al., 1981). Genome mining in this species points out an almost complete pathway and therefore the indication of a cycloartenol route in agreement with recent evolutionary studies (Gold et al., 2016).
Algae from diverse groups of phaeophyta (brow algae), rhodophyta (red algae), and chlorophyta (green algae) have triggered the interest of analytical biochemists since the initial characterization of fucosterol (cis-24-ethylidenecholesterol) in Fucus vesiculosus (Heilbron et al., 1934; Patterson, 1971; McKean and Nes, 1977; Heilbron and Kevles, 1988; Komura et al., 2014). Genome sequences have been produced for the brown algae Ectocarpus siliculosus (Cock et al., 2010) and Saccharina japonica (Ye et al., 2015). E. siliculosus exhibits a sterol profile with cholesterol, ergosterol and fucosterol as prominent compounds and also some 24-ethylsterols (Mikami et al., 2018). Sterol enzymes predicted from the E. siliculosus sequence were closely similar to those of the diatoms Thalassiosira oceanica and T. pseudomona (Lommer et al., 2012), however, the epoxidation of squalene to (3S)-2,3-oxidosqualene is done by the canonical squalene epoxidase, not by the alternative squalene epoxidase found in diatoms (Lu et al., 2014; Gold et al., 2016; Pollier et al., 2019). Two distinct SMTs were identified in genomes of E. siliculosus and E. subulatus sp. Ec32 genomes (Dittami et al., 2020), in agreement with sterol profiles. Further genome mining and experimental validation of candidate genes are required to document the apparent absence in these genomes of sterol-24-reductases (24RED) and sterol-22-desaturases (C22D) of the canonical type (Dittami et al., 2020). High amounts of fucosterol in brown algae are often accompanied by saringosterol, a 24-hydroxy-24-vinylcholesterol that arise from non-enzymatic oxidative conversion of fucosterol (Knights, 1970).
The red and brown algae synthesize cholesterol, with red algae being very strong producers (Tsuda et al., 1957; Gibbons, 1967). Chondrus crispus has more than 94% of cholesterol in its total sterols but desmosterol or Δ22-cholestenol were detected as well (Tasende, 2000). These species may represent a rich source of bio-based non-animal cholesterol. C. crispus and Cyanidioschyzon merolae were selected for in-depth genomic studies, from which a sterol pathway was inferred and modeled (Belcour et al., 2020). Sterol profiles were not established in the case of C. merolae but genomic datasets indicated the presence of a complete pathway from cycloartenol to cholesterol (Desmond and Gribaldo, 2009; Lu et al., 2014). The mixotrophic and thermophilic unicellular red algae Galdieria sulphuria and Cyanidium caldarium produce ergosterol and other dienic or polyenic ergosterol derivatives, in addition to 24-ethylsterols (Seckbach et al., 1993). Genomes of G. sulphuria and from Porphyridium purpureum have been sequenced, however, a curated catalog of sterol genes is not fully available, although annotations provided by databases (KEGG, MetaCyc, and others) indicate a cycloartenol route for sterol production in red algae and furthermore suggest a monophyletic evolutionary relationship between red algae and plants (Bhattacharya et al., 2013; Schonknecht et al., 2013).
Green algae from the chlorophyta like Chlorella sp., Volvox sp., Chlamydomonas sp., Ostreococcus sp., and some other genera like Monodus sp., and Ochromonas sp., from the chrysophyta (golden brown algae) have been studied decades ago (Moseley and Thompson, 1980; Gealt et al., 1981) with a continuous interest to depict the evolutionary relationship between, e.g., a fungal ergosterol pathway and an algal ergosterol pathway (Miller et al., 2012). The overall published data on algal sterols provides a clear-cut dichotomy of sterols structures found in large taxonomic groups (as already discussed in a previous section of this review): the sterol profiles established for all species of the chlorophyta and chrysophta exhibit C24β-methylsterols (brassicasterol, ergosterol) and C24β-ethylsterols (clionasterol, poriferasterol, and 7-dehydroporiferasterol). The technical requirements for the distinction of C24α - and C24β-ethylsterols (Rubinstein et al., 1976) most probably led to misnaming epimeric pairs of sterols here or there. Genome and proteomes of algae (Nguyen et al., 2011) are confirming the cycloartenol route for the production of 24-alkylsterols in green algae, which define a Chlorella-chlamydo-type of sterol pathways (Figure 2) (Miller et al., 2012). Pathways inferred from in silico analysis using canonical data sets as usual queries generally unclose orthologs and paralogs of steroidogenic enzymes. However, genes and enzymes acting on the sterol side chain modification (SMT, sterol-22-desaturases, sterol-24-reductase) or genes and enzymes required for the metabolization of the tetracyclic nucleus (sterone-3-reductase, sterol-C5-desaturase, sterol-C7-reductase and other putative enzymes) certainly deserve a further attention (Lu et al., 2014; Brumfield et al., 2017). Interestingly, the Chlamydomonas single SMT upon expression in yeast produces Δ7,22-poriferasterol, a C24β sterol epimer typical of algae (Brumfield et al., 2017). The access to novel sequence data for species like Chlorokybus atmophyticus (Wang et al., 2020) or Penium maragaritaceum (Jiao et al., 2020) will provide additional resources to enrich sterol profile records and to examine a sterol profile-genome congruence.
The access to novel sequenced genomes and the increasing quality of their expert annotation and curation is opening the search for interesting new genes, pathways and for a more exhaustive view upon the sterol biosynthetic capacities and distribution in eukaryotes (Figure 7). The integration of a considerable body of published data on the sterol composition of a multitude of species – sometimes in ancient and overlooked papers and repositories – with genome sequencing led us to tentatively visualize major taxonomic groups with sterolotypes, which may evolve as far as more information will be produced from genome surveys (Wong, 2019), especially those genomes coming from the oceans (Carradec et al., 2018; Canals et al., 2020). To reach this goal, indissociable efforts of investigating and validating accurate gene functions in dedicated heterologous expression systems will further support advanced phylogenomic studies (Desmond and Gribaldo, 2009; Gold et al., 2016). In the same vein, progresses in sterolomics will in turn provide users with powerful methods to envisage large-scale metaphenomics for a data-driven treatment of biological questions (Griffiths and Wang, 2019). Nonetheless, yet unknown genes and enzymes may well arise from forward and serendipitous genetic screenings. It is also worth noting that subtle variations in enzyme sequences like single amino acid substitution(s) may define a pathway, like for instance mutation(s) in cycloartenol synthase converts the latter into a LAS (Meyer et al., 2002). Consequently, genomic analyses per se are not sufficient to define sterolotypes, which are based on both decisive enzymes and accurate sterol compositions (Figure 2). The inherent limited potential of expert BLAST searches for predicting orthology and paralogy is especially emphasized when comparing protein sequences of selected species including canonical models, and enzyme-based sterolotypes. Species that belong to a same sterolotype as defined in this study display for each sterol biosynthetic enzymes a remarkably wide range of sequence identities (Supplementary Figure 1).
Figure 7. Sterolotype distribution over a tree of eukaryotes. A selection of species is tagged with sterolotypes presented in Figure 2. References about genomes and gene functions, and sterol profiles, are indicated by G1 to G8. G1, (Lu et al., 2014); G2, (Brumfield et al., 2017); G3, (Gold et al., 2016); G4, (Morikawa et al., 2009); G5, (Schaller, 2010); G6, (Sonawane et al., 2016); G7, (Lu et al., 2020); and G8, (Fügi et al., 2014). Species with no sterolotype indications are awaiting for a comprehensive mining of corresponding genomes. Gene nomenclature for Arabidopsis thaliana, Saccharomyces cerevisiae, Homo sapiens, and Chlamydomonas reinhardtii is given in Supplementary Table 1.
Sterolotypes defined in this paper readily stand on the AltSQE/SQE, CAS/LAS distinction, and on the sterol side chain modification enzymes (Figure 2). The exclusive choice of CAS or LAS is not fully understood as for instance the evolutionary conserved LAS in land plants has not been integrated as an essential contributor to sterol homeostasis. Sterolotypes flagged with CAS also highlight the remarkable conversion of cycloartenol to cholesterol in viridiplantae (Babiychuk et al., 2008; Ohyama et al., 2009; Sawai et al., 2014; Sonawane et al., 2016). This pathway recruits a particular sterol-Δ24-reductase paralog (SSR2 in Solanum lycopersicum) also found in rhodophyta (Calegario et al., 2016), for the synthesis of cholesterol. Distinct pathways for the production of that unique sterol in red algae or plants, and in mammals, speaks therefore in favor of convergent evolutionary process in sterol biology, as depicted in other eukaryotic groups in this brief overview.
Finally, sterols have raised interest from the biotech and industrial field due to their multiple pharmaceutical, cosmetic and food applications like blood cholesterol-lowering, anticancer, antiangiogenic, anti-inflammatory, antiatherosclerotic, anthelminthic, and antioxidant effects (Martin-Creuzburg and Elert, 2009; Martin-Creuzburg and Merkel, 2016). The sterol supply for a global market could possibly shift from agricultural waste products (Maniet et al., 2019) or plants (Zhang et al., 2020), to diatoms and other microalgae as a versatile system (Singh et al., 2017). Successful achievements in specific pathway engineering, for instance expected from the expression of limiting enzymes (Jaramillo-Madrid et al., 2020a), certainly require a further picturing of the intricate regulatory metabolic networks and cellular homeostasis of these producers (Marechal and Lupette, 2019), in order to provide at source to sink production of sterols compatible with the market demand (Luo et al., 2015).
SD and HS conceived the overview and wrote the manuscript with contributions from AB and QC. All authors contributed to the article and approved the submitted version.
The authors wish to acknowledge the European commission for a MCSA H2020 grant no 897283 to SD, Carlsberg group/Kronenbourg breweries for financial support (CNRS contract no 174144) to AB, and the University of Strasbourg for supporting QC (ATER contract 2020–2021). HS acknowledges funding from the Agence Nationale de la Recherche (grant ANR-20-CE44-0002-02).
The authors declare that the research was conducted in the absence of any commercial or financial relationships that could be construed as a potential conflict of interest.
The Supplementary Material for this article can be found online at: https://www.frontiersin.org/articles/10.3389/fpls.2021.665206/full#supplementary-material
Supplementary Figure 1 | Protein sequence comparison between species and sterolotypes. The orthologs were retrieved by BLAST search and the amino acid percent identity was reported in the matrix. Proteomes are imported from UniProt database. The accession numbers for Homo sapiens, HsLAS, Saccharomyces cerevisiae, ScLAS, ScSMT1, and Arabidopsis thaliana, AtCAS1, AtLAS1, AtSMT1, At plant SMT2 are indicated in Supplementary Table 1. Accessions for other protein sequences as follows. Phaeodactylum tricornutum: PtAltSQE, B7FXW1; PtCAS1, XP_002185678.1; PtSMT1, XP_002186194.1; Pt algal SMT2, XP_002178531.1; Chlorella variabilis: CvCAS1, XP_005848291.1; CvSMT1, XP_005845039.1; Cv algal SMT2, XP_005844298.1; Symbiodinium microadriaticum: SmCAS1, OLP86082.1; SmSMT1, OLP85101.1; Sm4-SMT, OLQ09145.1; SmAltSQE, CAE7673970.1; and Solanum lycopersicum: SlCAS1, NP_001233784.1; SlLAS1, XP_004240118.1; SlSMT1, XP_004229650.1; Sl plant SMT2, XP_004248642.1; SlSSR2, NP_001306251.1. Horizontal frames, species assigned to a sterolotype. Vertical frames, exemplified enzyme sequence diversity.
Akihisa, T., Hayashi, Y., Patterson, G. W., Shimizu, N., and Tamura, T. (1992). 4α-methylvernosterol and other sterols from Vernonia anthelmintica seeds. Phytochemistry 31, 1759–1763.
Alam, M. (1979). Dinoflagellate sterols I: sterol composition of the dinoflagellates of ? species. Steroids 33, 197–203.
Amo, M., Suzuki, N., Kawamura, H., Yamaguchi, A., Takano, Y., and Horiguchi, T. (2010). Sterol composition of dinoflagellates: different abundance and composition in heterotrophic species and resting cysts. Geochem. J. 44, 225–231.
Babiychuk, E., Bouvier-Nave, P., Compagnon, V., Suzuki, M., Muranaka, T., Van Montagu, M., et al. (2008). Allelic mutant series reveal distinct functions for Arabidopsis cycloartenol synthase 1 in cell viability and plastid biogenesis. Proc. Natl. Acad. Sci. U.S.A. 105, 3163–3168. doi: 10.1073/pnas.0712190105
Bajguz, A., Chmur, M., and Gruszka, D. (2020). Comprehensive overview of the brassinosteroid Biosynthesis pathways: substrates. products, inhibitors, and connections. Front. Plant Sci. 11:1034. doi: 10.3389/fpls.2020.01034
Banta, A. B., Wei, J. H., and Welander, P. V. (2015). A distinct pathway for tetrahymanol synthesis in bacteria. Proc. Natl. Acad. Sci. U.S.A. 112, 13478–13483. doi: 10.1073/pnas.1511482112
Belcour, A., Girard, J., Aite, M., Delage, L., Trottier, C., Marteau, C., et al. (2020). Inferring biochemical reactions and metabolite structures to understand metabolic pathway drift. iScience 23:100849. doi: 10.1016/j.isci.2020.100849
Belin, B. J., Busset, N., Giraud, E., Molinaro, A., Silipo, A., and Newman, D. K. (2018). Hopanoid lipids: from membranes to plant-bacteria interactions. Nat. Rev. Microbiol. 16, 304–315. doi: 10.1038/nrmicro.2017.173
Benveniste, P. (2004). Biosynthesis and accumulation of sterols. Annu. Rev. Plant Biol. 55, 429–457.
Berry, A. M., Harriott, O. T., Moreau, R. A., Osman, S. F., Benson, D. R., and Jones, A. D. (1993). Hopanoid lipids compose the Frankia vesicle envelope, presumptive barrier of oxygen diffusion to nitrogenase. Proc. Natl. Acad. Sci. U.S.A. 90, 6091–6094. doi: 10.1073/pnas.90.13.6091
Bhatt, P. N., and Bhatt, D. P. (1984). Changes in sterol content during leaf aging and in vitro differentiation in Solanum nigrum. J. Nat. Prod. 47, 426–432.
Bhattacharya, D., Price, D. C., Chan, C. X., Qiu, H., Rose, N., Ball, S., et al. (2013). Genome of the red alga Porphyridium purpureum. Nat. Commun. 4:1941.
Bird, C. W., Lynch, J. M., Pirt, F. J., and Reid, W. W. (1971). Steroids and squalene in Methylococcus capsulatus grown on methane. Nature 230, 473–474. doi: 10.1038/230473a0
Bobrovskiy, I., Hope, J. M., Nettersheim, B. J., Volkman, J. K., Hallmann, C., and Brocks, J. J. (2021). Algal origin of sponge sterane biomarkers negates the oldest evidence for animals in the rock record. Nat. Ecol. Evol. 5, 165–168. doi: 10.1038/s41559-020-01334-7
Bode, H. B., Zeggel, B., Silakowski, B., Wenzel, S. C., Reichenbach, H., and Muller, R. (2003). Steroid biosynthesis in prokaryotes: identification of myxobacterial steroids and cloning of the first bacterial 2,3(S)-oxidosqualene cyclase from the myxobacterium Stigmatella aurantiaca. Mol. Microbiol. 47, 471–481. doi: 10.1046/j.1365-2958.2003.03309.x
Bouvier, F., Rahier, A., and Camara, B. (2005). Biogenesis, molecular regulation and function of plant isoprenoids. Prog. Lipid Res. 44, 357–429.
Bouvier, P., Rohmer, M., Benveniste, P., and Ourisson, G. (1976). Delta8(14)-steroids in the bacterium Methylococcus capsulatus. Biochem. J. 159, 267–271. doi: 10.1042/bj1590267
Bouvier-Nave, P., Husselstein, T., and Benveniste, P. (1998). Two families of sterol methyltransferases are involved in the first and the second methylation steps of plant sterol biosynthesis. Eur. J. Biochem. 256, 88–96. doi: 10.1046/j.1432-1327.1998.2560088.x
Brouwer, P., Van Der Werf, A., Schluepmann, H., Reichart, G.-J., and Nierop, K. G. J. (2015). Lipid yield and composition of Azolla filiculoides and the implications for biodiesel production. Bioenergy Res. 9, 369–377.
Brown, M. S., Radhakrishnan, A., and Goldstein, J. L. (2018). Retrospective on cholesterol homeostasis: the central role of scap. Annu. Rev. Biochem. 87, 783–807. doi: 10.1146/annurev-biochem-062917-011852
Brumfield, K. M., Laborde, S. M., and Moroney, J. V. (2017). A model for the ergosterol biosynthetic pathway in Chlamydomonas reinhardtii. Eur. J. Phycol. 52, 64–74.
Buckner, F. S., Nguyen, L. N., Joubert, B. M., and Matsuda, S. P. T. (2000). Cloning and heterologous expression of the Trypanosoma brucei lanosterol synthase gene. Mol. Biochem. Parasitol. 110, 399–403. doi: 10.1016/s0166-6851(00)00267-x
Burki, F., Roger, A. J., Brown, M. W., and Simpson, A. G. B. (2020). The new tree of eukaryotes. Trends Ecol. Evol. 35, 43–55.
Calegario, G., Pollier, J., Arendt, P., De Oliveira, L. S., Thompson, C., Soares, A. R., et al. (2016). Cloning and functional characterization of cycloartenol synthase from the red seaweed Laurencia dendroidea. PLoS One 11:e0165954. doi: 10.1371/journal.pone.0165954
Canals, O., Obiol, A., Muhovic, I., Vaque, D., and Massana, R. (2020). Ciliate diversity and distribution across horizontal and vertical scales in the open ocean. Mol. Ecol. 29, 2824–2839. doi: 10.1111/mec.15528
Carradec, Q., Pelletier, E., Da Silva, C., Alberti, A., Seeleuthner, Y., Blanc-Mathieu, R., et al. (2018). A global ocean atlas of eukaryotic genes. Nat. Commun. 9:373.
Chakrabarti, R. S., Ingham, S. A., Kozlitina, J., Gay, A., Cohen, J. C., Radhakrishnan, A., et al. (2017). Variability of cholesterol accessibility in human red blood cells measured using a bacterial cholesterol-binding toxin. eLife 6:e23355. doi: 10.7554/eLife.23355
Chaudhary, K., and Roos, D. S. (2005). Protozoan genomics for drug discovery. Nat. Biotechnol. 23, 1089–1091.
Chiu, P. L., Patterson, G. W., and Fenner, G. P. (1985). Sterols of bryophytes. Phytochemistry 24, 263–266.
Cock, J. M., Sterck, L., Rouze, P., Scornet, D., Allen, A. E., Amoutzias, G., et al. (2010). The Ectocarpus genome and the independent evolution of multicellularity in brown algae. Nature 465, 617–621.
Conner, R. L., Landrey, J. R., and Czarkowski, N. (1982). The effect of specific sterols on cell size and fatty acid composition ofTetrahymena pyriformisW. J. Protozool. 29, 105–109.
Conte, M., Lupette, J., Seddiki, K., Mei, C., Dolch, L. J., Gros, V., et al. (2018). Screening for biologically annotated drugs that trigger triacylglycerol accumulation in the diatom Phaeodactylum. Plant Physiol. 177, 532–552. doi: 10.1104/pp.17.01804
Corey, E. J., Matsuda, S. P., and Bartel, B. (1993). Isolation of an Arabidopsis thaliana gene encoding cycloartenol synthase by functional expression in a yeast mutant lacking lanosterol synthase by the use of a chromatographic screen. Proc. Natl. Acad. Sci. U.S.A. 90, 11628–11632. doi: 10.1073/pnas.90.24.11628
Cornejo-Castillo, F. M., and Zehr, J. P. (2019). Hopanoid lipids may facilitate aerobic nitrogen fixation in the ocean. Proc. Natl. Acad. Sci. U.S.A. 116, 18269–18271. doi: 10.1073/pnas.1908165116
Cornforth, J. W. (2002). Sterol biosynthesis: the early days. Biochem. Biophys. Res. Commun. 292, 1129–1138.
Cosentino, R. O., and Aguero, F. (2014). Genetic profiling of the isoprenoid and sterol biosynthesis pathway genes of Trypanosoma cruzi. PLoS One 9:e96762. doi: 10.1371/journal.pone.0096762
Cvejic, J. H., Putra, S. R., El-Beltagy, A., Hattori, R., Hattori, T., and Rohmer, M. (2000). Bacterial triterpenoids of the hopane series as biomarkers for the chemotaxonomy of Burkholderia. Pseudomonas and Ralstonia spp. FEMS Microbiol. Lett. 183, 295–299. doi: 10.1111/j.1574-6968.2000.tb08974.x
Darnet, S., Fliesler, S. J., and Schaller, H. (2020). Worming our way toward multiple evolutionary origins of convergent sterol pathways. J. Lipid Res. 61, 129–132. doi: 10.1194/jlr.C119000600
Darnet, S., and Rahier, A. (2004). Plant sterol biosynthesis: identification of two distinct families of sterol 4α-methyl oxidases. Biochem. J. 378, 889–898. doi: 10.1042/BJ20031572
Darnet, S., and Schaller, H. (2019). Metabolism and biological activities of 4-methyl-sterols. Molecules 24:451.
De Rosa, M., Gambacorta, A., Minale, L., and Bu’lock, J. D. (1971). Bacterial triterpenes. J. Chem. Soc. Chem. Commun. 1971, 619–620. doi: 10.1039/C29710000619
Delgado-Baquerizo, M., Oliverio, A. M., Brewer, T. E., Benavent-Gonzalez, A., Eldridge, D. J., Bardgett, R. D., et al. (2018). A global atlas of the dominant bacteria found in soil. Science 359, 320–325. doi: 10.1126/science.aap9516
Desmond, E., and Gribaldo, S. (2009). Phylogenomics of sterol synthesis: insights into the origin, evolution, and diversity of a key eukaryotic feature. Genome Biol. Evol. 1, 364–381. doi: 10.1093/gbe/evp036
Dittami, S. M., Corre, E., Brillet-Gueguen, L., Lipinska, A. P., Pontoizeau, N., Aite, M., et al. (2020). The genome of Ectocarpus subulatus - A highly stress-tolerant brown alga. Mar. Genomics 52:100740. doi: 10.1016/j.margen.2020.100740
El-Sayed, N. M., Myler, P. J., Blandin, G., Berriman, M., Crabtree, J., Aggarwal, G., et al. (2005). Comparative genomics of trypanosomatid parasitic protozoa. Science 309, 404–409.
Fabris, M., Matthijs, M., Carbonelle, S., Moses, T., Pollier, J., Dasseville, R., et al. (2014). Tracking the sterol biosynthesis pathway of the diatom Phaeodactylum tricornutum. New Phytol. 204, 521–535. doi: 10.1111/nph.12917
Fagundes, M. B., Vendruscolo, R. G., Maroneze, M. M., Barin, J. S., De Menezes, C. R., Zepka, L. Q., et al. (2018). Towards a sustainable route for the production of squalene using cyanobacteria. Waste Biomass Valori. 10, 1295–1302.
Forestier, E., Romero-Segura, C., Pateraki, I., Centeno, E., Compagnon, V., Preiss, M., et al. (2019). Distinct triterpene synthases in the laticifers of Euphorbia lathyris. Sci. Rep. 9:4840. doi: 10.1038/s41598-019-40905-y
Frada, M. J., Rosenwasser, S., Ben-Dor, S., Shemi, A., Sabanay, H., and Vardi, A. (2017). Morphological switch to a resistant subpopulation in response to viral infection in the bloom-forming coccolithophore Emiliania huxleyi. PLoS Pathog. 13:e1006775. doi: 10.1371/journal.ppat.1006775
Friggeri, L., Hargrove, T. Y., Rachakonda, G., Blobaum, A. L., Fisher, P., De Oliveira, G. M., et al. (2018). Sterol 14alpha-demethylase structure-based optimization of drug candidates for human infections with the protozoan Trypanosomatidae. J. Med. Chem. 61, 10910–10921. doi: 10.1021/acs.jmedchem.8b01671
Fügi, M. A., Gunasekera, K., Ochsenreiter, T., Guan, X., Wenk, M. R., and Maser, P. (2014). Genome profiling of sterol synthesis shows convergent evolution in parasites and guides chemotherapeutic attack. J. Lipid Res. 55, 929–938. doi: 10.1194/jlr.M048017
Fujita, S., Ohnishi, T., Watanabe, B., Yokota, T., Takatsuto, S., Fujioka, S., et al. (2006). Arabidopsis CYP90B1 catalyses the early C-22 hydroxylation of C27. C28 and C29 sterols. Plant J. 45, 765–774. doi: 10.1111/j.1365-313X.2005.02639.x
Gallo, C., D’ippolito, G., Nuzzo, G., Sardo, A., and Fontana, A. (2017). Autoinhibitory sterol sulfates mediate programmed cell death in a bloom-forming marine diatom. Nat. Commun. 8:1292. doi: 10.1038/s41467-017-01300-1
Gallo, C., Landi, S., D’ippolito, G., Nuzzo, G., Manzo, E., Sardo, A., et al. (2020). Diatoms synthesize sterols by inclusion of animal and fungal genes in the plant pathway. Sci. Rep. 10:4204. doi: 10.1038/s41598-020-60993-5
Gallo, C., Nuzzo, G., D’ippolito, G., Manzo, E., Sardo, A., and Fontana, A. (2018). Sterol sulfates and sulfotransferases in marine diatoms. Methods Enzymol. 605, 101–138.
Gas-Pascual, E., Simonovik, B., Schaller, H., and Bach, T. J. (2015). Inhibition of cycloartenol synthase (CAS) function in tobacco BY-2 cells. Lipids 50, 761–772.
Gealt, M. A., Adler, J. H., and Nes, W. R. (1981). The sterols and fatty acids from purified flagella of Chlamydomonas reinhardi. Lipids 16, 133–136.
Giner, J. L., and Djerassi, C. (1991). Biosynthetic studies of marine lipids. 33. Biosynthesis of dinosterol, peridinosterol and gorgosterol: unusual patterns of bioalkylation in dinoflagellate sterols. J. Org. Chem. 56, 2357–2363.
Giner, J.-L., Wünsche, L., Andersen, R. A., and Djerassi, C. (1991). Dinoflagellates cyclize squalene oxide to lanosterol. Biochem. Syst. Ecol. 19, 143–145.
Giner, J.-L., Zhao, H., Boyer, G. L., Satchwell, M. F., and Andersen, R. A. (2009). Sterol chemotaxonomy of marine pelagophyte algae. Chem. Biodivers. 6, 1111–1130. doi: 10.1002/cbdv.200800316
Goad, L. J., and Goodwin, T. W. (1972). “The biosynthesis of plant sterols,” in Progress in Phytochemistry, eds L. Reinhold and Y. Liwschitz (London: Interscience Publishers).
Godzina, S. M., Lovato, M. A., Meyer, M. M., Foster, K. A., Wilson, W. K., Gu, W., et al. (2000). Cloning and characterization of the Dictyostelium discoideum cycloartenol synthase cDNA. Lipids 35, 249–255. doi: 10.1007/s11745-000-0520-3
Gold, D. A., Caron, A., Fournier, G. P., and Summons, R. E. (2017). Paleoproterozoic sterol biosynthesis and the rise of oxygen. Nature 543, 420–423. doi: 10.1038/nature21412
Gold, D. A., Grabenstatter, J., De Mendoza, A., Riesgo, A., Ruiz-Trillo, I., and Summons, R. E. (2016). Sterol and genomic analyses validate the sponge biomarker hypothesis. Proc. Natl. Acad. Sci. U.S.A. 113, 2684–2689. doi: 10.1073/pnas.1512614113
Griffiths, W. J., and Wang, Y. (2019). Sterolomics in biology, biochemistry, medicine. Trends Analyt. Chem. 120:115280. doi: 10.1016/j.trac.2018.10.016
Guo, D.-A., Mangla, A. T., Zhou, W., Lopez, M., Jia, Z., Derrek Nichols, S., et al. (1997). “Antifungal sterol biosynthesis inhibitors,” in Subcellular Biochemistry, ed. D. B. Roodyn (New York, NY: Springer US).
Hambleton, E. A., Jones, V. A. S., Maegele, I., Kvaskoff, D., Sachsenheimer, T., and Guse, A. (2019). Sterol transfer by atypical cholesterol-binding NPC2 proteins in coral-algal symbiosis. eLife 8:e43923. doi: 10.7554/eLife.43923
Hannich, J. T., Entchev, E. V., Mende, F., Boytchev, H., Martin, R., Zagoriy, V., et al. (2009). Methylation of the sterol nucleus by STRM-1 regulates dauer larva formation in Caenorhabditis elegans. Dev. Cell 16, 833–843. doi: 10.1016/j.devcel.2009.04.012
Heilbron, I., Phipers, R. F., and Wright, H. R. (1934). The chemistry of the algae. Part I. The algal sterol fucosterol. J. Chem. Soc., 343, 1572–1576.
Heilbron, J. L., and Kevles, D. J. (1988). Finding a policy for mapping and sequencing the human genome: lessons from the history of particle physics. Minerva 26, 299–314. doi: 10.1007/BF01096399
Holland, H. D. (2002). Volcanic gases, black smokers, and the Great Oxidation Event. Geochim. Cosmochim. Acta 66, 3811–3826.
Holligan, P. M., Viollier, M., Harbour, D. S., Camus, P., and Champagne-Philippe, M. (1983). Satellite and ship studies of coccolithophore production along a continental-shelf edge. Nature 304, 339–342.
Husselstein, T., Schaller, H., Gachotte, D., and Benveniste, P. (1999). Delta7-sterol-C5-desaturase: molecular characterization and functional expression of wild-type and mutant alleles. Plant Mol. Biol. 39, 891–906. doi: 10.1023/a:1006113919172
Jaramillo-Madrid, A. C., Abbriano, R., Ashworth, J., Fabris, M., Pernice, M., and Ralph, P. J. (2020a). Overexpression of key sterol pathway enzymes in two model marine diatoms alters sterol profiles in Phaeodactylum tricornutum. Pharmaceuticals 13:481. doi: 10.3390/ph13120481
Jaramillo-Madrid, A. C., Ashworth, J., Fabris, M., and Ralph, P. J. (2020b). The unique sterol biosynthesis pathway of three model diatoms consists of a conserved core and diversified endpoints. Algal Res. 48:101902.
Jiao, C., Sorensen, I., Sun, X., Sun, H., Behar, H., Alseekh, S., et al. (2020). The Penium margaritaceum genome: hallmarks of the origins of land plants. Cell 181, 1097.e12–1111.e12. doi: 10.1016/j.cell.2020.04.019
Kaneko, T., Sato, S., Kotani, H., Tanaka, A., Asamizu, E., Nakamura, Y., et al. (1996). Sequence analysis of the genome of the unicellular cyanobacterium Synechocystis sp. strain PCC6803. II. Sequence determination of the entire genome and assignment of potential protein-coding regions. DNA Res. 3, 109–136.
Keeling, P. J., and Palmer, J. D. (2008). Horizontal gene transfer in eukaryotic evolution. Nat. Rev. Genet. 9, 605–618.
Kidambi, S., and Patel, S. B. (2008). Cholesterol and non-cholesterol sterol transporters: ABCG5, ABCG8 and NPC1L1: a review. Xenobiotica 38, 1119–1139. doi: 10.1080/00498250802007930
Knoch, E., Sugawara, S., Mori, T., Poulsen, C., Fukushima, A., Harholt, J., et al. (2018). Third DWF1 paralog in Solanaceae, sterol Delta(24)-isomerase, branches withanolide biosynthesis from the general phytosterol pathway. Proc. Natl. Acad. Sci. U.S.A. 115, E8096–E8103. doi: 10.1073/pnas.1807482115
Kolesnikova, M. D., Xiong, Q., Lodeiro, S., Hua, L., and Matsuda, S. P. (2006). Lanosterol biosynthesis in plants. Arch. Biochem. Biophys. 447, 87–95.
Komura, T., Wada, S., and Nagayama, H. (2014). The Identification of fucosterol in the marine brown AlgaeHizikia fusiformis. Agric. Biol. Chem. 38, 2275–2276.
Kumari, P., Kumar, M., Reddy, C. R. K., and Jha, B. (2013). “Algal lipids, fatty acids and sterols,” in Functional Ingredients From Algae for Foods and Nutraceuticals, ed. H. Domínguez (Amsterdam: Woodhead), 87–134.
Kushiro, T., and Ebizuka, Y. (2010). “Triterpenes,” in Comprehensive Natural Products II, eds L. Hung-Wen and L. Mander (Amsterdam: Elsevier Science), 673–708.
Lamacka, M., and Sajbidor, J. (1997). Ergosterol determination in Saccharomyces cerevisiae. Comparison of different methods. Biotechnol. Tech. 11, 723–725.
Lamb, D. C., Jackson, C. J., Warrilow, A. G., Manning, N. J., Kelly, D. E., and Kelly, S. L. (2007). Lanosterol biosynthesis in the prokaryote Methylococcus capsulatus: insight into the evolution of sterol biosynthesis. Mol. Biol. Evol. 24, 1714–1721. doi: 10.1093/molbev/msm090
Leblond, J. D., Timofte, H. I., Roche, S. A., and Porter, N. M. (2011). Sterols of glaucocystophytes. Phycol. Res. 59, 129–134. doi: 10.1111/j.1472-4669.2008.00167.x
Lecain, E., Chenivesse, X., Spagnoli, R., and Pompon, D. (1996). Cloning by metabolic interference in yeast and enzymatic characterization of Arabidopsis thaliana sterol Δ7-reductase. J. Biol. Chem. 271, 10866–10873. doi: 10.1074/jbc.271.18.10866
Lee, A. K., Banta, A. B., Wei, J. H., Kiemle, D. J., Feng, J., Giner, J. L., et al. (2018). C-4 sterol demethylation enzymes distinguish bacterial and eukaryotic sterol synthesis. Proc. Natl. Acad. Sci. U.S.A. 115, 5884–5889. doi: 10.1073/pnas.1802930115
Lepesheva, G. I., Friggeri, L., and Waterman, M. R. (2018). CYP51 as drug targets for fungi and protozoan parasites: past, present and future. Parasitology 145, 1820–1836. doi: 10.1017/S0031182018000562
Lepesheva, G. I., Nes, W. D., Zhou, W., Hill, G. C., and Waterman, M. R. (2004). CYP51 from Trypanosoma brucei is obtusifoliol-specific. Biochemistry 43, 10789–10799. doi: 10.1021/bi048967t
Li, F. W., Brouwer, P., Carretero-Paulet, L., Cheng, S., De Vries, J., Delaux, P. M., et al. (2018). Fern genomes elucidate land plant evolution and cyanobacterial symbioses. Nat. Plants 4, 460–472. doi: 10.1038/s41477-018-0188-8
Li, J., Nagpal, P., Vitart, V., Mcmorris, T. C., and Chory, J. (1996). A role for brassinosteroids in light-dependent development of Arabidopsis. Science 272, 398–401. doi: 10.1126/science.272.5260.398
Lombard, J., and Moreira, D. (2011). Origins and early evolution of the mevalonate pathway of isoprenoid biosynthesis in the three domains of life. Mol. Biol. Evol. 28, 87–99.
Lommer, M., Specht, M., Roy, A. S., Kraemer, L., Andreson, R., Gutowska, M. A., et al. (2012). Genome and low-iron response of an oceanic diatom adapted to chronic iron limitation. Genome Biol. 13:R66.
Lovato, M. A., Hart, E. A., Segura, M. J., Giner, J. L., and Matsuda, S. P. (2000). Functional cloning of an Arabidopsis thaliana cDNA encoding cycloeucalenol cycloisomerase. J. Biol. Chem. 275, 13394–13397. doi: 10.1074/jbc.275.18.13394
Lu, Y., Jiang, J., Zhao, H., Han, X., Xiang, Y., and Zhou, W. (2020). Clade-specific sterol metabolites in dinoflagellate endosymbionts are associated with coral bleaching in response to environmental cues. mSystems 5:e00765-20. doi: 10.1128/mSystems.00765-20
Lu, Y., Zhou, W., Wei, L., Li, J., Jia, J., Li, F., et al. (2014). Regulation of the cholesterol biosynthetic pathway and its integration with fatty acid biosynthesis in the oleaginous microalga Nannochloropsis oceanica. Biotechnol. Biofuels 7:81. doi: 10.1186/1754-6834-7-81
Luo, X., Su, P., and Zhang, W. (2015). Advances in microalgae-derived phytosterols for functional food and pharmaceutical applications. Mar. Drugs 13, 4231–4254. doi: 10.3390/md13074231
Lupette, J., Jaussaud, A., Seddiki, K., Morabito, C., Brugiere, S., Schaller, H., et al. (2019). The architecture of lipid droplets in the diatom Phaeodactylum tricornutum. Algal Res. Biomass Biofuels Bioprod. 38:101415.
Mackenzie, A. S., Brassell, S. C., Eglinton, G., and Maxwell, J. R. (1982). Chemical fossils: the geological fate of steroids. Science 217, 491–504. doi: 10.1126/science.217.4559.491
Mangiarotti, A., Genovese, D. M., Naumann, C. A., Monti, M. R., and Wilke, N. (2019). Hopanoids, like sterols, modulate dynamics, compaction, phase segregation and permeability of membranes. Biochim. Biophys. Acta Biomembr. 1861:183060. doi: 10.1016/j.bbamem.2019.183060
Maniet, G., Jacquet, N., and Richel, A. (2019). Recovery of sterols from vegetable oil distillate by enzymatic and non-enzymatic processes. Compt. Ren. Chim. 22, 347–353.
Marechal, E., and Lupette, J. (2019). Relationship between acyl-lipid and sterol metabolisms in diatoms. Biochimie 169, 3–11. doi: 10.1016/j.biochi.2019.07.005
Marsan, M.-P., Muller, I., and Milon, A. (1996). Ability of clionasterol and poriferasterol (24-epimers of sitosterol and stigmasterol) to regulate membrane lipid dynamics. Chem. Phys. Lipids 84, 117–121.
Martin-Creuzburg, D., and Elert, E. V. (2009). “Ecological significance of sterols in aquatic food webs,” in Lipids in Aquatic Ecosystems, eds M. T. Arts, M. T. Brett, and M. Kainz (Berlin: Springer Science & Business Media), 43–64.
Martin-Creuzburg, D., and Merkel, P. (2016). Sterols of freshwater microalgae: potential implications for zooplankton nutrition. J. Plankton Res. 38, 865–877.
McKean, M. L., and Nes, W. R. (1977). Evidence for separate intermediates in the biosynthesis of 24α- and 24β-alkylsterols in tracheophytes. Phytochemistry 16, 683–686.
Men, S., Boutte, Y., Ikeda, Y., Li, X., Palme, K., Stierhof, Y. D., et al. (2008). Sterol-dependent endocytosis mediates post-cytokinetic acquisition of PIN2 auxin efflux carrier polarity. Nat. Cell Biol. 10, 237–244. doi: 10.1038/ncb1686
Mercer, E. I., London, R. A., Kent, I. S. A., and Taylor, A. J. (1974). Sterols, sterol esters and fatty acids of Botrydium granulatum, Tribonema aequale and Monodus subterraneus. Phytochemistry 13, 845–852. doi: 10.1016/S0031-9422(00)91149-X
Meyer, M. M., Xu, R., and Matsuda, S. P. T. (2002). Directed evolution to generate cycloartenol synthase mutants that produce lanosterol. Org. Lett. 4, 1395–1398.
Mikami, K., Ito, M., Taya, K., Kishimoto, I., Kobayashi, T., Itabashi, Y., et al. (2018). Parthenosporophytes of the brown alga Ectocarpus siliculosus exhibit sex-dependent differences in thermotolerance as well as fatty acid and sterol composition. Mar. Environ. Res. 137, 188–195. doi: 10.1016/j.marenvres.2018.02.003
Miller, M. B., Haubrich, B. A., Wang, Q., Snell, W. J., and Nes, W. D. (2012). Evolutionarily conserved Delta(25(27))-olefin ergosterol biosynthesis pathway in the alga Chlamydomonas reinhardtii. J. Lipid Res. 53, 1636–1645. doi: 10.1194/jlr.M027482
Mitova, M. I., Usov, A. I., Bilan, M. I., Stefanov, K. L., Dimitrova-Konaklieva, S. D., Tonov, D. P., et al. (1999). Sterols and polysaccharides in freshwater algae Spirogyra and Mougeotia. Z. Naturforsch. C 54, 1016–1020.
Moreau, R. A., Nystrom, L., Whitaker, B. D., Winkler-Moser, J. K., Baer, D. J., Gebauer, S. K., et al. (2018). Phytosterols and their derivatives: structural diversity, distribution, metabolism, analysis, and health-promoting uses. Prog. Lipid Res. 70, 35–61. doi: 10.1016/j.plipres.2018.04.001
Morikawa, T., Mizutani, M., Aoki, N., Watanabe, B., Saga, H., Saito, S., et al. (2006). Cytochrome P450 CYP710A encodes the sterol C-22 desaturase in Arabidopsis and tomato. Plant Cell 18, 1008–1022. doi: 10.1105/tpc.105.036012
Morikawa, T., Saga, H., Hashizume, H., and Ohta, D. (2009). CYP710A genes encoding sterol C22-desaturase in Physcomitrella patens as molecular evidence for the evolutionary conservation of a sterol biosynthetic pathway in plants. Planta 229, 1311–1322. doi: 10.1007/s00425-009-0916-4
Moseley, K. R., and Thompson, G. A. (1980). Lipid composition and metabolism of Volvox carteri. Plant Physiol. 65, 260–265.
Moss, G. P. (1989). Nomenclature of steroids (Recommendations 1989). Pure Appl. Chem. 61, 1783–1822.
Najle, S. R., Hernandez, J., Ocana-Pallares, E., Garcia Siburu, N., Nusblat, A. D., Nudel, C. B., et al. (2020). Genome-wide transcriptional analysis of Tetrahymena thermophila response to exogenous cholesterol. J. Eukaryot. Microbiol. 67, 209–222. doi: 10.1111/jeu.12774
Najle, S. R., Nusblat, A. D., Nudel, C. B., and Uttaro, A. D. (2013). The Sterol-C7 desaturase from the ciliate Tetrahymena thermophila is a Rieske Oxygenase, which is highly conserved in animals. Mol. Biol. Evol. 30, 1630–1643. doi: 10.1093/molbev/mst076
Nakamoto, M., Schmit, A. C., Heintz, D., Schaller, H., and Ohta, D. (2015). Diversification of sterol methyltransferase enzymes in plants and a role for beta-sitosterol in oriented cell plate formation and polarized growth. Plant J. 84, 860–874. doi: 10.1111/tpj.13043
Nes, C. R., Singha, U. K., Liu, J., Ganapathy, K., Villalta, F., Waterman, M. R., et al. (2012). Novel sterol metabolic network of Trypanosoma brucei procyclic and bloodstream forms. Biochem. J. 443, 267–277. doi: 10.1042/BJ20111849
Newton, M. S., Arcus, V. L., Gerth, M. L., and Patrick, W. M. (2018). Enzyme evolution: innovation is easy, optimization is complicated. Curr. Opin. Struct. Biol. 48, 110–116.
Nguyen, H. M., Baudet, M., Cuine, S., Adriano, J. M., Barthe, D., Billon, E., et al. (2011). Proteomic profiling of oil bodies isolated from the unicellular green microalga Chlamydomonas reinhardtii: with focus on proteins involved in lipid metabolism. Proteomics 11, 4266–4273. doi: 10.1002/pmic.201100114
Nusblat, A. D., Munoz, L., Valcarce, G. A., and Nudel, C. B. (2005). Characterization and properties of cholesterol desaturases from the ciliate Tetrahymena thermophila. J. Eukaryot. Microbiol. 52, 61–67. doi: 10.1111/j.1550-7408.2005.3279rr.x
Nuzzo, G., Gallo, C., D’ippolito, G., Manzo, E., Ruocco, N., Russo, E., et al. (2018). UPLC(-)MS/MS identification of sterol sulfates in marine diatoms. Mar. Drugs 17:E10. doi: 10.3390/md17010010
Ohyama, K., Suzuki, M., Kikuchi, J., Saito, K., and Muranaka, T. (2009). Dual biosynthetic pathways to phytosterol via cycloartenol and lanosterol in Arabidopsis. Proc. Natl. Acad. Sci. U.S.A. 106, 725–730. doi: 10.1073/pnas.0807675106
Ourisson, G., Albrecht, P., and Rohmer, M. (1982). Predictive microbial biochemistry — from molecular fossils to procaryotic membranes. Trends Biochem. Sci 7, 236–239.
Ourisson, G., and Nakatani, Y. (1994). The terpenoid theory of the origin of cellular life: the evolution of terpenoids to cholesterol. Chem. Biol. 1, 11–23.
Patterson, G. W. (1974). Sterols of some green algae. Comp. Biochem. Physiol. Part B Comp. Biochem. 47, 453–457.
Patterson, G. W., and Nes, W. D. (1991). Physiology and Biochemistry of Sterols. Champaign: American Oil Chemists’ Society.
Pearson, A., Flood Page, S. R., Jorgenson, T. L., Fischer, W. W., and Higgins, M. B. (2007). Novel hopanoid cyclases from the environment. Environ. Microbiol. 9, 2175–2188.
Pereira, A. L., and Vasconcelos, V. (2014). Classification and phylogeny of the cyanobiont Anabaena azollae strasburger: an answered question? Int. J. Syst. Evol. Microbiol. 64, 1830–1840. doi: 10.1099/ijs.0.059238-0
Piretti, M. V., Pagliuca, G., Boni, L., Pistocchi, R., Diamante, M., and Gazzotti, T. (1997). Investigation of 4-methyl sterols from cultured dinoflagellate algal strains. J. Phycol. 33, 61–67.
Poklepovich, T. J., Rinaldi, M. A., Tomazic, M. L., Favale, N. O., Turkewitz, A. P., Nudel, C. B., et al. (2012). The cytochrome b5 dependent C-5(6) sterol desaturase DES5A from the endoplasmic reticulum of Tetrahymena thermophila complements ergosterol biosynthesis mutants in Saccharomyces cerevisiae. Steroids 77, 1313–1320. doi: 10.1016/j.steroids.2012.08.015
Pollier, J., Vancaester, E., Kuzhiumparambil, U., Vickers, C. E., Vandepoele, K., Goossens, A., et al. (2019). A widespread alternative squalene epoxidase participates in eukaryote steroid biosynthesis. Nat. Microbiol. 4, 226–233. doi: 10.1038/s41564-018-0305-5
Raederstorff, D., and Rohmer, M. (1987). Sterol biosynthesis via cycloartenol and other biochemical features related to photosynthetic phyla in the amoeba Naegleria lovaniensis and Naegleria gruberi. Eur. J. Biochem. 164, 427–434. doi: 10.1111/j.1432-1033.1987.tb11075.x
Raederstorff, D., and Rohmer, M. (1988). Polyterpenoids as cholesterol and tetrahymanol surrogates in the ciliate Tetrahymena-pyriformis. Biochim. Biophys. Acta 960, 190–199. doi: 10.1016/0005-2760(88)90064-1
Rampen, S. W., Abbas, B. A., Schouten, S., and Sinninghe Damste, J. S. (2010). A comprehensive study of sterols in marine diatoms (Bacillariophyta): implications for their use as tracers for diatom productivity. Limnol. Oceanogr. 55, 91–105.
Rivero-Calle, S., Gnanadesikan, A., Del Castillo, C. E., Balch, W. M., and Guikema, S. D. (2015). Multidecadal increase in North Atlantic coccolithophores and the potential role of rising CO(2). Science 350, 1533–1537. doi: 10.1126/science.aaa8026
Roberts, C. W., Mcleod, R., Rice, D. W., Ginger, M., Chance, M. L., and Goad, L. J. (2003). Fatty acid and sterol metabolism: potential antimicrobial targets in apicomplexan and trypanosomatid parasitic protozoa. Mol. Biochem. Parasitol. 126, 129–142. doi: 10.1016/s0166-6851(02)00280-3
Rohmer, M. (1999). The discovery of a mevalonate-independent pathway for isoprenoid biosynthesis in bacteria, algae and higher plants. Nat. Prod. Rep. 16, 565–574. doi: 10.1039/a709175c
Rohmer, M., Bouvier, P., and Ourisson, G. (1979). Molecular evolution of biomembranes: structural equivalents and phylogenetic precursors of sterols. Proc. Natl. Acad. Sci. U.S.A. 76, 847–851. doi: 10.1073/pnas.76.2.847
Rosenwasser, S., Mausz, M. A., Schatz, D., Sheyn, U., Malitsky, S., Aharoni, A., et al. (2014). Rewiring host lipid metabolism by large viruses determines the fate of Emiliania huxleyi, a bloom-forming alga in the ocean. Plant Cell 26, 2689–2707. doi: 10.1105/tpc.114.125641
Rubinstein, I., Goad, L. J., Clague, A. D. H., and Mulheirn, L. J. (1976). The 220 MHz NMR spectra of phytosterols. Phytochemistry 15, 195–200.
Saar, J., Kader, J.-C., Poralla, K., and Ourisson, G. (1991). Purification and some properties of the squalene-tetrahymanol cyclase from Tetrahymena thermophila. Biochim. Biophys. Acta Gen. Sub. 1075, 93–101. doi: 10.1016/0304-4165(91)90080-z
Saenz, J. P., Sezgin, E., Schwille, P., and Simons, K. (2012). Functional convergence of hopanoids and sterols in membrane ordering. Proc. Natl. Acad. Sci. U.S.A. 109, 14236–14240.
Salvador-Castell, M., Tourte, M., and Oger, P. M. (2019). In search for the membrane regulators of Archaea. Int. J. Mol. Sci. 20:4434. doi: 10.3390/ijms20184434
Sawai, S., Ohyama, K., Yasumoto, S., Seki, H., Sakuma, T., Yamamoto, T., et al. (2014). Sterol side chain reductase 2 is a key enzyme in the biosynthesis of cholesterol, the common precursor of toxic steroidal glycoalkaloids in potato. Plant Cell 26, 3763–3774. doi: 10.1105/tpc.114.130096
Schaeffer, A., Bouvier-Navé, P., Benveniste, P., and Schaller H. (2000). Plant sterol-C24-methyl transferases: different profiles of tobacco transformed with SMT1 or SMT2. Lipids 35, 263–269. doi: 10.1007/s11745-000-0522-1
Schaeffer, A., Bronner, R., Benveniste, P., and Schaller, H. (2001). The ratio of campesterol to sitosterol that modulates growth in Arabidopsis is controlled by STEROL METHYLTRANSFERASE 2;1. Plant J. 25, 605–615. doi: 10.1046/j.1365-313x.2001.00994.x
Schaller, H. (2010). “Sterol and steroid biosynthesis and metabolism in plants and microorganisms,” in Comprehensive Natural Products II, eds L. Hung-Wen and L. Mander (Amsterdam: Elsevier Science), 755–787.
Schaller, H., Bouvier-Navé, P., and Benveniste, P. (1998). Overexpression of an Arabidopsis cDNA encoding a Sterol-C241-methyltransferase in tobacco modifies the ratio of 24-Methyl cholesterol to sitosterol and is associated with growth reduction. Plant Physiol. 118, 461–469. doi: 10.1104/pp.118.2.461
Schonknecht, G., Chen, W. H., Ternes, C. M., Barbier, G. G., Shrestha, R. P., Stanke, M., et al. (2013). Gene transfer from bacteria and Archaea facilitated evolution of an extremophilic eukaryote. Science 339, 1207–1210. doi: 10.1126/science.1231707
Seckbach, J., Ikan, R., Ringelberg, D., and White, D. (1993). Sterols and phylogeny of the acidophilic hot springs algae Cyanidium caldarium and Galdieria sulphuraria. Phytochemistry 34, 1345–1349.
Shimizu, Y., Alam, M., and Kobayashi, A. (1976). Letter: dinosterol, the major sterol with a unique side chain ion the toxic dinoflagellate, Gonyaulax tamarensis. J. Am. Chem. Soc. 98, 1059–1060. doi: 10.1021/ja00420a054
Silipo, A., Vitiello, G., Gully, D., Sturiale, L., Chaintreuil, C., Fardoux, J., et al. (2014). Covalently linked hopanoid-lipid A improves outer-membrane resistance of a Bradyrhizobium symbiont of legumes. Nat. Commun. 5:5106. doi: 10.1038/ncomms6106
Singh, R., Parihar, P., Singh, M., Bajguz, A., Kumar, J., Singh, S., et al. (2017). Uncovering potential applications of cyanobacteria and algal metabolites in biology, agriculture and medicine: current status and future prospects. Front. Microbiol. 8:515. doi: 10.3389/fmicb.2017.00515
Sonawane, P. D., Pollier, J., Panda, S., Szymanski, J., Massalha, H., Yona, M., et al. (2016). Plant cholesterol biosynthetic pathway overlaps with phytosterol metabolism. Nat. Plants 3:16205.
Sperling, P., Ternes, P., Zank, T. K., and Heinz, E. (2003). The evolution of desaturases. Prostaglandins Leukot. Essent. Fatty Acids 68, 73–95.
Strauss, J. F. III, Liu, P., Christenson, L. K., and Watari, H. (2002). Sterols and intracellular vesicular trafficking: lessons from the study of NPC1. Steroids 67, 947–951. doi: 10.1016/s0039-128x(02)00042-9
Summons, R. E., Bradley, A. S., Jahnke, L. L., and Waldbauer, J. R. (2006). Steroids, triterpenoids and molecular oxygen. Philos. Trans. R. Soc. Lond. B. Biol. Sci. 361, 951–968.
Suzuki, M., Xiang, T., Ohyama, K., Seki, H., Saito, K., Muranaka, T., et al. (2006). Lanosterol synthase in dicotyledonous plants. Plant Cell Physiol. 47, 565–571.
Taipale, S. J., Hiltunen, M., Vuorio, K., and Peltomaa, E. (2016). Suitability of phytosterols alongside fatty acids as chemotaxonomic biomarkers for phytoplankton. Front. Plant Sci. 7:212. doi: 10.3389/fpls.2016.00212
Takishita, K., Takaki, Y., Chikaraishi, Y., Ikuta, T., Ozawa, G., Yoshida, T., et al. (2017). Genomic evidence that methanotrophic endosymbionts likely provide deep-sea bathymodiolus mussels with a sterol intermediate in cholesterol biosynthesis. Genome Biol. Evol. 9, 1148–1160. doi: 10.1093/gbe/evx082
Tasende, M. G. (2000). Fatty acid and sterol composition of gametophytes and sporophytes of Chondrus crispus (Gigartinaceae, Rhodophyta). Sci. Mar. 64, 421–426.
Tomazic, M. L., Najle, S. R., Nusblat, A. D., Uttaro, A. D., and Nudel, C. B. (2011). A novel sterol desaturase-like protein promoting dealkylation of phytosterols in Tetrahymena thermophila. Eukaryot. Cell 10, 423–434. doi: 10.1128/EC.00259-10
Tomazic, M. L., Poklepovich, T. J., Nudel, C. B., and Nusblat, A. D. (2014). Incomplete sterols and hopanoids pathways in ciliates: gene loss and acquisition during evolution as a source of biosynthetic genes. Mol. Phylogenet. Evol. 74, 122–134. doi: 10.1016/j.ympev.2014.01.026
Tsuda, K., Akagi, S., and Kishida, Y. (1957). Discovery of cholesterol in some red algae. Science 126, 927–928. doi: 10.1126/science.126.3279.927
Urbina, J. A., Concepcion, J. L., Rangel, S., Visbal, G., and Lira, R. (2002). Squalene synthase as a chemotherapeutic target in Trypanosoma cruzi and Leishmania mexicana. Mol. Biochem. Parasitol. 125, 35–45. doi: 10.1016/s0166-6851(02)00206-2
Veron, B., Billard, C., Dauguet, J. C., and Hartmann, M. A. (1996). Sterol composition of Phaeodactylum tricornutum as influenced by growth temperature and light spectral quality. Lipids 31, 989–994. doi: 10.1007/BF02522694
Volkman, J. K. (2016). “Sterols in microalgae,” in The Physiology of Microalgae, eds M. Borowitzka, J. Beardall, and J. Raven (Berlin: Springer), 485–505.
Volkman, J. K., Smith, D. J., Eglinton, G., Forsberg, T. E. V., and Corner, E. D. S. (1981). Sterol and fatty acid composition of four marine haptophycean algae. J. Mar. Biol. Assoc. U. K. 61, 509–527.
Wang, S., Li, L., Li, H., Sahu, S. K., Wang, H., Xu, Y., et al. (2020). Genomes of early-diverging streptophyte algae shed light on plant terrestrialization. Nat. Plants 6, 95–106. doi: 10.1038/s41477-019-0560-3
Wei, J. H., Yin, X., and Welander, P. V. (2016). Sterol synthesis in diverse bacteria. Front. Microbiol. 7:990. doi: 10.3389/fmicb.2016.00990
Weng, J. K., and Noel, J. P. (2013). Chemodiversity in Selaginella: a reference system for parallel and convergent metabolic evolution in terrestrial plants. Front. Plant Sci. 4:119. doi: 10.3389/fpls.2013.00119
Wiersma, S. J., Mooiman, C., Giera, M., and Pronk, J. T. (2020). Squalene-tetrahymanol cyclase expression enables sterol-independent growth of Saccharomyces cerevisiae. Appl. Environ. Microbiol. 86:e00672-20. doi: 10.1128/AEM.00672-20
Xu, R., Fazio, G. C., and Matsuda, S. P. (2004). On the origins of triterpenoid skeletal diversity. Phytochemistry 65, 261–291. doi: 10.1016/j.phytochem.2003.11.014
Yakobov, N., Fischer, F., Mahmoudi, N., Saga, Y., Grube, C. D., Roy, H., et al. (2020). RNA-dependent sterol aspartylation in fungi. Proc. Natl. Acad. Sci. U.S.A. 117, 14948–14957. doi: 10.1073/pnas.2003266117
Ye, N., Zhang, X., Miao, M., Fan, X., Zheng, Y., Xu, D., et al. (2015). Saccharina genomes provide novel insight into kelp biology. Nat. Commun. 6:6986. doi: 10.1038/ncomms7986
Zhang, X., Lin, K., and Li, Y. (2020). Highlights to phytosterols accumulation and equilibrium in plants: biosynthetic pathway and feedback regulation. Plant Physiol. Biochem. 155, 637–649. doi: 10.1016/j.plaphy.2020.08.021
Zhou, W., Debnath, A., Jennings, G., Hahn, H. J., Vanderloop, B. H., Chaudhuri, M., et al. (2018). Enzymatic chokepoints and synergistic drug targets in the sterol biosynthesis pathway of Naegleria fowleri. PLoS Pathog. 14:e1007245. doi: 10.1371/journal.ppat.1007245
Keywords: phytosterol, cholesterol, algae, plant, eukaryote, prokaryote
Citation: Darnet S, Blary A, Chevalier Q and Schaller H (2021) Phytosterol Profiles, Genomes and Enzymes – An Overview. Front. Plant Sci. 12:665206. doi: 10.3389/fpls.2021.665206
Received: 07 February 2021; Accepted: 20 April 2021;
Published: 19 May 2021.
Edited by:
Eric Marechal, UMR 5168 Laboratoire de Physiologie Cellulaire Vegetale (LPCV), FranceReviewed by:
Angelo Fontana, Istituto di Chimica Biomolecolare, Consiglio Nazionale delle Ricerche (CNR), ItalyCopyright © 2021 Darnet, Blary, Chevalier and Schaller. This is an open-access article distributed under the terms of the Creative Commons Attribution License (CC BY). The use, distribution or reproduction in other forums is permitted, provided the original author(s) and the copyright owner(s) are credited and that the original publication in this journal is cited, in accordance with accepted academic practice. No use, distribution or reproduction is permitted which does not comply with these terms.
*Correspondence: Hubert Schaller, aHViZXJ0LnNjaGFsbGVyQGlibXAtY25ycy51bmlzdHJhLmZy
Disclaimer: All claims expressed in this article are solely those of the authors and do not necessarily represent those of their affiliated organizations, or those of the publisher, the editors and the reviewers. Any product that may be evaluated in this article or claim that may be made by its manufacturer is not guaranteed or endorsed by the publisher.
Research integrity at Frontiers
Learn more about the work of our research integrity team to safeguard the quality of each article we publish.